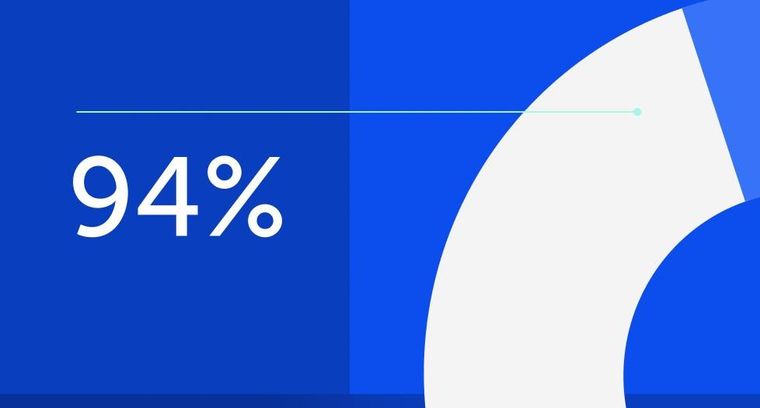
94% of researchers rate our articles as excellent or good
Learn more about the work of our research integrity team to safeguard the quality of each article we publish.
Find out more
REVIEW article
Front. Mar. Sci., 14 April 2025
Sec. Deep-Sea Environments and Ecology
Volume 12 - 2025 | https://doi.org/10.3389/fmars.2025.1547803
This article is part of the Research TopicEnvironmental Impacts & Risks of Deep-Sea Mining: Recommendations for Exploitation RegulationsView all 10 articles
Despite its remoteness, the deep sea is not spared from the impact of human activities. The emergence of industrial-scale deep-sea mining of polymetallic nodules on the abyssal plains (4-5 km depth) of the Clarion-Clipperton fracture zone in the equatorial Pacific is potentially the most threatening undertaking at present time. A primary aspect that must be comprehensively assessed in order to safeguard these abyssal benthic communities through the designation of marine protected areas and the spatial organization of prospective mining fields is connectivity, i.e., the continuity of species distributions in space and time. In this review we provide the current state of knowledge on connectivity through an examination of published literature focused on several animal groups from all benthic faunal size classes (megafauna, macrofauna, meiofauna) as well as Foraminifera and microbes. We highlight the main challenges associated with assessing connectivity in the deep sea and outline the key parameters required to achieve the idealised connectivity study for fauna and microbes.
The deep sea, defined as marine areas beyond the continental shelf at depths exceeding 200 m, is the largest continuous biome on Earth, amounting to over 95% of the global ocean volume (Danovaro et al., 2020; Webb et al., 2010). While largely inaccessible to most people, all humans benefit from ecosystem services provided by the deep sea, such as climate regulation, carbon sequestration, nutrient cycling and global fish stocks (Thurber et al., 2014; Webb et al., 2010). This largely unexplored environment hosts an astounding amount of biodiversity, potentially unmatched by any other on the planet (Ramirez-Llodra et al., 2010). Abyssal ecosystems are found at depths from 4-6 km, consisting mainly of soft sediments and covering 75% of the global ocean floor, of which less than 1% has been thoroughly investigated (Hughes et al., 2021; Ramirez-Llodra et al., 2010). Despite their remoteness, abyssal plains have not been spared from anthropogenic threats such as climate change, litter and deep seabed mining (Levin and Bris, 2015). Rising ocean temperatures are already altering large-scale deep water mass circulation patterns (e.g., Ditlevsen and Ditlevsen, 2023) while a warmer ocean is expected to cause severe declines in oxygen saturation (Deutsch et al., 2024; Levin, 2017) and nutrient export from surface waters (Smith et al., 2009; Sweetman et al., 2017), leading to global decreases in abyssal benthic biomass by the end of the century (Jones et al., 2014). Ocean acidification is causing a shallowing of the carbonate compensation depth, an important biogeographic boundary in the abyss (Simon-Lledó et al., 2023) by nearly 100 m since pre-industrial times, and is expected to rise several hundred meters more this century (Sulpis et al., 2018; Harris et al., 2023). These environmental changes have the potential to cause significant shifts in the availability of suitable habitats across large spatial scales, dramatically altering connectivity, i.e., the spatiotemporal continuity of abyssal species distributions.
