- Laboratory of Rheophysics and Macrokinetics, A. V. Luikov Heat and Mass Transfer Institute, National Academy of Sciences of Belarus, Minsk, Belarus
Electrophysical and rheological characteristics of ER fluids with TiO2 particles doped with aluminum and phosphorus and having anatase structure are considered in an extended temperature range (5–100°C). The mechanisms of leakage currents in the ER fluid in electric fields up to 3.5 kV/mm are determined. Conduction mechanisms such as thermionic ionization, electron injection from an electrode, thermal excitation of charges, field emission of electrons from an electrode, thermionic emission of electrons from an electrode, and field emission of electrons from traps are identified. Based on the analysis of the current-voltage characteristics of the ER fluid, the positive role of the additional doping of the dispersed phase particles with phosphorus compared with the known results for ER fluid with TiO2/Al particles was established. The use of double doping of particles of the dispersed phase made it possible to obtain stable dependences of the shear stress of electrorheological fluids on the electric field strength in the range of the temperature effects under study.
Introduction
A distinctive feature and the most important advantage of electrorheological fluids (ERF) is their controllability with the help of external electric fields, which allows their use for the creation of adaptive electromechanical devices of new generation. For the effective operation of such devices—heat exchangers, hydraulic systems, as well as devices used in dynamic conditions, for example, dampers and shock absorbers—fluids must exhibit a high ER-effect, stable in an extended temperature range. The main obstacle to obtaining the required results is the increase in conduction current in ER fluids with the temperature increase, which significantly reduces their rheological response. Due to the increase in the mobility of the charges and their quantity during heating, a breakdown situation arises, which does not allow for the application of an electric field of higher strength to obtain the required power indicators of the ER fluids. This is especially characteristic of ER fluids with particles of different oxides of an amorphous structure, in which there are bound charges at the interface and the characteristic Maxwell-Wagner type of polarization. In addition, the non-linearity of the dependencies of the electrorheological characteristics on temperature, which have maximum values of the shear stress τ already at a temperature of 50–70°C and show their further reduction, does not allow to adaptively control ER fluids to maintain a stable ER effect in dynamic systems, for example, in vibroprotection devices.
The results of both early and more recent investigations (Xu et al., 1992; Böse and Trendler, 2001; Yin and Zhao, 2001, 2006; Shang et al., 2007; Tang et al., 2010; Korobko et al., 2012) on the use of semiconductor-type particles as fillers of ER fluids are known. In particular, TiO2 particles with a crystalline structure (“anatase”) and high dielectric constant (ε = 90), which belong to electronic semiconductors, are considered. Their surface and bulk properties are modified by researchers with the introduction of various impurities into the structure, which, however, has not yet yielded the expected results of the thermal stability of the rheological parameters of the ER fluid based on them.
When studying the ER-effect, the features of electrical conduction mechanisms (leakage currents) in electrorheological fluids are of key importance. Detection of the features of the occurrence and manifestation of these mechanisms will allow us to explain the physical nature of their effect on the ER fluids with dispersed particles of various types and to assess the level of stability of their rheological parameters during thermal exposure. So, for example, electrorheological fluids with particles of a semiconductor type, in particular TiO2, showed a low rheological response to the influence of an external electric field (Yin and Zhao, 2001). The authors of this study showed that the ionic or atomic polarization of TiO2 does not create the polarization conditions necessary for the manifestation of a high ER effect. However, modern possibilities of applying the methods of modifying the crystal structure of a semiconductor allow researchers to purposefully change the bulk and surface properties of TiO2 particles to enhance their polarization and structural interaction, as well as optimize the number of charge carriers, including an extended temperature range.
The task of modifying the structure of TiO2 in order to improve the rheological parameters of the ER fluids was taken up by many researchers. Thus, some studies (Yin and Zhao, 2001, 2006; Shang et al., 2007; Tang et al., 2010) showed that doping of the crystal structure of titanium oxide with metal ions allows the ER effect to be significantly increased. The best results were shown by ERF containing TiO2 doped with Ce, La, Y, Al, Nb. The results of research by Tang et al. (2010) proved the effectiveness of ER fluids with particles of TiO2 doped with Y3+, Al3+, Nb3+ cations with high ionic potential (/aion).
