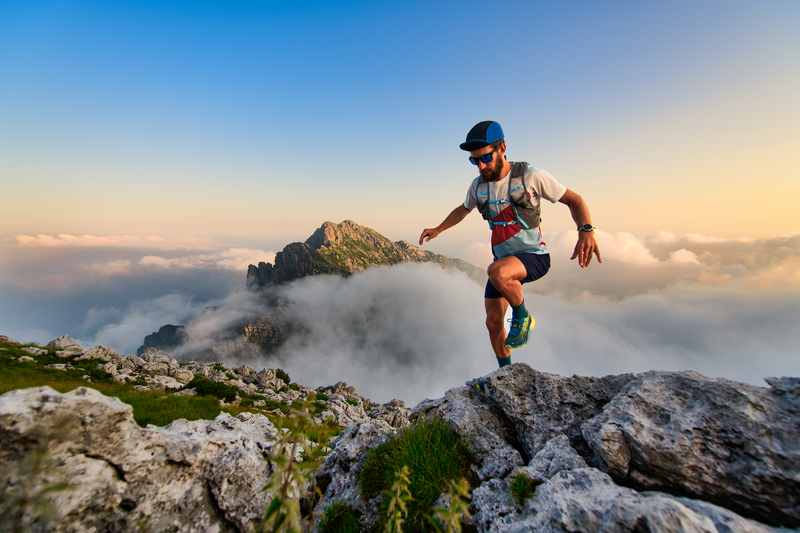
95% of researchers rate our articles as excellent or good
Learn more about the work of our research integrity team to safeguard the quality of each article we publish.
Find out more
ORIGINAL RESEARCH article
Front. Mater. , 22 March 2019
Sec. Smart Materials
Volume 6 - 2019 | https://doi.org/10.3389/fmats.2019.00045
This article is part of the Research Topic Controllable Electrorheological and Magnetorheological Materials View all 16 articles
Accurate, non-invasive measurements of blood pressure and its continuous monitoring are extremely important for personal health care. Arterial tonometry, a method that is used to provide a detailed image of a patient's cardiovascular health, shows promise for being a non-invasive alternative to current blood pressure measurement methods. However, its measurement accuracy is sensitive to patient variations such as the stiffness of the skin. Thus, this project intends to investigate the effect of skin properties (i.e., stiffness) on the accuracy of tonometric blood pressure measurements. To this end, a test platform, consisting of a pulsatile system and a tunable skin stiffness apparatus (or MR apparatus), is constructed. The cam-follower pulsatile system built based on in vivo testing of human pulses is used to generate realistic pulse waveforms. The MR apparatus is able to adjust its stiffness using Magneto-Rheological (MR) fluid whose apparent viscosity changes with applied magnetic fields. Placed at the surface of the MR apparatus, a cylinder with a frictionless plunger simulates a variable applanation force or “hold-down pressure” of tonometry by adjusting the added weights atop the cylinder. Using this test setup, a series of tests were performed by varying the input magnetic field and the weights, which effectively adjusts the skin stiffness and the hold-down pressure, respectively. The vertical displacement of the plunger caused by the internal pulse pressure was measured using a laser displacement sensor. The output displacement waveforms were analyzed with the focus on the peak amplitude difference of the waveforms, which is related to the augmentation index (a surrogate measure of arterial stiffness). The results show that there exists an “optimal” plunger weight or “hold-down pressure” that provides the most distinct output pulse waveforms. The results further show that the difference in the first two peak values decreases as the skin stiffness increases, indicating that the stiffer the skin property, the less the “hold-down pressure” effects on the accuracy of the tonometry measurements.
