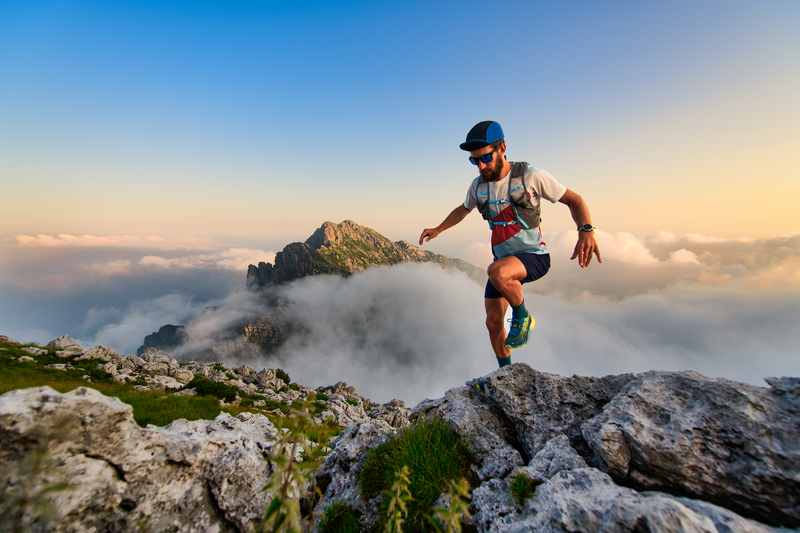
95% of researchers rate our articles as excellent or good
Learn more about the work of our research integrity team to safeguard the quality of each article we publish.
Find out more
ORIGINAL RESEARCH article
Front. Mar. Sci. , 20 March 2025
Sec. Aquatic Microbiology
Volume 12 - 2025 | https://doi.org/10.3389/fmars.2025.1565858
This article is part of the Research Topic Plankton Metabolisms and Interactions in Fluctuating Environments View all articles
Warming is a key factor influencing the function of the structure and function of phytoplankton communities. However, the impacts of temperature on phytoplankton resource use efficiency (RUE) in mountain rivers remain poorly understood. Here, the spatiotemporal patterns of phytoplankton community structure (biomass, community composition, and diversity), function (RUE), and the main environmental factors in a high-latitude mountainous stream were investigated to assess how temperature affects the phytoplankton RUE. The results showed that phytoplankton species richness, biomass, and RUE all increased with rising temperature, with species richness significantly higher. There was a shift in the phytoplankton community from dominated by Cyanophyta at lower temperatures to dominated by Cryptophyta at higher temperatures. Phytoplankton RUE was significantly positively correlated to species richness, but no significant relationship was observed between RUE and Pielou’s evenness. Furthermore, redundancy analysis and Mantel tests revealed that water temperature, nutrient (TP, and NH4+-N) and physicochemical variable (flow velocity, and dissolved oxygen) explained 40.40% of the overall variation in phytoplankton RUE. Phytoplankton RUE exhibited stronger responses to environmental variables than phytoplankton biomass or diversity. The results highlighted that temperature directly affected phytoplankton community composition and enhanced RUE by altering environmental conditions and biodiversity. Temperature plays a crucial role in shaping the structure and function of phytoplankton communities in rivers. Our results contribute to the deep understanding of the mechanisms by which temperature influences RUE providing a basis for the sustainable management and conservation of aquatic ecosystems and watersheds.
Global climate change, particularly temperatures rising, has become a key factor affecting ecosystem functioning (Rosenzweig et al., 2008; Archer, 2011; Nimma et al., 2024). As the primary producers in aquatic ecosystems, phytoplankton play crucial roles in the global carbon cycle, nutrient cycling, and primary production (Field et al., 1998; Paerl and Huisman, 2008). The resource use efficiency (RUE) of phytoplankton communities directly influences the productivity and ecological functions of water ecosystems, which in turn affects the ecological health and service functions of an entire watershed (Wurtsbaugh et al., 2019). RUE is typically influenced by multiple factors, including resource availability, growth rate, population dynamics, and ecological interactions (Tilman, 1982; Hooper et al., 2002; Anderson et al., 2022). Temperature, as a critical environmental driver of phytoplankton growth and distribution, not only directly affects their physiological processes but also indirectly influences their ecological functions by modulating other environmental parameters, such as dissolved oxygen, nutrient concentrations, and light availability (Sommer and Lengfellner, 2008; Maranón et al., 2012; Garzke et al., 2019).
In recent years, an increasing number of studies have focused on the effects of rising temperature on the RUE of phytoplankton communities, particularly on how temperature influences the ecological functions of phytoplankton by altering environmental conditions and biodiversity (Nico et al., 2014). These studies have generally shown that increased temperature generally accelerates phytoplankton growth rates, this effect exhibits different patterns across varying temperature regimes and is species-dependent (Klausmeier et al., 2004; Sherman et al., 2016). More importantly, temperature fluctuations can also impact the biodiversity of phytoplankton communities. Under warming conditions, certain species may gain a competitive advantage, leading to shifts in community structure, which, in turn, can alter the resource utilization efficiency of the community (Hooper et al., 2002). Many studies have examined the direct effects of temperature on phytoplankton growth rates, photosynthetic efficiency, and RUE. Experimental findings generally indicate that elevated temperatures often enhance phytoplankton growth rates, particularly within a certain optimal temperature range (Eppley, 1972; Mousing et al., 2014). For instance, higher temperatures have been shown to increase the rate of photosynthesis in phytoplankton, thereby improving their ability to utilize available resources such as nitrogen, phosphorus, and light (Wu et al., 2024). However, this effect can be heterogeneous, varying across species and environmental conditions. Some species may exhibit adaptive advantages at elevated temperatures, while others may experience growth suppression (Gibert, 2019). Temperature increases also influence RUE by altering their species composition and diversity. Species diversity within phytoplankton communities may decline under warmer conditions, particularly in temperate and cold-water ecosystems, potentially leading to reduced ecosystem stability and altered RUE (MaChado et al., 2019). Species adapting to warmer temperatures, such as certain algae and cyanobacteria, were dominant under higher thermal conditions, resulting in reduced community diversity and possibly affecting the overall functionality of the ecosystem (Barton et al., 2023). On the other hand, some research suggested that phytoplankton communities can maintain functional diversity and resource use efficiency through species turnover and community restructuring in response to elevated temperatures (García et al., 2018).