Most researchers studied the effect of modifying the structure of TiO2 on the rheological parameters at room temperature. There are only a few papers devoted to the study of the rheology of electrorheological fluids with modified TiO2 particles under the influence of temperature. The most complete studies of ER fluids with Ce-doped particles of TiO2 in the extended temperature range 10–100°C were presented by Yin and Zhao (2001). Studying the dielectric spectra, electrical conductivity and rheological indices of ERF, the authors found that doping the structure of TiO2 particles with cerium (Ce) not only increases the rheological response of the ERF, but also shifts the peak of the dependence τ (T) to a higher temperature (80°C) compared with the results obtained by using unmodified TiO2 (40°C). These results were accompanied by a significant increase in conduction current and, according to the authors, can be explained by an increase in the number of charges thermally activated in the modified composition of the TiO2 particles.
We have investigated the rheological and electrophysical properties of ERF with TiO2 particles, doped with aluminum, annealed at different temperatures (Korobko et al., 2018). It was determined that the highest ER-activity in the electric field at strength of only up to 1.5 kV/mm has an ER fluid with TiO2 particles doped by 7 mol.% aluminum annealed at a temperature of 600°C. However, a high leakage current does not allow an increase in the electric field strength of more than 1.5 kV/mm even at a temperature of 20°C.
Increasing the aluminum content to 12 moles% in doped TiO2 particles and the annealing temperature up to 700°C contributed to a decrease in leakage current, which made it possible to increase the electric field strength to 3.0 kV/mm and increase the maximum value of the shear stress in the ER-fluid by two times. Analysis of the electrical conductivity of the investigated compositions of ER fluids containing TiO2 particles doped with aluminum showed that high leakage currents in strong electric fields with strengths up to 3.0 kV/mm in the temperature range from 5 to 80°C are caused by mechanisms such as thermal excitation of charge carriers, thermoelectronic ionization, as well as the injection of electrons from the electrode and Schottky emission.
Possible ways of placing the impurity Al3+ cations in the TiO2 lattice have been considered (Kofstad, 1972). Since the ionic radius of the Al3+ cation (0.054 nm) is smaller than the radius of the Ti4+ cation (0.061 nm), it is possible to partially replace the Ti4+ cation with Al3+ in the lattice sites of TiO2. With the location of impurities in the lattice sites the concentration of free electrons decreases. If the impurity is in the interstices, the concentration of free electrons increases, however, with this method of introduction, Al3+ cations can play the role of scattering centers and cause a decrease in the mobility of charge carriers. Thus, when the substitution and interstitial impurities are present simultaneously in the anatase structure, the possible reason for the decrease in the electrical conductivity can be the reduction in the concentration and mobility of charge carriers.
The results of studies of the synthesis of mesoporous titanium dioxide doped with phosphorus and the determination of its protocatalytic activity (Yu et al., 2003) showed that phosphorus, when doped with TiO2, can be incorporated into the crystal structure, either at the oxygen position or in the lattice interstitial sides.
Features of Charge Transfer Mechanisms in Semiconductor Materials
It is known that if both acceptor and donor impurities are introduced into the semiconductor, then impurities are compensated. Donor electrons spontaneously transfer to the level of acceptor atoms. The need for these electronic transitions is due to the fact that in the absence of external exciting effects, electrons must occupy all lower energy levels, and since acceptor levels lie below donor levels, donor electrons move to free acceptor levels. Impurity atoms can be placed in the crystal lattice in two ways: either at the sites of the lattice—impurity substitutions, or in the interstitial site—intersitial impurity. The character of impurity substitutional atoms is determined by their valence, that is, aluminum is an acceptor, and phosphorus is a donor. If the impurity atoms are introduced into the interstices of the lattices, then the sign of the impurity conductivity is determined by their electronegativity. If the electronegativity of impurity atoms is greater than that of the main atoms of the lattice, then the impurity has acceptor properties; if it is smaller, then the impurities are donors (Mitrofanov and Phogel, 1965). According to Pauling, the relative electronegativity of Ti is 1.8, Al−1.5, P−2.1. Thus, aluminum and phosphorus atoms in the anatase structure can be both donors and acceptors, depending on their location in the crystal lattice of the material.
In the present work, in order to reduce the magnitude of conduction currents (leakage) and increase the effective strength of a constant electric field with increasing intensity of temperature effects, the characteristics of ERF containing semiconductor TiO2 particles doped with aluminum and phosphorus are investigated and compared with similar indicators for ER fluids, containing TiO2 particles doped with aluminum only.
The focus is on assessing the rheological and electrophysical characteristics of ER fluids, as well as identifying features of existing conduction mechanisms that create leakage currents under various external influences and as a result of modifying the crystal structure of TiO2 particles.
