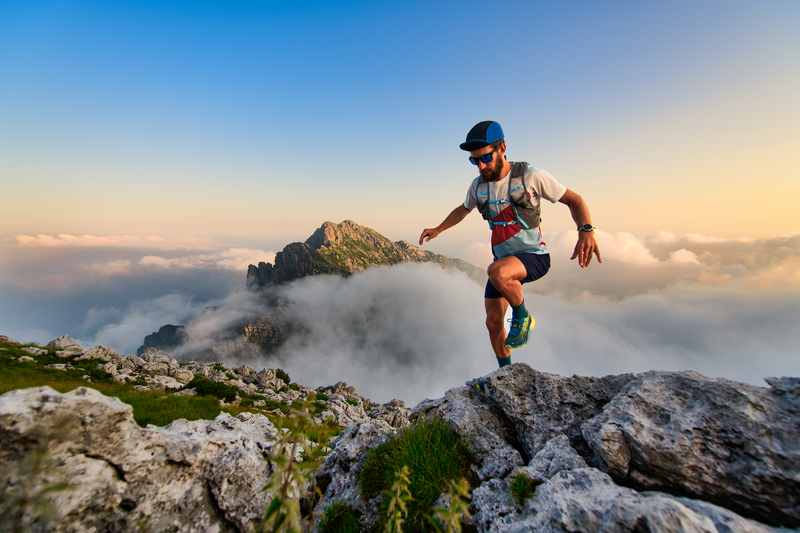
95% of researchers rate our articles as excellent or good
Learn more about the work of our research integrity team to safeguard the quality of each article we publish.
Find out more
ORIGINAL RESEARCH article
Front. Mar. Sci. , 10 March 2025
Sec. Marine Pollution
Volume 12 - 2025 | https://doi.org/10.3389/fmars.2025.1562111
The present study was undertaken to examine the impact of varying concentrations of divalent zinc cation (Zn2+) on the growth, antioxidant levels, fatty acid composition, and related gene expression in a pennate diatom, Phaeodactylum tricornutum. As a prevalent environment contaminant, zinc is introduced into aquatic ecosystems via agricultural and industrial processes, exerting toxic effects on aquatic biota. P. tricornutum was exposed to gradient Zn2+ concentrations (0.99–1000.23 μM), with growth tracked spectrophotometrically. Antioxidant biomarkers, fatty acid profiles, and Zn-responsive gene expression were analyzed via biochemical assays, gas chromatography, and qRT-PCR, respectively. The results showed that appropriate concentrations of Zn2+ were essential for the growth of P. tricornutum, but high concentrations of Zn2+ (1000.23 μM) significantly inhibited its growth. Zinc stress also led to the production of reactive oxygen species (ROS), which in turn triggered oxidative stress, as evidenced by changes in antioxidant enzyme activities and lipid peroxidation levels. Furthermore, zinc stress affected the fatty acid composition of P. tricornutum, particularly in the group exposed to high concentrations of Zn2+. There was a notable reduction in the levels of polyunsaturated fatty acids (PUFAs) and highly unsaturated fatty acids (HUFAs), while the levels of saturated fatty acids (SFAs) and monounsaturated fatty acids (MUFAs) increased. Gene expression analyses indicated alterations in the expression of zinc transporter proteins and antioxidant-related genes, suggesting that P. tricornutum adapts to zinc stress through the regulation of gene expression. These findings provide new insights into the understanding of the physiological and molecular responses of microalgae to zinc pollution and a scientific basis for evaluating the potential impacts of zinc pollution on aquatic ecosystems and developing bioremediation strategies.
As a common contaminant, zinc is released into natural waters from livestock farming wastewater, municipal wastewater, and various industrial effluents including those from electroplating, electronics, and metal cleaning (Chong et al., 2000; Chan et al., 2014; Chen et al., 2022). Generally, zinc has also been extensively used as feeding additives to increase fishery production in aquaculture (Shahpar and Johari, 2019). However, only partial zinc can be metabolized by livestock, causing a large number of residues in the excreta (Hardy et al., 1987). The element is thus easily discharged to the water through leaching and direct runoff, and thus spreading heavy metal contamination.
As a heavy metal, zinc exhibited various hazards on the aquatic life. High concentrations of zinc can be toxic to aquatic organisms, leading to impaired growth, respiratory systems, reproduction, and survival rates in fish and invertebrates. Studies have shown that zinc exposure can disrupt physiological functions in aquatic species. Zinc can damage gill tissue, interfere with acid-base and ion regulation, and result in hypoxia (Damseth et al., 2024). Zinc exposure can also affect the growth rate and development processes of fish, impairing their estrogenic and androgenic functions, leading to abnormal differentiation (Paschoalini and Bazzoli, 2021).
Zinc contamination also affects the growth and function of aquatic plants and phytoplankton, crucial for aquatic ecosystems. High zinc levels (2.20-3.30 mg L-1) significantly reduce the growth rate and final yield of Synechocystis aquatilis f. aquatilis by approximately 60% and 50%, respectively, compared to lower concentrations (0.21 mg L-1) (do Carmo e Sá et al., 2004). Elevated divalent zinc cation (Zn2+) levels have been shown to decrease the variable chlorophyll fluorescence (Fv/Fm) in Chlorella pyrenoidosa, indicating rapid PSII inactivation (Plekhanov and Chemeris, 2003). Mallick and Mohn (2003) observed substantial inhibition of PSII photochemistry in Scenedesmus after zinc exposure (Mallick and Mohn, 2003). Küpper et al. (1998) proposed that heavy metals inhibit photosynthesis by substituting Mg2+ in chlorophyll, with related detrimental effects described in higher plants and green algae (Küpper et al., 1998). The formation of metal-substituted chlorophylls has also been identified in cyanobacteria and Chlorella (De Filippis, 1979; Eckardt et al., 1992).