Temperature influences the resource utilization efficiency of phytoplankton not only through direct effects on their physiological processes but also through interactions with other environmental factors (Gerhard et al., 2022). For instance, rising temperatures can alter nutrient concentrations in the water, dissolved oxygen levels, and light conditions, all of which can further regulate the patterns of resource acquisition and utilization by phytoplankton (Vincent, 2010). Some studies analyzed the link between flow velocity and phytoplankton biomass/composition in a freshwater lake. They found a negative chlorophyll-a-flow velocity correlation, and that turbulence inhibited biomass and affected composition (Li et al., 2013). Rajwa-Kuligiewicz et al. (2015) explained the temperature-dissolved oxygen relationship: higher water temperature reduces oxygen solubility. They also analyzed temperature effects on aquatic organisms and how temperature caused water stratification impacts dissolved oxygen distribution (Rajwa-Kuligiewicz et al., 2015). The study used a mixed-effects model to show low iron content reduces phytoplankton growth rate, highlighting iron-copper interactions in marine phytoplankton (Guo et al., 2012). Lobus and Kulikovskiy (2023) expounded on phytoplankton role in aquatic ecosystem biogeochemistry. They noted phytoplankton efficient use of major and trace elements and explored its nutrient assimilation efficiency and importance in the element cycle (Lobus and Kulikovskiy, 2023). Some studies suggested that higher temperatures not only accelerated nutrient release but also intensified competition between phytoplankton and other organisms, such as zooplankton and bacteria. The combined effects of these changes could alter phytoplankton RUE and community stability (Kent et al., 2007; Azani et al., 2021; Du et al., 2022). Additionally, temperature changes may further regulate phytoplankton nutrient acquisition and resource utilization patterns by affecting symbiotic or competitive relationships between phytoplankton and bacteria (Du et al., 2022). However, although the existing studies have highlighted the potential effects of temperature on the RUE of phytoplankton communities, the mechanisms by which temperature indirectly regulates phytoplankton ecological functions through changes in environmental conditions and biodiversity remain insufficiently understood. In particular, how temperature influences RUE and community stability of phytoplankton by altering species composition, interspecific interactions, and resource allocation strategies is a critical area for further research.
Mountain river ecosystems are characterized by steep altitude gradients, fast-flowing waters, a scarcity of fish, and low temperatures. With an average temperature of 19°C in summer and -12°C in winter, the temperature gradient of this area is generated by seasonal changes. Moreover, it is rich in nutrients such as nitrogen and phosphorus. Its unique climate, nutrient conditions, as well as geographical and vegetation environment, provide an ideal setting for studying how phytoplankton respond to seasonal temperature changes. This involves the relationships among phytoplankton growth, community structure, species composition, the interactions between temperature and nutrients, and the supply of organic matter derived from vegetation. In the present study, the primary environmental factors and phytoplankton community in the Taizicheng River ecosystem over one year were investigated. Phytoplankton RUE was used as a measure of ecosystem functioning in this mountain stream in the north of China. The objectives are to better understand the complex relationships between temperature, environmental factors, and phytoplankton community, and to shed light on the mechanisms by which temperature influences the RUE of phytoplankton. Specifically, this focused on the following aspects: (1) to examine the distribution of phytoplankton communities and their RUE along a temperature gradient; (2) to investigate the influence of environmental variables on phytoplankton dynamics and RUE; (3) to explore the mechanisms through which temperature affects phytoplankton RUE.
Taizicheng River, located in the Chongli District in Zhangjiakou, China, is a mountain river of significant importance, particularly as a crucial part of the 2022 Winter Olympic’ core zone (Figure 1). The river springs from forest-clad mountains, with its basin surrounded by mountains with a vegetation coverage exceeding 65%. The dominant forest types in the region are Betula platyphylla Suk and Larix thibetica. In the upstream section of the river, the thick vegetation close to the riverbanks cast shade, which leads to a decrease in the water temperature of the neighboring areas. The river spans a length of 30.5 km, with an average width of approximately 2.0 m, and a general depth of less than 0.5 m. The river, with an altitude from 1180 m to 1910 m and rapid flow, lies in a region of typical continental monsoon climate: cool-humid summers and long-cold winters. The average temperature in summer is around 19 °C and averages -12 °C in winter. The flow velocity of the river varies between 0.06 and 1.96 m/s. In the rainy season, due to the increased water volume, the stream flows more rapidly. In contrast, during winter, when the region encounters freezing conditions and snowfall, since the precipitation takes the form of snow, the runoff from the mountains drops substantially. The freezing and snowfall period in the region lasts from November to March. The area receives approximately 500 mm of rainfall annually. Mountain runoff following precipitation is the primary water source for the river. The river is abundant in nutrients such as nitrogen and phosphorus, and the concentrations of these nutrients display substantial seasonal fluctuations.
A total of 11 sampling sites were established along the river to cover upstream and downstream features, while areas close to tributaries were also included to assess the impact of additional water input (Figure 1). At the first tributary (Mazhangzi), Sites Z01-Z03 were set. At the second tributary, Sites T02-T06 were designated. Along the river’ mainstream, T07-T10 were set up. All investigations and measurements were conducted quarterly from May 2020 to February 2021 (May 2020, August 2020, November 2020, and February 2021). The locations sampled were the same every quarter. The basic information of the sample points was displayed in Supplementary Tables S1, S2. Water temperature, oxidation-reduction potential, dissolved oxygen (DO), pH, and total dissolved solids (TDS) were measured in situ using a multiparameter sonde (YSI Professional Plus, USA). Water flow velocity was measured at the river surface using a SonTek FlowTracker2 (Summers Ridge Rd, San Diego, CA, USA). At each site, two 1-L surface water samples were collected in previously cleaned polyethylene bottles: one for element analyses, and the other for physicochemical analyses. The water samples were sent to the laboratory within 24h to determine physicochemical factors and elements. Phytoplankton samples were collected by filtering 500mL of surface water using a No.13 (112μm) nested with a No.25 plankton net (64μm) at 11 sampling stations with the Taizicheng River. Sampling was repeated three times at each sampling point. Since the stream is shallow, we did not set multiple vertical sampling points. Samples were immediately preserved with 5% acidic Lugol’s solution, settled for 24 hours at room temperature, and siphon-concentrated into 50-mL amber bottles for further phytoplankton counting.
The chemical oxygen demand (COD), ammonia nitrogen (NH4+-N), total nitrogen (TN), total phosphorus (TP), and nitrogen (NO3–N) were analyzed in the laboratory based on the National Standard of China (GB3838-2002). The copper (Cu), calcium (Ca), iron (Fe), zinc (Zn), and Cobalt (Co) were determined by inductively coupled plasma mass spectrometer (ICP-MS) (Agilent 7800, Heng Sheng Mass Spectrometer Company, Shanghai, China).
The identification and counting of phytoplankton were carried out within chambers with the aid of an inverted microscope (Motic AE31, sourced from Xiamen, China) set at a magnification of 400×. For the cells, colonies, and filaments, their taxonomic classification was determined to the lowest achievable level by referring to the taxonomic illustrations and descriptions of freshwater algae provided by Hu and Wei (2006) (Hu and Wei, 2006). In each sample, a minimum of 500 specimens were counted (Yang et al., 2017). The biovolume was estimated by making use of standard geometric formulae. Subsequently, based on the measured species-specific cell volumes and by applying a specific weight factor of 1 mg/mm³, the abundance data were converted into fresh weight values.
The function of phytoplankton was evaluated according to RUE. Considering the significance of the nitrogen and phosphorus ratio in forecasting the structural composition and seasonal succession of phytoplankton, the ratio of TN to TP served as the foundation for computing phytoplankton RUE (Abell et al., 2010; Moon et al., 2021). It should be noted that RUE differed from the rate of biomass production as it depended on an estimation of standing stock (Robert et al., 2008; Sterner, 2008). Phytoplankton RUE was quantified by us through the ratio of phytoplankton biomass to the N/P ratio (Equation 1). The calculation formula is as follows:
where RUE represents the resource use efficiency of phytoplankton; BIO (Phytoplankton) is the phytoplankton biomass (mg/L); TN is the total nitrogen concentration (mg/L), and TP is the total phosphorus concentration (mg/L).