It is known that a change in the conduction current (leakage current) of a semiconductor with a change in the electric field strength is due to the effect of the field on the mobility (μ) and the concentration of charge carriers in its volume. The change in charge mobility in an electric field, both in weak and strong fields, is determined, respectively, by the dependences μ ~ 1/√E and μ ~ E3/2. The concentration of charge carriers in semiconductors in strong fields, for which the linearity of the current-voltage characteristics (CVC) in the coordinates I(U) is not typical, increases noticeably with increasing electric field strength.
A number of studies (Sze, 1981; Shalimova, 1985), described several different mechanisms for the emergence and movement of charge carriers in a semiconductor under the influence of an external electric field. The mechanism of thermoelectronic ionization, in which the electron is detached from the atom (the Poole-Frenkel effect), acts first in an external electric field. The magnitude of the potential barrier that an electron must overcome when moving to the conduction band decreases when exposed to an external electric field. The linear character of the current-voltage characteristics (CVC) in the coordinates ln (j/E) from √E indicates that the conduction current is due to the Poole-Frenkel effect and can be calculated by the formula (1):
where is the electron concentration in the conduction-band in the absence of the electric field, L is the semiconductor layer thickness, e is the electron charge, T is the absolute temperature, U is the voltage, ε is the relative permittivity, ε0 is the electric constant, k is Boltzmann's constant (k = 8.62· × 10−5 eV/K), μ is charge mobility in the conduction-band, and J represents the current.
When exposed to strong electric fields in a semiconductor, a mechanism of excitation of charge carriers, caused by the impact of impact ionization, manifests itself. With external semiconductor heating, the change in the current value due to an increase in the number of charge carriers moved to the conduction-band is described by formula (2):
where ω represents the activation energy of process of excitation, and k is the Boltzmann's constant. In the presence of this process, the CVC is constructed in the Arrhenius coordinate system (lnj−1/kT) and is approximated by a linear dependence.
The current flowing through thin layers of a semiconductor is determined not only by its own charge carriers. When an electric field is applied, the electrons injected from the electrode into the semiconductor are drawn deep into the semiconductor and, passing through it, cause a current to flow. Such a current is not associated with its own electrons in the conduction band of the material, but with charge carriers injected from the cathode. The presence levels capture of electrons (traps) leads to a strong decrease in current, since the capture levels, being initially empty, capture the overwhelming part of injected electrons, preventing them from moving when exposed to an electric field.
Thus, the electrons trapped in the traps create a space charge, but do not participate in the charge transfer through the semiconductor in the capacitor gap. The presence of traps significantly reduces the amount of spatial-charge-limited current (SCLC). Its dependence on the electric field is represented by the formula (3):
where θ represents the proportionality coefficient and depends on the depth of the traps.
The experimental dependences of the CVC are plotted in logarithmic coordinates lnj–lnE and must be linear in the presence of a SCLC in a semiconductor.
The thermal emission of electrons from a heated metal into the conduction band of a semiconductor through a potential barrier in an electric field is called thermoelectron emission (Schottky emission). In this case, the value of the conduction current depends on the temperature, electric field strength, and the height of the metal-semiconductor potential barrier. The current caused by the Schottky emission mechanism can be described by the Richardson-Dashman equation (Sze, 1981; Shalimova, 1985):
where , CRD is the Richardson constant and φb represent the potential barrier height.
The presence of current in a semiconductor caused by thermoelectronic emission is determined according to the linearity of the dependence of the experimental results of the CVC in the coordinate system lnj –√E.
Electrons can enter the conduction band not only due to the kinetic energy of their thermal motion, which is observed during thermoelectronic emission, but as a result of the tunnel effect, in which the energy of the charge carrier is insufficient to overcome the potential barrier. In this case, the current caused by strong electric field strength (field emission) is described by the well-known Fowler-Nordheim equation (Fowler and Nordheim, 1928):
where represents the electron work function of metal, m the electron mass, where h–Planck's constant.
The linearity of the experimental results of the CVC in the coordinate system ln j/E−1/√E. determines the presence of current caused by the field emission mechanism in a semiconductor.
With strong electric fields or heating, the Poole-Frenkel effect transforms into trap tunneling or field emission of electrons from traps. The presence of current caused by the field emission of electrons from the traps is determined by the linearity of the experimental results of the CVC in the coordinates of the field emission ln (j/E2) −1/E. The current caused by this conduction mechanism can be described by the following expression:
Thus, the main, most typical mechanisms of charge transfer in semiconductors materials, that allow us to study the physical nature and estimate the values of the corresponding conduction currents in them are:
- thermoelectronic ionization–the Poole-Frenkel equation (coordinates ln(j/E)–√E);
- thermal excitation of charges (coordinates ln j−1/kT);
- impact ionization;
- injection from the electrode (SCLC) (coordinates lnj–lnE);
- thermoelectron emission (Schottky emission)–Richardson-Dashman equation, (coordinates ln j–√E);
- tunnel effect–the Fowler-Nordheim equation (coordinates ln(j/E)−1/√E);
- trap tunneling (field emission of electrons), coordinates ln (j/E2)−1/E).