Human skin is a very complex organ that can be separated into three different layers; the epidermis, the dermis, and the hypodermis or subcutaneous tissue (Geerligs, 2006). All of these layers work to define the mechanical properties and characteristics of the skin. Due to the human skin being the main barrier in determining a patient's vitals, it is imperative that we understand its mechanical properties and how it affects the measurements of vital signals. Research into the human skin's mechanical properties will help in the creation of accurate, continuous, and non-invasive vital monitoring systems. Internal vital measurements, such as blood pressure, are extremely important in determining the health of a patient's cardiovascular system. In the United States, cardiovascular diseases have long been the leading cause of death. Hypertension is one of the most prevalent contributors to these diseases and has been known to increase the risk for all atherosclerotic cardiovascular diseases an average of 2 to 3-fold (Kannel, 1996). Currently, the “gold standard” for non-invasive blood pressure measurement is blood pressure “cuffs” (Chung et al., 2013). However, the need to constrict the arm with the inflated cuff can cause discomfort for the patient and make it less desirable for continuous monitoring. More importantly, cuffs only measure the systolic and diastolic values and brachial artery pressure, which do not provide a complete picture of a patient's internals (Smulyan et al., 2003; Singh et al., 2017). Furthermore, “white coat” hypertension, where a patient has high blood pressure due to anxiety during the measurement, has been known to increase a patient's blood pressure as much as 30 mmHg (Beevers et al., 2001). Due to these downsides of measuring blood pressure using cuffs, other ways of non-invasive measurement should be investigated.
Recently, the arterial tonometry method is being used widely as it overcomes limitations of the conventional cuff-based blood pressure measurements. The tonometry method places a pressure sensor on the surface of the skin where the radial artery is located to measure blood pressure. Unlike the cuff devices, which require pressurizations, the tonometry method applies a minimal pressure on the skin. Moreover, with tonometry, the actual arterial pulse waveform, augmentation index, and systolic and diastolic pressures can be continuously monitored which can provide better diagnoses of heart-related diseases (Smulyan et al., 2003; Tomiyama et al., 2014; Langwieser et al., 2015). Tonometry and its applications have shown great promise in the field of non-invasive continuous blood pressure monitoring. Langwieser et al. studied arterial blood pressure tonometry's use in cardiac intensive care. Usually, an invasive catheter is used to continuously measure arterial blood pressure. However, it was shown that arterial tonometry for intensive care patients is feasible and provided reasonable results when compared to the catheter method (Langwieser et al., 2015). Additionally, Digiglio et al. developed a method that uses a “microflotronic” arterial tonometry system. By utilizing a combination of microfluid and electronic devices, they successfully developed a completely non-invasive, nearly imperceptible device that is able to be worn on the wrist and provide continuous blood pressure monitoring (Digiglio et al., 2014).
While it has been proven that arterial tonometry is a viable replacement for current, invasive blood pressure monitoring techniques, there are some issues that need to be addressed. Arterial tonometry has been shown to be a very sensitive process that is accurate but can be unreliable (Weiss et al., 1996). If the device's contact with the skin over the artery is misaligned or even tilted slightly, the results can be affected. Furthermore, the precise measurement of blood pressure can be affected if the device places insufficient pressure on the artery (Shioya and Dohi, 2013). The mechanical properties of the skin will also affect the measurements read by the tonometer. Using an indentation test, which uses a method similar to a tonometer, it was shown that the skin responded differently for different age groups. For the same indentation force and speed, the response for the young people's skin was much more elastic than the response of the older people's skin (Zahouani et al., 2009). Since both the indentation test and arterial tonometry require a small external force application, the effect of a patient's age on indentation response may hold for the arterial tonometry as it did for the indentation test. This correlation is corroborated by Boyer et al. whose study showed, through in vivo indentation testing, that the stiffness and damping of the human skin decreased with increasing age (Boyer et al., 2009). In their computational work, Singh et al. studied the accuracy of tonometers and the problem of patient variability, specifically age, on radial artery tonometry to design new tonometers. It was found that the skin stiffness for age groups from 35–45 to 55–65 and the shape of the tonometer plunger were significant and must be accounted for in device calibration for tonometers (Singh et al., 2017). These studies suggest that the age-related change in skin properties may affect the results of arterial tonometry. Furthermore, accounting for the variability of the human body, specifically, the age-dependency of skin stiffness is critical to developing blood pressure monitoring devices.
Besides conventional arterial tonometer measurements, it is imperative to understand the role of skin property on wearable, non-invasive blood pressure monitoring devices. The wearable devices make constant contact with the skin during the measurement process, so the skin's properties play an important role in their measurement accuracy. When under stress, skin shows anisotropic and viscoelastic properties which would unusually distort the expansion of the artery and therefore external pressure measurements (Khatyr et al., 2004). Woo et al. studied a tissue-informative mechanism to obtain blood pressure measurements non-invasively using a wearable pressure sensor module. By modeling the subcutaneous tissue using a spring and damping constant, they found that the stiffness of the subcutaneous tissue changes with respect to average blood pressure. Using this relationship, they were then able to measure radial blood pressure without any additional pressure from the measurement device (Woo et al., 2014).
