- 1Institute for Materials Research, Hasselt University, Diepenbeek, Belgium
- 2IMEC vzw Division IMOMEC, Diepenbeek, Belgium
- 3Soft Matter Physics Laboratory, Université Libre de Bruxelles, Campus La Plaine, Brussels, Belgium
Solid-supported lipid membranes are popular models that connect biological and artificial materials used in bio-technological applications. Controlling the lipid organization and the related functions of these model systems entails understanding and characterizing their phase behavior. Quartz crystal microbalance with dissipation monitoring (QCM-D) is an acoustic-based surface-sensitive technique which is widely used in bio-interfacial science of solid-supported lipid membranes. Its sensitivity to mass and energy dissipation changes at the solid-lipid layer-liquid interface allows the detection of phase transformations of solid-supported membrane geometries. In this perspective, we highlight this valuable feature and its related methodology, review current advances and briefly discuss future perspectives. Furthermore, a specific example is also provided on the ability of QCM-D to detect changes in lipid organization of cholesterol containing solid-supported lipid vesicle layers (SVLs) upon the addition of aspirin.
Introduction
Fundamental knowledge about membrane phases and their related structure and organization is crucial, since they are the physical frontier between the inner and outer parts of the cell. Many processes such as cellular adhesion or membrane fusion are controlled by the nature of the phases within cell membranes (Singer and Nicholson, 1972; Brown and London, 1998a,b; Simons and Vaz, 2004; Engelman, 2005). The main constituents of biological membranes are lipid bilayers with embedded proteins that add specific functionality to the cell membrane. Any given cell membrane consists of more than 100 different lipid species (Yeagle, 2005). The complexity of biological membranes makes simpler lipid bilayer structures useful model systems to study the membrane physico-chemical properties as well as interactions with other relevant biomolecules (Pokorny et al., 2006; Peetla et al., 2009; Kato et al., 2010; Brender et al., 2012).
In an aqueous environment, lipid molecules self-assemble by an entropy driven process into bilayer sheets, constituting the building blocks of biological membranes (Marsh, 2012). Their amphiphilic nature confers lipids a rich thermotropic and lyotropic phase behavior, characterized by their lateral organization, their molecular ordering and the mobility of the lipid molecules within the bilayer (Blicher et al., 2009; Eeman and Deleu, 2010; Hirst et al., 2011; Marsh, 2012). When temperature is the driving field of the transition, several mesophases can be encountered, and their existence and location in the phase diagram depend on the nature of the polar head group and on the length and degree of saturation of the alkyl chains. Lipid thermodynamics has been the subject of intense experimental and theoretical research, in an effort to link phase behavior and lateral organization of membranes to cell function mechanisms (Heimburg, 1998; Tristam-Nagle and Nagle, 2004; Almeida et al., 2005; Mouritsen, 2010). The literature on phase transitions of free standing lipid systems is vast, being mostly carried out using state-of-the-art characterization techniques such as differential scanning calorimetry (DSC) (Lewis et al., 1996; Heimburg, 1998; Ebel et al., 2001), nuclear magnetic resonance (NMR) (Watts and Spooner, 1991; Purusottam et al., 2015) X-ray scattering (Sun et al., 1994; Tristam-Nagle et al., 2002), and fluorescence microscopy (Zhao et al., 2007; Hirst et al., 2013).
The advent of nanotechnology raised the interest in different lipid geometries, like solid-supported bilayers (SLBs), supported layers of vesicles (SVLs) and supported lipid monolayers (SLMs). Solid-supported lipid membranes play an essential role in the development of sensor platforms, lipid bilayer-enveloped nanodevices and protocells (Yee et al., 2004; Castellana and Cremer, 2006; Liu et al., 2009; Kang et al., 2018). The latter are especially important for the future development and applications of lipid-functionalized nanoparticles for cancer and photodynamic therapy (Ashley et al., 2011; Rahman et al., 2014). The presence of the solid surface is believed to affect the organization of lipid membranes, specifically the phase behavior of SLBs. Upon the main phase transition, the substrate restricts lipid motion and decreases the energy difference between the two lipid phases (gel and disordered) as compared to free standing lipid films (Mangiarotti and Wilke, 2015). Phase behavior of solid-supported lipid membranes has been studied by several methods like fluorescence microscopy (Lin et al., 2006), atomic force microscopy (Attwood et al., 2013; Takahashi et al., 2016), and ellipsometry (Szmodis et al., 2010). Understanding the influence of the solid substrate on lipid film phases is essential for the design of lipid-functionalized multifunctional platforms. For instance, the influence of substrate topography on membrane organization is a practically unexplored issue limited to very few studies (see, for instance, Hoopes et al., 2011; Losada-Pérez et al., 2018).
In this context, quartz crystal microbalance with dissipation monitoring (QCM-D) has recently emerged and is steadily growing as a versatile technique to detect and characterize the phase behavior of different solid-supported lipid membrane geometries. In this perspective we will discuss the methodology and its usefulness, revise the current advances and provide a specific example of its use for more complex systems.
QCM-D and Lipid Membrane Phase Behavior
When an AC voltage is applied onto an AT-cut quartz crystal sandwiched between two electrodes, a mechanical deformation is induced and the crystal vibrates in thickness-shear mode. The shear waves will propagate as evanescent waves on the upper sensor surface and decay across the boundary between the crystal and the medium (air or liquid) (Johannsmann, 2015). The penetration depth δ of a 5 MHz shear wave in water is δ ~ 250 nm, rendering QCM-D surface-specific. Commercial QCM-D instruments measure both frequency and dissipation shifts, Δf and ΔD, of the quartz crystal upon the presence of an additional layer. Dissipation occurs when the driving voltage to the crystal is shut off and the energy from the oscillating crystal dissipates from the system. The energy dissipation of the sensor's oscillation is defined as D = Elost/2πEstored, the ratio between the dissipated energy during one vibration cycle and the total kinetic and potential energy of the crystal at that moment. Combined frequency and dissipation measurements make this technique very suitable for gravimetric and viscoelastic characterization of thin (nano/meso-scale) films. Excellent reviews on the working principle and data analysis can be found in Dixon (2008), Reviakine et al. (2011), Johannsmann (2015). In the last decade, QCM-D has been successfully used in biotechnology and environmental science as a tool for investigating the structure and mechanics of soft and solvated interfaces (Dixon, 2008; Kanazawa and Cho, 2009; Campos et al., 2015), as well as hydrodynamic phenomena in non-uniform nanostructured surfaces (Shpigel et al., 2018). It has been particularly relevant in the field of lipid biophysics, as a useful technique to understand the kinetics of formation of supported lipid bilayers (Keller and Kasemo, 1998; Richter et al., 2003; Cho et al., 2010; Lind and Cárdenas, 2016), and their interactions with relevant biomolecules (Mechler et al., 2007; Michanek et al., 2010; Nielsen and Otzen, 2013).