Halting the current loss of biodiversity is of highest priority in order to ensure the continuation of the provision of the vital ecosystem services and functions that humans depend on. This urgency is reflected in several global initiatives such as the United Nations (UN) 17 Sustainable Development Goals (and notably SDG14, https://www.un.org/sustainabledevelopment/oceans/), the Rio Convention of Biological Diversity (https://www.cbd.int/) and the UN Agreement on Marine Biological Diversity of Areas beyond National Jurisdiction (BBNJ, https://www.un.org/bbnjagreement/en); the latter being especially relevant to deep seabed mining (DSM) since the majority of mineral resources lie beyond national waters. In the case of the deep-sea realm in particular, which lacks distinctly visible boundaries such as those of terrestrial environments, our ability to conserve biodiversity is inextricably linked not only to the identification of species but also to their distribution and how they are connected between the different locations where they occur. Thus, understanding connectivity is crucial for the assessment of vulnerability and for defining mitigation measures in threatened habitats.
The deep sea is currently being targeted for the potential future extraction of three types of mineral deposits: polymetallic nodules found at abyssal plains (PMN), cobalt-rich ferromanganese crusts on seamounts (CFC), and polymetallic massive sulfides at hydrothermal vents (PMS). In this review we focus on polymetallic nodules, which form via the precipitation of various minerals such as manganese, nickel, copper, and cobalt from the seawater (hydrogenetic) and/or the sediment pore water (diagenic) onto an organic nucleus (Hein et al., 2020; Thiel, 2005). Their formation is one of the slowest known geological processes (Dutkiewicz et al., 2020), progressing at a rate of 2-10 mm/million years; effectively, a non-renewable resource by human standards (Boetius and Haeckel, 2018; Verlaan and Cronan, 2022). The nodules are found at the surface of soft sediments on abyssal plains in the Pacific, Atlantic and Indian oceans. The polymetallic nodule fields in the Clarion-Clipperton Fracture Zone (CCZ, Figure 1) in the Pacific cover huge expanses of the seafloor; up to ca. 80% the size of the contiguous United States of America (Wedding et al., 2015). Dispersal barriers such as large and continuous topographical structures (e.g., submarine mountain ridges) and/or steep physical gradients (e.g., temperature, pressure, oxygen) are absent, meaning that the connectivity of benthic communities could operate across thousands of km. Our understanding of fundamental processes, such as dispersal and connectivity, that regulate biodiversity at such large scales, is severely limited; thus, synthesizing our currently available knowledge across all benthic size classes [i.e., microbes (<32 µm), meiofauna (> 32 µm), macrofauna (>300 µm), megafauna (>1 cm), Figure 2], is critical to guide conservation efforts as well as future research.
Figure 1. Map of the Clarion-Clipperton Fracture Zone with designated exploration contract areas and Areas of Particular Environmental Interest (APEI). Source: ISA, October 2024.
Figure 2. Fauna of the Clarion-Clipperton zone. Left to right, top to bottom: (A) Amperima sp. indet, (B) Ectinosomatidae gen. indet, (C) Ophiotholia saskia, (D) Corallimorphus sp. indet, (E) Euplectellidae gen. indet, (F) Salix daleus, (G) Ceramonema sp., (H) Bathystylodactylus echinus. Image credits: (A, D, E, H) Courtesy of/extracted from Simon-Lledo et al., 2023 (https://zenodo.org/records/8172728); (B) Katja Uhlenkott; (C, F): Magdalini Christodoulou; (G): Tania Campinas Bezerra.
The umbrella-term “connectivity” is used ubiquitously to refer to (i) genetic, (ii) demographic, (iii) biological, (iv) ecological, (v) structural, and/or (vi) functional connectivity (Selkoe et al., 2016). All seek to describe and understand the continuity of species distributions in space and time via different avenues. Beta diversity, which is defined as the shift in species composition among sites (Whittaker, 1960), can be used as an indirect measure of connectivity between communities and more generally to study biogeography (i.e., distribution of taxa across space and time). The biogeography of organisms is controlled by four fundamental processes: dispersal, ecological drift, selection, and mutation (Hanson et al., 2012). It is thought that the scale, direction, frequency and intensity of species dispersal is most influential for deep-sea connectivity (Baco et al., 2016; Hilário et al., 2015). The interaction of these processes manifests in two major biogeographical patterns: the taxa-area relationship, i.e., the positive relationship between the number of taxa in an area and the size of that area (Arrhenius, 1921); and the distance-decay relationship, i.e., the decline in community similarity over increasing geographical distance (Nekola and White, 1999). Several studies have identified taxa-area and distance-decay relationships in marine microbes, with stronger patterns in heterogeneous and island-like habitats than in continuous ones (Zinger et al., 2011, 2014). Microbial dispersal is shaped by intrinsic taxon-specific traits (e.g., spore formation, morphology, habitat specificity) and extrinsic factors such as population size (Anderson et al., 2015), topographic continuity (Schauer et al., 2010; Bienhold et al., 2016), and environmental gradients (Bienhold et al., 2012; Ruff et al., 2015; Varliero et al., 2019; Ramírez et al., 2019; Orcutt et al., 2021). Although differences in environmental factors (e.g., particulate organic carbon flux to the seafloor, water depth, and sediment composition) that can potentially affect microbial biogeography are known to exist across the CCZ (Washburn et al., 2021a), the study of microbial connectivity is hindered by the paucity of knowledge regarding the diversity of microbes inhabiting the seafloor.
