- School of Metallurgy and Environment, Central South University, Changsha, China
Nickel-cobalt sulfides (NixCoyS4) are promising supercapacitor materials due to their high capacitance, while the sluggish kinetics in terms of charge transfer limits their energy density. To achieve both high energy and power density for the NixCoyS4–based supercapacitor, sulfur-doped reduced graphene oxide (rGO) is incorporated into NixCoyS4 by an in situ growth—ion exchange strategy to synthesize NixCoyS4/rGO composites. Profited from the synergetic effect between rGO and NixCoyS4, the Ni1.64Co2.40S4/rGO electrode delivers high specific capacitance of 1,089 F g−1 at 1 A g−1, and remains 92.6% of its original capacitance at 20 A g−1 (1,008 F g−1). Asymmetric supercapacitors assembled with active carbon (AC) and Ni1.64Co2.40S4/rGO (Ni1.64Co2.40S4/rGO//AC) offer both high specific capacitance (265.5 F g−1 at 1 A g−1) and superior rate capability at 50 A g−1 (recovering 63.6% of the capacitance determined at 1 A g−1). In addition, the assembled device exhibits a high capacitance retention of 92.6% after 10,000 cycles at 10 A g−1, which implies an excellent cyclic stability. Ragone plot reveals that the energy density of Ni1.64Co2.40S4/rGO//AC asymmetric supercapacitor do not vanish as it delivers 30.4 Wh kg−1 at 10 kW kg−1, demonstrating its promising application.
Introduction
With gradual consumption of fuel energy and the growing environmental problems, is the need to develop renewable energies and corresponding energy storage and conversion devices is urgent (Zhong et al., 2012; Liu et al., 2018a; Zhang Q. et al., 2018). Lithium ion battery (Li et al., 2015; Liu et al., 2016; Zhong et al., 2017; Wang J. et al., 2018), sodium-ion battery (Chen J. et al., 2018; Yan et al., 2018), Lithium-Sulfur battery (Tan et al., 2018; Wu F. et al., 2018), and supercapacitor (Gilshteyn et al., 2017; Saha et al., 2018) are representative electrochemical energy storage devices. A supercapacitor (SC) is one of the most promising energy storage devices due to their desirable characteristics, such as high power-density, super-long cycle life and high safety performance, and it plays an important role in both automobile and electronic industry (Simon et al., 2014; Eftekhari et al., 2017; Zhang L. et al., 2018). Classified by different energy storage mechanisms, electrical double layer capacitors (EDLCs), and pseudocapacitors (PCs) show differentiated advantages in the specific field. Although EDLCs deliver fast electron transfer, their bottleneck lies in the low specific capacitance problem. On the contrary, PCs deliver satisfying specific capacitance, but poor charge transfer ability limits their application. Neither of them can be used to build a capacitor that possesses both high energy density and power density. Developing a hybrid capacitor combining with the advantages of EDLCs and PCs is an effective strategy to remedy the deficiency.
To fulfill the above design, developing advanced materials with both high capacitance and rate capability are the key factors. Normally, the composite formed with proper composition of two materials results in complementary advantages. As known to all, reduced graphene oxide (rGO) is one of the most promising electrode materials for EDLCs due to its large specific surface area, exceptionally high electronic conductivity and high theoretical specific capacitance (Booth et al., 2008; Lee et al., 2008; Ke and Wang, 2016). When rGO is introduced into the composite properly, it enhances not only the electronic conductivity of composite, but also the structural stability of the electrode (Ke and Wang, 2016; Deng et al., 2017). Therefore, taking reduced graphene oxide as a framework is an effective way to reinforce the mechanical strengths of the composites. Nickel-cobalt sulfide is one of the most promising one among various pseudocapacitor electrode materials, such as metal oxides, metal sulfides, and conductive polymers (Jinlong et al., 2017; Wen et al., 2017; Zhao et al., 2017; Liang et al., 2018). Although, there is some doubt about the definition of pseudocapacitance (Brousse, 2015; Jiang and Liu, 2019), many studies reported that transition metal sulfides were classified as one kind of pseudocapacitive materials (Xiong et al., 2015; Wang et al., 2019). However, some studies classify transition metal sulfides as battery-type electrode materials (Mohamed et al., 2018; Ma et al., 2019). Putting this controversial issue aside, it has been proven that nickel-cobalt sulfides (NixCoyS4) exhibit high electronic conductivity and flexibility due to the lower optical band gap of Ni–Co and electronegativity of sulfur (Chen et al., 2013; Zhu et al., 2015). Great efforts have been devoted to the fabrication of composites, such as NiCo2S4/rGO (Annamalai et al., 2017; Nan et al., 2018; Sarkar et al., 2018; Wang Y. et al., 2018), CoNi2S4/rGO (Gao et al., 2018), NiS/rGO (Qu et al., 2018), C-NiCo2S4 (Yuan et al., 2017; Mohamed et al., 2018), CNTs-NiCo2S4 (Li D. et al., 2016), and so on. However, the influence of Ni/Co ratio of nickel-cobalt sulfide materials on the characteristics and the pseudocapacitance performances are not systematically studied.
In this work, we prepare a series of NixCoyS4/rGO composites with various Ni/Co ratios in the raw materials (Ni/Co = 2.0, 1.0, 0.5, and 0.25), in which the bimetallic Ni-Co hydroxy-carbonates in-situ grow onto the rGO framework during the synthesis process. We demonstrate that asymmetric supercapacitor (Ni1.64Co2.40S4/rGO//AC) assembled with Ni1.64Co2.40S4/rGO composite (positive electrode) and active carbon (negative electrode) exhibits excellent electrochemical performance with outstanding capacitance retention of 92.6% after 10,000 cycles at high current density of 10 A g−1 and high energy density of 30.4 Wh kg−1 at the power density of 10 kW kg−1. Our results offer new insights into developing advanced electrode materials for supercapacitors with high energy and power density.
Experimental
The graphene oxide (GO) was purchased from Suzhou Tanfeng technology co LTD, and all of other chemical reagents were provided by Sinopharm Chemical Reagent Corp in an analytical grade that were used as-received without further purification.
Synthesis of NixCoyS4
In a typical synthesis of precursors, 0.025 g GO was dissolved into 100 mL deionized water to form homogeneous brown solution by ultrasonic dispersion. A certain mass of NiCl2·6H2O, CoCl2·6H2O (molar ratios of Ni: Co are 2:1, 1:1; 1:2, and 1:4) and 1.6365 g urea were dissolved into the GO solution under stirring. After stirring for 30 min, the obtained suspension was transferred into a 200 mL Teflon-lined autoclave. The hydrothermal reaction was carried out at 120°C for 10 h so that the bimetallic Ni-Co hydroxy-carbonates in-situ grow onto the GO framework. The products were washed with deionized water and collected through filtration, and then which was lyophilized. Based on the Ni/Co ratio in the raw materials, the as-obtained precursors were labeled as NC2010, NC1515, NC1020, and NC0624, respectively.
For synthesizing the NixCoyS4/rGO by sulfurizing the aforementioned precursors, the representative processes are described as followed. First, 0.2 g precursor was dispersed into 100 mL deionized water and the mixture was stirred for 30 min. Subsequently, 1.7156 g Na2S·9H2O was added into the suspension and stirred for 30 min. Then, the mixture was transferred into a 200 mL Teflon-lined autoclave, which was heated at 180°C for 8 h. After cooling naturally to room temperature, the black product was collected in the same way as former procedure. To further illuminate the content of Ni, Co and S in the NixCoyS4/rGO composites, the Inductively Coupled Plasma-Optical Emission Spectrometry (ICP-OES) results and the C-S analysis results of NixCoyS4/rGO composites are shown in Table S1. The as-prepared products were labeled as Ni2.15Co1.37S4/rGO, Ni1.64Co2.40S4/rGO, Ni1.02Co2.98S4/rGO, Ni0.60Co3.60S4/rGO on the basis of ICP and C-S analysis results.