The effect of temperature on phytoplankton RUE was assessed using linear regression analysis. We separately analyzed the total samples datasets to assess variations in phytoplankton biomass and diversity along the temperature gradient. Diversity and community composition analyses of the phytoplankton community were performed using the phyloseq package (Charlop-Powers and Brady, 2015). Alpha diversity was measured using the “estimate_richness” function in phyloseq. Three biodiversity indices, namely species richness, Shannon-Wiener diversity, and Pielou’s evenness, were investigated. Species richness was defined as the numerical count of distinct species within the community. The diversity of phytoplankton (H) was was computed based on the Shannon-Wiener index (Jiang et al., 2014), and the calculation formula is presented in Equation 2. The evenness of phytoplankton (J) was determined using Pielou’s evenness index (Pielou, 1966), with the formula shown in Equation 3.
Where ni is the number of individuals of species i within a given area, N is the number of total individuals of all species, S is the total species number, and ln S denotes the natural logarithm of the total number of species. It represents the highest possible value of the Shannon-Wiener diversity index achievable when the community attains a state of perfect uniformity with being the number of species.
To visualize the relative abundance of phytoplankton groups and the most abundant species in the community, stacked bar plots were created at the phylum, and specie levels using the “transform_sample_counts” function. The effect of temperature on phytoplankton biomass and diversity, as well as the effects of phytoplankton biomass and diversity on RUE, were determined through linear regression analysis.
Environmental factors with a variance inflation factor (VIF) <10 were selected for RDA analysis to examine the relationship between RUE and environmental variables, using the Canoco 5.0. We conducted Hierarchical Partitioning (HP) analysis with the rdacca.hp package in R 4.4.2 to determine the independent contribution of each environmental variable to the total variance of phytoplankton RUE (Lai et al., 2021).
Furthermore, Mantel test relying on Pearson’s rank correlation was employed to ascertain the associations between community structure (biomass and diversity) or RUE and environmental variables using the vegan, corrplot, and dplyr packages in R 4.4.2 (Yang et al., 2017). Ultimately, partial least squares path modeling (PLS-PM) was utilized by us to illustrate the relationships among phytoplankton community structure, RUE, environmental variables, and temperature levels using partial least squares path modeling in the plspm package in R 4.4.2. Non-significant paths were removed from the model. The goodness-of-fits (GoF) measured the overall predictive power of the model (Benjamini and Hochberg, 1995; Pike, 2011).
All statistical analyses were conducted using R 4.2.2 and visualized with the “ggplot2” package (Ginestet, 2011).
Phytoplankton resource use efficiencies (RUE) was significantly positively correlated with temperature (Figure 2). The temperature distribution of all sampling sites ranged from -6 to 22°C, and RUE increased significantly along the temperature gradient (r=0.51, p<0.001). Among them, the sampling points were denser at -6-7°C, and sparse in the range of 15-22°C. The RUE of total phytoplankton at 10-22°C was significantly higher than that at 0-10°C (Supplementary Figure S1).
Figure 2. Liner relationships between phytoplankton resource use efficiency (RUEN/P) and temperature. RUEN/P was calculated as phytoplankton biomass per unit total N/P. Spearman correlation coefficients (r) are provided for regressions across all samples.
Phytoplankton were primarily classified into the phylum Bacillariophyta (53.89%), Chlorophyta (13.61%), Euglenophyta (12.71%), Pyrrophyta (7.93%), Cryptophyta (6.07%) and Cyanophyta (5.32%) (Figure 3). The highest proportion of Bacillariophyta within the community indicated that they may play a key role in the material circulation and energy flow within this particular ecosystem. Furthermore, a significant relationship was observed between the relative abundances of these phytoplankton phyla and the water temperature. The water temperature remained at or below 10°C in streams, Bacillariophyta and Cyanophyta emerged as the dominant phyla. Their physiological adaptations likely enable them to thrive in such relatively cold water conditions. However, in streams with temperatures ranging from 10 - 22°C, a distinct shift in dominance occurred. Bacillariophyta, Chlorophyta, and Cryptophyta became the dominant groups, while Cyanophyta was scarcely detected. This shift indicated that different phytoplankton phyla have varying temperature-tolerance ranges and competitive abilities under different thermal conditions. At the species level, a marked difference in species richness was noted between the two temperature ranges. Species richness was significantly higher at 10-22°C compared to temperature ≤10°C. Notably, Synedra acus and Navicula capitatoradiata were absent at 10-22°C (Supplementary Figure S2). This absence suggested that these species have a narrow temperature tolerance and are better adapted to the cooler conditions below 10°C.
Figure 3. Relative abundances of phytoplankton at phylum level. The samples were arranged in increasing order of water temperature. In the figure, F represents a temperature range of 0-10 °C, and S represents a temperature range of 10-22 °C.
The distribution characteristics of phytoplankton Biomass, Shannon-Wiener diversity, and Pielou’s evenness along temperature gradients were shown in Supplementary Figure S3 and they were significantly correlated to the temperature across all samples (Figure 4). Temperature exhibited a significant positive correlation with phytoplankton biomass (r = 0.43, p < 0.01) (Figure 4a). Similarly, temperature was positively correlated with phytoplankton richness (r = 0.45, p < 0.01) (Figure 4b). However, phytoplankton evenness significantly decreased with increasing temperature across all samples (r = -0.35, p < 0.01) (Figure 4c).
Figure 4. This figure showed the relationship between phytoplankton biomass, diversity, temperature, and RUE. (a) represented the relationship between temperature and phytoplankton biomass. (b) represented the relationship between temperature and phytoplankton Shannon index. (c) represented the relationship between temperature and phytoplankton evenness. (d) represented the relationship between phytoplankton biomass and RUE. (e) represented the relationship between phytoplankton Shannon index and RUE. (f) represents the relationship between phytoplankton evenness and RUE. RUE standed for resource utilization efficiency. Pearson correlation coefficient (r) is shown in Figure. Shades of gray represent 95% confidence intervals. The unit of temperature is ° C.
Phytoplankton RUE exhibited a significant positive correlation with biomass (r = 0.33, p < 0.05) and species richness (r = 0.49, p < 0.001) (Figure 4d, e), while no significant relationship was observed between phytoplankton RUE and Pielou’s evenness (Figure 4f). Similarly, RUE was positively correlated with both species richness (r = 0.60, p < 0.01) and evenness (r = 0.51, p < 0.05) at temperature between10-20°C. In contrast, no significant relationships were found between phytoplankton RUE and special richness or evenness at temperature ≤ 10°C (Supplementary Figure S4).
The environmental factors have different distribution characteristics along the temperature gradient in Taizicheng River (Supplementary Table S3). The first two axes of the RDA revealed that water temperature, nutrient, and physicochemical variable explained 40.40% of the overall variation in phytoplankton RUE across the entire stream (Figure 5). Water temperature (WT), TP, flow velocity (FV), Cu, and Ca were strongly correlated with each other at p < 0.01, while chlorophyll-a (Chl-a) and NH4+-N showed negative correlation with water WT. Furthermore, hierarchical partitioning analysis indicated that the independent contribution of each of the 18 environmental variables accounted for 60.70% of the total variation in phytoplankton RUE. Among these, water temperature explained the largest proportion of the variation in RUE, followed by Chl-a, DO, TC, Ca, and Fe.