Despite the significance of skin property on blood pressure measurements, limited studies investigated the skin stiffness effect on arterial tonometry measurements. Furthermore, there exists a growing need for establishing a more holistic view of the skin stiffness effect on wearable, non-invasive blood pressure monitoring systems in order to develop such devices. Using human subjects for skin effect studies would be resource intensive and time-consuming. Therefore, this study intends to investigate the effect of skin stiffness on the arterial tonometry measurements. To this end, a test setup that is able to simulate the blood pulsations and variations of skin stiffness is created. To obtain meaningful results without involving human subjects, it is critical to have a component that can generate realistic human pulse waveforms and provide them consistently. In this study, a cam system is employed, which was created based on in vivo testing in a separate study, to provide “standard” pulse waveforms. With its mechanical simplicity and consistent motions, a cam system can provide the reference pulse waveforms required in this study. Another critical component is a tunable stiffness element. For the current study, a tunable stiffness apparatus was constructed based on Magneto-Rheological (MR) fluid. MR fluid is a controllable fluid, comprised of micron-sized iron particles suspended in a binding agent, often a type of oil. The apparent viscosity of MR fluids can be controlled by applying external magnetic fields. The ability to change the state of MR fluids, by the applied magnetic field, from liquid to semi-solid is fast (in milliseconds) and reversible. In the current study, the MR fluid is placed into a fluid container to create an artificially tunable skin stiffness apparatus. The pulses generated by the cam system are sent through a silicone tube that is submerged in MR fluid. Using an electromagnet, the stiffness of the MR fluid is varied. To simulate varying hold-down pressures of a tonometer, a cylinder with a frictionless plunger and a stack of weights is placed on top of the MR apparatus with the plunger resting on the tube surface. A precision laser sensor is used to measure the displacement of the plunger while varying the magnetic fields and weights. These “output pulse” data is compared with the actual pressure waveform in the tube to assess the effect of the skin stiffness effect of the tonometry measurements.
This section presents the design and fabrication of the test platform used in this study and the working principles of each element. The test platform has two main components: (1) the cam system and (2) the artificially tunable skin stiffness apparatus. The cam system was used to generate uniform, continuous reference waveforms and the tunable skin stiffness apparatus was used to adjust its stiffness.
In order to conduct this study, a device that is able to consistently and continuously reproduce a human pulse waveform was needed. To this end, a cam pulsation system is selected as a cost-effective way of generating a reference human pulse waveform. The cam system was designed and constructed in a separate study conducted by Yang et al. While details of the design and the performance validation of the cam system can be found in the reference (Yang et al., 2019), this section briefly explains how the system was created. Figure 1 shows the design process for developing a disk cam, the key element of the cam pulsation system. The shape of this cam was designed by collecting in vivo data of the pulse waveform and mapping the results to a circular pattern. The in vivo data was taken from the arterial pulse of a young, male subject using the radial pulse measurement system shown in Figure 1A. The pulse data taken was then averaged into the pulse waveform, which shows three distinct peaks as shown in Figure 1B, which shows the normalized blood pressure over a period of time. This pulse waveform was then converted to radial coordinates (see Figure 1C). Figure 1D shows the machined final cam based on the shape in radial coordinates.
Figure 1. (A) In vivo data collection using robotic tonometry system to get an example pulse waveform, (B) Normalized blood pressure of waveform vs. time, (C) Conversion of waveform into a radial geometry, (D) Manufactured cam disk from radial waveform (Yang et al., 2019).
Figure 2 shows the full assembly of the cam pulsation system, consisting of a cam, an electric motor, and a piston unit that includes a spring and bellows. When the cam rotates, it presses the bellows in such a way that a human pulse waveform is output to a silicone tube. The piston is kept in contact with the cam by a return spring. By moving this spring closer to the cam system, the pressure in the system can be shifted up and down. The cam system also includes a pressure sensor that collects the internal pressure of the system, which can be monitored on an oscilloscope. By varying the voltage supplied to the motor, the speed of the rotating cam is increased and the “heart rate” of the simulated pulse can be adjusted.