Materials Characterization
Structure, morphology, and microstructure of the precursors and the corresponding NixCoyS4/rGO composites were characterized by X-ray diffraction using Cu Kα (λ = 1.5406 Å) radiation (XRD, Bruker D8 AdvanceRint-2000), scanning electron microscopy (SEM, Sirion 200), and transmission electron microscopy (TEM, Tecnai G12, 200 kV). The Surface elemental valence of the sample was characterized by X-ray photoelectron spectroscopy (XPS, PHI5600, PerkineElmer).
Electrochemical Measurement
Circular Ni foams were cleaned in 1.0 mol L−1 KOH solution with ultrasonic cleaning for 30 min to remove impurities on the surface, and washed with deionized water three times. The preparation of electrodes was followed in the same procedure as described in previous work (Dong et al., 2018), and the diameter of Ni foam for positive and negative electrode is 12 and 16 mm, respectively.
Electrochemical tests were performed in three-electrode systems with 6 mol L−1 KOH as electrolyte, the working electrode was prepared by mixing NixCoyS4/rGO composites, conductive additive (Super P), and binder (60 wt% PTFE emulsion) in a ratio of 8:1:1, Hg/HgO electrode as the reference electrode and Pt electrode as the counter electrode, respectively. By contrast, electrochemical tests carried out in the asymmetric supercapacitor were based on the abovementioned working electrode (positive electrode) and active carbon electrode (negative electrode), in which the active carbon electrode was prepared by mixing active carbon, conductive additive (Super P) and binder (60 wt% PTFE emulsion) in a ratio of 85:10:5. Cyclic voltammetry (CV) and electrochemical impedance spectroscopy (EIS) were tested by a CHI 660D electrochemical workstation (Chenhua Instruments, Shanghai). Galvanostatic charge-discharge (GCD) tests were carried out with the LAND electrochemical test system. Additional experimental details are described in the Supplementary Material of the work.
Result and Discussion
The crystallographic characteristics of the as-obtained precursors and NixCoyS4/rGO composites are characterized by XRD. As shown in Figure S2, for all samples, most of the diffraction peaks can be indexed to NixCoy(CO3)0.5(OH) (x+y = 1) that is derived from Co(CO3)0.5(OH)·0.11H2O (JCPDS Card No.48-0083). No impurity peaks were observed, which illustrates the substitution of Co by Ni during the growth of crystals with the increasing of nickel contents in the precursors (Xiao and Yang, 2011; Wan et al., 2013). With the decrease of Ni/Co ratio, the intensities of the diffraction peaks increase gradually, suggesting that the Co element plays a role in improving the crystallinity of precursor. For the XRD patterns of NixCoyS4/rGO composites (Figure 1), all of the XRD patterns of all NixCoyS4/rGO composites show weak diffraction peaks. The diffraction peaks at the 2θ of 31.4°, 38.2°, 50.1°, and 55.1° are ascribed to (311), (400), (511), and (440) planes of the cubic NiCo2S4 (JCPDS Card No.20-0782) or CoNi2S4 (JCPDS Card No.24-0334). The Ni1.64Co2.40S4/rGO composite owns the strongest diffraction peaks, reflecting the highest crystallinity among all samples.
SEM and TEM technique were used to characterize the morphology of the precursors and NixCoyS4/rGO composites as shown in Figures S3, S4 and Figure 2. Figures S3a–d show SEM images and EDS results of NC2010, NC1515, NC1020, and NC0624, respectively. For all precursors, a nano-needle type of Ni-Co compounds were embedded onto the surface of rGO network. With the decrease of Ni/Co ratio, the size of nano-needles gradually increases as shown in Figure S3. The NC0624 possess maximum microscopic size with a longitudinal length larger than 2 μm, indicating that Co plays a crucial role in the vertical growth of crystal. EDS results reveals that the ratio of Ni/Co content decrease from 1.5 to 0.2, which is in well-accordance with our experimental design. Furthermore, the TEM and high-resolution transmission electron microscope (HRTEM) images of NC1515 are carried out to further illustrate the morphology and microstructure of the precursor. As shown in Figure S4, the Ni-Co compounds with solid nano-needle morphology evenly distributed on the surface of rGO with transparent morphology, and the diameter of the nanoneedle is about 20 nm. The interplanar crystal spacing of 0.88 nm in the HRTEM image (Figure S4d) can be referred to the (100) plane of NixCoy(CO3)0.5(OH).
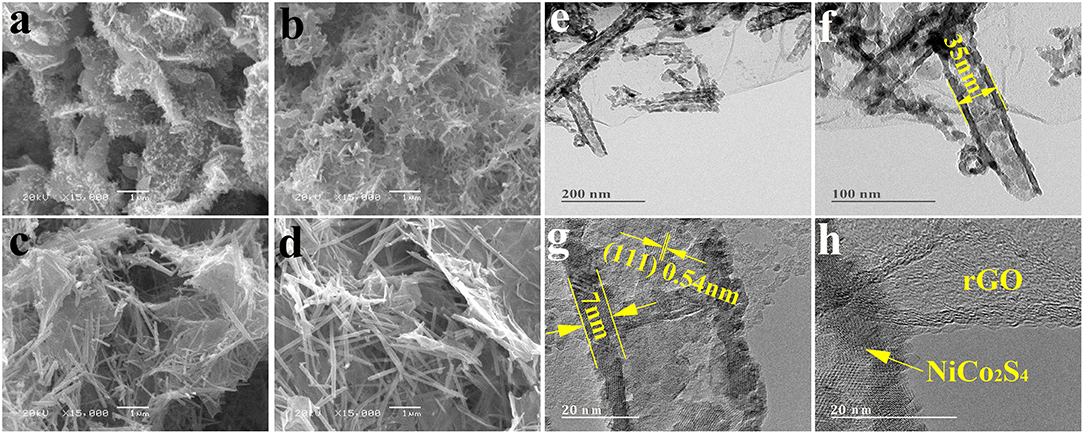
Figure 2. The morphology of NixCoyS4/rGO composites. (a–d) SEM images of NixCoyS4/rGO composites; Ni2.15Co1.37S4/rGO (a), Ni1.64Co2.40S4/rGO (b), Ni1.02Co2.98S4/rGO (c), and Ni0.60Co3.60S4/rGO (d). (e–h) TEM and HRTEM images of Ni1.64Co2.40S4/rGO; TEM images of Ni1.64Co2.40S4/rGO (e,f); HRTEM images of Ni1.64Co2.40S4/rGO (g,h).
Figures 2a–d show SEM images of Ni2.15Co1.37S4/rGO, Ni1.64Co2.40S4/rGO, Ni1.02Co2.98S4/rGO, and Ni0.60Co3.60S4/rGO composites, respectively. It is obvious that the sulfides inherit the morphological features of corresponding precursors with the NixCoyS4 decorated on the surface of rGO. It is worth noting that the TEM images of Ni1.64Co2.40S4/rGO (see Figure S4 and Figures 2e–h) confirms that the solid nano-needles transfer to nano-tube during ion exchange process from the precursor to sulfides. Specifically, the hollow structures with a rough surface possess an outer diameter of about 35 nm, which is larger than the diameter of the precursor (20 nm). The TEM results of precursors and Ni1.64Co2.40S4/rGO composite suggest that the Ni2+ ions and Co2+ ions diffused outwards from the precursor and combined with sulfur ions to form sulfides. The EDS results of NixCoyS4/rGO composites in Figure S1 reveals that Ni, Co, and S elements exist on the surface of the composites. In addition, it is worth noting that the S-doped graphene is also formed during the ion-exchange process, which is evidenced by a set of comparison experiments. Specifically, the GO was reduced in Na2S·9H2O solution under the same condition as preparing NixCoyS4/rGO composites, which can be confirmed by the C1s, and O1s XPS spectra in Figure S5. Moreover, the characteristic of sulfur peak in the EDS spectra (Figure S6) and C = S, C-S-C bonds in S2p spectra of aforementioned rGO sample confirm the existence of sulfur in rGO, which is beneficial to improve the pseudo-capacitance because of the heteroatom doping of S (Liu et al., 2017).