Figure 5. Relationships among phytoplankton RUE, temperature, and environmental variables. WT, water temperature; DO, dissolved oxygen; Chl-a, chlorophyll a.
Figure 6 illustrates the relationships between phytoplankton community structure, function, and environmental factors, with the specific information provided in Supplementary Table S4. Phytoplankton RUE exhibited stronger responses to 19 environmental variables than phytoplankton biomass and diversities (Figure 6). Phytoplankton diversity was significantly correlated with DO (r = 0.34, p < 0.01), FV (r = 0.28, p < 0.01) and WT (r = 0.21, p < 0.01). Additionally, WT, DO, FV, TP, and Chl-a exhibited significant correlations with phytoplankton RUE. Furthermore, phytoplankton RUE was more strongly associated with NH4+-N and Ca. Figure 6 also presents the correlations among various water quality parameters, excluding those with high collinearity (│r│ > 0.7). Specifically, FV, DO, TC, TP, and COD were significantly correlated with WT, Ca and Cu were strongly correlated with FV, and Fe was strongly correlated with DO. These results suggested that various water quality parameters are interrelated, potentially influencing phytoplankton community structure and function through both synergistic and direct effects.
Figure 6. Relationships between environmental variables and phytoplankton community structure and function. A pairwise comparison of environmental factors was displayed with a color gradient denoting Pearson’s correlation coefficient at the bottom left. The phytoplankton community was related to each environment factor by Mantel test at the upper right. WT, water temperature; FV, flow velocity; DO, dissolved oxygen; TN, total nitrogen; TP, total phosphorus; Biomass, phytoplankton biomass; Shannon, species diversity; Evenness, Pielou’s evenness. Only significant Pearson’s correlations among environmental variables are shown (p < 0.05).
The partial least squares path model constructed accounted for 72.50% of the variation in phytoplankton RUE, by integrating water temperature, environmental factors, and phytoplankton community structure (Figure 7; Supplementary Table S5). WT showed a significant positive effect on phytoplankton Shannon-Wiener diversity and a negative effect on evenness, with the direct effects of 0.337 (p < 0.01) and -0.334 (p < 0.01), respectively. Environmental variables, on the other hand, had weak and negative effects on both evenness and Shannon diversity. Furthermore, environmental factors were the most significant drivers of phytoplankton RUE, with direct effects 0.702 (p < 0.01). Notably, temperature significantly affected water properties, with direct effects of 0.732 (p < 0.01), indicating that WT also exerted indirect positive effects on RUE by altering environmental variables and evenness.
Figure 7. Presents partial least squares path models that illustrate the relationships among water temperature, environmental variables, phytoplankton community structure, and phytoplankton function. The goodness of fit index (GoF = 0.409) was utilized to assess the prediction performance of the models. Blue lines signify positive effects, while red lines denote negative effects. The numbers adjacent to each arrow represent the partial correlation coefficients corresponding to each causal relationship. For the sake of simplicity, the partial correlation coefficients less than 0.05 were not displayed. The properties encompassed TP, TC, NO3–N, DO, COD, TDS, and FV.
Temperature changes can alter the physical structure of habitats and hydrological properties in aquatic ecosystems (Grimm et al., 2008). Phytoplankton are a crucial component of river ecosystems and are highly sensitive to environmental change (Jun et al., 2021). Understanding how temperature influences river phytoplankton community structure and functioning is essential for maintaining the balance and stability of freshwater ecosystems.
Temperature level, as a key environmental factor, was significantly positively correlated with RUE across trophic levels, indicating that temperature plays an important role in driving the enhancement of phytoplankton RUE. As the temperature rises, the enzyme activity of phytoplankton is enhanced, and the rates of photosynthesis and respiration accelerate, which leads to an improvement in their RUE (Leles and Levine, 2023). The RUE of total phytoplankton at 10-22°C was significantly higher than that at 0-10°C. This is because the physiological activities of cells are more active at higher temperatures, enabling them to more efficiently uptake and utilize nutrients in the environment (Moore et al., 2021). The comparison of phytoplankton biomass, composition, and diversity across samples in Taizicheng River provided valuable insight into the effects of temperature on the structure and function of phytoplankton communities in cold temperate mountain streams. In our study, phytoplankton diversity, especially species richness, significantly increased with temperature, suggesting that increasing temperature can significantly enhance phytoplankton biomass and richness. The increase in temperature promotes the growth and reproduction of phytoplankton. Within the suitable temperature range, relatively higher temperatures provide more favorable conditions for the metabolism of phytoplankton. This accelerates the rate of cell division, thereby increasing the biomass (Gao et al., 2024). A long-term warming experiment observed a 67.00% increase in phytoplankton species richness, suggesting that higher temperatures may foster a more diverse and stable phytoplankton community by improving ecological functions and species richness (Yvon-Durocher et al., 2015). This result is consistent with our findings. Previous studies have shown that phytoplankton communities were highly sensitive to temperature fluctuations. Experimental studies and model simulations demonstrated that increasing temperatures enhanced the competitiveness of specific functional types of phytoplankton (such as heat-resistant green algae and cyanobacteria), which in turn affected community structure and ecological functions (Deng et al., 2014; Filiz et al., 2020). Our results also revealed a significant shift in phytoplankton community composition along the temperature gradient, from a large number of cyanobacteria exist in 0-10°C to disappear into cryptophyta in 10-20°C. This shift in community composition is likely due to the variations in environmental conditions driven by temperature variations or local changes (Yang et al., 2022; Tian et al., 2024). Some species in the phyla Bacillariophyta and Cyanophyta have a relatively good adaptability to low-temperature environments. They can maintain a certain level of physiological activity at low temperatures and utilize the nutrients in the environment for growth. As the temperature rises, species in the phyla Chlorophyta and Cryptophyta are more capable of adapting to such temperature changes. They possess stronger competitive abilities at higher temperatures and can utilize the abundant light and nutrients for rapid growth. In contrast, the Cyanophyta may be more sensitive to temperature changes, and their growth is inhibited within this temperature range (Repasch et al., 2022). In addition, we observed single species bloom at lower temperature levels (e.g., cyanobacteria), and these bloom events were characterized by lower species diversity and higher biomass. Cryptophyta species at higher temperature levels can serve as a biomarker for water pollution and climate change due to their sensitivity to changes in temperature and light response (Stomp et al., 2007). The photosynthesis of Cryptophyta contributes to carbon dioxide, participates in the global carbon cycle, and helps mitigate climate change (Parker and Hay, 2005). In our model, only 44.80% of the total variation in phytoplankton community structure was explained by environmental variables. This may be due to the lack of unverified variables, such as some trace elements and micropollutants, which are also important in shaping phytoplankton communities. Another possible explanation could be the influence of microbial interactions, which contributed to changes in phytoplankton diversity and community composition (Xue et al., 2018).