Another key component to conduct this study is a tunable stiffness apparatus. It will enable us to test the effect of skin stiffness on perceived blood pressure at the surface of the artificial skin. In this study, the tunable skin stiffness apparatus was designed based on MR fluid whose apparent properties can be controlled by external magnetic fields. The apparatus, shown in Figure 3A, has a container with a cavity in the center and a tube, which represents a radial artery, running across the middle. The tube is connected to the inlet shown on the outside of the container, stretched over the cavity, and connected to a plug on the right side. The inlet of the container is then connected to the cam system. The apparatus has a flexible membrane made of 3M VHBTM Tape stretched over the MR fluid-filled cavity, depicted in the top of Figure 3A. The apparatus was designed to be placed on an electromagnet. A power supply is connected to the electromagnet in order to supply up to 24 V to it. As the electromagnet is turned on, the fluid in the container becomes stiffer which uniformly constricts the tube, which is illustrated in Figure 3B. By increasing the magnetic field, one should be able to suppress the pulsation felt in the tubing that is submerged in the MR fluid.
Figure 3. Variable skin stiffness apparatus: (A) overhead view showing the apparatus assembled and disassembled, (B) Reaction in apparatus with and without magnetic field.
To study the magnetic field distribution in the MR apparatus, a simulation was conducted using the Finite Element Method Magnetics (FEMM) software. It is important to ensure that the electromagnet can activate the MR fluids with sufficiently strong and reasonably uniform magnetic fields. Figure 4A shows the simulation setup and results of the simulation in a cross section of the experimental setup shown in Figure 3A. The electromagnet was modeled using copper solenoid coils and an iron core. The MR apparatus is shown in the figure as the aluminum housing with iron at the center. Note that, in fabricating the MR apparatus, an iron core (or iron extension in Figure 4A) is used at its bottom portion that directly contacts with the electromagnet in order to direct maximum magnetic fields through the MR fluid. In order to increase the accuracy of the simulation, note also that the B-H curve of the MR fluid (MRF-140CG) was assigned in the cavity of the MR apparatus, where the MR fluid is. To further analyze the result, the magnetic flux density was taken across the black dashed line shown in Figure 4A. As shown in Figure 4B, the magnetic flux density values are ~40 ± 5 mT, having the maximum near the edge of the MR housing. The flux density drops drastically in the middle of the graph (between the distance 8 and 12 mm) because this is the place where the air filled tube is located. From the graph, the magnetic field was ~36 mT at the center of the magnet. These results corroborate the experimental measurements used in this study.
Figure 4. FEMM simulation results: (A) Contour plot depicting the simulated magnetic field, (B) Magnetic field strength along the sample line.
Figure 5 shows the plunger-cylinder system with a stack of weights. While the MR apparatus adjusts the stiffness level of the skin, the plunger-cylinder system is designed to vary the “hold-down pressure” by adding or removing the weights atop the plunger. As shown in Figure 5, the plunger-cylinder system is fixed to the top cover of the MR apparatus. The bottom of the frictionless plunger is placed on top of the apparatus (on top of the submerged tube). As the tube expands due to the pulse pressure, the plunger moves up and down. Thus, this displacement of the plunger is directly related to the perceived pressure at the surface measured by a tonometer. In other words, the tunable stiffness apparatus along with the plunger-cylinder setup can be used to control and test the effect of skin/tissue stiffness and hold down pressure on perceived pressure at the surface of the skin.
Figure 5. Frictionless plunger used in the experimental setup. Applanation force or “hold-down pressure” is varied by adjusting the weights atop the cylinder with a frictionless plunger.
In the current study, the MR apparatus is intended to vary a range of its stiffness similar to the age-dependent human skin stiffness variation. According to a study by Boyer et al. human skin stiffness values could range between ~20 N/m at the oldest to ~70 N/m at the youngest, about a 350% difference (Boyer et al., 2009). Thus, to justify the efficacy of the MR apparatus for the age-dependent stiffness study, the target stiffness range should be close to 350%. To evaluate the stiffness range of MR apparatus, a series of indentation testing was conducted on the MR device using a Dynamic Mechanical Analyzer (DMA). During the test, the force vs. indentation depth was measured while varying the magnetic field from 0 to 36 mT. The results show that the stiffness value produced by the MR device ranges between ~300 and ~900 N/m for the magnetic field range considered in this study. Although the actual stiffness values between human skin and “MR skin” are different, the percent range of stiffness of the MR device is 300%. The results show that the MR system is capable of producing a range of stiffness in proportion to the age-dependent skin stiffness variation. Thus, the MR apparatus is suitable to investigate the effect of skin stiffness on arterial tonometry measurements, considering the skin stiffness variation over time. Please note that mimicking the exact in vivo human skin properties is out of the scope of the current study.