Elevated zinc concentrations can also cause oxidative stress by generating reactive oxygen species (ROS) that harm cellular components, though the exact mechanism remains unclear (López-Millán et al., 2005). It is suggested that this stress may disrupt metabolic processes, including the antioxidant defense system or photosynthetic electron transport (Nable, 1988; Cakmak, 2000). Tripathi and Gaur (2004) demonstrated that high zinc concentrations increased lipid peroxidation and membrane permeability while depleting sulfhydryl content in Scenedesmus (Tripathi and Gaur, 2004). Ajitha et al. (2021) suggested a significant decrease in protein content in green algae at elevated zinc and mercury levels. Weckx and Clijsters (1997) also found that toxic zinc concentrations stimulated lipoxygenase activity and lipid peroxidation. Zinc may affect the marine nitrogen cycle by reducing the growth of phytoplankton, which causes a reduced release of amino acids and alters the types of released algae-derived amino acids (Huang et al., 2019). Additionally, zinc can inhibit photosynthetic electron transport, generating harmful oxygen species like and H2O2 (Kappus, 1985). Weckx and Clijsters (1997) observed elevated H2O2 levels in Phaseolus vulgaris 172 hours after zinc treatment.
This study focuses on the commonly found pennate diatom Phaeodactylum tricornutum in coastal marine environments, aiming to elucidate the effects of varying concentrations of Zn2+ on its growth, antioxidant levels, fatty acid composition and related expression of key genes. The findings of this study are intended to provide valuable insights into the impact of Zn2+ concentrations in seawater on the growth and metabolic processes of marine phytoplankton.
The axenic P. tricornutum CCMP2561 was obtained from the Laboratory of Applied Microalgae Biology, Ocean University of China. Cells were cultivated in f/2 medium (Guillard and Ryther, 1962), and shaken at 22 ± 1°C and 100 rpm under illumination from LED lamps with an irradiance of 100 µmol m-2 s-1 on a 14: 10 h light: dark photoperiod. The late-logarithmic growth phase cultures were used as inoculums, which were centrifuged at 4000 g for 5 min and resuspended into the fresh medium. Final serial concentrations (0.99, 7.88, 70.88, 1000.23 μM) of Zn2+ (the zinc salt is ZnCl2) were then added to P. tricornutum cultures. The P. tricornutum cultures were harvested at the logarithmic growth phase to compare growth, antioxidant activity, zinc-related gene expression and fatty acid composition. All treatments were carried out in triplicate. Cultures were sampled every day. Cell growth was determined using optical density (OD) readings at 750 nm with a UV-2010 (Hitachi, Japan) spectrophotometer in a 1 cm-light-path cuvette.
Generally, 50 mL cultures were collected, centrifuged, and washed 2 times with phosphate buffered saline buffer (PBS, 50 mMNa2HPO4 and 50 mM NaH2PO4 at pH 7.4) at 4000 g for 10 min. Cell pellets were then homogenized in 1mL PBS and then centrifuged at 3000 g, 4 °C for 10 min. The supernatant was collected for superoxide dismutase (SOD) activity, malondialdehyde (MDA) content and reduced glutathione (GSH) content analysis using the commercial kits (Nanjing Jiancheng Bioengineering Co. Ltd, Nanjing, PR China) according to the manufacturer’s instructions. Protein concentration in the supernatant was measured by the Bradford assay (Bradford, 1976) to normalize the enzyme activity (U/mg prot) or chemical content (nmol/mg prot or mg/g prot).
We analyzed the fatty acid composition as described in our previous publication (Qiao et al., 2015). On the seventh day of algal cell growth, around 0.1-0.5 g of algal cells were gathered by centrifuging in a screw-capped test tube measuring 12 cm × 1.5 cm. Five hundred microliter of toluene with the internal standard (0.1 mg glyceryl triheptadecanoate) and 1 mL of 0.5 M NaOH methanol solution were gradually added. After vortexing and mixing, the mixture was placed in a water bath at 80°C for 20 min, with constant shaking. Once cooled to room temperature, add 1 mL of 10:100 (v/v) acetyl chloride in anhydrous methanol and repeat the above warm bath step. After cooling the mixture to room temperature for 5 min, add 1 mL of 6% K2CO3 and 400 μL of n-hexane, vortex and mix well, and the supernatant was separated by centrifuging at 4000 g for 1 min, with the upper layer being collected. The resulting fatty acid methyl esters were then subjected to analysis via gas chromatography (GC-1949; Panna, Changzhou, China) equipped with a fused silica capillary column (Supelco SP-2560: 100 m × 0.25 mm, film thickness 0.20 µm; Bellefonte, PA, USA). The heater was kept at 260°C using nitrogen as the carrier gas, while the column temperature increased from 140 to 260°C at a rate of 10°C per min. Fatty acids were identified by correlating the relative retention times with those of the reference standards (Merck, Rahway, NJ, USA).