To further probe the formation of nickel-cobalt sulfides (NixCoyS4) and the S-doped rGO in the sulfide composite, XPS (X-ray photoelectron spectroscopy) is utilized to analyze the elemental composition and valence state of the material. Figure 3 shows survey, Ni 2p, Co 2p, S 2p, C 1s, and O 1s spectrum of the Ni1.64Co2.40S4/rGO composite, and Table S2 lists the peak fitting parameters of all spectrums. As shown in Figure 3A, it can be confirmed that Ni, Co, S, C, and O elements exist on the surface of Ni1.64Co2.40S4/rGO composite. In high resolution spectrums of Ni 2p and Co 2p, the spectrums can be classified into 2p 3/2 and 2p 1/2 sections, and the corresponding satellite peaks are labeled as “Sat” in the plot. Specifically, the peaks at 853.5 and 870.8 eV in Ni 2p correspond to Ni2+, and the peaks at 857.7 and 875.5 eV can be assigned to Ni3+, confirming the coexistence of both Ni2+ and Ni3+ in the composite. Similarly, the peaks located at 779.0 eV, 794.1 eV in Co 2p corresponds to the Co3+, and the peaks at 782.2 eV, 798.8 eV of Co 2p spectrums associates to the Co2+, suggesting the coexistence of Co3+ and Co2+ in Ni1.64Co2.40S4/rGO composite as well (Fan et al., 2017; Qin et al., 2017). For the S 2p spectrum, the peak located at 161.8 eV for S 2p 3/2 is attributed to S2− or C-S-C bonds on the surface (Chen X. et al., 2014), while the peak at 162.9 eV for S 2p1/2 can be ascribed to S-M (metal) bonds. In addition, the peak at a high binding energy of 169.6 eV (satellite) can be attributed to the highly oxidized state sulfur species (S4+) (Ma et al., 2016). The added Na2S·9H2O act as both of S2− sources and reductant during the high temperature ion-exchange reaction, in which part of S2− loses electrons and been oxidized to the higher-valence, and the GO been reduced to rGO simultaneously. Furthermore, the C 1s spectrum exhibits a major peak at 284.7 eV attributed to C-S-C bonds (Wenfang et al., 2015). Additionally, a peak located at 285.9 eV in the C 1s spectra corresponds to the C = C bond (aromatic-linked carbon), while the weak peak at 289.2 eV can be ascribed to the carboxyl groups (C = O) and sp2 hybridized carbon from rGO, the low strength of the peak at 289.2 eV also indicates that the graphene has been reduced (Qin et al., 2017). The S 2p and C 1s XPS results reveal the existence of S-M (M = Ni or Co) and C-S-C bonds, respectively, which verifies the doping of S into rGO and the formation of transition metal sulfides in the composite material. Based on the XRD, SEM, TEM, and XPS results as analyzed in Figures 1–3, we proofed that the NixCoyS4/rGO composites can be synthesized by such in situ growth—ion exchange strategy.
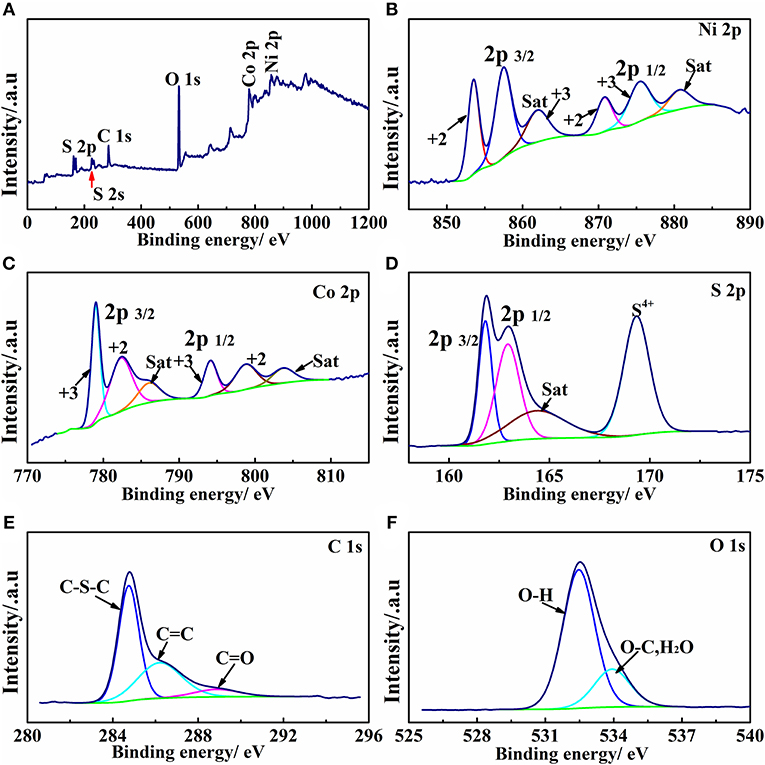
Figure 3. XPS spectrum of Ni1.64Co2.40S4/rGO composite: survey scan (A), High-resolution spectra of Ni 2p (B), Co 2p (C), S 2p (D), C 1s (E), and O 1s (F), respectively.
The electrochemical performances of NixCoyS4/rGO composites are measured by cyclic voltammetry at various scan rates in a three-electrode cell as shown in Figure 4. For all composites, anodic and cathodic peaks with good symmetry indicate the good reversibility of the materials. The positions of anodic and cathodic peaks shift to the more positive and more negative directions with the increase of scan rates from 1 mV s−1 to 100 mV s−1, which implies an increase of the polarization. It is known to all that the relationship between scan rate and current density is an effective method to distinguish capacitive contribution by the i = aνb formula. Specifically, when the b value is 1/2, the electrode shows a total diffusion-limited behavior (i = aν1/2); and when the b value is 1, the electrode shows a capacitive process (Lim et al., 2015). Figures 4E–H show the b values of Ni2.15Co1.37S4/rGO, Ni1.64Co2.40S4/rGO, Ni1.02Co2.98S4/rGO and Ni0.60Co3.60S4/rGO as deduced from the slope of log (i)—log (v) curves, which are 0.55, 0.78, 0.86, and 0.89, respectively. The b values of the electrodes increase with the decrease of Ni/Co ratio, which implies that the Ni2.15Co1.37S4/rGO composite is mainly diffusion-controlled kinetics behavior, and gradually transforms to the capacitive behavior for the Ni0.60Co3.60S4/rGO composites. As the switch of kinetics behavior from diffusion-controlled to capacitive behavior, it will accelerate the charge transfer process so as to improve the rate capability of the NixCoyS4/rGO composites.