The primary purpose of this experiment was to study the effect of stiffness of the skin by evaluating the difference between the perceived pressure at the surface of the submerged tube and the actual pressure being supplied by the cam system. While the pulse was being supplied by the cam system, the pressure inside of the tube was measured using two methods. One was using a pressure sensor connected directly to the cam system to measure the actual pressure. The second method was a plunger with adjustable weight fixed to the MR apparatus with the plunger head resting on the flexible membrane (silicone skin) and tube, similar to the method utilized by tonometers. A laser displacement sensor was used to measure the vertical displacement of the plunger, which will be used to indirectly determine the pressure in the submerged tube. By adding weights to the plunger, the “hold-down pressure” is effectively being increased. The actual pressure measured by the pressure sensor and the displacement measured by the laser were measured for analysis. Power was supplied to the laser, electromagnet, and cam system using a 30 V DC lab power supply.
The full experimental setup is depicted in Figure 6. The cam system (left) sends a simulated pulse to the tunable skin stiffness apparatus (the black cylinder on the right). The apparatus is placed on top of an electromagnet and the plunger is fastened on top. Finally, a laser sensor is placed above the piston to accurately measure its vertical displacement. Two variables were adjusted to determine their effect. The magnetic field applied to the electromagnet and the weight on top of the plunger were varied to determine the effect of skin stiffness and hold down pressure on the system.
Figure 6. Experimental test setup including a cam system, a silicon tube, a laser sensor, and the adjustable skin stiffness apparatus.
This section presents the experimental results obtained by varying the magnetic fields and the weights. By changing the weights, the effect of “hold-down” pressure was studied. To examine the effect of skin stiffness, the magnetic field was varied from 0 to 36 mT as measured using an axial gauss meter directly above the center of the electromagnet. The displacement of the plunger was measured and plotted for combinations of these changes. The displacement waveform results are compared with the reference pulse waveform. In particular, the distance between the first and second peak of the displacement response was analyzed for different weights and magnetic fields to assess the effect of stiffness.
In this section, the effect of “hold-down pressure” is studied to evaluate how the applied pressure affects the tonometry measurements. For this study, the mass load on the plunger is varied from 15 to 105 g in 15 g increments. No magnetic field was applied for these tests in order to establish a baseline understanding of the effect of hold down pressure. Each data point for all masses was normalized by dividing by the maximum displacement found across all tests. Figure 7 shows the normalized displacement waveforms of the system with varying mass loading. As shown in the figure, the waveforms increase as the mass increases from 15 to 75 g, and they decrease as the mass further increases. This pattern is attributed to the fact that as the mass on the plunger is increased, the silicone tube increasingly deforms. Therefore, when the tube expands to its original dimensions from the pressure supplied by the cam system, the displacement is larger with increased initial deformation of the tube. In this case, the 75 g load provided the largest displacement. When the mass is increased past 75 g and the pressure supplied by the cam system is insufficient to expand the tube and lift the plunger, the displacement decreases again. The results indicate that the measured blood pressure using a tonometer would change depending on the hold-down pressure of the tonometer. Furthermore, the results show that there exists an optimum hold pressure when measuring blood pressure using tonometric methods. These results are consistent with the computational work performed by Singh et al. who determined that there was an optimum hold-down level for the plunger when using radial artery tonometry (Singh et al., 2017). For the range of mass used in this study, the mass load of 75 g applies an “optimal” hold-down pressure on the artificial artery that enables most distinct pulse readings. This study suggests the patient-to-patient accuracy may be affected depending on the hold-down pressure used by the machine. For such a system to be used for blood pressure monitoring, the hold-down pressure must be calibrated for the patient's specific skin properties for truly accurate results.
Figure 7. Variations of the normalized displacement (pulse waveform) over time while changing the “hold-down” pressure by varying the mass from 15 to 105 g with an increment of 15 g.