Materials and Research Methods
Samples of double doping of aluminum and phosphorus TiO2 and samples of doped-aluminum TiO2 were synthesized by the sol-gel method. The alloying and structuring components were introduced into the freshly precipitated TiO2 sol during the synthesis process. Dried samples were annealed at Tan = 700°C (Murashkevich et al., 2017).
The ER-activity of the obtained samples of doped TiO2 was determined from the change in shear stress and current density of the ER fluids containing 20 wt.% of the dispersed phase in the dielectric oil, with a change in the electric field strength of up to 3.5 kV/mm. The shear stress (τ) and the current density (j) of the ER fluids were determined using a Physica MCR 301 rheometer from Anton Paar in a coaxial-cylindrical measuring cell with an inner cylinder diameter of 26.7 mm and an external cylinder of 28.9 mm. The maximum value of the permissible current density was 30 μA/cm2, the temperature varied from 5 to 100°C, and the shear rate varied from 0.1 to 100.0 s−1. The conduction mechanisms causing the leakage currents in the ER fluids were judged by the nature of the current-voltage characteristics (CVC) in the corresponding coordinates. Dielectric characteristics of ER fluids were determined using an E7-20 impedance meter, which allows measuring capacitance C and dielectric loss tangent tan δx in the frequency range of f from 25 Hz to 1 MHz (the measuring signal voltage used in the experiments is 1 V).
Results and Discussion
Dielectric spectra ε′(f) and ε″(f), dependences of current density (j) and shear stress (τ) on electric field strength (E) from 0 to 3.5 kV/mm when the temperature changes (T) were considered in determining the effect of double doping of TiO2 with aluminum and phosphorus on the electrophysical and rheological characteristics of the ER fluids. Sample ER fluid −1 (ERF-1) contains 20 wt.% TiO2, doped with aluminum and phosphorus expressed as elements, respectively, 10 mol.% Al and 1 mol.% P. Sample ER fluid −2 (ERF-2) contains 20 wt.% TiO2, doped with 10 mol.% Al.
The frequency dependences of the real (ε′) and imaginary (ε″) components of the complex dielectric constant (ε = ε′ − iε″) determine the features of polarization of the material under consideration. The polarization ability of particles is determined by the difference between the relative dielectric constant (ε′) at a frequency below and above the relaxation frequency fr, at which the dielectric loss factor is maximum (). Hao et al. (1998) determined that to achieve a high ER-effect, suspensions should have interfacial polarization, i.e., the relaxation frequency should be in the range of 0.1 to 1,000 kHz and at large values of the dielectric loss factor, the ER effect will be higher.
Figure 1 shows the dependences of the real (ε′) and imaginary (ε″) components of the dielectric constant of ERF-1 and ERF-2 on the frequency of the electric field.
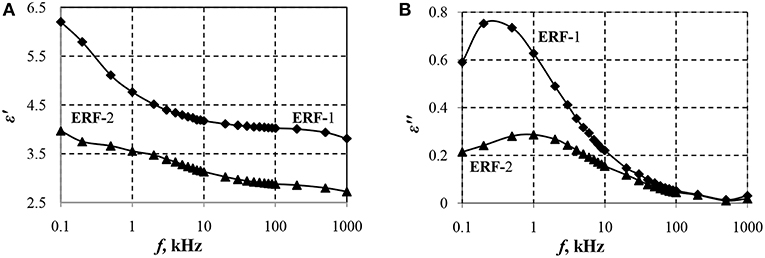
Figure 1. Dependences of the real ε′ (A) and imaginary ε″ (B) components of the dielectric constant of ERF-1 and ERF-2 on the field frequency.
Dependences ε′ (f) and ε″(f) confirm the presence of interfacial polarization in ERF-1 and ERF-2 (Figure 1). Double doping of aluminum and phosphorus TiO2 (ERF-1) increases Δε′ and ε″ at the relaxation frequency (ε″ERF−1 = 0.75 for frERF−1 ≈ 0.2 Hz; when frERF−2 ≈ 1.0 Hz), that increases the polarization ability of ERF-1 (Δε′ERF-1 > Δε′ERF-2).