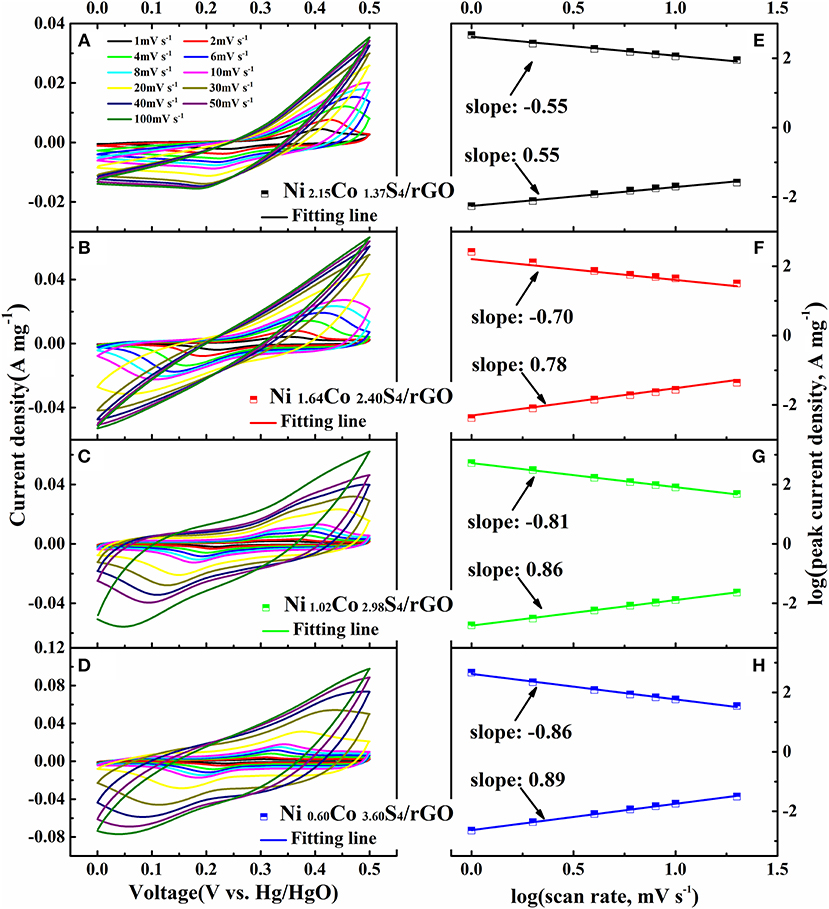
Figure 4. Electrochemical properties of NixCoyS4/rGO composites at three-electrode cells. CV curves of Ni2.15Co1.37S4/rGO, Ni1.64Co2.40S4/rGO, Ni1.02Co2.98S4/rGO, and Ni0.60Co3.60S4/rGO at different scan rates (A–D); relationship between scan rate and current density of Ni2.15Co1.37S4/rGO, Ni1.64Co2.40S4/rGO, Ni1.02Co2.98S4/rGO, and Ni0.60Co3.60S4/rGO (E–H).
The CV results of Ni2.15Co1.37S4/rGO, Ni1.64Co2.40S4/rGO, Ni1.02Co2.98S4/rGO, and Ni0.60Co3.60S4/rGO at 1 mV s−1 are presented in Figure 5A. Obviously, the Ni2.15Co1.37S4/rGO material has the largest integral area, indicating the highest specific capacitance as compared to other samples. The specific capacitance of the material decreases with the decrease of Ni/Co ratio in NixCoyS4/rGO composites, which is related to the microstructure transformation of the material. Specifically, Ni1.64Co2.40S4/rGO consists of tiny one-dimensional nano-needles that convince to the sufficient contact between the active material and the electrolyte during the electrode process. In addition, the response current of the CV curves is a combination of capacitor and diffusion-controlled behavior. The respective contribution between diffusion-controlled and capacitive capacitance can be calculated by the following equation (Lim et al., 2015):
The capacitance proportion of diffusion-controlled process and capacitor-like processes are expressed by k1ν and k, respectively. The capacitive-contribution of Ni1.64Co2.40S4/rGO is 79.83% at 1 mV s−1 as shown in Figure S7, which confirms that the specific capacitance is mainly contributed by the capacitive-like process. Besides, the difference between the oxidation potential and reduction potential decrease obviously (the potential differences of NixCoyS4/rGO composites are 0.132 V (Ni2.15Co1.37S4/rGO), 0.104 V (Ni1.64Co2.40S4/rGO), 0.089 V (Ni1.02Co2.98S4/rGO), and 0.093 V (Ni0.60Co3.60S4/rGO) respectively), indicating an gradually improved reversibility of the composites, which is beneficial to improve the rate performance of the electrodes. Figures 5B,C shows the rate capability of the composites as the current density range from 1 A g−1 to 20 A g−1. It is noteworthy that the rate performance is improved distinctively with the decrease of Ni/Co molar ratio in composites. Specifically, when the current density increase to 20 A g−1, the Ni1.64Co2.40S4/rGO, Ni1.02Co2.98S4/rGO, and Ni0.60Co3.60S4/rGO composites can recover 92.6, 99.5, and 99.6% of their capacitance determined at 1 A g−1, respectively (Table S3). In contrast, the Ni2.15Co1.37S4/rGO composite can only retain 68.9% of its capacitance determined at 1 A g−1 under the same condition (Table S3). Our detailed electronic conductivity results (Figure 5D) evidence that higher Co content in NixCoyS4/rGO composites is beneficial to improve the electronic conductivity of the composites, which is the main reason for the improvement of rate performance. Considering the merits of capacitance and rate capability of the composites, Ni1.64Co2.40S4/rGO exhibits the best comprehensive performance in the application of supercapacitor, which delivers the capacitance of 1,089 F g−1 at the current density of 1 A g−1, and retains 1,008 F g−1 at the current density of 20 A g−1. The GCD curves of Ni2.15Co1.37S4/rGO, Ni1.64Co2.40S4/rGO, Ni1.02Co2.98S4/rGO, and Ni0.60Co3.60S4/rGO at the current density of 1 A g−1 show nearly symmetric GCD curves, implying the high coulomb efficiency of the charge-discharge process (Figure 5E and Figure S8). Meanwhile, the time corresponding to the discharge platform gradually shortens with the decrease of Ni/Co ratio, indicating that the proportion of pseudocapacitance originated from Faraday reaction reduces. It is in well-accordance with the result of CV results as shown in Figure 5A. Moreover, the GCD curves of Ni1.64Co2.40S4/rGO at various current densities in Figure 5F suggests that the electrode have excellent rate performance.
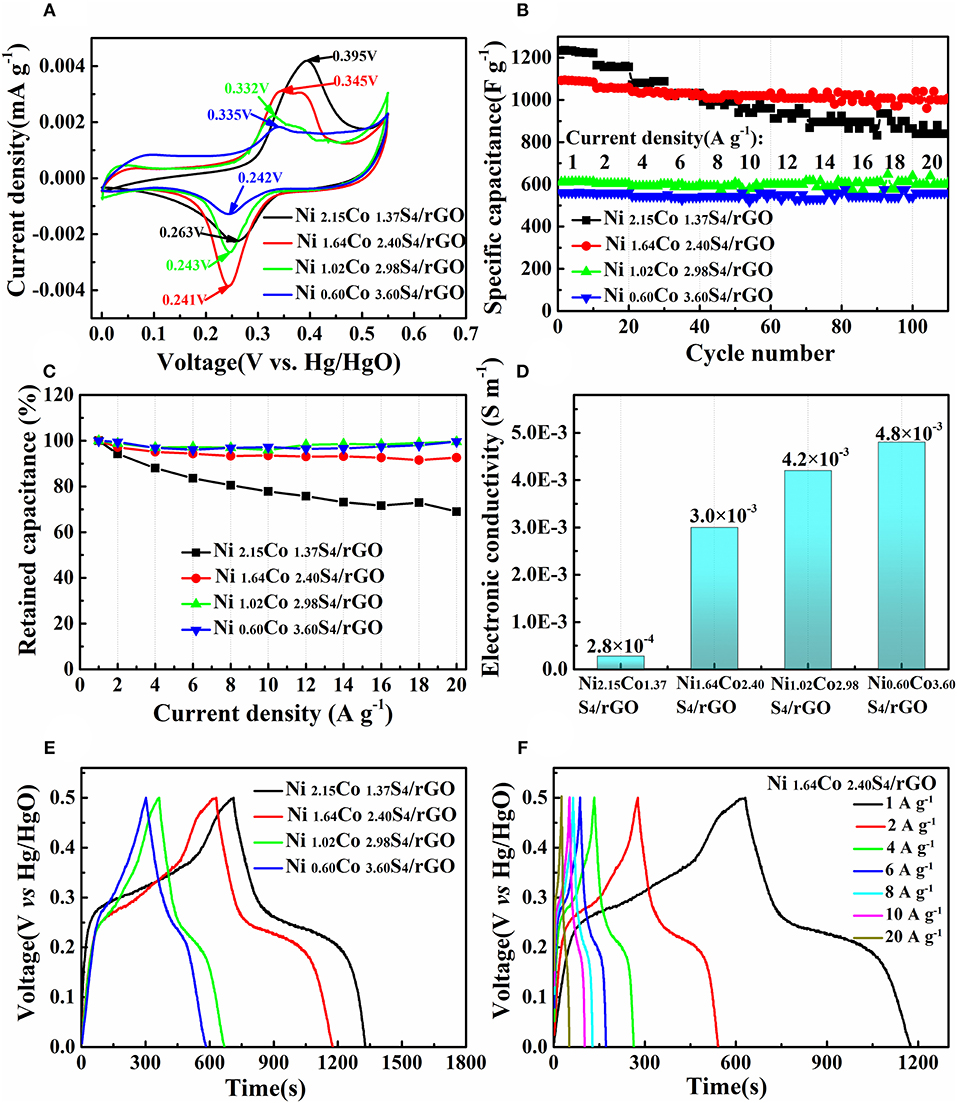
Figure 5. CV curves of NixCoyS4/rGO composites at the scan rate of 1 mV s−1 (A); rate performance of NixCoyS4/rGO composites at various current densities from 1 A g−1 to 20 A g−1 (B); capacitance retention of NixCoyS4/rGO composites with the increase of current density (C); electronic conductivity of NixCoyS4/rGO composites (D); GCD curves of different composites at the current densities of 1 A g−1 (E); GCD curves of Ni1.64Co2.40S4/rGO at various current densities from 1 A g−1 to 20 A g−1 (F).