A recent application of QCM-D is detecting phase transitions within supported lipid layers of different geometries, namely, SLBs and SVLs. This feature is especially advantageous in since it does not require the use of labels, long temperature equilibration times or a large amount of sample. In liquid-mode, both Δf and ΔD vary with temperature following the viscosity and density temperature dependence of the liquid medium. Upon a first-order phase transition in the adsorbed lipid layer, both Δf and ΔD differ from their regular temperature dependence. The shape of the frequency and dissipation shift curves and their respective temperature derivatives are reminiscent of the enthalpy jump and its heat capacity derivative typically obtained from calorimetric measurements. Thus, extrema in the first-order derivative of frequency and dissipation curves stand as a token of the layer phase transitions. This approach is convenient since it does not require reference measurements with bare surfaces and enables an easy determination of the phase transition temperatures. A recent work by Reviakine and co-workers provides a comprehensive view of the mechanisms by which QCM-D detects lipid phase transitions and the related relative contribution of thickness, stiffness and hydrodynamic channels to the phase transition Δf and ΔD signatures (Peschel et al., 2016). Upon heating lipid bilayers in both SVLs and SLBs change from a thicker and stiffer gel phase to a thinner and less stiff liquid disordered one (see Figure 1A). In the case of thin and stiff SLBs the dominant mechanisms by which QCM-D detects the transition are related to the change in thickness and changes in the viscoelastic properties of the SLBs. In thicker and softer SVLs, additional hydrodynamic effects related to changes in the geometry of adsorbed vesicles enter at play. As a consequence, the size of the maximum in SVLs is several orders of magnitude larger than the one observed in SLBs. Figure 1B displays the temperature dependence frequency shift for a SVL at the main transition of dipamitoylphosphatidylcholine (DPPC), a well-known lung surfactant, upon heating at a rate of 0.2°C/min. DPPC melting is reversible upon cooling and a typical hysteretic behavior characteristic of first-order phase transitions is observed (see Figure 1C). Figures 1D,E show how the transition parameters in SVLs (peak maximum corresponding to the transition temperature, and peak width) are nearly overtone-independent for frequency and dissipation derivatives. In turn, in SLBs the transition parameters are overtone dependent due to a stronger opposing effect between stiffness and thickness contributions, as compared to SVLs, where this competition is counterbalanced by hydrodynamic dissipation changes (see for instance, Peschel et al., 2016).
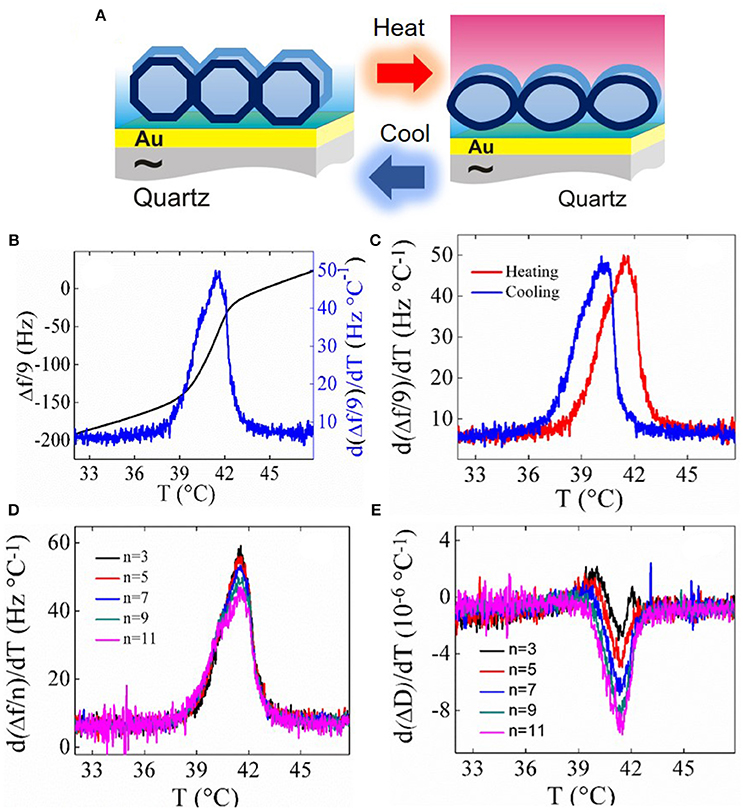
Figure 1. (A) Schematic of changes in SVLs upon the main phase transition (B) temperature dependence of the frequency shift d(Δf /9)/dT (black solid line) and its first-order derivative (blue solid line) of a DPPC SVL upon heating at 0.2°C/min. (C) Hysteresis observed between heating and cooling runs at the same rate. (D) Overtone dependence of d(Δf /n)/dT and (E) overtone dependence of dΔD/dT.