This section evaluates the effect of tunable stiffness on pulse waveform measurements. For this part of the study, the cam system is supplied with 10 V to set the cycle time of each pulse at around 950 ms, which is about 60 beats per minute (bpm). This is because the average human heartbeat (or pulse) ranges from 60 to 80 bpm. Moreover, this study was performed at a fixed load of 75 g because it was found in Figure 7 that a 75 g load created the most pronounced displacement values.
Figure 8 shows the plunger displacement waveform results for a 75 g load with no magnetic field and with the maximum magnetic field of 36 mT. For comparison, these were plotted against the pressure measurements taken from the cam system's pressure sensor. The results show that the displacement waveform does not perfectly match the inner pressure due to the “skin effect.” The skin (the silicone membrane and MR fluid around the tube) filters the inner pressure signals (pulse waveform traveling in the tube). As shown in Figure 8, the inner pressure displays valleys after each peak, but the displacement response plateaus until it decreases to the next peak. This is attributed to the dampening of the MR skin. Furthermore, the figure shows that the displacement waveform decreased drastically with the maximum magnetic field (36 mT) as compared to that of the zero magnetic field case. The results indicate that the change in viscosity of the MR fluid successfully affected the displacement waveform and therefore is able to simulate the skin stiffness effect.
Figure 8. Pressure and normalized displacement vs. time for a 75 g load with and without an applied magnetic field.
It is clear to see that the application of the magnetic field had a large impact on the displacement of the plunger as well as the distance between peaks. Therefore, to study this effect further, more tests were run by incrementing the magnetic field from 0 to 36 mT by 6 mT steps. The results for a 75 g load with varying magnetic fields are shown in Figure 9. As shown in the figure, the displacement waveform decreases as the applied magnetic field increases, indicating that the stiffness of the MR skin surrounding the tube clearly affected the external displacement of the plunger at the surface. The figure further shows that the rate of decrease of the waveform magnitudes is larger at lower magnetic fields. At higher magnetic fields, the displacement response seems to approach a saturation point. Furthermore, the results show a more sluggish response, represented by the decrease in the slope of the initial rise as well as the decrease in the distance between the first and second peaks, or ΔP, with increasing magnetic field. Overall, the results indicate the displacement of the plunger (i.e., perceived pressure) was lower when the MR fluid around the tube was stiffer. These results can be related to several studies that report on the age-dependent human skin stiffness variation (i.e., the elastic modulus of skin decreases as the age increases). Thus, the accuracy of a tonometer will be affected by the stiffness of the patient's skin and therefore the age of the patient.
Figure 9. Variation of normalized displacement curves for a 75 g load with a varying magnetic field from 0 to 36 mT.
The most holistic view of this study was examined by finding the magnitude differences between the first and second peak (ΔP) of each waveform for 75, 90, and 105 g loads with increasing magnetic flux density. The ΔP-values can be related to the augmentation index (AI), which is a ratio of the first two peak magnitudes of pulse waveforms. Clinically, the AI is widely used as a surrogate marker for cardiovascular diseases. Figure 10 shows a variation of ΔP as the mass on the plunger (the hold down pressure) and the magnetic flux density (skin stiffness) vary. It is important to note that only masses above 75 g were considered for this analysis. This is due to the trend found in Figure 7. It was found that the displacement waveforms for masses above 75 g would mirror those for masses below 75 g. As shown in Figure 10, the differences in the peak displacement values (ΔP) were mostly proportional to increasing hold down pressure while being inversely proportional to the increasing magnetic field. The results further show that, as the magnetic field increases, the changes in ΔP for different hold down pressures became insignificant, with the peak displacements being almost identical at the maximum magnetic field of 36 mT. This would suggest that the hold-down pressure plays a higher role in accuracy when examining older patients with skin that is less stiff (lower magnetic fields cases for this study). On the same note, it suggests that for younger patients with stiffer skin (higher magnetic field cases), the hold-down pressures may not be an issue when using tonometers. With the older patients being at a higher risk of cardiac events, it is important to note that their results are affected most. Furthermore, while the trends are fairly consistent for each mass load, the curve of the 105 g load crosses over the 75 and 90 g curves after the 18 mT results. This is most likely attributed to experimental error and the post-processing of data. The experimental set up used may not have been accurate enough once the results began to converge toward one another. Also, as one can see in Figure 9, the second peak becomes more and more indeterminate as the magnetic field increases. Therefore, to combat this, an average of several points along the second peak was used to get an average of the second peak value. This may have caused the results to skew the trends after the 18 mT mark when greater precision was needed to accurately show the differences between the curves.