To demonstrate the practical application of Ni1.64Co2.40S4/rGO composite for energy storage, an asymmetric supercapacitor marked as Ni1.64Co2.40S4/rGO//AC was assembled, in which Ni1.64Co2.40S4/rGO acts as the positive material and active carbon (AC) acts as the negative material. As shown in Figure 6A, the shape of the CV curve shows slight differences at various scan rate, indicating that the Ni1.64Co2.40S4/rGO//AC asymmetric supercapacitor has good charge-discharge characteristics. The specific capacitances of Ni1.64Co2.40S4/rGO//AC deduced from the integral area of CV curves at various current density are calculated and shown in Figure 6B. High capacitance (270.7 F g−1 at 1 mV s−1) and high capacitance retention (68.5% at 100 mV s−1) confirm the superior rate performance of the device, which is attributed to the proper composition and hollow structure of Ni1.64Co2.40S4. The relationship between scan rate and current density of Ni1.64Co2.40S4/rGO//AC during the charge-discharge process is also calculated as shown in Figure 6C. The b value in the charge and discharge process are −0.70 and 0.77, respectively, which is quite consistent with the previous results as measured in the three-electrode cell. The specific capacitance and the corresponding GCD curves of the Ni1.64Co2.40S4/rGO//AC asymmetric supercapacitor at various current densities are presented in Figures 6D,E, the specific capacitances are 265.5, 209.4, 195.6, 191.3, 180.0, and 168.8 F g−1 at the current density of 1, 10, 20, 30, 40, and 50 A g−1 respectively. The high capacitance retention of 63.6% at 50 A g−1 as compared to 1 A g−1 further verifies the excellent rate performance of the asymmetric supercapacitor. Furthermore, the long-term cycling measurements of the Ni1.64Co2.40S4/rGO//AC at 10 A g−1 (as shown in Figure 6F) evidence the good cycling stability as it can retain 92.6 % of its initial capacitance after 10,000 times, demonstrating the high cyclic stability in terms of electrochemical and mechanical properties of the Ni1.64Co2.40S4/rGO composites. Eventually, the high columbic efficiency approaching 100% and the symmetric GCD curves of the last few loops (Figures 6G,H) confirm the excellent electrochemical performance of the Ni1.64Co2.40S4/rGO//AC asymmetric supercapacitor.
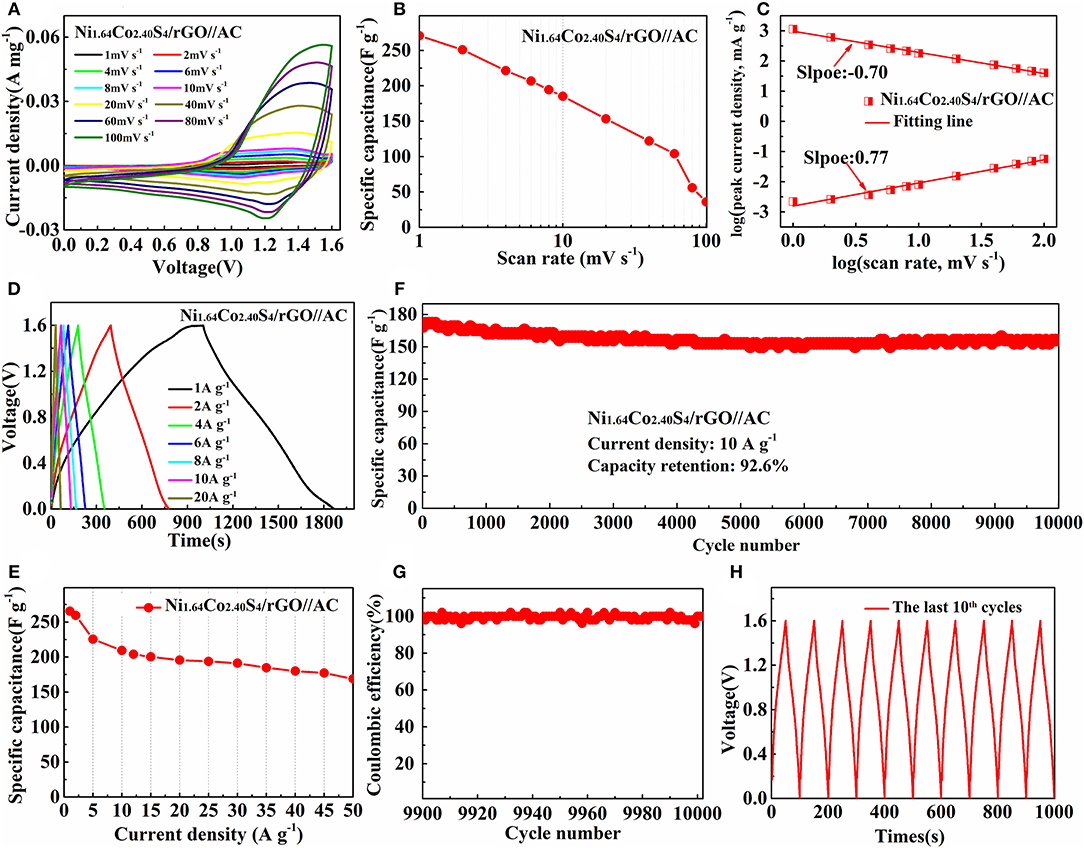
Figure 6. Electrochemical properties of Ni1.64Co2.40S4/rGO//AC asymmetric supercapacitor. CV curves of Ni1.64Co2.40S4/rGO//AC at different scan rates (A); specific capacitance of Ni1.64Co2.40S4/rGO//AC calculated from CV curves (B); relationship between scan rate and current density of Ni1.64Co2.40S4/rGO//AC (C); GCD curves of Ni1.64Co2.40S4/rGO//AC at various current densities (D); average specific capacitances in dependence of cycle number at various current densities from 1 A g−1 to 20 A g−1 (E); cycle performance of Ni1.64Co2.40S4/rGO//AC at the current density of 10 A g−1 (F); columbic efficiency of Ni1.64Co2.40S4/rGO//AC at the last 100 cycles (G); GCD curves of Ni1.64Co2.40S4/rGO//AC at the last 10 cycles of 10000 cycles (H).