Recent Advances in Lipid Phase Behavior by QCM-D
The use of QCM-D as a technique to monitor phase transitions in biomimetic lipid layers was initially proposed by Ohlsson et al. (2011). The authors measured frequency and dissipation shifts at different overtones for supported vesicle layers of ditricosanoylglycerophosphocholine (DTPC) while performing heating and cooling cycles. The frequency and dissipation signatures were later fitted to a Voigt-based viscoelastic model (Voinova et al., 1999) extracting effective viscosity and thickness of the vesicle layers. Reversible anomalous changes in effective viscosity and thickness were observed for SVLs at around the expected main phase transition temperature. In contrast, bare reference surfaces exposed to the same buffer display a linear temperature dependence owing to linear frequency and dissipation coefficient of the sensor and the regular temperature dependence of the density and viscosity of the buffer. Upon heating, the transition from a high to a low-viscosity state agrees with the gel to liquid disordered phase transition. Interestingly, the authors carried out a systematic study to explore the influence of the substrate by changing the liquid medium conditions (addition of divalent ions and pH change). They concluded that, despite small shifts in transition temperature and layer thickness were observed upon a change of liquid medium conditions, SVLs transitions are predominantly determined by the part of the membrane that is not in contact with the solid substrate. The Voigt-based model approach was later applied to monitor the main phase transition for SVLs of DPPC on gold (Losada-Pérez et al., 2014) and to map phase diagrams of SVLs containing mixtures of phospholipids with different alkyl chains DPPC, DMPC and DSPC (Losada-Pérez et al., 2015b) showing a very good agreement with previous calorimetric measurements of free-standing lipid vesicles (Mabrey and Sturtevant, 1976; Fidorra et al., 2009). However, caution should be taken when analyzing the phase behavior of non-fully continuous supported lipid layers using a Voigt-based model, since this model assumes the presence of a homogeneous viscoelastic film (Johannsmaan et al., 2009).
QCM-D was later applied to study the phase transitions of SLBs and SVLs following the derivative approach described in the previous section. Wagernau and Tufenkji proposed the frequency derivative to detect the main transition in SLBs of pure phospholipids (Wargenau and Tufenkji, 2014), showing clear peaks d(Δf /n)/dT at the main phase transition of DMPC. Pramanik et al. applied this approach to SVLs containing negatively charged phospholipids exposed to aqueous buffers of different ionic strength, showing that the main transition temperature increases with increasing NaCl salt concentration. In addition, the authors found that QCM-D is sensitive to an additional transition arising from long time annealing at low temperatures of negatively charged SVLs of dimyristoylphosphatidylserine (DMPS) (Pramanik et al., 2016). The additional transition appears at low temperatures between the highly-ordered lamellar crystalline (Lc) and the lamellar gel (Lβ) phases, as previously observed for free-standing systems by calorimetric and FT-IR measurements (Lewis and McElhany, 2000). Hasan et al. extended the study to phase transitions in binary mixtures of phospholipids DMPC and DPPC for both SLBs and SVLs. Their results revealed that even for such a small alkyl chain difference (two ethyl groups), coexisting nanodomains (DPPC-rich, DMPC-rich, and rich in the lipid mixture) could be still detected in SLBs (Hasan and Mechler, 2017). An interesting testing ground of lipid phase transitions are mixtures of phospholipids with cholesterol, that exhibit an intermediate phase, the liquid ordered phase, whose existence plays an essential role for the formulation of the so-called lipid raft hypothesis (Simons and Ikonen, 1997; Mouritsen, 2010). Mixtures of DMPC with cholesterol were studied using both SLBs and SVLs, revealing the presence of cholesterol-rich and cholesterol-poor domains at low cholesterol concentrations. In both lipid geometries the gradual change in shape and size of the derivative peaks upon increasing cholesterol concentration reflected a loss of cooperativity, indicating the formation of a homogeneous liquid-ordered phase. For SLBs the main transition is suppressed over 10% cholesterol content in pure DI water (Hasan and Mechler, 2015), while for SVLs the main transition was still observed at 20% cholesterol content in HEPES buffer (Losada-Pérez et al., 2015a). Such large difference in cholesterol concentration threshold for main transition suppression between SLBs and SVLs reflects the strong influence of the presence of ions on the phase transition behavior of lipid membranes and the influence of supported lipid layer geometry.
The study of supported membranes phase behavior by QCM-D was also recently employed to evaluate disruption of SLBs and SVLs using the phase transition temperature as an indicator of the extent of membrane interactions with peptides or drugs. Specifically, a model toxicity study of imidazolium-based ionic liquids, considered as environmentally friendly organic solvents, was carried out for a SVL of DMPC. QCM-D revealed that interactions with a model SVL are mainly driven by the hydrophobic components of the ionic liquid, the longer the hydrophobic chain, the larger the decrease in phase transition temperature and larger membrane disorder by weakening phospholipid chain interactions (Losada Pérez et al., 2016). Another useful application of QCM-D phase transition studies is the screening of model anesthetics, such as ethanol. By exposing supported SLBs and SVLs of DMPC to increasing ethanol concentration, a gradual decrease in the main transition temperature and transition cooperativity is observed, making the derivative approach a straightforward method for biosensing purposes (Peschel et al., 2016).