Pelagic larval duration (PLD, the amount of time a larva spends in the water column before settlement), or the more inclusive pelagic propagule duration (PPD, including the embryonic phase) greatly modulates dispersal capacity as currents can transport taxa hundreds of km from their birth place. Although it has been suggested that marine connectivity is typically underestimated (Manel et al., 2019), a significant gap of knowledge with respect to PPD of deep-sea taxa hinders even the most simplistic estimates of dispersal. By knowing the PPD and the speed and direction of currents we can estimate the upper limit of dispersal distance and infer probable trajectories (Hilário et al., 2015). However, due to the scarcity of molecular data, and data on life histories of CCZ fauna, circulation, accurate modelling and precise validation it is currently impossible to accurately predict connectivity in this region. In addition to PPD, knowledge of other biological traits, such as fecundity, spawning periodicity and seasonality are necessary to validate biophysical models. Likewise, we are unable to identify which areas offer high quality habitat for any given taxon and thus able to foster population growth (i.e., sources) and which are low quality habitat, in themselves unable to sustain a population without being linked to a source (i.e., sinks) (Pulliam, 1988).
Inferring potential dispersal trajectories has traditionally relied on Lagrangian particle tracking which models the movement of neutrally buoyant particles in a fluid (Viegas et al., 2024; Young et al., 2012). Generating these models is challenging in deep-sea ecosystems for which water-current and high-resolution bathymetrical mapping is limited (Hilário et al., 2015). In fact, for taxa with long-distance dispersal capacities, connectivity is more important than habitat quality for the successful design of a Marine Protected Area (MPA) network (Berglund et al., 2012). In practice however, connectivity is one of the least frequently applied ecological criteria for the designation of MPAs despite its importance for securing conservation of biodiversity, sustainability, population resilience and persistence (Balbar and Metaxas, 2019). In this context, “ecological connectivity” has now been listed in the BBNJ agreement as an indicative criterium for the establishment of MPAs. Many challenges persist, such as the paucity of genetic data, disagreements on how to apply genetic metrics to inform policy and the aptly-described research-implementation gap between those who generate (geneticists) and analyze data (bioinformaticians) and those who use the data to inform management actions (ecologists) and decision-making (legislators) (Hogg, 2024).
Dispersal and thereby, connectivity, can be inferred through genetic methods. This approach is based on the assumption that genetic differences between specimens from different locations will be small if they are connected by dispersal and vice versa. The various DNA-based techniques (e.g., microsatellites, haplotype networks, genotypic fingerprinting, Single Nucleotide Polymorphisms [SNPs]) that are used typically require a minimum number of conspecifics from several locations in order to be informative with respect to population structure. Low faunal densities, restricted sampling, limited information on species distributions coupled to habitat heterogeneity, lack of time series covering seasonal and interannual variability, the largely undescribed diversity of the CCZ and cryptic speciation (i.e., distinct species that are morphologically identical) pose significant challenges to achieving population-level resolution. An additional complication arises from the high proportion of taxa found at just a single sampling location (singletons), a consistent pattern in morphological and DNA-based investigations of all size classes in the CCZ (e.g., Christodoulou et al., 2020; Hauquier et al., 2019; Macheriotou et al., 2020; Simon-Lledó et al., 2019; Wilson, 2017). Even though singletons could represent an artefact of under-sampling, by definition, connectivity can only be assessed for taxa that are present in at least two locations, resulting in a substantial gap of knowledge for a large fraction of these assemblages. For meiofaunal taxa, the most abundant metazoans in the CCZ, the inability to achieve species-level resolution significantly hinders investigation into population dynamics. This limitation makes it challenging to determine whether these taxa follow an “island” or “stepping-stone” model of population structure through Isolation-By-Distance (IBD) (Vrijenhoek, 1997), whether alternative modes of differentiation (e.g., “genomic islands” [Malinsky et al., 2015]) predominate, or whether broad dispersal with weak genetic structuring, consistent with the “everything is everywhere” hypothesis (Baas Becking, 1934), is in effect.