Each treatment group collected 250 mL of algal culture, which was centrifuged at 12,000 g for 10 min at 4°C to obtain the pellet. The pellet was then frozen in liquid nitrogen and stored at -80°C. Total RNA was extracted using the Trizol method, and reverse transcription was performed using the PrimeScript RT Reagent Kit (including gDNA Eraser, TaKaRa Biotech Co., Dalian, China) to synthesize single-stranded cDNA. qRT-PCR was conducted using the cDNA templates generated from the reverse transcription reaction and 14 pairs of specific primers (see Table 1). The products were detected using the StepOnePlus™ Real-Time PCR System (Thermo, USA). Some primers were sourced from literatures (Zhang et al., 2020), while the remaining primers (pFAD6、PTD4、PTD5a、PTD5b and PTD6) were designed using Primer Premier 5.0 software. The 18S rDNA gene was used as the internal control (Du et al., 2019). Primer efficiencies were validated via standard curves and data were processed using the 2-ΔΔCt method.
Statistical evaluations were conducted utilizing one-way analysis of variance (ANOVA), followed by Duncan’s post hoc test, to assess differences among groups. Before applying ANOVA, we assessed homogeneity of variances through Levene’s test. Each experiment was conducted in triplicate. Results are expressed as mean ± standard deviation (SD), with statistical significance established at P < 0.05.
Within 24 hours after inoculation, no significant differences were observed among groups (Figure 1). After this period, while the growth of P. tricornutum was significantly inhibited at a concentration of 1000.23 μM (Zn4), the growth trends in the other two treatment groups at varying Zn2+ concentrations were similar to that of the Zn1 group. Specifically, the growth of Zn3 group (70.88 μM) exhibited slight inhibition compared to the Zn1 group.
Zinc is an essential micronutrient for many enzymatic and metabolic processes in algae. Within the range of Zn2+ concentrations from 0.99 to 70.88 μM, P. tricornutum is capable of normal growth. Huang et al. (2022) also reported that P. tricornutum can tolerate Zn2+ at a concentration of 60 μM without any adverse effects on growth. However, excessive concentrations of Zn2+ can be toxic, leading to inhibited growth, as seen in the Zn4 group. The slight inhibition observed in the Zn3 group might be attributed to the Zn2+ concentration approaching the threshold of toxicity for this species and affecting essential physiological processes such as photosynthesis or enzyme activities, which are crucial for growth. Excessive Zn2+ displaces Mg2+ in chlorophyll and Rubisco carboxylase, impairing light capture and carbon fixation efficiency, ultimately inhibiting photosynthesis (Nowicka, 2022). The significant inhibition at 1000.23 μM could be due to the disruption of cellular homeostasis, leading to oxidative stress and damage to cellular components. This is in line with findings that high concentration of Zn2+ can induce the production of ROS, which can cause oxidative damage to lipids, proteins, and DNA (DalCorso, 2012; Hamed et al., 2017; Ajitha et al., 2021). To further elucidate the disruption of cellular homeostasis caused by ROS, we assessed the antioxidant levels of P. tricornutum under varying Zn2+ concentrations.
As illustrated in Figure 2a, by the 7th day of the experiment, MDA content in P. tricornutum cells showed a trend of initial increase followed by a decrease with rising Zn2+ concentrations. In particular, the MDA content in the Zn2 and Zn3 treatment groups increased to varying degrees compared to the Zn1 group. Conversely, the MDA content in the Zn4 group was significantly lower than that in the Zn3 group (P < 0.05). The superoxide dismutase (SOD) enzyme activity increased with increasing Zn2+ concentration (Figure 2b), with the Zn4 group exhibiting the highest enzyme activity. Additionally, as depicted in Figure 2c, GSH content exhibited a similar trend to that of MDA content. Notably, the GSH content of the Zn3 group was higher than those of the other treatment groups.
Figure 2. Effects of different Zn2+ concentrations on expression of zinc-related genes. The effect of different Zn2+ concentrations on the activities of (a) MDA content, (b) SOD enzymes, and (c) GSH content. The different letters indicate that there was signifcant diference between different treatment groups.
MDA serves as a biomarker for lipid peroxidation, reflecting the extent of cellular damage induced by ROS (Niki, 2008). There was a tendency for the MDA content to increase in the Zn2 and Zn3 treated groups compared to the Zn1 group, indicating that these concentrations induced cellular oxidative stress. Furthermore, the decrease in MDA content in Zn4 group, despite the high Zn2+ concentration, could be attributed to the downregulation of fatty acid-related genes, such as PFAD6 and PTD4 (Figure 3). This downregulation may reduce the availability of polyunsaturated fatty acids (PUFAs), which are more prone to lipid peroxidation (Table 2). Consequently, the reduction in TFAC and the downregulation of fatty acid desaturase genes may collectively contribute to the observed decrease in MDA levels. This suggests that P. tricornutum may adjust its fatty acid metabolism to mitigate oxidative stress under high Zn2+ conditions. Moreover, the trend of SOD enzyme activity increasing with Zn2+ concentration reinforces the notion of a complex response to oxidative stress. The highest SOD activity in the Zn4 group indicates that the cells attempted to mitigate the effects of high ROS levels. GSH serves as a critical antioxidant by scavenging ROS, stabilizing thiol-containing enzymes, and participating in the regeneration of reduced vitamin E, thereby reflecting the organism’s capacity to combat oxidative stress (Averill-Bates, 2023). The observed changes in GSH content and MDA levels suggest a relationship between oxidative stress and antioxidant defense mechanisms. The increased GSH content in the Zn3 group suggests an adaptive response to moderate zinc-induced oxidative stress.
Table 2. The fatty acid profiles (% of total fatty acids) and total fatty acid content (TFAC, mg g-1) of P. tricornutum cells under different Zn2+ concentrations on day 7.