Effect of Aspirin in the Phase Behavior of Cholesterol-Containing Phospholipids
Membrane fluidity has implications in biological processes by allowing diffusion of small molecules and lateral diffusion of proteins. Fluidity also controls membrane mechanical properties and thus the ability to form pores. Cholesterol is a well-known membrane fluidity regulator and has the ability to control the lateral organization of membranes, providing mechanical strength by increasing membrane stiffness. At high concentrations, cholesterol partitions unevenly within the hydrophobic core of phospholipid bilayers, leading to cholesterol-rich domains (Barrett et al., 2013). At very high concentrations (>40%) immiscible cholesterol bilayers can form, acting as a risk factor for high blood pressure and hypertension (Alsop et al., 2014). Conversely, aspirin counteracts cholesterol's condensing effect, inhibiting the formation of cholesterol rich-domains by residing in the head group region (Barrett et al., 2012; Alsop et al., 2014). A recent calorimetric and diffraction study has shown that aspirin inhibits the formation of the liquid ordered phase in free-standing multilamellar vesicles of DPPC containing a high cholesterol concentration (Alsop et al., 2015). Here we show that this effect is also observed by QCM-D for solid-supported SVLs containing a similar amount of cholesterol. DPPC and cholesterol were purchased from Avanti Polar Lipids (Alabaster, AL) and aspirin (ASA) (O-Acetylsalicylic acid, 99%) from Alfa Aesar (Thermo Fischer Scientific). Spectroscopic grade chloroform (assay 99.3% stabilized with about 0.6% ethanol) was obtained from Analar (Normapur). HEPES buffer (pH 7.4) consisting of 10 mM HEPES from Fisher Scientific (assay 99%) and 150 mM NaCl from Sigma-Aldrich (assay ≥99.5%) was used for hydration of the dried lipids. The quantities of lipids to reach the desired mixture concentrations were determined gravimetrically using a Sartorius balance yielding a maximal mole fraction uncertainty of ± 0.002. Vesicle Preparation. The lipid or lipid mixture was first dissolved in spectroscopic grade chloroform and the solvent was then evaporated under a mild flow of nitrogen in a round bottomed flask. The resulting lipid film was kept under vacuum overnight to remove residual solvent. Then, the lipid was hydrated with HEPES buffer and in the case of DPPC:chol mixture with buffer containing 6 mM aspirin. Hydration to 1 mg/mL was carried out under continuous stirring in a temperature controlled water bath at 60°C. Small unilamellar vesicles (SUVs) were formed by extrusion through a filter support (Avanti Polar Lipids) with a pore size of 100 nm for 25 times. Vesicle effective sizes and polydispersities were determined by dynamic light scattering (Zeta Pals, Brookhaven Instruments Corporation). The obtained average diameter was ~150 ± 40 nm. QCM-D experiments were performed as follows. First, a baseline with pure HEPES buffer was established at 16°C and afterwards lipid vesicles were injected over the sensor chip with a flow rate of 50 μL/min. After 20 min the pump was switched off. Subsequent temperature scans with alternating heating and cooling were performed at a rate of 0.2°C/min, maintaining 60 min of stabilization between successive ramps. Although not shown here, vesicle adsorption onto gold-coated quartz crystals yielded similar Δf and dissipation values (Δf /9 ~ −300 Hz and ΔD ~ 50) for all DPPC, DPPC+32.5 mol% cholesterol hydrated with buffer and DPPC + 32.5 mol% cholesterol hydrated in buffer containing 6 mM aspirin systems. Figure 2A displays the temperature dependence of d(Δf /n)/dT (9th overtone) for SVLs supported on a gold-coated quartz substrate, consisting of pure DPPC and DPPC + 32.5 mol% cholesterol with and without 6 mM aspirin. DPPC SVLs display a large maximum in d(Δf /n)/dT as a signature of the main phase transition at Tm ~ 41.5°C. This temperature agrees well with previous differential scanning calorimetric measurements of multilamellar DPPC vesicle dispersions (Tm ~ 41.6°C) (Benesch et al., 2011; Redondo-Morata et al., 2012). This temperature is slightly lower than the observed for planar supported lipid bilayers using AFM and ellipsometry (Leonenko et al., 2004; Redondo-Morata et al., 2012; González-Henríquez et al., 2017), since the presence of the solid surfaces induces a stronger decoupling between the two lipid leaflets during melting, as compared to 3-D supported vesicle layers and multilamellar vesicle dispersions. In SVLs containing 32.5% mole fraction of cholesterol no transition is detectable, indicating the presence of a homogeneous liquid ordered phase, in agreement with previous calorimetric measurements of multilamellar DPPC + chol vesicles (Mannock et al., 2010). The addition of 6 mM aspirin to systems of DPPC + 32.5% cholesterol leads to the appearance of a new transition (small peak). The peak is broader than that taking place the main transition of DPPC, indicating that the transition is less cooperative, and takes place at a higher temperature. Figure 2B shows the transition peak upon heating for the overtones measured. The transition parameters (temperature and peak width) are nearly overtone independent, as observed for SVLs of DPPC in previous works using QCM-D (Peschel et al., 2016). Subsequent heating and cooling runs show that the transition is reversible and takes place within the same temperature range considering the hysteresis between heating and cooling (see Figure 2C).
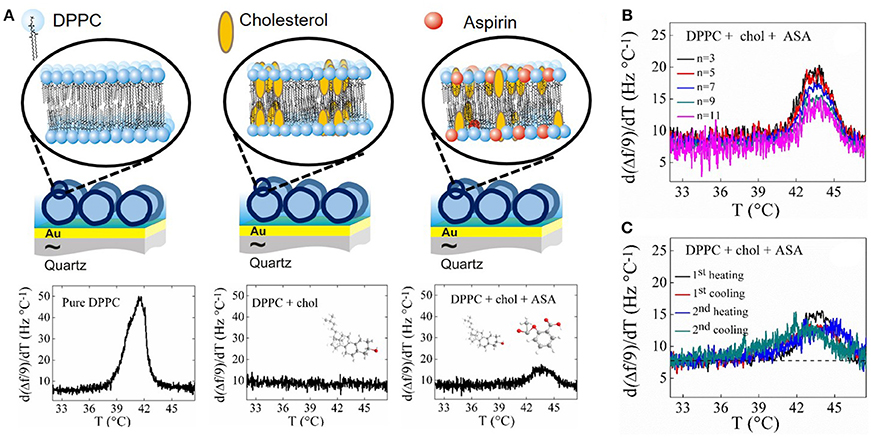
Figure 2. (A) Schematic of a SVL (not to scale) on a Au-coated quartz crystal including the temperature profiles upon the first heating run of d(Δf /9)/dT and dΔD/dT for SVLs of pure DPPC, DPPC + 32.5% cholesterol and DPPC + 32.5% cholesterol with 6 mM aspirin. (B) Overtone dependence of d(Δf /n)/dT for DPPC + 32.5% cholesterol with 6 mM aspirin SVL at the phase transition. (C) d(Δf /n)/dT response upon successive heating and cooling runs for SVL of DPPC + 32.5% cholesterol with 6 mM aspirin.