The nodule fields of the CCZ (Figure 1) are part of the “Area” (i.e., seabed and ocean floor and subsoil thereof, beyond the limits of national jurisdiction, Article 1, UNCLOS) which along with “its resources are the common heritage of mankind” (Article 136, UNCLOS) and thus “no State shall claim or exercise sovereignty or sovereign rights over any part of the Area or its resources” (Article 137, UNCLOS). The International Seabed Authority (ISA) is “the organization through which States Parties to UNCLOS organize and control all mineral-resources-related activities in the Area for the benefit of humankind as a whole” and “has the mandate to ensure the effective protection of the marine environment from harmful effects that may arise from deep-seabed-related activities.” (https://www.isa.org.jm/). To this end, eleven Areas of Particular Environmental Interest (APEIs) measuring 400 x 400 km have been placed along the outer perimeter of the CCZ (Figure 1), and two more in the central region. The latter two were designed taking into consideration boundaries of Exclusive Economic Zones and contract areas and therefore do not meet the size specified in the original criteria of the environmental management plan for the CCZ (ISA, 2021). The APEIs will be excluded from future mining operations (and the impacts thereof) and are meant to be representative of the habitats found in the CCZ in order to safeguard regional biodiversity as well as ecosystem structure and functioning (ISA, 2021; McQuaid et al., 2020). Moreover, the APEIs are intended “to maintain sustainable populations and to capture the full range of habitats and communities by being large enough to maintain minimum viable population sizes for species potentially restricted to a subregion of the Clarion-Clipperton Fracture Zone, without being affected by mining plumes from any activities immediately adjacent to an area” (ISBA/22/LTC/12, https://isa.org.jm/files/files/documents/isba-22ltc-12_1.pdf). For this to be possible, it must be proven that the APEIs are large enough to sustain viable populations themselves and/or are linked to another APEI via dispersal. Moreover, we maintain that similar considerations are needed for the areas that will potentially be mined in the future and should thus guide the assignment of the size and spatial layout of these sites. Of equal importance is the concurrent protection of the pelagic realm along with the seabed, as the water column is expected to be highly impacted by sediment plumes generated by the separation of nodules and sediments by the nodule collector (i.e., collector plume) and those derived from nodule washing aboard the surface vessel (i.e., return plume) (Drazen et al., 2020; Wedding et al., 2013). It is well-known that benthic productivity is subsidized by that of overlaying surface waters in the form of detritus, nutrients and prey; thus any effort to protect the former must include the latter in order to be effective (O’Leary and Roberts, 2018).
Investigating any aspect of biodiversity in the CCZ is a challenging endeavor due to the remoteness of the region and the need for large research vessels, sophisticated equipment and trained personnel to obtain samples from such depth. Moreover, connectivity in the marine realm is particularly complicated because dispersal “is arguably the least understood demographic process in the sea” (Swearer et al., 2019). The transport of an individual from its birth to recruit location, takes place in a three-dimensional space which is influenced by the movement of water masses on a local and global scale as well as behavior, developmental mode, ontogenetic vertical migration, embryo and larval buoyancy, predation, food availability, developmental rate, longevity, and physiological tolerances. These processes have been studied for very few deep-sea animals, and are restricted to species that occur in densities large enough to allow for systematic analyses. Dispersal of less abundant species can only be addressed by assuming similarities to model organisms to which they are related to, or share characteristics with. The vast majority of marine benthic taxa have a free-living dispersive larval stage, while only six out of 40 marine phyla exhibit direct development (i.e., nematodes, chaetognaths, gastrotrichs, kinorhynchs, gnathostomulids and tardigrades) (Pechenik, 1999). From the early days of deep-sea exploration, it was assumed that the “extreme” environmental conditions would select for unique larval development and adaptation. However, with few exceptions, the development strategies found in shallow-water systems are also present in the deep sea (Young, 2003). Brooding was once predicted to be the predominant mode of development in the deep sea, particularly in the extensive and nutrient-poor abyssal plains, nevertheless