Analysis of fatty acid composition on the seventh day of cultivation identified the main fatty acids across treatments as C14: 0 (5.29% to 6.64%), C16: 0 (10.83% to 16.11%), C16: 1n-7 (17.19% to 19.74%), and C20: 5n-3 (13.25% to 26.88%) (Table 2). Total fatty acid content (TFAC) exhibited a gradual decrease with increasing Zn2+ concentration, with the Zn3 and Zn4 groups showing a significant decrease compared to the Zn1 group (P < 0.05). The saturated fatty acid (SFA) and monounsaturated fatty acid (MUFA) contents of the Zn4-treated group were significantly increased, whereas polyunsaturated fatty acid (PUFA) and highly unsaturated fatty acid (HUFA) were significantly decreased (P < 0.05). The DHA to EPA ratio in the Zn4 group also significantly increased relative to the other groups (P < 0.05). As the concentration of Zn2+ increases, the TFAC shows a gradually declining trend, with those in the Zn3 and Zn4 groups being significantly lower than that in the Zn1 group (P < 0.05).
Alterations in fatty acid profiles under different Zn2+ concentrations are potentially linked to the cellular response to oxidative stress. Oxidative stress can modify membrane fatty acid composition to maintain appropriate cell membrane fluidity and ensure normal membrane physiological functions. Akladious and Mohamed (2017) used sunflower as a study material and found that Zn stress significantly altered the fatty acid composition in sunflower leaves. Singh et al. (2002) and others explored the changes in lipid and fatty acid profiles in cyanobacteria under stress conditions. In the present study, the total fatty acid content (TFAC) exhibited a gradual decline with increasing Zn2+ concentration, especially in the Zn4 group, showing a significant decrease compared to the Zn1 group. In addition, SFA and MUFA significantly increased and PUFA and HUFA contents significantly decreased in the Zn4 group compared with the other groups, which may be related to the impact of zinc stress on the structure and function of cell membranes. The DHA/EPA ratio was calculated as the ratio of DHA to EPA content, and the DHA/EPA ratio increased by 7.7-fold in Zn4 compared to Zn1 (Table 2), which may indicate alterations in the activities of fatty acid desaturases under different Zn2+ concentrations, thereby affecting both the synthesis and desaturation processes of fatty acids (Coughlan et al., 2022).
Figure 3 shows the expression levels of five fatty acid desaturase genes under different Zn2+ concentrations. These genes include PFAD6, PTD4, PTD5a, PTD5b, and PTD6, which play crucial roles in fatty acid metabolism. PFAD6 is involved in the conversion of C16:2n-4 to C16:3n-4, increasing the unsaturation of fatty acids. PTD4 and PTD5a/b are involved in the elongation and desaturation of fatty acids, leading to the production of eicosapentaenoic acid (EPA) and docosahexaenoic acid (DHA). PTD6 is also involved in the desaturation process. Under the zinc stress, the relative expression levels of five fatty acid desaturase genes showed a trend of first increasing and then decreasing (P < 0.05). Among them, PFAD6 and PTD4 genes had the highest expression levels under the concentration of Zn2. The expression of PFAD6 gene was the lowest at the Zn2+ concentration of Zn4, which was significantly lower than that of the Zn1 group. The expression of PTD4 gene under the concentration of Zn4 was also significantly lower than those of the Zn2 and Zn3 groups (P < 0.05); However, there was no significant difference compared to the Zn1 group (P > 0.05). The Zn2+ concentration of Zn3 can significantly increase the expression of PTD5a, PTD5b and PTD6. However, the expression of all three genes reached the lowest level at the Zn4 group, which was significantly lower than that of the Zn1 group. This is consistent with the trend in fatty acid composition, where a reduction in desaturase expression led to a decrease in unsaturated fatty acid content in the Zn4 group.
Figure 3. Effects of different Zn2+ concentrations on expression of fatty acid desaturases. The different letters indicate that there was signifcant diference between different treatment groups.
Fatty acid desaturases play critical roles in the regulation of cell membrane lipid composition and the adaptation to environmental stresses (Xiao et al., 2022). In the present study, P. tricornutum may adapt to oxidative stress by regulating these genes, and thereby change its fatty acid desaturation capacity to maintain membrane fluidity and stability (Goodarzi et al., 2020). Domergue et al. (2002) demonstrated that PTD5 can adapt to a variety of substrates and efficiently carry out desaturation reactions to synthesize EPA through pulse experiments. The most well-known one is the desaturation of C20: 4n-3 to produce EPA (Smith et al., 2021). In the present study, a marked downregulation of PTD5a and PTD5b expression was observed in the Zn4 group (Figure 3), which corresponded with a significant reduction in the relative content of EPA. Consequently, this led to an increased DHA/EPA ratio (Table 2). A small amount of DHA can be synthesized in P. tricornutum. PTD4 primarily collaborates with certain elongases to elongate and desaturate ARA (C20: 4n-6) and EPA, resulting in the production of DPA (C22: 5n-3) and DHA (C22: 6n-3) (Dolch and Maréchal, 2015; Shanab et al., 2018). Corresponding to alterations in fatty acid content, the expression of PTD4 was significantly downregulated in the Zn4 treatment group (Figure 3), which was associated with a notable reduction in DPA and DHA levels compared to the control group. This reduction subsequently led to a decrease in the content of HUFAs (Table 2). PFAD6 is predominantly localized on the inner membrane of chloroplasts and belongs to the prokaryotic pathway of fatty acid metabolism. This enzyme primarily facilitates the conversion of C16: 2n-4 to C16: 3n-4, thereby increasing the unsaturation of fatty acids, which is crucial for preserving chloroplast membrane fluidity (Dolch and Maréchal, 2015; Zhu et al., 2018). Notably, PFAD6 (a chloroplast-localized desaturase) exhibited peak expression at Zn2 (70.88 μM), followed by a sharp decline at higher concentrations (Figure 3). This sensitivity suggests that chloroplast membrane fluidity is preferentially compromised under zinc stress, potentially explaining the growth inhibition observed in Zn3 (Figure 1). In summary, the changes in fatty acid desaturase and fatty acid composition were consistent, and zinc stress led to changes in fatty acid desaturase and fatty acid composition, which may be one of the strategies of P. tricornutum to adapt to zinc stress.