Phytoplankton RUE exhibited a significant positive correlation with species richness (Figure 4), indicating that species richness acted as a predictor of RUE in the phytoplankton community. This relationship supported the coupling of biodiversity and productivity of phytoplankton communities in freshwater lakes and marine systems (Robert et al., 2008; Striebel et al., 2009). Along the temperature gradient, we observed that increasing temperature levels could significantly enhance both phytoplankton species richness and RUE (Figures 2, 4). These findings were consistent with several theoretical and empirical studies (Cardinale, Bradley, 2011). One study reported that increasing genus-level phytoplankton diversity resulted in higher RUE (Robert et al., 2008). Additionally, a decrease in species richness was shown to reduce biomass (or abundance) in focal trophic groups, leading to less complete depletion of resources used by those groups (Cardinale et al., 2006). Surprisingly, we found that the positive relationship between RUE and species richness became more pronounced in communities with higher species richness, suggesting that the effect of species richness on RUE was stronger in more diverse communities. However, phytoplankton RUE exhibited no significant correlation with phytoplankton evenness in any of the samples from the Taizicheng River. This result contrasts with findings from Lake Nansihu in northern China (Tian et al, 2017), where species evenness exhibited a significant negative correlation with RUE. He et al. studied the relationship between the phytoplankton community and environmental factors in the waters near Macau. They found that ammonia nitrogen was significantly negatively correlated with the richness, evenness, and Shannon of phytoplankton. Nitrite nitrogen and nitrate nitrogen were positively correlated with the evenness and Shannon of phytoplankton (He et al., 2022). This inconsistency may be attributed to the differing sampling sites and disturbance events across these studies (Jun et al., 2021). The fast flow of mountain streams disrupts the link between RUE and evenness. Uneven resources prompt phytoplankton to adapt by using resources at different times or places. Temperature changes the dominant phytoplankton groups. This impacts interactions, and the resource-getting ways of different species may break the RUE-evenness connection. Nutrients such as TP and NH4+-N, along with flow and oxygen, explain much of the RUE variation. Their unique combination in mountain streams breaks the typical RUE-evenness link found in other waters. It is worth noting that the lack of significant correlation between species evenness and RUE was primarily observed in low-temperature conditions in mountain streams (Mooney et al., 2009; Van Der Plas, 2019). Yang et al. focused on subtropical rivers in southeastern China to explore the impact of urbanization on the structure and function (RUE) of the phytoplankton community. The study revealed that as urbanization levels increased, the biomass of diatoms showed an upward trend, while the diversity and resource use efficiency of phytoplankton decreased significantly. Moreover, changes in environmental factors were significantly correlated with phytoplankton diversity and resource use efficiency (Yang et al., 2022). The Lab warming experiments on subtropical freshwater phytoplankton showed warming cut species richness and led to different community trends. Green algae dominated at medium temps, cyanobacteria at high temps. The highest resource use efficiency was in the warmest treatment, proving warming impacts phytoplankton species, diversity, and ecosystem functions (Moresco et al., 2024).
The temperature could significantly enhance phytoplankton RUE by modifying the environmental variables (Figure 7). The warming and elevation differences at sampling sites may lead to substantial hydrological modifications, which have an impact on the structure and function of aquatic communities (Fausch et al., 2002). Higher elevations, characterized by more variable hydrological conditions, generally display more prominent ecological responses, highlighting the intricate interaction among climate change, and freshwater biodiversity (García et al., 2018). Alterations in water quality, including increasing nitrogen and phosphorus levels, flow velocity, and dissolved oxygen, may favor certain species or a few dominant species that can survive in these stressful conditions. Hypoxia conditions often led to dominance by certain cyanobacteria and green algae, which were well-adapted to low-oxygen environments, thus altering community structure (Cui et al., 2023). The flow rate in downstream was faster and the increasing human activities might not only alter the amounts of nutrients but also change the forms and proportions of nutrients and physicochemical variables, thereby resulting in adverse impacts on water quality (Ji et al., 2024). But it may also have a certain physical effect on the attachment and growth of phytoplankton. The results indicated that phytoplankton RUE exhibited a more pronounced response to water flow velocity (Figure 6). Therefore, the variation in water quality contributed to a higher RUE of phytoplankton by changing the nutrient status. Furthermore, the concentration of nutrients affects the growth and metabolism of phytoplankton, shifting the community composition of both phytoplankton and micro-invertebrates and thus affect the efficiency of resource use (Chessman et al., 2010; Weijters et al., 2010; Zhang et al., 2021). The positive effects of phytoplankton diversity on ecosystem functioning declined with the increase in environmental stress or multiple disturbances (Steudel et al., 2012; Jun et al., 2021). In general, temperature-induced environmental change exerts multiple on phytoplankton communities, including alterations in community composition, biodiversity, and finally ecosystem function.
Temperature exerted an influence on RUE via the potential pathway mediated by the modification of environmental conditions and phytoplankton diversity (Figure 7). Environmental variables are the most important driving factors for phytoplankton RUE. The synergistic and direct effects among environmental factors jointly influence the structure and function of the phytoplankton community (Righetti et al., 2019; Zhang et al., 2023). Changes in water temperature not only directly affect the physiological activities of phytoplankton, but also indirectly influence the growth, reproduction, and community composition of phytoplankton by altering environmental factors such as nutrient concentrations and dissolved oxygen content in the water body. To commence, the phytoplankton diversity and biomass within the river are principally affected by the direct influence of physicochemical variables and hydrodynamic conditions (Isabwe et al., 2018). Additionally, multi-trophic communities in rivers jointly execute a regulatory role in the process of external stressors modifying RUE, which may be correlated with niche partitioning (Cardinale, Bradley, 2011). The research discovered that phytoplankton communities with a larger number of species enable diverse ecosystems to acquire a large portion of biologically available resources (Cardinale, Bradley, 2011). An increase in species richness can lead to a higher occupancy of trophic niches and consequently, a higher overall RUE, potentially because different phytoplankton species exhibit different affinities for specific forms of nutrients (Guedes et al., 2019). Consequently, temperature-induced species alterations affect the efficiency of phytoplankton in resource utilization by attenuating niche partitioning and varying community composition. Meanwhile, the RUE was persistently augmented by temperature-driven environmental stresses exerted on phytoplankton cells. Phytoplankton are not only directly affected by temperature but also indirectly influenced by interactions with other aquatic organisms such as zooplankton, bacteria, and other algal species. Future investigations are essential to further explore how these interactions between phytoplankton and other aquatic organisms impact resource utilization efficiency and community stability under diverse temperature circumstances.
By characterizing the relationships between the phytoplankton community and temperature-related environmental variables in mountain streams, we found that the phytoplankton species richness, biomass, and RUE were progressively increased with ascending temperature levels, and the species richness exhibited a significant augmentation under high-temperature condition. Our results signified that temperature was intimately associated with these alterations in the phytoplankton community. Temperature led to a reduction in Cyanophyta biomass and an increment in Cryptophyta biomass in the Taizicheng River. Our findings also implied that temperature enhanced the resource use efficiency of the phytoplankton community by modifying the environment and biodiversity in mountain rivers. Future research could further probe into how temperature governs the ecological function of phytoplankton communities by influencing species diversity, including functional diversity, via more elaborate experiments and model simulations. In general, our work constitutes substantial progress in comprehending the role of temperature in molding the structure and function of phytoplankton communities in mountain streams. It furnishes a theoretical foundation for practical ecological protection and water quality management.