Figure 2 shows the dependences of the current density and shear stress ERF-1 and ERF-2 on the electric field strength.
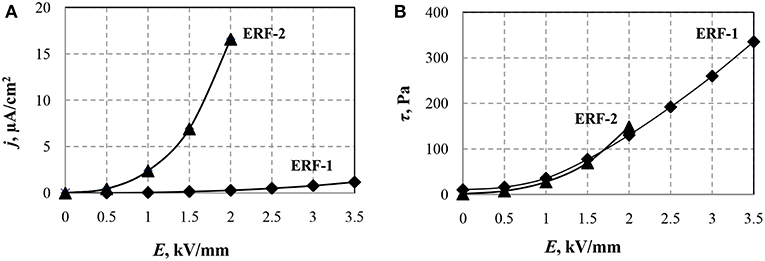
Figure 2. Dependencies of current density (A) and shear stress (B) of ERF-1 and ERF-2 on the electric field strength at a temperature of 20°C and shear rate of 100 s−1.
It can be seen from the above dependences that even at E > 2 kV/mm, significant leakage currents in the ERF-2 do not allow an increase in the electric field strength. The shear stress of the ERF-1 with double doping of aluminum and phosphorus TiO2 particles is two times greater than the maximum achievable for ERF-2 in the considered range of electric fields. The double doping leads to a significant decrease in the current ERF-1, which allows increasing the electric field strength.
The effect of the electric field strength on the current density and the shear stress of ERF-1 and ERF-2 with temperature changes are shown in Figure 3.
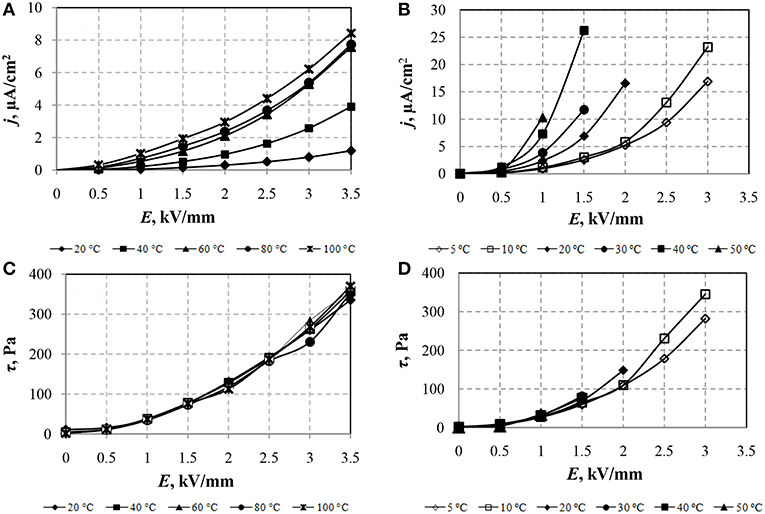
Figure 3. Dependences of current density (A,B) and shear stress (C,D) of ERF-1 (A,C) and ERF-2 (B,D) on the electric field strength with temperature change. Shear rate 100 s−1.
In Figures 3A,B, in which the CVC in the coordinates of the current density (j) from the electric field strength (E) are shown, it can be seen that heating the ER fluids samples increases the leakage current density in the entire range of variation of the electric field. Maximum value of current density in ERF-1 is three times less than in ERF-2. It is necessary to note the significant intensity of the current density increase in ERF-2 with increasing temperature at all electric field strengths compared with its smooth change for ERF-1.
Figure 3C shows that the shear stress of the ERF-1 almost does not change with increasing temperature, the current density of the ERF-1 decreases with a temperature decrease to 20°C (Figure 3A). The maximum value of the current density ERF-1 (8.8 μA/cm2) obtained at T = 100°C and E = 3.5 kV/mm. According to previously collected data (Yin and Zhao, 2001), the shear stress of Ce-doped TiO2 ER fluid increases with temperatures at 80°C and, with a further increase in temperature to 100°C, the shear stress of the ER fluid decreases.
To identify the conduction mechanisms causing the leakage currents of the ER fluids, the CVC constructed in different coordinates is to be considered. The presence of a mechanism of thermionic ionization in the formation of leakage currents can be determined by the type of dependences of the current—voltage characteristics in the coordinates ln (j/E) on √E, as shown in Figure 4.