To further elucidate the effects of Zn2+ concentration on the P. tricornutum cells, we assessed the expression of genes related to Zn2+ transport, chelation, and excretion. Generally, Zn2+ was transported into cell by the transmembrane zinc transporter (ZUPT) and two-pore calcium channel (TPC1A) (Grass et al., 2002; Zhang et al., 2020). ZUPT was significantly upregulated at Zn3 group (P < 0.05), which suggested enhanced Zn2+ uptake at this concentration (Figure 4). However, TPC1A exhibited a significant decrease in the Zn4 group, indicating that high concentrations of Zn2+ downregulated calcium ion channels to reduce cellular uptake of Zn2+. The genes of gamma glutamylcysteine synthetase (γ-ECS), glutathione synthase (GS), and phytochelatin synthase (PCs) play an important role in heavy metal tolerance and detoxification by synthesizing glutathione and phytochelatin (Ducruix et al., 2006; Kumar et al., 2016). These genes underwent a trend of first increasing and then decreasing, with GS and PCs significantly decreasing in the Zn4 group (P < 0.05). Phytochelatins often chelate heavy metal ions in the vacuole, so the expression levels of vacuolar iron transporter (VIT) and ATP-binding cassette transporter (ABCT) responsible for the transport of Zn2+ from the cytoplasm to the vacuole were assessed (Salt et al., 2008; Labarbuta et al., 2017). The expression of the ABCT gene showed no significant differences among all groups (P > 0.05), while the VIT gene was significantly upregulated in the Zn3 group (P < 0.05), increasing nearly 4.8 times compared to the Zn1 group. Free Zn2+ in the cytoplasm can be exported from the cell through P1B-type ATPase transporters (HMA2) (Kushwaha et al., 2018; Li et al., 2022). We observed that HMA2 also underwent a trend of first increasing and then decreasing, with the Zn3 group significantly higher than the other groups (P < 0.05).
Figure 4. Effects of different Zn2+ concentrations on expression of zinc-related genes. The different letters indicate that there was signifcant diference between different treatment groups.
From the above results, it can be speculated that the expression levels of ZUPT and TPC1A genes gradually increase with increasing Zn2+ concentration to enhance zinc uptake (Figure 4), but the uptake channel is downregulated when the concentration of Zn2+ exceeds the cell’s tolerance level. With the increase in Zn2+ intake, the cells also activate their antioxidant system to counteract the oxidative stress caused by Zn2+, as mainly reflected in the rising trends of SOD and GSH (Figures 2b, c), as well as the high expression of GS and PCs (Figure 4). The increase in GSH is beneficial for promoting the function of PCs, which is consistent with previous reports (Leitenmaier and Küpper, 2013; Kushwaha et al., 2018). Meanwhile, the detoxification effect of vacuoles on heavy metals has also been enhanced, with extremely high levels of VIT gene expression (Figure 4). Studies have shown that plants regulate the accumulation and sequestration of heavy metals through transport proteins in the vacuole, thereby reducing their toxicity (Jogawat et al., 2021). The high expression of the VIT gene involved in the transport and storage mechanisms of metals is closely associated with heavy metal tolerance (Thapa et al., 2012). As another transport protein on the vacuole, ABCT shows a non-linear relationship with Zn2+ concentration, indicating that this gene may not be involved in the transport of Zn2+. While detoxifying in the vacuole, the cells also activate Zn2+ efflux on the plasma membrane, as indicated by the high expression of HMA2, which is consistent with previous reports (Zhang et al., 2020) (Figure 4).
Our study demonstrated that appropriate Zn2+ concentrations promote the growth and production of antioxidant activities in P. tricornutum, whereas Zn2+ concentrations that reach a threshold inhibit algal growth and lead to oxidative stress. To counteract this oxidative stress, P. tricornutum cells increased the expression of antioxidant enzymes, enhanced heavy metal detoxification, upregulated the expression of fatty acid desaturases, and altered fatty acid composition to improve membrane fluidity. In conclusion, this study elucidates the response of P. tricornutum to different Zn2+ concentrations, emphasizing the critical need for monitoring and managing Zn2+ concentrations in aquatic environments. However, the study’s focus on short-term responses to of P. tricornutum zinc stress highlights the need for future research to explore long-term adaptation mechanisms and the responses of other phytoplankton species. Such studies will provide a more comprehensive understanding of the impacts of zinc pollution on diverse aquatic communities and enhance the applicability of the findings to broader ecosystems.
The original contributions presented in the study are included in the article. Further inquiries can be directed to the corresponding author.