Conclusion
We highlight a simple approach for the detection of phase transitions in lipid membranes adsorbed to solid substrates using QCM-D. The mechanisms behind the detection of phase transitions rely on changes in film thickness and viscoelastic properties, which translate in changes in frequency and dissipation shifts. This allows the detection of phase transformations in very thin 2D-SLBs and 3D-SVLs. For the latter, the phase transition signatures are more evident in supported vesicle layers, owing to additional hydrodynamic effect arising from changes in their geometry. Although quite recent, this QCM-D feature has proven useful in detecting phase transitions in pure phospholipids and in mapping phase diagrams in binary phospholipid mixtures. Changes in the phase behavior of phospholipid membranes monitored by QCM-D have been also used to evaluate model membrane interactions with peptides, ionic liquids and model anesthetics, relevant in membrane toxicity and biosensing studies. As shown in this perspective, QCM-D is capable of detecting subtle changes in membrane organization induced by the addition of aspirin on cholesterol containing phospholipid membranes. There is plenty of room in fundamental biointerfacial science to apply the methodology described. For instance, exploring the existence of frequently elusive transitions in solid-supported membranes, like the ripple phase, extending phase transitions in complex multicomponent lipid mixtures and proteoliposomes with membrane proteins inserted, or evaluating the effect of the topology of nanostructured surfaces on supported lipid organization. The study of equilibrium phase transitions by QCM-D provides a solid starting point for the development of approaches that envisage dynamic, non-equilibrium phenomena in the presence of temperature gradients or osmotic stress that have actual relevance in cellular regulation processes.
Author Contributions
All authors co-wrote the submitted perspective. PL-P and FR searched the literature on the topic review current advances and briefly discuss future perspectives. SN, YD, and PL-P prepared the lipid vesicle samples and performed the experiments shown using QCM-D.
Conflict of Interest Statement
The authors declare that the research was conducted in the absence of any commercial or financial relationships that could be construed as a potential conflict of interest.
References
Almeida, P. F., Pokorny, A., and Hinderliter, A. (2005). Thermodynamics of membrane domains. Biochim. Biophys. Acta Biomembr. 1720, 1–13. doi: 10.1016/j.bbamem.2005.12.004
Alsop, R. J., Barrett, M. A., Zheng, S., Dies, H., and Rheinstädter, M. C. (2014). Acetylsalicylic acid (ASA) increases the solubility of cholesterol when incorporated in lipid membranes. Soft Matter 10, 4275–4286. doi: 10.1039/C4SM00372A
Alsop, R. J., Toppozini, R., Marquardt, D., Kucerka, N., Harroun, T. A., and Rheinstädter, M. C. (2015). Aspirin inhibits formation of cholesterol rafts in fluid lipid membranes. Biochim. Biophys. Acta 1848, 805–812. doi: 10.1016/j.bbamem.2014.11.023
Ashley, C. E., Carnes, E. C., Phillips, G. K., Padilla, D., Durfee, P. N., Brown, P. A., et al. (2011). The targeted delivery of multicomponent cargos to cancer cells via nanoporous particle-supported lipid bilayers. Nat. Mater. 10, 389–397. doi: 10.1038/nmat2992
Attwood, S. J., Choi, Y., and Leonenko, Z. (2013). Preparation of DOPC and DPPC supported planar lipid bilayers for atomic force microscopy and atomic force spectroscopy. Int. J. Mol. Sci. 14, 3514–3539. doi: 10.3390/ijms14023514
Barrett, M. A., Zheng, S., Roshankar, G., Alsop, R. J., Belanger, R. K., Huynh, C., et al. (2012). Interaction of aspirin (acetylsalicylic acid) with lipid membranes. PLoS ONE 7:e34357. doi: 10.1371/journal.pone.0034357
Barrett, M. A., Zheng, S., Toppozini, R., Alsop, R. J., Dies, H., Wang, A., et al. (2013). Solubility of cholesterol in lipid membranes and the formation of immiscible cholesterol plaques at high cholesterol concentration. Soft Matter 9, 9342–9351. doi: 10.1039/c3sm50700a
Benesch, M. G., Mannock, D. A., Lewis, R. N., and McElhany, R. N. (2011). A calorimetric and spectroscopic comparison of lathosterol and cholesterol on the thermotropic phase behavior and organization of dipamitoylphosphatidylcholine bilayer membranes. Biochemistry 50, 9982–9997. doi: 10.1021/bi200721j
Blicher, A., Wodzinska, K., Fidorra, M., Winterhalter, M., and Heimburg, T. (2009). The temperature dependence of lipid membrane permeability, its quantized nature, and the influence of anesthetics. Biophys. J. 96, 4581–4591. doi: 10.1016/j.bpj.2009.01.062
Brender, J., Salamekh, S., and Ramamoorthy, A. (2012). Membrane-disruption and early events in the aggregation of the diabetes related peptide IAPP from a molecular perspective. Acc. Chem. Res. 116, 3650–3658. doi: 10.1021/ar200189b
Brown, D. A., and London, E. (1998a). Structure and origin of ordered lipid domains in biological membranes. J. Membr. Biol. 164, 103–114. doi: 10.1007/s002329900397
Brown, D. A., and London, E. (1998b). Function of lipid rafts in biological membranes. Annu. Rev. Cell. Dev. Biol. 14, 111–136. doi: 10.1146/annurev.cellbio.14.1.111
Campos, J., Jiménez, C., Trigo, C., Ibarra, P., Rana, D., Thiruganesh, R., et al. (2015). Quartz crystal microbalance with dissipation monitoring: a powerful tool for bionanoscience and drug discovery. J. Biosc. 9, 249–260. doi: 10.1166/jbns.2015.1310
Castellana, E. T., and Cremer, P. S. (2006). Solid supported lipid bilayers: from biophysical studies to sensor design. Surf. Sci. Rep. 61, 429–444. doi: 10.1016/j.surfrep.2006.06.001
Cho, N. J., Frank, C. W., Kasemo, B., and Höök, F. (2010). Quartz crystal microbalance with dissipation monitoring of supported lipid bilayers on various substrates. Nat. Protoc. 5, 1096–1106. doi: 10.1038/nprot.2010.65
Dixon, M. C. (2008). Quartz crystal microbalance with dissipation monitoring: enabling real-time characterization of biological materials and their interactions. J. Biomol. Tech. 19, 151–158.