planktonic embryonic/larval development is equally or even more prevalent. Taxa such as peracarid crustaceans, nematodes and many polychaetes, that make up a large proportion of benthic CCZ diversity (Rabone et al., 2023) are phylogenetically constrained to brood embryos and therefore connectivity depends on adult mobility (Brix et al., 2020). An association between species life-habits and dispersal strategies has been recently hypothesized to explain the more regionally restricted dispersal patterns of nodule-growing species compared to sediment dwelling or swimming fauna (Simon-Lledó et al., 2025). Regardless of potential strategies that may enhance dispersal distance (e.g., increased parental investment, arrested development or long planktonic duration), successful planktonic dispersal and, hence, connectivity, may be reduced in mined areas, as earlier life stages are the most vulnerable to disturbances like the release of contaminants into the water column (Mestre et al., 2019). In addition to active adult migration and embryonic/larval dispersal, episodic disturbances of the seabed or deep-reaching eddies (Aleynik et al., 2017) could resuspend sediment and its infaunal organisms into the water column, and their dispersal from this point would thus be modulated by water currents in the same way as for planktonic life-stages. Other forms of transport include rafting and hitchhiking (Winston, 2012). It is even possible that the nodules themselves serve as stepping stones for dispersal if organisms travel from one nodule to the next. Moreover, it has been shown that population structure patterns can be species-specific, rendering generalizations misleading (Miller and Gunasekera, 2017).
In light of the many anthropogenic impacts that marine ecosystems are being faced with, and the need to preserve threatened biodiversity, connectivity has become a parameter of primary importance in determining resilience, as it helps to define the ability of an ecosystem to recover post-impact (Gollner et al., 2017), and in the design of area-based management tools, including MPAs (CBD, 2009; Lagabrielle et al., 2014). In order to be sustained in the long-term, threatened populations in one locality need to be replenished by a source population to which they are connected to via dispersal or migration. Thus, risk of (local) extinction and the resulting loss of biodiversity can only be realistically assessed when connectivity is understood while each taxon group presents their own challenges when trying to infer connectivity (e.g., difficulties with DNA amplification, complex taxonomy, low abundances, inability to sort bulk sediment samples into individual specimens, etc).
In this review we provide the current state of knowledge of connectivity and address the major impediments to examining it for the major benthic faunal size classes in the CCZ. We examined published literature focused on megafauna, macrofauna, meiofauna, Foraminifera, and microbes with the aim of revealing shared or contrasting beta diversity patterns. Finally, we present the characteristics of an idealized connectivity study to guide future research.
The largest animals of the benthos, i.e., megafauna (>1 cm), are also known to be the least abundant, particularly at abyssal depths (Rex et al., 2006). This low abundance, combined with high diversity, has largely constrained the study of connectivity in CCZ megafaunal populations. This is due to the difficulty of sampling specimens of the same species across locations, as well as the limited baseline knowledge to which newly collected specimens can be compared. Studies based on specimens collected by Remotely Operated Vehicles (ROVs) from multiple phyla in the eastern CCZ (Exploration area UK-1), have shown that typically less than 30% of specimens matched published morphological or genetic descriptions, the remainder being new to science (Dahlgren et al., 2016; Amon et al., 2017a, b; Rabone et al., 2023). Using both morphological and molecular evidence, 55 specimens in the western CCZ (APEI 1, 4, 7) were classified into 48 morphotypes belonging to five phyla, from which only nine were known species, and 39 potentially new to science (Bribiesca-Contreras et al., 2022). With the exception of two ophiuroid taxa (Laming et al., 2021), we still know very little about reproduction strategies or larval typology of CCZ megafauna, as collecting and linking larvae to described adult specimens is extremely challenging at abyssal depths (Kersten et al., 2019). There is a pressing need for detailed taxonomic studies incorporating genetic data (e.g., Bribiesca-Contreras et al., 2022; Christodoulou et al., 2020; Kersken et al., 2019) to expand reference sequence databases and to build robust species inventories to support connectivity research of CCZ megafauna.