For positioning the level of as-obtained Ni1.64Co2.40S4/rGO composite, we carefully investigated and compared the energy density and power density of supercapacitors using similar materials from the peer-reviewed papers. Ragone plot of these asymmetric supercapacitors derived from GCD tests are summarized and plotted in Figure 7 and Table S4. Specifically, the Ni1.64Co2.40S4/rGO//AC asymmetric supercapacitor can reach the maximum energy density of 47.2 Wh kg−1 at the power density of 200 W kg−1, and retains an energy density of 30 Wh kg−1 even at high power density of 10 kW kg−1. Impressively, the Ni1.64Co2.40S4/rGO//AC asymmetric supercapacitor presented in this work is comparable or even superior to those of previous reported transition metal sulfides-based asymmetric supercapacitors and other supercapacitors (listed in Table S5), such as NCS/rGO/CNT-3//AC asymmetric supercapacitors (21.06 Wh kg−1 at the power density of 729 W kg−1 and 14.43 Wh kg−1 at the power density of 3646 W kg−1) (Chiu and Chen, 2018), rGO/CoNiSx/N–C//AC asymmetric supercapacitors (32.9 Wh kg−1 at the power density of 229.2 W kg−1 and 20.9 Wh kg−1 at the power density of 1833.3 W kg−1) (Chen Q. et al., 2018), NiCo2S4@CNFs//AC@pCNFs asymmetric supercapacitors (32.1 Wh kg−1 at the power density of 67.6 W kg−1 and 5.3 Wh kg−1 at the power density of 1,270 W kg−1) (Liu et al., 2018b), GCM//H-GCS FASC (24.6 Wh kg−1 at the power density of 459.3 W kg−1 and 17.7 Wh kg−1 at the power density of 9005.3 W kg−1) (Wu S. et al., 2018), NSC-900/MnO2//NSC-900 asymmetric supercapacitors (39.15 Wh kg−1 at the power density of 901 W kg−1 and 28.8 Wh kg−1 at the power density of 8,907 W kg−1) (Miao et al., 2018), NiCo2S4@NiO//AC (30.38 Wh kg−1 at the power density of 288 W kg−1) (Huang et al., 2016), NiCo2S4/RGO//AC(24.4 Wh kg−1 at the power density of 750 W kg−1) (Li Z. et al., 2016), NiCo2S4 nanotube arrays-Ni foam//RGO (31.5 Wh kg−1 at the power density of 156.6 W kg−1) (Chen H. et al., 2014), Co9S8 nanoflake//AC (31.4 Wh kg−1 at the power density of 200 W kg−1 and 26.3 Wh kg−1 at the power density of 4,000 W kg−1) (Rakhi et al., 2014).
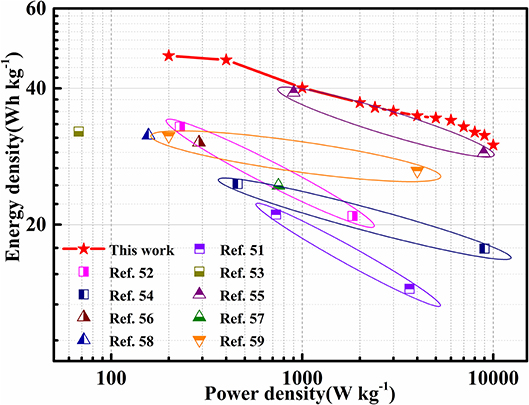
Figure 7. Comparison of energy densities among as-constructed Ni1.64Co2.40S4/rGO//AC with recently reported asymmetric supercapacitors.
Conclusion
We have reported an in situ growth—ion exchange method to synthesize NixCoyS4/rGO composites with high energy and power density. We concluded from the composition, electronic conductivity, and electrochemical measurements that proper Ni/Co molar ratio in NixCoyS4/rGO composites is vital to synthesize the material with high capacitance and rate capability. Here the as-prepared Ni1.64Co2.40S4/rGO composite shows the best overall electrochemical performance among the obtained samples as it delivers a specific capacitance of 1,089 F g−1 at 1 A g−1, and it maintains 1,008 F g−1 at high current density of 20 A g−1. The derived Ni1.64Co2.40S4/rGO//AC asymmetric supercapacitor offers high energy density of 47.2 Wh kg−1 (200 W kg−1), together with a superior cyclic stability (maintaining 92.6 % of capacitance retention after 10,000 cycles at 10 A g−1). Through this study, the synthesized and characterized Ni1.64Co2.40S4/rGO composite provides a promising material for fabricating supercapacitors with both high energy and power density.
Data Availability
All datasets generated for this study are included in the manuscript/Supplementary Files.
Author Contributions
The design and conduction of the experiment, the acquisition of data, the writing and modification of the paper are completed by MD. ZW and JW helped perfecting the experimental ideas and technical route. GY devoted many efforts to revise the manuscript. HG and XL gave advices during the discussion of the experimental results.
Funding
This research was supported by the National Science Foundation of China (51674295, 51804344), the Fundamental Research Funds for the Central University of Central South University (2018zzts017), the Hunan Provincial Innovation Foundation for Postgraduate (CX2018B088).
Conflict of Interest Statement
The authors declare that the research was conducted in the absence of any commercial or financial relationships that could be construed as a potential conflict of interest.
Supplementary Material
The Supplementary Material for this article can be found online at: https://www.frontiersin.org/articles/10.3389/fmats.2019.00176/full#supplementary-material
Supporting information for publication is available. XRD patterns, SEM images, TEM images of precursors; XPS, SEM, and EDS results of GO and rGO-Na2S·9H2O; GCD curves of Ni2.15Co1.37S4/rGO, Ni1.02Co2.98S4/rGO, and Ni0.60Co3.60S4/rGO; C-S and ICP results of NixCoyS4/rGO composites.
References
Annamalai, K. P., Liu, L., and Tao, Y. (2017). Highly exposed nickel cobalt sulfide–rGO nanoporous structures: an advanced energy-storage electrode material. J. Mater. Chem. A 5, 9991–9997. doi: 10.1039/C7TA01735A
Booth, T. J., Blake, P., Nair, R. R., Jiang, D., Hill, E. W., Bangert, U., et al. (2008). Macroscopic graphene membranes and their extraordinary stiffness. Nano Lett. 8, 2442–2446. doi: 10.1021/nl801412y
Brousse, T. (2015). To be or not to be pseudocapacitive? J. Electrochem. Soc. 162, A5185–A5189. doi: 10.1149/2.0201505jes
Chen, H., Jiang, J, Zhang, L., Xia, D., Zhao, Y., Guo, D., et al. (2014). In situ growth of NiCo2S4 nanotube arrays on Ni foam for supercapacitors: maximizing utilization efficiency at high mass loading to achieve ultrahigh areal pseudocapacitance. J. Power Sources 254, 249–257. doi: 10.1016/j.jpowsour.2013.12.092
Chen, H., Jiang, J., Zhang, L., Wan, H., Qi, T., and Xia, D. (2013). Highly conductive NiCo2S4 urchin-like nanostructures for high-rate pseudocapacitors. Nanoscale 5, 8879–8883. doi: 10.1039/c3nr02958a
Chen, J., Li, L., Wu, L., Yao, Q., Yang, H., Liu, Z., et al. (2018). Enhanced cycle stability of Na0.9Ni0.45Mn0.55O2 through tailoring O3/P2 hybrid structures for sodium-ion batteries. J. Power Sources 406, 110–117. doi: 10.1016/j.jpowsour.2018.10.058
Chen, Q., Miao, J., Quan, L., Cai, D., and Zhan, H. (2018). Bimetallic CoNiSx nanocrystallites embedded in nitrogen-doped carbon anchored on reduced graphene oxide for high-performance supercapacitors. Nanoscale 10, 4051–4060. doi: 10.1039/C7NR08284C
Chen, X., Chen, X., Xu, X., Yang, Z., Liu, Z., Zhang, L., et al. (2014). Sulfur-doped porous reduced graphene oxide hollow nanosphere frameworks as metal-free electrocatalysts for oxygen reduction reaction and as supercapacitor electrode materials. Nanoscale 6, 13740–13747. doi: 10.1039/C4NR04783D