NM: Investigation, Data curation, Project administration, Formal analysis, Writing – original draft. QS: Data curation, Visualization, Formal analysis, Writing – review & editing. PS: Methodology, Investigation, Writing – review & editing. SD: Investigation, Writing – review & editing. HQ: Conceptualization, Methodology, Formal analysis, Supervision, Writing – review & editing. YX: Formal analysis, Supervision, Writing – review & editing.
The author(s) declare financial support was received for the research, authorship, and/or publication of this article. This research was funded by the Shandong Provincial Natural Science Foundation of Shandong Province (ZR2024MC078), Fund of Yantai Key Laboratory of Quality and Safety Control and Deep Processing of Marine Food (QSCDP202302), National Natural Science Foundation of China (grant No. 32072997), the Science and Technology Innovation Development Plan of Yantai (2024ZDCX024) and Talent Induction Program for Youth Innovation Teams in Colleges and Universities of Shandong Province (2022-2024). The funders had no role in study design, data collection and analysis, decision to publish, or preparation of the manuscript.
We thank Prof. Xianghong Gong for experimental techniques guidance.
The authors declare that the research was conducted in the absence of any commercial or financial relationships that could be construed as a potential conflict of interest.
The authors declare that no Generative AI was used in the creation of this manuscript.
All claims expressed in this article are solely those of the authors and do not necessarily represent those of their affiliated organizations, or those of the publisher, the editors and the reviewers. Any product that may be evaluated in this article, or claim that may be made by its manufacturer, is not guaranteed or endorsed by the publisher.
Ajitha V., Sreevidya C. P., Sarasan M., Park J. C., Mohandas A., Singh I. S. B., et al. (2021). Effects of zinc and mercury on ROS-mediated oxidative stress-induced physiological impairments and antioxidant responses in the microalga Chlorella vulgaris. Environ. Sci. pollut. Res. Int. 28, 32475–32492. doi: 10.1007/s11356-021-12950-6
Akladious S. A., Mohamed H. I. (2017). Physiological role of exogenous nitric oxide in improving performance, yield and some biochemical aspects of sunflower plant under zinc stress. Acta Biol. Hung 68, 101–114. doi: 10.1556/018.68.2017.1.9
Averill-Bates D. A. (2023). The antioxidant glutathione. Vitam Horm. 121, 109–141. doi: 10.1016/bs.vh.2022.09.002
Bradford M. M. (1976). A rapid and sensitive method for the quantitation of microgram quantities of protein utilizing the principle of protein-dye binding. Anal. Biochem. 72, 248–254. doi: 10.1016/0003-2697(76)90527-3
Cakmak I. (2000). Tansley Review No. 111 Possible roles of zinc in protecting plant cells from damage by reactive oxygen species. New Phytol. 146, 185–205. doi: 10.1046/j.1469-8137.2000.00630.x
Chan A., Salsali H., McBean E. (2014). Heavy metal removal (Copper and zinc) in secondary effluent from wastewater treatment plants by microalgae. ACS Sustain. Chem. Eng. 2, 130–137. doi: 10.1021/sc400289z
Chen X., Lin H., Dong Y., Li B., Yin T., Liu C. (2022). Simultaneous high-efficiency removal of sulfamethoxazole and zinc (II) from livestock and poultry breeding wastewater by a novel dual-functional bacterium, Bacillus sp. SDB4. Environ. Sci. pollut. R 29, 6237–6250. doi: 10.1007/s11356-021-15804-3
Chong A. M. Y., Wong Y. S., Tam N. F. Y. (2000). Performance of different microalgal species in removing nickel and zinc from industrial wastewater. Chemosphere 41, 251–257. doi: 10.1016/S0045-6535(99)00418-X
Coughlan R., Moane S., Larkin T. (2022). Variability of essential and nonessential fatty acids of Irish rapeseed oils as an indicator of nutritional quality. Int. J. Food Sci. 2022, 7934565. doi: 10.1155/2022/7934565
DalCorso G. (2012). “Heavy Metal Toxicity in Plants,” in Plants and Heavy Metals (Dordrecht: Springer), 1–25. doi: 10.1007/978-94-007-4441-7_1
Damseth S., Thakur K., Kumar R., Kumar S., Mahajan D., Kumari H., et al. (2024). Assessing the impacts of river bed mining on aquatic ecosystems: A critical review of effects on water quality and biodiversity. HydroResearch 7, 122–130. doi: 10.1016/j.hydres.2024.01.004
De Filippis L. F. (1979). The effect of heavy metal compounds on the permeability of chlorella cells. Z. für Pflanzenphysiologie 92, 39–49. doi: 10.1016/S0044-328X(79)80151-8
do Carmo e Sá M., Pezzato L. E., Ferreira Lima M. M. B., de Magalhães Padilha P. (2004). Optimum zinc supplementation level in Nile tilapia Oreochromis niloticus juveniles diets. Aquaculture 238, 385–401. doi: 10.1016/j.aquaculture.2004.06.011
Dolch L. J., Maréchal E. (2015). Inventory of fatty acid desaturases in the pennate diatom Phaeodactylum tricornutum. Mar. Drugs 13, 1317–1339. doi: 10.3390/md13031317