Figure 10. Variation of the first two peak differences as a function of magnetic flux density for the mass of 75, 90, and 105 g.
This study has investigated the effect of skin stiffness on arterial tonometry. To adjust the skin stiffness, a tunable skin stiffness apparatus was successfully created using Magneto-Rheological (MR) fluid and an electromagnet. This apparatus was implemented along with a cam system that was able to continuously provide a human radial pulse waveform obtained by in vivo testing. To simulate the hold down pressure of tonometers, a frictionless plunger was employed in the test setup. By varying the weight atop the plunger, the effective “hold-down pressure” of the system was able to be adjusted. The displacement of the plunger was monitored for changing both the magnetic field applied as well as the weight atop the plunger head. The results show that the accuracy of measurements taken by a tonometer can be affected by both skin stiffness as well as hold-down pressure. The results of this study indicate that the hold-down pressure of the machine, as well as the stiffness of the patient's skin, can skew the results of a tonometer. The results further showed that as the stiffness of the MR fluid decreased, the difference in the magnitude of the first and second peaks in the pulse waveform increased. These results imply that, as age increased and stiffness of the skin decreased, the accuracy of the arterial tonometry measurements would be affected more heavily by the “hold-down pressure.”
Beyond the arterial tonometry methods for blood pressure measurements, the study on the skin effect may have implications regarding Oriental Medicine (OM) or traditional Chinese Medicine. Pulse diagnosis in OM, which examines radial blood pulsations on the wrist using a “three finger” method, is used to predict internal problems in a patient and is thought to even be able to narrow down which organ is having an issue. This method is completely non-invasive and is believed by many to be very effective (Dharmananda, 2000). Arterial tonometry is very similar to the traditional Chinese “three finger” method in a sense that both methods use a small exterior pressure in order to measure and analyze the interior pressure of the artery. Consequently, the skin effect can play an important role in pulse diagnoses in OM as well.
All authors listed have made a substantial, direct and intellectual contribution to the work, and approved it for publication.
This work was supported by the Korea Institute of Oriental Medicine (grant no. K18022) funded by the Korean government. The financial support is greatly appreciated.
The authors declare that the research was conducted in the absence of any commercial or financial relationships that could be construed as a potential conflict of interest.
Beevers, G., Lip, G., and O'Brien, E. (2001). Blood pressure measurement. BMJ 323, 981–985. doi: 10.1136/bmj.322.7292.981
Boyer, G., Laquièze, L., Le Bot, A., Laquièze, S., and Zahouani, H. (2009). Dynamic indentation on human skin in vivo: ageing effects. Skin Res Technol. 15, 55–67. doi: 10.1111/j.1600-0846.2008.00324.x
Chung, E., Chen, G., Alexander, B., and Cannesson, M. (2013). Non-invasive continuous blood pressure monitoring: a review of current applications. Front. Med. 7, 91–101. doi: 10.1007/s11684-013-0239-5
Dharmananda, S. (2000). The Significance of Traditional Pulse Diagnosis in The Modern Practice of Chinese Medicine. Portland, OR: Institute for Traditional Medicine. Available online at: http://www.itmonline.org/arts/pulse.htm
Digiglio, P., Li, R., Wang, W., and Pan, T. (2014). Microflotronic arterial tonometry for continuous wearble non-invasive hemodynamic monitoring. Ann. Biomed. Eng. 42, 2278–2288. doi: 10.1007/s10439-014-1037-1
Geerligs, M. (2006). A literature review of the mechanical behavior of the stratum corneum, living epidermis and the subcutaneous fat tissue. Phillips Res. Technical Note: PR-TN 2006/00450.
Kannel, W. B. (1996). Blood pressure as a cardiovascular risk factor prevention and treatment. J. Am. Med. Assoc. 275, 1571–1576.