Figure 4 shows that the currents of the ERF-1 are lower than the currents of the ERF-2. The CVCs in the coordinates ln (j /E) from √E for ERF-1 and ERF-2 have a linear character in electric field strength E ≥1 kV/mm. To determine the effect of heating on the increase in conductivity of the ER fluids, the experimental results are presented in the Arrhenius coordinate system ln J from 1/T, as shown in Figure 5.
The decrease leakage currents in ERF-1 compared to ERF-2 due to thermoelectric ionization (Figure 4) and thermal excitation (Figure 5) confirms the assumption of a decrease conductivity due to a decrease in the concentration of charge carriers. This is due to the joint presence of donor and acceptor impurities, as well as impurities of interstitial and substitution in the structure of TiO2. Figure 5 shows that the increase in current in ERF-1 is significantly influenced by the thermal excitation of charge carriers and their transfer to the conduction band when heated to 60°C (1/T = 0.003). With a further increase in temperature to 100°C (1/T = 0.00268), the current increases weakens, and in electric field strength E ≥ 2.0 kV/mm, the current increase is minor. The dependences in the Arrhenius coordinates for ERF-2 are linear in the whole range of variations of the electric field strength and temperature from 5 to 50°C.
To determine the space-charge-limited current (SCLC) created by electrons injected from the electrode in the ER fluid, the CVC in logarithmic coordinates lnj–lnE is to be considered (Figure 6).
Figure 6 shows that the CVCs for ERF-1 and ERF-2 are linear in the whole range of the electric field strength and temperature. The CVC for ERF-1 has a piecewise linear approximation at 200°C.
The SCLC in the ERF-1, associated with the trapping of electrons on traps, is significantly lowwe than in the ERF-2 and slightly changes with a temperature increase from 60 to 100°C. This result can be explained by the presence of a larger number of acceptor traps and their faster filling with increasing temperature in ERF-1 compared to ERF-2.
To identify the mechanism of conductivity in the ER fluid due to the thermoelectronic emission of Schottky, the experimental results are graphically presented in the coordinate system ln j from √E in Figure 7.
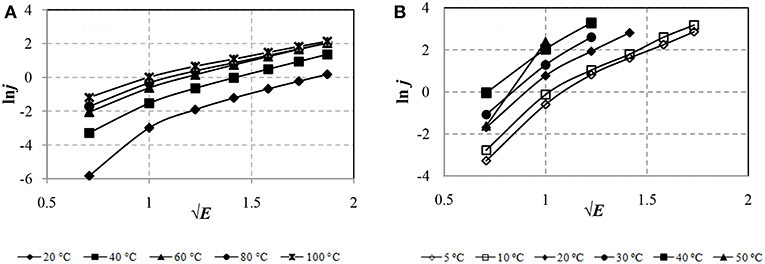
Figure 7. CVC of ERF-1 (A) and ERF-2 (B) 2 samples in event thermoelectronic emission (Schottky mechanism).
Figure 7 shows that the leakage currents of ERF-1 and ERF-2 in electric field strength E ≥1.0 kV / mm (√E = 1) are due to the Schottky mechanism (thermoelectronic emission), as the CVC is approximated by a linear dependence at E from 1.0 to 3.5 kV/mm (from √E = 1 to √E = 1.87) in the whole range of temperatures. ERF-1 currents are significantly lower than ERF-2 currents. It is possible that a decrease in the current due to the Schottky effect may be associated with an increase in the height of the metal-semiconductor interfacial potential barrier for aluminum and phosphorus double doped TiO2 (ERF-1) compared with only aluminum doped TiO2(ERF-2).
To identify the mechanism of conductivity due to field emission of electrons from a metal, Figure 8 presents the CVC of ERF-1 and ERF-2 in the Fowler-Nordheim coordinates.
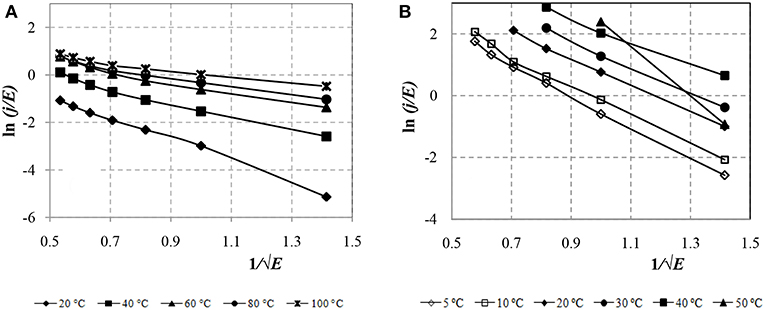
Figure 8. CVC of the ERF-1 (A) and ERF-2 (B) samples in event of field emission of electrons from the electrode metal.