Ebel, H., Grabitz, P., and Heimburg, T. (2001). Enthalpy and volume changes in lipid membranes. I. the proportionality of heat and volume changes in the lipid melting transition and its implication for the elastic constants. J. Phys. Chem. B 105, 7353–7360. doi: 10.1021/jp010515s
Eeman, M., and Deleu, M. (2010). From biological membranes to biomimetic model membranes. Biotechnol. Agron. Soc. Environ. 14, 719–736. Available online at: http://www.pressesagro.be/base/text/v14n4/719.pdf
Engelman, D. M. (2005). Membranes are more mosaic than fluid. Nature 438, 578–580. doi: 10.1038/nature04394
Fidorra, M., Heimburg, T., and Seeger, H. M. (2009). Melting of individual lipid components in binary mixtures studied by FTIR spectroscopy, DSC and Monte Carlo simulations. Biochim. Biophys. Acta 1788, 600–607. doi: 10.1016/j.bbamem.2008.12.003
González-Henríquez, C. M., Villegas-Opazo, V. A., Sagredo-Oyarce, D. H., Sarabia-Vallejos, M. A., and Terraza, C. A. (2017). Thermal response analysis of phospholipid bilayers using ellipsometric techniques. Biosensors 7:E34. doi: 10.3390/bios7030034
Hasan, Y. I., and Mechler, A. (2015). Viscoelastic changes measured in partially suspended single bilayer membranes. Soft Matter 11, 5571–5579. doi: 10.1039/C5SM00278H
Hasan, Y. I., and Mechler, A. (2017). Nanoviscosity measurements revealing domain formation in biomimetic membranes. Anal. Chem. 89, 1855–1862. doi: 10.1021/acs.analchem.6b04256
Heimburg, T. (1998). Mechanical aspects of lipid thermodynamics. Estimation of the mechanical properties close to the chain melting transition from calorimetry. Biochim. Biophys. Acta Biomembr. 1415, 147–162. doi: 10.1016/S0005-2736(98)00189-8
Hirst, L. S., Ossowski, A., Fraser, M., Geng, J., Selinger, J. V., and Selinger, R. L. (2013). Morphology transition in lipid vesicles due to in-plane order and topological defects. Proc. Natl. Acad. Sci. U. S. A. 110, 3242–3247. doi: 10.1073/pnas.1213994110
Hirst, L., Uppamoochikkal, P., and Lor, C. (2011). Phase separation and critical phenomena in biomimetic ternary lipid mixtures. Liq. Cryst. 38, 1735–1747. doi: 10.1080/02678292.2011.615947
Hoopes, M. I., Faller, R., and Longo, M. L. (2011). Lipid domain depletion at small localized bends imposed by a step geometry. Langmuir 27, 2783–2788. doi: 10.1021/la104504p
Johannsmaan, D., Reviakine, I., and Richter, R. P. (2009). Dissipation in films of adsorbed nanospheres studied by quartz crystal microbalance (QCM). Anal. Chem. 81, 8167–8176. doi: 10.1021/ac901381z
Johannsmann, D. (ed.). (2015). “The quartz crystal microbalance in soft matter research,” in Fundamentals and Modelling (Switzerland: Springer). Available online at: https://books.google.be/books?id=IkAqBAAAQBAJ&printsec=frontcover&hl=es#v=onepage&q&f=false
Kanazawa, K., and Cho, N. J. (2009). Quartz crystal microbalance as a sensor to characterize macromolecular assembly dynamics. J. Sensors 2009:824947. doi: 10.1155/2009/824947
Kang, M., Tuteja, M., Centrone, A., Topgaard, D., and Leal, C. (2018). Nanostructured lipid based films for substrate-mediated applications in biotechnology. Adv. Funct. Mater. 28:1704356. doi: 10.1002/adfm.201704356
Kato, A., Tsuji, A., Yanagisawa, M., Saeki, D., Juni, K., Morimoto, Y., et al. (2010). Phase separation on a phospholipid membrane inducing a characteristic localization of DNA accompanied by its structural transition. J. Phys. Chem. Lett. 1, 3391–3395. doi: 10.1021/jz101376m
Keller, C. A., and Kasemo, B. (1998). Surface specific kinetics of vesicle adsorption measured with a quartz crystal microbalance. Biophys. J. 75, 1397–1402. doi: 10.1016/S0006-3495(98)74057-3
Leonenko, Z. V., Finot, E., Ma, H., Dahms, T. E., and Cramb, D. T. (2004). Investigation of temperature-induced phase transitions in DOPC and DPPC phospholipid bilayers using temperature-controlled scanning force microscopy. Biophys. J. 86, 3783–3793. doi: 10.1529/biophysj.103.036681
Lewis, R. N., and McElhany, R. N. (2000). Calorimetric and spectroscopic studies of the thermotropic phase behavior of lipid bilayer model mebranes composed of a homologous series of linear saturated phosphatidylserines. Biophys. J. 79, 2043–2055. doi: 10.1016/S0006-3495(00)76452-6
Lewis, R. N., Pohle, W., and McElhany, N. (1996). The interfacial structure of phospholipid bilayers: differential scanning calorimetry and Fourier transform infrared spectroscopic studies of 1,2-dipalmitoyl-sn-glycero-3-phosphorylcholine and its dialkyl and acyl-alkyl analogs. Biophys. J. 70, 2736–2746. doi: 10.1016/S0006-3495(96)79843-0
Lin, W. C., Blanchette, C. D., Ratto, T. V., Longo, M. L. (2006). Lipid asymmetry in DLPC/DSPC-supported lipid bilayers: A combined AFM and fluorescence microscopy study. Biophys. J. 90, 228–237. doi: 10.1529/biophysj.105.067066
Lind, T. K., and Cárdenas, M. (2016). Understanding the formation of supported lipid bilayers via vesicle fusion–A case that exemplifies the need for the complementary method approach. Biointerphases 11:020801. doi: 10.1116/1.4944830