Khatyr, F., Imberdis, C., Vescovo, P., Varchon, D., and Lagarde, J. M. (2004). Model of the viscoelastic behaviour of skin in vivo and study of anisotropy. Skin Res. Technol. 10, 96–103. doi: 10.1111/j.1600-0846.2004.00057.x
Langwieser, N., Prechtl, L., Meidert, A. S., Hapfelmeier, A., Bradaric, C., Ibrahim, T., et al. (2015). Radial artery applanation tonometry for continuous noninvasive arterial blood pressure monitoring in the cardiac intensive care unit. Clin. Res. Cardiol. 104, 518–524. doi: 10.1007/s00392-015-0816-5
Shioya, K., and Dohi, T. (2013). “Blood pressure measurement device based on the arterial tonometry method with micro triaxial force sensor,” in 2389–92. United States: Transducers & Eurosensors XXVII (Barcelona). doi: 10.1109/Transducers.2013.6627287
Singh, P., Choudhury, M. I., Roy, S., and Prasad, A. (2017). Computational study to investigate effect of tonometer geometry and patient-specific variability on radial artery tonometry. J. Biomech. 58, 105–112. doi: 10.1016/j.jbiomech.2017.04.023
Smulyan, H., Siddiqui, D. S., Carlson, R. J., London, G. M., and Safar, M. E. (2003). Clinical utility of aortic pulses and pressures calculated from applanated radial-artery pulses. Hypertension 42, 150–155 doi: 10.1161/01.HYP.0000084051.34269.A9
Tomiyama, H., Odaira, M., Kimura, K., Matsumoto, C., Shiina, K., Eguchi, K., et al. (2014). Differences in effects of age and blood pressure on augmentation index. Am. J. Hypertens. 27:12. doi: 10.1093/ajh/hpu082
Weiss, B. M., Spahn, D. R., Rahmig, H., Rohling, R., and Pasch, T. (1996). Radial artery tonometry: moderately accurate but unpredictable technique of continuous non-invasive arterial pressure measurement. Br. J. Anaesth. 76, 405–411.
Woo, S. H., Choi, Y. Y., Kim, D. J., Bien, F., and Kim, J. J. (2014). Tissue-informative mechanism for wearable non-invasive continuous blood pressure monitoring. Sci. Rep. 4:6618. doi: 10.1038/srep06618
Yang, T. H., Jo, G., Koo, J. H., Woo, S. Y., Kim, J. U., and Kim, Y. M. (2019). A compact pulsatile simulator based on cam-follower mechanism for generating radial pulse waveforms. Biomed. Eng. Online 18:1. doi: 10.1186/s12938-018-0620-3
Zahouani, H., Pailler-Mattei, C., Sohm, B., Vargiolu, R., Cenizo, V., and Debret, R. (2009). Characterization of the mechanical properties of a dermal equivalent compared with human skin in vivo by indentation and static friction tests. Skin Res. Technol. 15, 68–76. doi: 10.1111/j.1600-0846.2008.00329.x
Keywords: magneto-rheological fluid, skin properties, blood pressure, sub-cutaneous tissue, pulse waveforms, tonometry
Citation: Coon A, Yang T-H, Kim Y-M, Kang H and Koo J-H (2019) Application of Magneto-Rheological Fluids for Investigating the Effect of Skin Properties on Arterial Tonometry Measurements. Front. Mater. 6:45. doi: 10.3389/fmats.2019.00045
Received: 01 December 2018; Accepted: 25 February 2019;
Published: 22 March 2019.
Edited by:
Seung-Bok Choi, Inha University, South KoreaReviewed by:
Young Choi, University of Maryland, United StatesCopyright © 2019 Coon, Yang, Kim, Kang and Koo. This is an open-access article distributed under the terms of the Creative Commons Attribution License (CC BY). The use, distribution or reproduction in other forums is permitted, provided the original author(s) and the copyright owner(s) are credited and that the original publication in this journal is cited, in accordance with accepted academic practice. No use, distribution or reproduction is permitted which does not comply with these terms.
*Correspondence: Jeong-Hoi Koo, a29vQG1pYW1pb2guZWR1
Disclaimer: All claims expressed in this article are solely those of the authors and do not necessarily represent those of their affiliated organizations, or those of the publisher, the editors and the reviewers. Any product that may be evaluated in this article or claim that may be made by its manufacturer is not guaranteed or endorsed by the publisher.
Research integrity at Frontiers
Learn more about the work of our research integrity team to safeguard the quality of each article we publish.