Figure 8 shows that the conductivity of the ERF-1 and ERF-2 samples is due to the Fowler-Nordheim tunneling mechanism, since the CVC is approximated by a linear dependence. The double doping of TiO2 with aluminum and phosphorus reduces the leakage current in ERF-1 caused by the field emission of electrons from a metal.
To identify the mechanism of conduction of the field emission of electrons from the traps, the CVC is constructed in the coordinates ln (j/E2) from 1/E. Figure 9 shows the CVC characteristics of the ERF-1 and ERF-2 samples in the field emission coordinates at different temperatures.
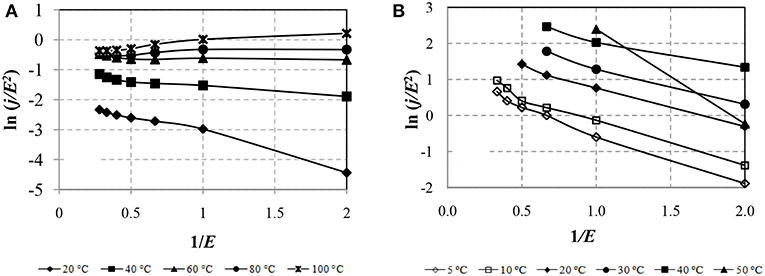
Figure 9. CVC of samples ERF-1 (A) and ERF-2 (B) in the coordinates of the field emission from the traps.
Figure 9 shows that the leakage currents of ERF-1 and ERF-2, are due to the conduction mechanism, known as field emission of electrons from traps, and the leakage currents of ERF-1 are much less at T ≥ 60°C and depend little on the electric field strength and temperature.
Analysis of the CVC of the materials under study made it possible to determine the mechanisms of formation (transfer) of charge (electron) in ER-fluids with semiconductor dispersed fillers with various modifications of their structure, which determine the rheological behavior of fluids on temperature changes (the impact mechanism is manifested with much stronger energy effects).
Double doping TiO2 with aluminum and phosphorus significantly reduces leakage currents in ERF with TiO2/Al/P compared to currents in ERF with TiO2/Al caused by all the above mentioned conduction mechanisms. This may be due to an increase in the number of TiO2 lattice defects during double doping, which are charge recombination centers, as well as an increase in the degree of recombination of electrons and holes, since the simultaneous introduction of donor and acceptor impurities leads to mutual compensation of charge carriers. In addition, the results obtained may be due to an increase in the metal-semiconductor interfacial potential barrier when using aluminum and phosphorus double doped TiO2 compared with the use of aluminum doped TiO2.
Conclusion
The results of the study of the rheological and electrophysical characteristics of the ER fluid with TiO2 particles doped with aluminum and phosphorus and their comparison with the characteristics of the ER fluid with TiO2 particles doped with aluminum alone, revealed the following regularities and behavioral features of ER fluids with semiconductor dispersed materials having modified crystal structures (anatase), in electric fields of direct current up to 3.5 kV/mm in the temperature range of 5–100°C:
- Double doping of TiO2/Al/P allowed to increase the value of ε′ in the ERF by 1.5 times compared to ERF with TiO2/Al, which indicates an increase in the polarization ability of the particles of the modified material of the dispersed phase.
- A comparison of the conduction currents of the considered EPF samples in the range of the electric field strength from 0 to 3.5 kV/mm showed that with double doping of TiO2/Al /P the leakage current is 30 times lower. This made it possible to increase the value of the electric field strength to 3.5 kV/mm, in contrast to the maximum achievable value in the ERF with TiO2/Al–only 2 kV/mm.
- Analysis of the results of measuring the shear stress in an electric field with a temperature change in the range from 20 to 100°C showed that all the dependences of the shear stress on the electric field strength τ (E) do not depend on temperature only for ERF with TiO2/Al/P.
- The determination of the linearity of the dependences of the CVC in the corresponding coordinates, which are characteristic for the measurement of semiconductors of materials, made it possible to determine the main mechanisms charge transfer induce in leakage currents in the samples of ER fluids. It has been established that when exposed to a strong electric field taking into account heating in an extended temperature range, leakage currents are induced by conduction mechanisms such as thermoelectronic ionization, electron injection from an electrode, thermal excitation of charges, thermoelectronic emission, field emission from a metal (tunnel effect), field emission of electrons traps (trap tunneling).
Author Contributions
EK conceived the study, revised the manuscript, and supervised the work. ZN performed the experiments, contributed to data analysis and manuscript review.