Domergue F., Lerchl J., Zähringer U., Heinz E. (2002). Cloning and functional characterization of Phaeodactylum tricornutum front-end desaturases involved in eicosapentaenoic acid biosynthesis. Eur. J. Biochem. 269, 4105–4113. doi: 10.1046/j.1432-1033.2002.03104.x
Du B., Zhang Z., Liu W., Ye Y., Lu T., Zhou Z., et al. (2019). Acute toxicity of the fungicide azoxystrobin on the diatom Phaeodactylum tricornutum. Ecotox Environ. Safe 168, 72–79. doi: 10.1016/j.ecoenv.2018.10.074
Ducruix C., Junot C., Fiévet J. B., Villiers F., Ezan E., Bourguignon J. (2006). New insights into the regulation of phytochelatin biosynthesis in A. thaliana cells from metabolite profiling analyses. Biochimie 88, 1733–1742. doi: 10.1016/j.biochi.2006.08.005
Eckardt C. B., Pearce G. E. S., Keely B. J., Kowalewska G., Jaffé R., Maxwell J. R. (1992). A widespread chlorophyll transformation pathway in the aquatic environment. Organic Geochemistry 19, 217–227. doi: 10.1016/0146-6380(92)90038-Y
Goodarzi A., Namdjoyan S., Soorki A. A. (2020). Effects of exogenous melatonin and glutathione on zinc toxicity in safflower (Carthamus tinctorius L.) seedlings. Ecotoxicol Environ. Safe 201, 110853. doi: 10.1016/j.ecoenv.2020.110853
Grass G., Wong M. D., Rosen B. P., Smith R. L., Rensing C. (2002). ZupT is a Zn (II) uptake system in Escherichia coli. J. Bacteriol 184, 864–866. doi: 10.1128/jb.184.3.864-866.2002
Guillard R. R. L., Ryther J. H. (1962). Studies of marine planktonic diatoms. I. Cyclotella nana Hustedt, and Detonula confervacea (cleve) Gran. Can. J. Microbiol. 8, 229–239. doi: 10.1139/m62-029
Hamed S. M., Zinta G., Klöck G., Asard H., Selim S., AbdElgawad H. (2017). Zinc-induced differential oxidative stress and antioxidant responses in Chlorella sorokiniana and Scenedesmus acuminatus. Ecotox Environ. Safe 140, 256–263. doi: 10.1016/j.ecoenv.2017.02.055
Hardy R. W., Sullivan C. V., Koziol A. M. (1987). Absorption, body distribution, and excretion of dietary zinc by rainbow trout (Salmo gairdneri). Fish Physiol. Biochem. 3, 133–143. doi: 10.1007/bf02180415
Huang A., Wang Y., Duan J., Guo S., Xie Z. (2022). Differential Response of Phaeodactylum tricornutum and Cylindrotheca fusiformis to High Concentrations of Cu2+ and Zn2+. Water 14, 3305. doi: 10.3390/w14203305
Huang W. Q., Zhou Y. P., Tan L. J., Wang J. T. (2019). Effect of heavy metal Zinc on the output of amino acids in marine algae. Mar. Sci. 43, 11–18. doi: 10.11759/hykx20190126002
Jogawat A., Yadav B., Chhaya, Narayan O. P. (2021). Metal transporters in organelles and their roles in heavy metal transportation and sequestration mechanisms in plants. Physiol. Plant 173, 259–275. doi: 10.1111/ppl.13370
Kappus H. (1985). Lipid peroxidation: mechanisms, analysis, enzymology and biological relevance. Oxid. Stress 273, 273–310. doi: 10.1016/B978-0-12-642760-8.50016-8
Kumar A., Dixit G., Singh A. P., Dwivedi S., Srivastava S., Mishra K., et al. (2016). Selenate mitigates arsenite toxicity in rice (Oryza sativa L.) by reducing arsenic uptake and ameliorates amino acid content and thiol metabolism. Ecotoxicol Environ. Safe 133, 350–359. doi: 10.1016/j.ecoenv.2016.06.037
Küpper H., Küpper F., Spiller M. (1998). In situ detection of heavy metal substituted chlorophylls in water plants. Photosynth Res. 58, 123–133. doi: 10.1023/A:1006132608181
Kushwaha A., Hans N., Kumar S., Rani R. (2018). A critical review on speciation, mobilization and toxicity of lead in soil-microbe-plant system and bioremediation strategies. Ecotoxicol Environ. Safe 147, 1035–1045. doi: 10.1016/j.ecoenv.2017.09.049
Labarbuta P., Duckett K., Botting C. H., Chahrour O., Malone J., Dalton J. P., et al. (2017). Recombinant vacuolar iron transporter family homologue PfVIT from human malaria-causing Plasmodium falciparum is a Fe (2+)/H (+) exchanger. Sci. Rep. 7, 42850. doi: 10.1038/srep42850
Leitenmaier B., Küpper H. (2013). Compartmentation and complexation of metals in hyperaccumulator plants. Front. Plant Sci. 4. doi: 10.3389/fpls.2013.00374
Li T., Li J., Zhan X., Wang X., He B., Cao F., et al. (2022). Application of exogenous iron alters the microbial community structure and reduces the accumulation of cadmium and arsenic in rice (Oryza sativa L.). Nanomaterials (Basel) 12 (8):1311. doi: 10.3390/nano12081311
López-Millán A. F., Ellis D. R., Grusak M. A. (2005). Effect of zinc and manganese supply on the activities of superoxide dismutase and carbonic anhydrase in Medicago truncatula wild type and raz mutant plants. Plant Sci. 168, 1015–1022. doi: 10.1016/j.plantsci.2004.11.018
Mallick N., Mohn F. H. (2003). Use of chlorophyll fluorescence in metal-stress research: a case study with the green microalga Scenedesmus. Ecotoxicol Environ. Safe 55, 64–69. doi: 10.1016/s0147-6513(02)00122-7