Chiu, C. T., and Chen, D. H. (2018). One-step hydrothermal synthesis of three-dimensional porous Ni-Co sulfide/reduced graphene oxide composite with optimal incorporation of carbon nanotubes for high performance supercapacitors. Nanotechnology 29:175602. doi: 10.1088/1361-6528/aaaff5
Deng, Y., Xu, A., Lu, W., Yu, Y., Fua, C., and Shua, T. (2017). Graphene-based ordered mesoporous carbon hybrids with large surface areas for supercapacitor. New J. Chem. 42, 7043–7048. doi: 10.1039/C7NJ03923A
Dong, M., Wang, Z., Li, X., Guo, H., and Wang, J. (2018). A smart architecture of nickel-cobalt sulfide nanotubes assembled nanoclusters for high-performance pseudocapacitor. J. Alloys Compd. 765, 505–511. doi: 10.1016/j.jallcom.2018.06.179
Eftekhari, A., Li, L., and Yang, Y. (2017). Polyaniline supercapacitors. J. Power Sources 347, 86–107. doi: 10.1016/j.jpowsour.2017.02.054
Fan, Y.-M., Liu, Y., Liu, X., Liu, Y., and Fan, L.-Z. (2017). Hierarchical porous NiCo2S4-rGO composites for high-performance supercapacitors. Electrochim. Acta 249, 1–8. doi: 10.1016/j.electacta.2017.07.175
Gao, Z., Chen, C., Chang, J., Chen, L., Wang, P., Wu, D., et al. (2018). Enhanced cycleability of faradic CoNi2S4 electrode by reduced graphene oxide coating for efficient asymmetric supercapacitor. Electrochim. Acta 281, 394–404. doi: 10.1016/j.electacta.2018.05.194
Gilshteyn, E. P., Amanbayev, D., Anisimov, A. S., Kallio, T., and Nasibulin, A. G. (2017). All-nanotube stretchable supercapacitor with low equivalent series resistance. Sci. Rep. 7:17449. doi: 10.1038/s41598-017-17801-4
Huang, Y., Shi, T., Jiang, S., Cheng, S., Tao, X., Zhong, Y., et al. (2016). Enhanced cycling stability of NiCo2S4@NiO core-shell nanowire arrays for all-solid-state asymmetric supercapacitors. Sci. Rep. 6:38620. doi: 10.1038/srep38620
Jiang, Y., and Liu, J. (2019). Definitions of pseudocapacitive materials: a brief review. Energy Environ. Mater. 2, 30–37. doi: 10.1002/eem2.12028
Jinlong, L., Tongxiang, L., Meng, Y., Ken, S., and Hideo, M. (2017). Performance comparison of NiCo2O4 and NiCo2S4 formed on Ni foam for supercapacitors. Compos. Part B 123, 28–33. doi: 10.1016/j.compositesb.2017.05.021
Ke, Q., and Wang, J. (2016). Graphene-based materials for supercapacitor electrodes – a review. J. Materiom. 2, 37–54. doi: 10.1016/j.jmat.2016.01.001
Lee, C., Wei, X., Kysar, J. W., and Hone, J. (2008). Measurement of the elastic properties and intrinsic strength of monolayer graphene. Science 321, 385–388. doi: 10.1126/science.1157996
Li, D., Gong, Y., and Pan, C. (2016). Facile synthesis of hybrid CNTs/NiCo2S4 composite for high performance supercapacitors. Sci. Rep. 6:29788. doi: 10.1038/srep29788
Li, L., Chen, Z., Zhang, Q., Xu, M., Zhou, X., Zhu, H., et al. (2015). A hydrolysis-hydrothermal route for the synthesis of ultrathin LiAlO2-inlaid LiNi0.5Co0.2Mn0.3O2 as a high-performance cathode material for lithium ion batteries. J. Mater. Chem. A 3, 894–904. doi: 10.1039/C4TA05902F
Li, Z., Ji, X., Han, J., Hu, Y., and Guo, R. (2016). NiCo2S4 nanoparticles anchored on reduced graphene oxide sheets: in-situ synthesis and enhanced capacitive performance. J. Colloid Interface Sci. 477, 46–53. doi: 10.1016/j.jcis.2016.05.038
Liang, K., He, W., Deng, X., Ma, H., and Xu, X. (2018). Controlled synthesis of NiCo2S4 hollow spheres as high-performance electrode materials for supercapacitors. J. Alloys Compd. 735, 1395–1401. doi: 10.1016/j.jallcom.2017.11.153
Lim, E., Jo, C., Kim, H., Kim, M. H., Mun, Y., Chun, J., et al. (2015). Facile synthesis of Nb2O5@carbon core-shell nanocrystals with controlled crystalline structure for high-power anodes in hybrid supercapacitors. ACS Nano 9, 7497–7505. doi: 10.1021/acsnano.5b02601
Liu, S., Cai, Y., Zhao, X., Liang, Y., Zheng, M., Hu, H., et al. (2017). Sulfur-doped nanoporous carbon spheres with ultrahigh specific surface area and high electrochemical activity for supercapacitor. J. Power Sources 360, 373–382. doi: 10.1016/j.jpowsour.2017.06.029
Liu, Y., Fan, X., Huang, X., Liu, D., Dou, A., Su, M., et al. (2018a). Electrochemical performance of Li1.2Ni0.2Mn0.6O2 coated with a facilely synthesized Li1.3Al0.3Ti1.7(PO4)3. J. Power Sources 403, 27–37. doi: 10.1016/j.jpowsour.2018.09.082
Liu, Y., Jiang, G., Sun, S., Xu, B., Zhou, J., Zhang, Y., et al. (2018b). Decoration of carbon nanofibers with NiCo2S4 nanoparticles for flexible asymmetric supercapacitors. J. Alloys Compd. 731, 560–568. doi: 10.1016/j.jallcom.2017.10.078
Liu, Y., Wang, Q., Zhang, Z., Dou, A., Pan, J., and Su, M. (2016). Investigation the electrochemical performance of layered cathode material Li1.2Ni0.2Mn0.6O2 coated with Li4Ti5O12. Adv. Powder Technol. 27, 1481–1487. doi: 10.1016/j.apt.2016.05.008
Ma, L., Chen, T., Li, S., Gui, P., and Fang, G. (2019). A 3D self-supported coralline-like CuCo2S4@NiCo2S4 core-shell nanostructure composite for high-performance solid-state asymmetrical supercapacitors. Nanotechnology 30:255603. doi: 10.1088/1361-6528/ab08fb
Ma, L., Hu, Y., Chen, R., Zhu, G., Chen, T., Lv, H., et al. (2016). Self-assembled ultrathin NiCo2S4 nanoflakes grown on Ni foam as high-performance flexible electrodes for hydrogen evolution reaction in alkaline solution. Nano Energy 24, 139–147. doi: 10.1016/j.nanoen.2016.04.024
Miao, L., Zhu, D., Liu, M., Duan, H., Wang, Z., Lv, Y., et al. (2018). N, S Co-doped hierarchical porous carbon rods derived from protic salt: facile synthesis for high energy density supercapacitors. Electrochim. Acta 274, 378–388. doi: 10.1016/j.electacta.2018.04.100
Mohamed, S. G., Hussain, I., and Shim, J. J. (2018). One-step synthesis of hollow C-NiCo2S4 nanostructures for high-performance supercapacitor electrodes. Nanoscale 10, 6620–6628. doi: 10.1039/C7NR07338K
Nan, H., Han, J., Luo, Q., Yin, X., Zhou, Y., Yao, Z., et al. (2018). Economically synthesized NiCo2S4/reduced graphene oxide composite as efficient counter electrode in dye-sensitized solar cell. Appl. Surf. Sci. 437, 227–232. doi: 10.1016/j.apsusc.2017.12.175
Qin, H., Yang, S., Zhao, W., Yang, Z., Li, X., Li, H., et al. (2017). Synthesis of mesoporous NiCo2S4 deposited on reduced graphite oxide assistant by co-polymer Pluronic F127 for high-performance supercapacitor. Appl. Surf. Sci. 420, 77–82. doi: 10.1016/j.apsusc.2017.05.048
Qu, C., Zhang, L., Meng, W., Liang, Z., Zhu, B., Dang, D., et al. (2018). MOF-derived α-NiS nanorods on graphene as an electrode for high-energy-density supercapacitors. J. Mater. Chem. A 6, 4003–4012. doi: 10.1039/C.7T.A.11100B.