The original contributions presented in the study are included in the article/Supplementary Material. Further inquiries can be directed to the corresponding author.
The manuscript presents research on animals that do not require ethical approval for their study.
LJ: Conceptualization, Data curation, Formal Analysis, Investigation, Methodology, Software, Visualization, Writing – original draft, Writing – review & editing. HZ: Conceptualization, Funding acquisition, Project administration, Resources, Supervision, Writing – review & editing. ZW: Supervision, Writing – review & editing. YT: Supervision, Writing – review & editing. WT: Supervision, Writing – review & editing. ZL: Supervision, Writing – review & editing.
The author(s) declare that financial support was received for the research and/or publication of this article. This research was funded by the National Major Science and Technology Program for Water Pollution Control and Treatment, grant number No. 2017ZX07101. And the Discipline Construction Program of Huayong Zhang, Distinguished Professor of Shandong University, School of Life Sciences, grant number 61200082363001. The APC was funded by North China Electric Power University.
The authors would like to acknowledge with great gratitude for the support of National Major Science and Technology Program for Water Pollution Control and Treatment (No. 2017ZX07101) and the Discipline Construction Program of Huayong Zhang, Distinguished Professor of Shandong University, School of Life Sciences (61200082363001).
The authors declare that the research was conducted in the absence of any commercial or financial relationships that could be construed as a potential conflict of interest.
The author(s) declare that no Generative AI was used in the creation of this manuscript.
All claims expressed in this article are solely those of the authors and do not necessarily represent those of their affiliated organizations, or those of the publisher, the editors and the reviewers. Any product that may be evaluated in this article, or claim that may be made by its manufacturer, is not guaranteed or endorsed by the publisher.
The Supplementary Material for this article can be found online at: https://www.frontiersin.org/articles/10.3389/fmars.2025.1565858/full#supplementary-material
Abell J. M., Özkundakci D., Hamilton D. P. (2010). Nitrogen and phosphorus limitation of phytoplankton growth in New Zealand lakes: implications for eutrophication control. Ecosystems 13, 966–977. doi: 10.1007/s10021-010-9367-9
Anderson S. I., Franzè G., Kling J. D., Wilburn P., Kremer C. T., Menden-Deuer S., et al. (2022). The interactive effects of temperature and nutrients on a spring phytoplankton community. Limnology Oceanography 67, 634–645. doi: 10.1002/lno.12023
Azani N., Ghaffar M., Suhaimi H., Azra M., Hassan M., Jung L., et al. (2021). The impacts of climate change on plankton as live food: A review (IOP Publishing), 012005.
Barton S., Padfield D., Masterson A., Buckling A., Smirnoff N., Yvon-Durocher G. (2023). Comparative experimental evolution reveals species-specific idiosyncrasies in marine phytoplankton adaptation to warming. Global Change Biol. 29, 5261–5275. doi: 10.1111/gcb.v29.18
Benjamini Y., Hochberg Y. (1995). Controlling the false discovery rate: a practical and powerful approach to multiple testing. J. R. Stat. Society: Ser. A 57, 289–300. doi: 10.1111/j.2517-6161.1995.tb02031.x
Cardinale B. J., Srivastava D. S., Emmett Duffy J., Wright J. P., Downing A. L., Sankaran M., et al. (2006). Effects of biodiversity on the functioning of trophic groups and ecosystems. Nature 443, 989–992. doi: 10.1038/nature05202
Cardinale, Bradley J. (2011). Biodiversity improves water quality through niche partitioning. Nature 472 (7341), 86–89. doi: 10.1038/nature09904
Charlop-Powers Z., Brady S. F. (2015). Phylogeo: an r package for geographic analysis and visualization of microbiome data. Bioinformatics 31, 2909–2911. doi: 10.1093/bioinformatics/btv269
Chessman B., Growns I., Currey J., Plunkett-Cole N. (2010). Predicting diatom communities at the genus level for the rapid biological assessment of rivers. Freshw. Biol. 41, 317–331. doi: 10.1046/j.1365-2427.1999.00433.x
Cui Z., Gao W., Li Y., Wang W., Wang H., Liu H., et al. (2023). Dissolved oxygen and water temperature drive vertical spatiotemporal variation of phytoplankton community: Evidence from the largest diversion water source area. Int. J. Environ. Res. Public Health 20 (5), 4307. doi: 10.3390/ijerph20054307
Deng J., Qin B., Paerl H. W., Zhang Y., Chen Y. (2014). Effects of nutrients, temperature and their interactions on spring phytoplankton community succession in lake taihu, China. PloS One 9, e113960. doi: 10.1371/journal.pone.0113960
Du C., Li G., Xia R., Li C., Zhu Q., Li X., et al. (2022). New insights into cyanobacterial blooms and the response of associated microbial communities in freshwater ecosystems. Environ. pollut. 309, 119781. doi: 10.1016/j.envpol.2022.119781
Fausch K. D., Torgersen C. E., Baxter C. V., Li H. W. (2002). Landscapes to riverscapes: bridging the gap between research and conservation of stream fishes: A continuous view of the river is needed to understand how processes interacting among scales set the context for stream fishes and their habitat. BioScience 52, 483–498. doi: 10.1641/0006-3568(2002)052[0483:LTRBTG]2.0.CO;2
Field C. B., Behrenfeld M. J., Randerson J. T., Falkowski P. (1998). Primary production of the biosphere: integrating terrestrial and oceanic components. Science 281, 237–240. doi: 10.1126/science.281.5374.237
Filiz N., Işkın U., Beklioğlu M., Öğlü B., Cao Y., Davidson T. A., et al. (2020). Phytoplankton community response to nutrients, temperatures, and a heat wave in shallow lakes: an experimental approach. Water 12 (12), 3394. doi: 10.3390/w12123394
Gao S., Yang W., Li X., Zhou L., Liu X., Wu S., et al. (2024). Cryptochrome PtCPF1 regulates high temperature acclimation of marine diatoms through coordination of iron and phosphorus uptake. ISME J. 18, wrad019. doi: 10.1093/ismejo/wrad019
García F. C., Bestion E., Warfield R., Yvon-Durocher G. (2018). Changes in temperature alter the relationship between biodiversity and ecosystem functioning. Proc. Natl. Acad. Sci. 115, 201805518. doi: 10.1073/pnas.1805518115
Garzke J., Connor S. J., Sommer U., O’connor M. I. (2019). Trophic interactions modify the temperature dependence of community biomass and ecosystem function. PloS Biol. 17, e2006806. doi: 10.1371/journal.pbio.2006806