Liu, J., Stace-Naughton, A., Jiang, X., and Brinker, C. J. (2009). Porous nanoparticle supported lipid bilayers (protocells) as delivery vehicles. J. Am. Chem. Soc. 131, 1354–1355. doi: 10.1021/ja808018y
Losada-Pérez, P., Jiménez-Monroy, K. L., van Grinsven, B., Leys, J., Janssens, S., Peeters, M., et al. (2014). Phase transitions in lipid vesicles detected by a complementary set of methods: heat-transfer measurements, adiabatic scanning calorimetry, and dissipation-mode quartz crystal microbalance. Phys. Status Solidi A 211, 1377–1388. doi: 10.1002/pssa.201431060
Losada-Pérez, P., Khorshid, M., and Renner, F. U. (2016). Interactions of aqueous imidazolium based ionic liquid mixtures with solid supported phospholipid vesicles. PLoS ONE 11:e0163518. doi: 10.1371/journal.pone.0163518
Losada-Pérez, P., Khorshid, M., Yongabi, D., and Wagner, P. (2015a). Effect of cholesterol on the phase behaviour of solid-supported lipid vesicle layers, J. Phys. Chem. B 119, 4985–4992. doi: 10.1021/acs.jpcb.5b00712
Losada-Pérez, P., Mertens, N., de Medio-Vasconcelos, B., Slenders, E., Leys, J., Peeters, M., et al. (2015b). Phase transitions of binary lipid mixtures: a combined study by adiabatic scanning calorimetry and quartz crystal microbalance with dissipation monitoring. Adv. Cond. Mat. Phys. 2015:479318. doi: 10.1155/2015/479318
Losada-Pérez, P., Polat, O., Parikh, A. N., Seker, E., and Renner, F. U. (2018). Engineering the interface between lipid membranes and nanoporous gold: a study by quartz crystal microbalance with dissipation monitoring. Biointerphases 13:011002. doi: 10.1116/1.5010249
Mabrey, S., and Sturtevant, J. M. (1976). Investigation of phase transitions of lipids and lipid mixtures by sensitivity differential scanning calorimetry. Proc. Natl. Acad. Sci. U.S.A. 73, 3862–3866. doi: 10.1073/pnas.73.11.3862
Mangiarotti, A., and Wilke, N. (2015). Energetics of the phase transition in free-standing versus supported lipid membranes. J. Phys. Chem. B 119, 8718–8724. doi: 10.1021/acs.jpcb.5b04397
Mannock, D. A., Lewis, R. N., and McElhany, R. N. (2010). A calorimetric and spectroscopic comparison of the effects of ergosterol and cholesterol on the thermotropic phase behavior and organization of dipamitoylphosphatidylcholine bilayer membranes. Biochim. Biophys. Acta 1798, 376–388. doi: 10.1016/j.bbamem.2009.09.002
Marsh, D. (2012). Thermodynamics of phospholipid self-assembly. Biophys. J. 102, 1079–1087. doi: 10.1016/j.bpj.2012.01.049
Mechler, A., Praporski, S., Atmuri, K., Boland, M., Separovic, F., and Martin, L. L. (2007). Specific and selective peptide-membrane interactions revealed using quartz crystal microbalance. Biophys. J. 93, 3907–3916. doi: 10.1529/biophysj.107.116525
Michanek, A., Kristen, N., Höök, F., Nylander, T., and Sparr, E. (2010). RNA and DNA interactions with zwitterionic and charged lipid membranes–a DSC and QCM-D study. Biophys. Biochim. Acta 1798, 829–838. doi: 10.1016/j.bbamem.2009.12.009
Mouritsen, O. G. (2010). The liquid ordered state comes of age. Biochim. Biophys. Acta Biomembr. 1798, 1286–1288. doi: 10.1016/j.bbamem.2010.02.017
Nielsen, S. B., and Otzen, D. E. (2013). Quartz crystal microbalances as tools for probing protein-membrane interactions. Methods Mol. Biol. 974, 1–21. doi: 10.1007/978-1-62703-275-9_1
Ohlsson, G., Tigerström, A., Höök, F., and Kasemo, B. (2011). Phase transitions in adsorbed lipid vesicles measured using a quartz crystal microbalance with dissipation monitoring. Soft Matter 7, 10749–10755. doi: 10.1039/c1sm05923h
Peetla, C., Rao K. S., and Labhasetwar, V. (2009). Relevance of biophysical interactions of nanoparticles with a model membrane in predicting cellular uptake: study with TAT peptide-conjugated nanoparticles. Mol. Pharmaceutics 6, 1264–1276. doi: 10.1021/mp9000662
Peschel, A., Langhoff, A., Uhl, E., Dathathreyan, A., Haindl, S., Johannsmann, D., et al. (2016). I. Lipid phase behavior studied with a quartz crystal microbalance: a technique for biophysical studies with applications in screening. J. Chem. Phys. 145:204904. doi: 10.1063/1.4968215
Pokorny, A., Yandek, L. E., Elegbede, A. I., Hinderliter, A., and Almeida, P. F. F. (2006). Temperature and composition dependence of the interaction of δ-lysin with ternary mixtures of sphingomyelin/cholesterol/POPC. Biophys. J. 91, 2184–2197. doi: 10.1529/biophysj.106.085027
Pramanik, S., Senneca, S., Ethirajan, A., Neupane, S., Renner, F. U., and Losada-Pérez, P. (2016). Ionic strength dependent vesicle adsorption and phase behaviour of anionic phospholipids on a gold substrate. Biointerphases 11:019006. doi: 10.1116/1.4939596
Purusottam, R. N., Sénicourt, L., Lacapère, J. J., and Tekely, P. (2015). Probing the gel to liquid-crystalline phase transition and relevant conformation changes in liposomes by 13C magic-angle spinning NMR spectroscopy. Biochim. Biophys. Acta Biomembr. 1848, 3134–3139. doi: 10.1016/j.bbamem.2015.09.011
Rahman, M., Yu, E., Forman, E., Roberson-Mailloux, C., Tung, J., Tringe, J., et al. (2014). Modified release from lipid bilayer coated mesoporous silica nanoparticles using PEO-PPO-PEO triblock copolymers. Colloids Surf. B 122, 818–822. doi: 10.1016/j.colsurfb.2014.08.013