To aid molecular connectivity analyses, image-based morphological characterization of megafauna has been applied as a proxy to study community distribution patterns at increasingly larger spatial scales across the CCZ. This approach counters the lower taxonomic resolution of image-based identification, partially addressed using protocols to deal with uncertainty (Horton et al., 2021, 2024), with large numbers of spatially widespread morphospecies occurrences obtained from seabed surveys (Amon et al., 2016; Vanreusel et al., 2016; Simon-Lledó et al., 2019, 2020). From these we know that at short distances (<30 km) communities largely differed between seamounts and adjacent abyssal plains (Cuvelier et al., 2020; Durden et al., 2021); the majority of morphotypes (60-70%) found on CCZ seamounts were typically unique, suggesting limited connectivity between the two. At local scales, distances <10-20 km, community assembly patterns appear to be strongly regulated by niche partitioning mechanisms associated with seabed topography (Simon-Lledó et al., 2019), nodule abundance (Amon et al., 2016; Simon-Lledó et al., 2019) or even the presence of small rock-outcrops (Mejía-Saenz, et al., 2023); features that largely vary within the limits of contract areas and APEIs (see Jones et al., 2021). Even though community structure can differ at distances <300 km (Simon-Lledó et al., 2020; Durden et al., 2021; Jones et al., 2021), ~40% of megafauna morphotypes were typically shared across different abyssal plain locations on a regional scale (<1000 km).
At ocean basin scale (<5000 km), methodological standardization and taxonomical alignment of >50000 megafaunal specimen occurrences from seabed imagery across 14 sites identified a total of 411 distinct megafaunal morphospecies (APSMA catalogue v1, https://zenodo.org/records/8172728, https://zenodo.org/records/8172728). From these, only five were found across all 14 sites; four sponge and one anemone species, i.e., the carnivorous demosponge Axoniderma mexicana sp inc., the stalked hexactinellid Hyalonema tylostylum sp. inc, the discoidal-flat hexactinellid Docosaccus maculatus sp. inc., the nodule encrusting demosponge Thenea sp. indet, and the anemone Hormathiidae gen. indet. Eight morphospecies were shared between 13, 12, 11, and 10 locations while 52 were found only in two locations and 124 were singletons. Most remarkably, phylum-level taxonomic replacements revealed the existence of a lower and upper abyssal biogeographic province in the CCZ, likely separated by the large-scale niche-filtering effect of the calcite carbon compensation depth above and below 4500 m, respectively (Simon-Lledó et al., 2023). Additional macroecological analyses of the APSMA dataset suggest an association between species life-habits and regional dispersal patterns in CCZ communities (Simon-Lledó et al., 2025). Sessile taxa that only grow on nodules exhibited significantly higher species turnover than sediment-dwelling or swimming megafauna, suggesting a more restricted dispersal ability in nodule epifauna (Simon-Lledó et al., 2025). However, while these provide an empirical basis to direct future exploration or formulate new hypotheses, outcomes of image-based studies must be coupled with more extensive specimen collection and subsequent molecular analyses (e.g., barcoding) to address the real genetic connectivity among CCZ megafaunal populations, and thereby the congruence between both approaches.
Deep-sea macrofauna is defined as organisms retained on a 300 µm mesh sieve. The macrofauna sensu stricto excludes dominant meiofaunal taxa (e.g., nematodes, copepods), while the macrofauna sensu lato includes all metazoans (Hessler and Jumars, 1974; Lins et al., 2021). Another distinction is made between the macro-infauna, living in the sediments, and the epibenthic fauna or suprabenthos living within the benthic boundary layer. Macro-infauna and suprabenthos of the CCZ are typically sampled with a box corer and epibenthic sledge, respectively. The macrofauna sensu stricto is dominated by polychaetes, tanaids and isopods, although their relative contributions and species composition vary according to sampling gears and habitats (Kaiser et al., 2023).