Rakhi, R. B., Alhebshi, N. A., Anjum, D. H., and Alshareef, H. N. (2014). Nanostructured cobalt sulfide-on-fiber with tunable morphology as electrodes for asymmetric hybrid supercapacitors. J. Mater. Chem. A 9, 16190–16198. doi: 10.1039/C4TA03341H
Saha, S., Samanta, P., Murmu, N. C., and Kuila, T. (2018). A review on the heterostructure nanomaterials for supercapacitor application. J. Energy Storage 17, 181–202. doi: 10.1016/j.est.2018.03.006
Sarkar, A., Bera, S., and Chakraborty, A. K. (2018). NiCo2S4 nanorod embedded rGO sheets as electrodes for supercapacitor. AIP Conf. Proc. 1942:140029. doi: 10.1063/1.5029160
Simon, P., Gogotsi, Y., and Dunn, B. (2014). Where do batteries end and supercapacitors begin? Mater. Sci. 343, 1210–1211. doi: 10.1126/science.1249625
Tan, L., Li, X., Wang, Z., Guo, H., and Wang, J. (2018). Lightweight reduced graphene oxide@MoS2 interlayer as polysulfide barrier for high-performance lithium-sulfur batteries. ACS Appl. Mater. Interfaces 10, 3707–3713. doi: 10.1021/acsami.7b18645
Wan, H., Jiang, J., Yu, J., Xu, K., Ling, M., Li, Z., et al. (2013). NiCo2S4 porous nanotubes synthesis via sacrificial templates: high-performance electrode materials of supercapacitors. CrystEngComm 15, 7649–7651. doi: 10.1039/c3ce41243a
Wang, H., Liang, M., Ma, C., Shi, W., Duan, D., He, G., et al. (2019). Novel dealloying-fabricated NiCo2S4 nanoparticles with excellent cycling performance for supercapacitors. Nanotechnology 30:235402. doi: 10.1088/1361-6528/ab0605
Wang, J., Zhang, G., Liu, Z., Li, H., Liu, Y., Wang, Z., et al. (2018). Li3V(MoO4)3 as a novel electrode material with good lithium storage properties and improved initial coulombic efficiency. Nano Energy 44, 272–278. doi: 10.1016/j.nanoen.2017.11.079
Wang, Y., Yang, L., Liu, Y., Zhao, Q., Ding, F., Zou, P., et al. (2018). Colorimetric determination of dopamine by exploiting the enhanced oxidase mimicking activity of hierarchical NiCo2S4-rGO composites. Mikrochim. Acta 185, 496. doi: 10.1007/s00604-018-3035-8
Wen, Y., Peng, S., Wang, Z., Hao, J., Qin, T., Lu, S., et al. (2017). Facile synthesis of ultrathin NiCo2S4 nano-petals inspired by blooming buds for high-performance supercapacitors. J. Mater. Chem. A 5, 7144–7152. doi: 10.1039/C7TA01326D
Wenfang, D., Zhang, Y., Yang, L., Tan, Y., Ma, M., and Xie, Q. (2015). Sulfur-doped porous carbon nanosheets as an advanced electrode material for supercapacitors. RSC Adv. 5, 13046–13051. doi: 10.1039/C4RA14820G
Wu, F., Chen, S., Srot, V., Huang, Y., Sinha, S. K., Van Aken, P. A., et al. (2018). A sulfur-limonene-based electrode for lithium-sulfur batteries: high-performance by self-protection. Adv. Mater. 30:e1706643. doi: 10.1002/adma.201706643
Wu, S., Hui, K. S., and Hui, K. N. (2018). Carbon nanotube@manganese oxide nanosheet core-shell structure encapsulated within reduced graphene oxide film for flexible all-solid-state asymmetric supercapacitors. Carbon N. Y. 132, 776–784. doi: 10.1016/j.carbon.2017.12.051
Xiao, J., and Yang, S. (2011). Sequential crystallization of sea urchin-like bimetallic (Ni, Co) carbonate hydroxide and its morphology conserved conversion to porous NiCo2O4 spinel for pseudocapacitors. RSC Adv. 1:588. doi: 10.1039/c1ra00342a
Xiong, X., Waller, G., Ding, D., Chen, D., Rainwater, B., Zhao, B., et al. (2015). Controlled synthesis of NiCo2S4 nanostructured arrays on carbon fiber paper for high-performance pseudocapacitors. Nano Energy 16, 71–80. doi: 10.1016/j.nanoen.2015.06.018
Yan, G., Dugas, R., and Tarascon, J.-M. (2018). The Na3V2(PO4)2 F-3/carbon na-ion battery: its performance understanding as deduced from differential voltage analysis. J. Electrochem. Soc. 165, A220–A227. doi: 10.1149/2.0831802jes
Yuan, D., Huang, G., Yin, D., Wang, X., Wang, C., and Wang, L. (2017). Metal-organic framework template synthesis of NiCo2S4@C encapsulated in hollow nitrogen-doped carbon cubes with enhanced electrochemical performance for lithium storage. ACS Appl. Mater. Interfaces 9, 18178–18186. doi: 10.1021/acsami.7b02176
Zhang, L., Hu, X., Wang, Z., Sun, F., and Dorrell, D. G. (2018). A review of supercapacitor modeling, estimation, and applications: a control/management perspective. Renew. Sustain. Energy Rev. 81, 1868–1878. doi: 10.1016/j.rser.2017.05.283
Zhang, Q., Chen, H., Luo, L., Zhao, B., Luo, H., Han, X., et al. (2018). Harnessing the concurrent reaction dynamics in active Si and Ge to achieve high performance lithium-ion batteries. Energy Environ. Sci. 11, 669–681. doi: 10.1039/C8EE00239H
Zhao, F., Huang, W., Shi, Q., Zhou, D., Zhao, L., and Zhang, H. (2017). Low temperature fabrication of hydrangea-like NiCo2S4 as electrode materials for high performance supercapacitors. Mater. Lett. 186, 206–209. doi: 10.1016/j.matlet.2016.09.110
Zhong, S., Hong, W., Zhang, X., Liu, J., Li, Y., Shi, Y., et al. (2017). Synthesis of xLiVPO4F center dot yLi3V2(PO4)3/C composite as a potential cathode material for Li-ion batteries. Ionics (Kiel). 23, 813–819. doi: 10.1007/s11581-016-1875-y
Zhong, S., Wu, L., Zheng, J., and Liu, J. (2012). Preparation of high tap-density 9LiFePO4 center dot Li3V2(PO4)(3)/C composite cathode material by spray drying and post-calcining method. Powder Technol. 219, 45–48. doi: 10.1016/j.powtec.2011.12.005
Keywords: nickel-cobalt sulfides, in-situ growth, high energy density, asymmetric supercapacitor, high cyclic stability
Citation: Dong M, Wang Z, Wang J, Guo H, Li X and Yan G (2019) Controlled Synthesis of NixCoyS4/rGO Composites for Constructing High-Performance Asymmetric Supercapacitor. Front. Mater. 6:176. doi: 10.3389/fmats.2019.00176
Received: 29 May 2019; Accepted: 08 July 2019;
Published: 24 July 2019.
Edited by:
Shengkui Zhong, Soochow University, ChinaReviewed by:
Biao Gao, Wuhan University of Science and Technology, ChinaChaopeng Fu, Shanghai Jiao Tong University, China
Copyright © 2019 Dong, Wang, Wang, Guo, Li and Yan. This is an open-access article distributed under the terms of the Creative Commons Attribution License (CC BY). The use, distribution or reproduction in other forums is permitted, provided the original author(s) and the copyright owner(s) are credited and that the original publication in this journal is cited, in accordance with accepted academic practice. No use, distribution or reproduction is permitted which does not comply with these terms.
*Correspondence: Guochun Yan, aGFwcHl5Z2MmI3gwMDA0MDtjc3UuZWR1LmNu