Gerhard M., Schlenker A., Hillebrand H., Striebel M. (2022). Environmental stoichiometry mediates phytoplankton diversity effects on communities’ resource use efficiency and biomass. J. Ecol. 110, 430–442. doi: 10.1111/1365-2745.13811
Gibert J. P. (2019). Temperature directly and indirectly influences food web structure. Sci. Rep. 9, 5312. doi: 10.1038/s41598-019-41783-0
Ginestet C. (2011). ggplot2: elegant graphics for data analysis. Journal of the Royal Statistical society: series A 174, 245–246. doi: 10.1111/j.1467-985X.2010.00676_9.x
Grimm N., Faeth S., Golubiewski N., Briggs J. J. S. (2008). Global change and the ecology of cities. Science 319 (5864), 756–760. doi: 10.1126/science.1150195
Guedes I. A., Beatriz A., Pacheco F., Vilar M. C. P., Azevedo S. M. F. O. (2019). Intraspecific variability in response to phosphorus depleted conditions in the Cyanobacteria Microcystis aeruginosa and Raphidiopsis raciborskii. Harmful Algae 86, 96–105. doi: 10.1016/j.hal.2019.03.006
Guo J., Lapi S., Ruth T. J., Maldonado M. T. (2012). The effects of iron and copper availability on the copper stoichiometry of marine phytoplankton 1. J. Phycology 48, 312–325. doi: 10.1111/j.1529-8817.2012.01133.x
He R., Luo H., He N., Chen W., Yang F., Huang W., et al. (2022). Phytoplankton communities and their relationship with environmental factors in the waters around Macau. Int. J. Environ. Res. Public Health 19, 7788. doi: 10.3390/ijerph19137788
Hooper D. U., Buchmann N., Degrange V., Díaz S. M., Spehn E. M. (2002). Species diversity, functional diversity and ecosystem functioning. Science 312, 846a–848a. doi: 10.1126/science.312.5775.846a
Isabwe A., Yang J. R., Wang Y., Liu L., Chen H., Yang J. (2018). Community assembly processes underlying phytoplankton and bacterioplankton across a hydrologic change in a human-impacted river. Sci. Total Environ. 630, 658–667. doi: 10.1016/j.scitotenv.2018.02.210
Ji L., Zhang H., Wang Z., Tian Y., Tian W., Liu Z. (2024). Ecological stoichiometry characteristic of phytoplankton in mountain stream. Water 16, 2541. doi: 10.3390/w16172541
Jiang Y.-J., He W., Liu W.-X., Qin N., Ouyang H.-L., Wang Q.-M., et al. (2014). The seasonal and spatial variations of phytoplankton community and their correlation with environmental factors in a large eutrophic Chinese lake (Lake Chaohu). Ecol. Indic. 40, 58–67. doi: 10.1016/j.ecolind.2014.01.006
Jun R., Yang X., Yu H., Chen Y. M., Yang K. J. (2021). Structural and functional variations of phytoplankton communities in the face of multiple disturbances. J. Environ. Sci. v.100, 289–299. doi: 10.1016/j.jes.2020.07.026
Kent A. D., Yannarell A. C., Rusak J. A., Triplett E. W., Mcmahon K. D. (2007). Synchrony in aquatic microbial community dynamics. ISME J. 1, 38–47. doi: 10.1038/ismej.2007.6
Klausmeier C. A., Litchman E., Daufresne T., Levin S. A. (2004). Optimal nitrogen-to-phosphorus stoichiometry of phytoplankton. Nature 429, 171. doi: 10.1038/nature02454
Lai J., Zou Y., Zhang JY., Peres-Neto P. (2013). rdacca.hp: an R package for generalizing hierarchical and variation partitioning in multiple regression and canonical analysis[J]. .bioRxiv. 2021. doi: 10.1101/2021.03.09.434308
Leles S. G., Levine N. M. (2023). Mechanistic constraints on the trade-off between photosynthesis and respiration in response to warming. Sci. Adv. 9 (35), eadh8043. doi: 10.1126/sciadv.adh8043
Li F., Zhang H., Zhu Y., Xiao Y., Chen L. (2013). Effect of flow velocity on phytoplankton biomass and composition in a freshwater lake. Sci. Total Environ. 447, 64–71. doi: 10.1016/j.scitotenv.2012.12.066
Lobus N. V., Kulikovskiy M. S. (2023). The co-evolution aspects of the biogeochemical role of phytoplankton in aquatic ecosystems: A review. Biology 12, 92. doi: 10.3390/biology12010092
MaChado K. B., Vieira L. C. G., Nabout J. C. (2019). Predicting the dynamics of taxonomic and functional phytoplankton compositions in different global warming scenarios. Hydrobiologia 830, 115–134. doi: 10.1007/s10750-018-3858-7
Maranón E., Cermeno P., Latasa M., Tadonléké R. D. (2012). Temperature, resources, and phytoplankton size structure in the ocean. Limnology Oceanography 57, 1266–1278. doi: 10.4319/lo.2012.57.5.1266
Moon D. L., Scott J. T., Johnson T. R. (2021). Stoichiometric imbalances complicate prediction of phytoplankton biomass in US lakes: Implications for nutrient criteria. Limnology Oceanography 66, 2967–2978. doi: 10.1002/lno.11851
Mooney H., Larigauderie A., Cesario M., Elmquist T., Hoegh-Guldberg O., Lavorel S., et al. (2009). Biodiversity, climate change, and ecosystem services. Curr. Opin. Environ. Sustainability 1, 46–54. doi: 10.1016/j.cosust.2009.07.006
Moore C. E., Meacham-Hensold K., Lemonnier P., Slattery R. A., Benjamin C., Bernacchi C. J., et al. (2021). The effect of increasing temperature on crop photosynthesis: from enzymes to ecosystems. J. Exp. Bot. 72, 2822–2844. doi: 10.1093/jxb/erab090
Moresco G. A., Dias J. D., Cabrera-Lamanna L., Baladán C., Bizic M., Rodrigues L. C., et al. (2024). Experimental warming promotes phytoplankton species sorting towards cyanobacterial blooms and leads to potential changes in ecosystem functioning. Sci. Total Environ. 924, 171621. doi: 10.1016/j.scitotenv.2024.171621
Mousing E. A., Ellegaard M., Richardson K. (2014). Global patterns in phytoplankton community size structure—evidence for a direct temperature effect. Mar. Ecol. Prog. Ser. 497, 25–38. doi: 10.3354/meps10583
Nico R., Helmsing E., Van D., Wolf M., Mooij L. (2014). Community stoichiometry in a changing world: combined effects of warming and eutrophication on phytoplankton dynamics. Ecol. A Publ. Ecol. Soc. America. 95, 1485–1495. doi: 10.1890/13-1251.1
Nimma D., Devi O. R., Laishram B., Ramesh J. V. N., Boddupalli S., Tirth V., et al. (2024). Implications of climate change on freshwater ecosystems and their biodiversity. Desalination And Water Treat 321, 100889. doi: 10.1016/j.dwt.2024.100889
Paerl H. W., Huisman J. (2008). Climate. Blooms like it hot. Sci. 320, 57–58. doi: 10.1126/science.1155398
Parker J. D., Hay M. E. (2005). Biotic resistance to plant invasions? Native herbivores prefer non-native plants. Ecol. Lett. 8 (9). doi: 10.1111/j.1461-0248.2005.00799.x
Pielou E. C. (1966). Species-diversity and pattern-diversity in the study of ecological succession. J. Theor. Biol. 10, 370–383. doi: 10.1016/0022-5193(66)90133-0
Pike N. (2011). Using false discovery rates for multiple comparisons in ecology and evolution. Methods Ecol. Evol. 2, 278–282. doi: 10.1111/j.2041-210X.2010.00061.x
Rajwa-Kuligiewicz A., Bialik R. J., Rowiński P. M. (2015). Dissolved oxygen and water temperature dynamics in lowland rivers over various timescales. J. Hydrology Hydromechanics 63, 353–363. doi: 10.1515/johh-2015-0041