Redondo-Morata, L., Giannotti, M. I., and Sanz, F. (2012).Influence of cholesterol on the phase transition of lipid bilayers: a temperature-controlled force spectroscopy study. Langmuir 28, 12851–12860. doi: 10.1021/la302620t
Reviakine, I., Johannsmann, D., and Richter, R. P. (2011). Hearing what you cannot see and visualizing what you hear: interpreting quartz crystal microbalance data from solvated interfaces. Anal. Chem. 83, 8838–8848. doi: 10.1021/ac201778h
Richter, R., Mukhopadhyay, A., and Brisson, A. (2003). Pathways of lipid vesicle deposition on solid surfaces: a combined QCM-D and AFM study. Biophys. J. 85, 3035–3047. doi: 10.1016/S0006-3495(03)74722-5
Shpigel, N., Levi, M. D., Sigalov, S., Daikhin, L., and Aurbach, D. (2018). In situ real-time mechanical and morphological characterization of electrodes for electrochemical energy storage and conversion by electrochemical quartz crystal microbalance with dissipation monitoring. Acc. Chem. Res. 51, 69–79. doi: 10.1021/acs.accounts.7b00477
Simons, K., and Ikonen, E. (1997). Functional rafts in cell membranes. Nature 387, 569–572. doi: 10.1038/42408
Simons, K., and Vaz, W. L. (2004). Model systems, lipid rafts, and cell membranes. Annu. Rev. Biophys. Biomol. Struct. 33, 269–295. doi: 10.1146/annurev.biophys.32.110601.141803
Singer, S. J., and Nicholson, G. L. (1972). The fluid mosaic model of the structure of cell membranes. Science 175, 720–731. doi: 10.1126/science.175.4023.720
Sun, W., Suter, R. M., Knewtson, M. A., Worthington, C. R., Tristam-Nagle, S., Zhang, R., et al. (1994). Order and disorder in fully hydrated unoriented bilayers of gel-phase dipalmitoylphosphatidylcholine. Phys. Rev. E 49, 4665–4676. doi: 10.1103/PhysRevE.49.4665
Szmodis, A. W., Blanchette, C. D., Longo, M. L., Orme, C. A., and Parikh, A. N. (2010). Thermally induced phase separation in supported bilayers of glycosphingolipid and phospholipid mixtures. Biointerphases 5, 120–130. doi: 10.1116/1.3524295
Takahashi, H., Miyagi, A., Redondo-Morata, L., and Scheuring, S. (2016). Temperature-controlled high-speed AFM: real-time observations of ripple phase transitions. Small 12, 6106–6113. doi: 10.1002/smll.201601549
Tristam-Nagle, S., Liu, Y., Legleiter, J., and Nagle, J. F. (2002). Structure of gel phase DMPC determined by X-ray diffraction. Biophys. J. 83, 3324–3335. doi: 10.1016/S0006-3495(02)75333-2
Tristam-Nagle, S., and Nagle, J. F. (2004). Lipid bilayers: thermodynamics, structure, fluctuations and interactions. Chem. Phys. Lipids 127, 3–14. doi: 10.1016/j.chemphyslip.2003.09.002
Voinova, M. V., Rodahl, M., Jonson, M., and Kasemo, B. (1999). Viscoelastic acoustic response of layered polymer films at fluid-solid interfaces: Continuum mechanics approach. Phys. Script. 59, 391–396. doi: 10.1238/Physica.Regular.059a00391
Wargenau, A., and Tufenkji, N. (2014). Direct detection of the gel-fluid phase transition of a single supported phospholipid bilayer using quartz crystal microbalance with dissipation monitoring. Anal. Chem. 86, 8017–8020. doi: 10.1021/ac5019183
Watts, A., and Spooner, P. J. (1991). Phospholipid phase transitions as revealed by NMR. Chem. Phys. Lipids 57, 195–211. doi: 10.1016/0009-3084(91)90076-N
Yee, C. K., Anweg, M. L., and Parikh, A. N. (2004). Membrane photolithography: direct micropatterning and manipulation of fluid phospholipid membranes in the aqueous phase using deep-uv light. Adv. Mater. 16, 1184–1189. doi: 10.1002/adma.200306498
Keywords: QCM-D, model mebranes, phase transitions biological condensed matter, viscoelastic properties, biointerfaces
Citation: Neupane S, De Smet Y, Renner FU and Losada-Pérez P (2018) Quartz Crystal Microbalance With Dissipation Monitoring: A Versatile Tool to Monitor Phase Transitions in Biomimetic Membranes. Front. Mater. 5:46. doi: 10.3389/fmats.2018.00046
Received: 31 May 2018; Accepted: 12 July 2018;
Published: 13 August 2018.
Edited by:
Joshua A. Jackman, Stanford University, United StatesReviewed by:
Vincent Ball, Université de Strasbourg, FranceAgata Weronika Pomorska, Institute of Catalysis and Surface Chemistry, Polish Academy of Sciences (PAN), Poland
Heike Böehm, Max-Planck-Institut für Medizinische Forschung, Germany
Patricia Hegger, Max-Planck-Institut für Medizinische Forschung, Germany, in collaboration with reviewer HB
Copyright © 2018 Neupane, De Smet, Renner and Losada-Pérez. This is an open-access article distributed under the terms of the Creative Commons Attribution License (CC BY). The use, distribution or reproduction in other forums is permitted, provided the original author(s) and the copyright owner(s) are credited and that the original publication in this journal is cited, in accordance with accepted academic practice. No use, distribution or reproduction is permitted which does not comply with these terms.
*Correspondence: Frank U. Renner, ZnJhbmsucmVubmVyQHVoYXNzZWx0LmJl
Patricia Losada-Pérez, cGxvc2FkYXBAdWxiLmFjLmJl