Conflict of Interest Statement
The authors declare that the research was conducted in the absence of any commercial or financial relationships that could be construed as a potential conflict of interest.
References
Böse, H., and Trendler, A. (2001). Comparison of rheological and electric properties of ER fluids based on different materials. Int. J. of Modern Phys. B. 15, 626–633. doi: 10.1142/S0217979201005088
Fowler, R. H., and Nordheim, L. (1928). Electron emission in intense electric fields. Proc. Roy. Soc. 109, 173–181. doi: 10.1098/rspa.1928.0091
Hao, T., Kawai, A., and Ikazaki, F. (1998). Mechanism of the electrorheological effect: evidence from the conductive, dielectric, and surface characteristics of water-free Electrorheological fluids. Langmuir 14, 1256–1262. doi: 10.1021/la971062e
Kofstad, P. (1972). Nonstoichiometry, Diffusion and Electrical Conductivity in Binary Metal Oxides. New York, NY: Wiley.
Korobko, E., Novikova, Z., Murashkevich, A., and Kharlamova, I. (2018). Effect of the filler composition on the Electrorheological response of dielectric suspensions in an enlarged temperature range. J. Intell. Mater. Syst. Struc. 29, 242–249. doi: 10.1177/1045389X17704073
Korobko, E. V., Novikova, Z. A., and Kharlamova, I. M. (2012). Structural and Rheological Features of the Behavior of Nanoscale Electrosensitive Dispersions. Preprint No. 1. Minsk: A. V. Luikov Heat and Mass Transfer Institute, National Academy of Sciences of Belarus.
Mitrofanov, V., and Phogel, V. (1965). Physics and Chemistry of Semiconductors. Leningrad: Shipbuilding.
Murashkevich, A. N., Alisenok, O. A., Zharsky, M. I., Korobko, E. V., and Novikova, Z. A. (2017). The effect of the conditions for producing nano-sized titanium dioxide modified with aluminum on the effectiveness of its use in electrorheological dispersions. Colloid J. 79, 65–72. doi: 10.1134/S1061933X17010100
Shang, Y. L., Jia, Y. L., Liao, F. H., Li, J. R., Li, M. X., Wang, J., et al. (2007). Preparation, microstructure and electrorheological property of nanosized TiO2 particle materials doped with metal oxides. J. Mater. Sci. 42, 2586–2590. doi: 10.1007/s10853-006-1336-5
Tang, H., He, J., and Jacques, P. (2010). Giant electrorheological effects of aluminum-doped TiO2 nanoparticles. Particuology 8, 442–446. doi: 10.1016/j.partic.2010.07.007
Xu, Y. Z., Liang, R. F., Hao, T., Zhang, Q. Z., Xu, Z. M., and Chen, Y. H. (1992). “Design of high performance dry electrorheological fluid,” in Proceedings of the International Conference ERF. (Singapore).
Yin, J. B., and Zhao, X. P. (2001). Temperature effect of rare earthdoped TiO2 electrorheological fluids. J. Phys. D Appl. Phys. 34, 2063–2067. doi: 10.1088/0022-3727/34/13/317
Yin, J. B., and Zhao, X. P. (2006). Enhanced electrorheological activity of mesoporous Cr-doped TiO2 from activated pore wall and high surface area. J. Phys. Chem. B 110, 12916–12925. doi: 10.1021/jp0554588
Keywords: electrorheological fluids, conductivity, electric field, ER-effect, double doped particles TiO2 particles
Citation: Korobko EV and Novikova ZA (2019) Features of the Mechanisms of Conductivity of the Electrorheological Fluids With Double Doped TiO2 Particles Under External Temperature Effects. Front. Mater. 6:132. doi: 10.3389/fmats.2019.00132
Received: 29 October 2018; Accepted: 20 May 2019;
Published: 07 June 2019.
Edited by:
Miao Yu, Chongqing University, ChinaReviewed by:
Xufeng Dong, Dalian University of Technology (DUT), ChinaJianbo Yin, Northwestern Polytechnical University, China
Copyright © 2019 Korobko and Novikova. This is an open-access article distributed under the terms of the Creative Commons Attribution License (CC BY). The use, distribution or reproduction in other forums is permitted, provided the original author(s) and the copyright owner(s) are credited and that the original publication in this journal is cited, in accordance with accepted academic practice. No use, distribution or reproduction is permitted which does not comply with these terms.
*Correspondence: Evguenia V. Korobko, ZXZrb3JvYmtvQGdtYWlsLmNvbQ==