Repasch M., Scheingross J. S., Hovius N., Vieth-Hillebrand A., Mueller C. W., Höschen C., et al. (2022). River organic carbon fluxes modulated by hydrodynamic sorting of particulate organic matter. Geophysical Res. Lett. 49, e2021GL096343. doi: 10.1029/2021GL096343
Righetti D., Vogt M., Gruber N., Psomas A., Zimmermann N. E. (2019). Global pattern of phytoplankton diversity driven by temperature and environmental variability. Sci. Adv. 5, eaau6253. doi: 10.1126/sciadv.aau6253
Robert P., Solimini A., Andersen T., Tamminen T. (2008). Diversity predicts stability and resource use efficiency in natural phytoplankton communities. Proc. Natl. Acad. Sci. U.S.A. 105 (13), 5134–5138. doi: 10.1073/pnas.0708328105
Rosenzweig C., Karoly D., Vicarelli M., Neofotis P., Wu Q., Casassa G., et al. (2008). Attributing physical and biological impacts to anthropogenic climate change. Nature 453, 353–357. doi: 10.1038/nature06937
Sherman E., Moore J. K., Primeau F., Tanouye D. (2016). Temperature influence on phytoplankton community growth rates. Global Biogeochemical Cycles 30, 550–559. doi: 10.1002/2015GB005272
Sommer U., Lengfellner K. (2008). Climate change and the timing, magnitude, and composition of the phytoplankton spring bloom. Global Change Biol. 14, 1199–1208. doi: 10.1111/j.1365-2486.2008.01571.x
Sterner R. W. (2008). On the phosphorus limitation paradigm for lakes. Int. Rev. Hydrobiology 93, 433–445. doi: 10.1002/iroh.200811068
Steudel H., Friedl L., Kessler L. W. (2012). Biodiversity effects on ecosystem functioning change along environmental stress gradients. Ecol Lett. 15, 1397–1405. doi: 10.1111/j.1461-0248.2012.01863.x
Stomp M., Huisman J., Stal L. J., Matthijs H. C. P. (2007). Colorful niches of phototrophic microorganisms shaped by vibrations of the water molecule. Isme J. doi: 10.1038/ismej.2007.59
Striebel M., Behl S., Stibor H. (2009). The coupling of biodiversity and productivity in phytoplankton communities: consequences for biomass stoichiometry. Ecology 2009, 90. doi: 10.1890/08-1409.1
Tian W., Huayongzhang J., Mingshenghuang L., Hai %J Environmental Science, Research P (2017). Biodiversity effects on resource use efficiency and community turnover of plankton in Lake Nansihu, China, Vol. 24. doi: 10.1007/s11356-017-8758-2
Tian W., Wang Z., Kong H., Tian Y., Huang T. (2024). Temporal–spatial fluctuations of a phytoplankton community and their association with environmental variables based on classification and regression tree in a shallow temperate mountain river. Microorganisms 12 (8), 1612. doi: 10.3390/microorganisms12081612
Tilman D. (1982). Resource competition and community structure (Princeton University Press), No. 17.
Van Der Plas F. (2019). Biodiversity and ecosystem functioning in naturally assembled communities. Biol. Rev. 94, 1220–1245. doi: 10.1111/brv.2019.94.issue-4
Vincent W. F. (2010). Microbial ecosystem responses to rapid climate change in the arctic. Isme J. 4, 1087–1090. doi: 10.1038/ismej.2010.108
Weijters M. J., Janse J. H., Alkemade R., Verhoeven J. T. A. (2010). Quantifying the effect of catchment land use and water nutrient concentrations on freshwater river and stream biodiversity. Aquat. Conserv. Mar. Freshw. Ecosyst. 19, 104–112. doi: 10.1002/aqc.v19:1
Wu Y., Ye Q., Chen S., Jiang L., Chen K., Ma S., et al. (2024). Climate changes drive phytoplankton community through complementarity and selection effects in a large mesotrophic reservoir. J. Oceanology Limnology. 43 (1), 119–133. doi: 10.1007/s00343-024-3260-4
Wurtsbaugh W. A., Paerl H. W., Dodds W. K. (2019). Nutrients, eutrophication and harmful algal blooms along the freshwater to marine continuum. Wiley Interdiscip. Reviews: Water 6, e1373. doi: 10.1002/wat2.1373
Xue Y., Chen H., Yang J. R., Liu M., Huang B., Yang J. (2018). Distinct patterns and processes of abundant and rare eukaryotic plankton communities following a reservoir cyanobacterial bloom. ISME J. 12, 2263–2277. doi: 10.1038/s41396-018-0159-0
Yang Y., Chen H., Al M. A., Ndayishimiye J. C., Yang J. R., Isabwe A., et al. (2022). Urbanization reduces resource use efficiency of phytoplankton community by altering the environment and decreasing biodiversity. J. Environ. Sci. 112, 140–151. doi: 10.1016/j.jes.2021.05.001
Yang J. R., Lv H., Isabwe A., Liu L., Yu X., Chen H., et al. (2017). Disturbance-induced phytoplankton regime shifts and recovery of cyanobacteria dominance in two subtropical reservoirs. Water Res. 120, 52–63. doi: 10.1016/j.watres.2017.04.062
Yvon-Durocher G., Allen A. P., Cellamare M., Dossena M., Gaston K. J., Leitao M., et al. (2015). Five years of experimental warming increases the biodiversity and productivity of phytoplankton. PloS Biol. 13 (12), e1002324. doi: 10.1371/journal.pbio.1002324
Zhang G., Liu Z., Zhang Z., Ding C., Sun J. (2023). The impact of environmental factors on the phytoplankton communities in the Western Pacific Ocean: HPLC-CHEMTAX approach. doi: 10.3389/fmars.2023.1185939
Keywords: phytoplankton community, mountain stream, temperature, environmental factors, community function, aquatic ecosystem
Citation: Ji L, Zhang H, Wang Z, Tian Y, Tian W and Liu Z (2025) Temperature orchestrates phytoplankton community and environment in mountain stream for enhancing resource use efficiency. Front. Mar. Sci. 12:1565858. doi: 10.3389/fmars.2025.1565858
Received: 23 January 2025; Accepted: 03 March 2025;
Published: 20 March 2025.
Edited by:
Ningdong Xie, University of Florida, United StatesCopyright © 2025 Ji, Zhang, Wang, Tian, Tian and Liu. This is an open-access article distributed under the terms of the Creative Commons Attribution License (CC BY). The use, distribution or reproduction in other forums is permitted, provided the original author(s) and the copyright owner(s) are credited and that the original publication in this journal is cited, in accordance with accepted academic practice. No use, distribution or reproduction is permitted which does not comply with these terms.
*Correspondence: Huayong Zhang, emhhbmdodWF5b25nQHNkdS5lZHUuY24=
Disclaimer: All claims expressed in this article are solely those of the authors and do not necessarily represent those of their affiliated organizations, or those of the publisher, the editors and the reviewers. Any product that may be evaluated in this article or claim that may be made by its manufacturer is not guaranteed or endorsed by the publisher.
Research integrity at Frontiers
Learn more about the work of our research integrity team to safeguard the quality of each article we publish.