Nable R. O. (1988). Resistance to boron toxicity amongst several barley and wheat cultivars: A preliminary examination of the resistance mechanism. Plant Soil 112, 45–52. doi: 10.1007/BF02181751
Niki E. (2008). Lipid peroxidation products as oxidative stress biomarkers. Biofactors 34, 171–180. doi: 10.1002/biof.5520340208
Nowicka B. (2022). Heavy metal–induced stress in eukaryotic algae—mechanisms of heavy metal toxicity and tolerance with particular emphasis on oxidative stress in exposed cells and the role of antioxidant response. Environ. Sci. pollut. R 29, 16860–16911. doi: 10.1007/s11356-021-18419-w
Paschoalini A. L., Bazzoli N. (2021). Heavy metals affecting Neotropical freshwater fish: A review of the last 10 years of research. Aquat Toxicol. 237, 105906. doi: 10.1016/j.aquatox.2021.105906
Plekhanov S. E., Chemeris Y. K. (2003). Early toxic effects of zinc, cobalt, and cadmium on photosynthetic activity of the green alga Chlorella pyrenoidosa Chick S-39. Biol. Bull. 30, 506–511. doi: 10.1023/A:1025806921291
Qiao H., Wang J., Zhang L., Sun C., Ma J., Song Z., et al. (2015). An improved direct transesterification method for fatty acid determination of Phaeodactylum tricornutum. J. Appl. Phycology 27, 697–701. doi: 10.1007/s10811-014-0376-5
Salt D. E., Baxter I., Lahner B. (2008). Ionomics and the study of the plant ionome. Annu. Rev. Plant Biol. 59, 709–733. doi: 10.1146/annurev.arplant.59.032607.092942
Shahpar Z., Johari S. A. (2019). Effects of dietary organic, inorganic, and nanoparticulate zinc on rainbow trout, Oncorhynchus mykiss larvae. Biol. Trace Elem Res. 190, 535–540. doi: 10.1007/s12011-018-1563-z
Shanab S. M. M., Hafez R. M., Fouad A. S. (2018). A review on algae and plants as potential source of arachidonic acid. J. Adv. Res. 11, 3–13. doi: 10.1016/j.jare.2018.03.004
Singh S., Sinha R. P., Haeder D. P. (2002). Role of lipids and fatty acids in stress tolerance in Cyanobacteria. Acta Protozool 41, 297–308.
Smith R., Jouhet J., Gandini C., Nekrasov V., Marechal E., Napier J. A., et al. (2021). Plastidial acyl carrier protein Δ9-desaturase modulates eicosapentaenoic acid biosynthesis and triacylglycerol accumulation in Phaeodactylum tricornutum. Plant J. 106, 1247–1259. doi: 10.1111/tpj.15231
Thapa G., Sadhukhan A., Panda S. K., Sahoo L. (2012). Molecular mechanistic model of plant heavy metal tolerance. BioMetals 25, 489–505. doi: 10.1007/s10534-012-9541-y
Tripathi B. N., Gaur J. P. (2004). Relationship between copper- and zinc-induced oxidative stress and proline accumulation in Scenedesmus sp. Planta 219, 397–404. doi: 10.1007/s00425-004-1237-2
Weckx J., Clijsters H. (1997). Zn phytotoxicity induces oxidative stress in primary leaves of Phaseolus vulgaris. Plant Physiol. Bioch 35, 405–410.
Xiao R., Zou Y., Guo X., Li H., Lu H. (2022). Fatty acid desaturases (FADs) modulate multiple lipid metabolism pathways to improve plant resistance. Mol. Biol. Rep. 49, 9997–10011. doi: 10.1007/s11033-022-07568-x
Zhang X., Xu D., Huang S., Wang S., Han W., Liang C., et al. (2020). The effect of elevated pCO2 on cadmium resistance of a globally important diatom. J. Hazard Mater 396, 122749. doi: 10.1016/j.jhazmat.2020.122749
Keywords: Phaeodactylum tricornutum, fatty acids, zinc stress, antioxidant activity, gene expression
Citation: Ma N, Su Q, Song P, Dong S, Qiao H and Xu Y (2025) Effects of different Zn2+ levels on antioxidant activity, fatty acid composition, and related gene expression in Phaeodactylum tricornutum. Front. Mar. Sci. 12:1562111. doi: 10.3389/fmars.2025.1562111
Received: 17 January 2025; Accepted: 17 February 2025;
Published: 10 March 2025.
Edited by:
Carlos Gravato, University of Lisbon, PortugalReviewed by:
Tiago Filipe Simões, Polytechnic Institute of Leiria, PortugalCopyright © 2025 Ma, Su, Song, Dong, Qiao and Xu. This is an open-access article distributed under the terms of the Creative Commons Attribution License (CC BY). The use, distribution or reproduction in other forums is permitted, provided the original author(s) and the copyright owner(s) are credited and that the original publication in this journal is cited, in accordance with accepted academic practice. No use, distribution or reproduction is permitted which does not comply with these terms.
*Correspondence: Hongjin Qiao, cWlhb2hqQGxkdS5lZHUuY24=; Yingjiang Xu, eHV5aW5namlhbmcyMDIwQDE2My5jb20=
Disclaimer: All claims expressed in this article are solely those of the authors and do not necessarily represent those of their affiliated organizations, or those of the publisher, the editors and the reviewers. Any product that may be evaluated in this article or claim that may be made by its manufacturer is not guaranteed or endorsed by the publisher.
Research integrity at Frontiers
Learn more about the work of our research integrity team to safeguard the quality of each article we publish.