Polychaetes are the most abundant and diverse taxon in macro-infaunal assemblages of the CCZ (e.g., Kaiser et al., 2024; Washburn et al., 2021b). Suprabenthic species are less prominent, and mostly belong to the family Polynoidae (Bonifácio et al., 2021). Polychaete connectivity in CCZ has been addressed at community level through beta diversity patterns, and at species level through spatial genetic structure. In a quantitative study of the macro-infauna including four contract areas in the eastern CCZ (BGR, GSR, IOM, IFREMER) and APEI3 (Figure 1), Bonifácio et al. (2020) found a significant decay of community similarity by distance across contract areas but only when omitting APEI3 from the analysis. As contract areas and the APEI3 are separated by the Clarion fracture, it may act as a dispersal barrier for polychaetes. Out of 275 macro-infaunal polychaete morphospecies, only one was shared among all five sites, two were shared between APEI3 and at least one contract area, but 104 were shared by at least two contract areas (Bonifácio et al., 2020; Washburn et al., 2021b). A different pattern arose from suprabenthic polychaetes, particularly polynoids (Bonifácio et al., 2021; Bonifácio and Menot, 2018). Contrary to macro-infaunal polychaetes, polynoids were more abundant and diverse in APEI3 than BGR, GSR and IFREMER. From a total of 95 morphospecies, 11 were shared between the APEI and at least one contract area, the same number was shared among contract areas but not with the APEI. Across the contract areas, the rate of morphospecies turnover was estimated between 0.04 to 0.07 morphospecies/km, with corresponding mean geographic range of 14-25 km (Bonifácio et al., 2020), which would fall at the upper limit (5-14 km) of the expected length of potential mining fields (Volkmann and Lehnen, 2018). Species turnover was largely driven by singletons, which represented about half of the specimens (Bonifácio et al., 2020; Stewart et al., 2023). The study of spatial genetic structure is so far limited to eight widespread and abundant species, including five infaunal species, one nodule-dwelling species and two suprabenthic species (Bonifácio et al., 2021; Stewart et al., 2023). Even for these abundant species, the sample size remained small due to low faunal densities in the CCZ, with number of specimens ranging from 6-146 where the species were most abundant, and 1-3 where the species were least abundant. As species delimitation was the primary purpose of genetic analyses, population connectivity was inferred from haplotype networks of the mitochondrial barcoding genes Cytochrome Oxidase I (COI) and 16S (Bonifácio et al., 2021; Stewart et al., 2023). All eight species showed high haplotype diversity but no phylogeographic structure, suggesting high connectivity across the CCZ and even beyond. The reproductive mode was inferred from egg size for three species, two of which were likely brooders, and one was likely a broadcast spawner (Stewart et al., 2023).
Few studies have focused on molecular connectivity of isopods in the CCZ. Whereas first attempts provided a general assessment of isopod diversity using the COI fragment (Janssen et al., 2015), a follow-up study investigated select species to specifically address connectivity (Janssen et al., 2019). However, no isopod species were found beyond the margins of a single contract area. Whereas later studies were limited to the BGR, GSR and IFREMER contract areas, a more recent species delimitation approach was carried out including the aforementioned contract areas but also the IOM, the DISCOL experimental area (Peru Basin) and APEI3 using the 16S fragment in addition to COI (Brix et al., 2020). The authors showed that connectivity between areas largely depended life history. Whereas less mobile species tended to show a rather limited distribution range, more mobile species from the families Munnopsidae and Demosomatidae were found in samples up to 5000 km apart (Brix et al., 2020). They also showed that isopod assemblages in contract areas within the CCZ were more similar to one another than they were to APEI3 or the more distant DISCOL area. Still, the number of shared species between areas was limited; of the 170 Molecular Operational Taxonomic Unit (MOTUs) just three were shared between four areas, seven between three areas and 11 between two areas. The areas with the highest number of shared MOTUs were the BGR and GSR area with 13 and 14 shared MOTUs, respectively (Brix et al., 2020). Only two species were found in all five areas. Notably, one of these, which was morphologically identified as Macrostylis metallicola (Riehl and Smet, 2020), belonged to the burrowing family Macrostylidae. In general this family showed the narrowest distribution, rarely exceeding the boundaries of a single contractor area. Considering only morphology, M. metallicola was found in five different areas. However, this species showed high genetic variability resulting in the delimitation into three different MOTUs while exhibiting minimal morphological variation. This suggests M. metallicola constitutes a cryptic species complex. Another species from the partly-swimming family Nannoniscidae distributed across five contract areas was reported and described as Nannoniscus hilario by Kaiser et al. (2021). Bober (2018) showed genetic connectivity of the swimming Munnopsidae between the CCZ and Atlantic waters for Acanthocope galatheae (Wolff, 1962), which occurs across the Atlantic (Malyutina et al., 2018). Studies on CCZ isopods (reviewed by Kaiser et al., 2023) so far have shown that the majority of species exhibited limited distribution ranges in the CCZ with most being found in a few or a single contract area. Nevertheless, wider distributions of several thousand kilometers have also been recorded, often correlating with isopod lifestyle.
Ophiuroids are one of the most abundant macro-and megafaunal groups found in the CCZ (Simon-Lledó et al., 2023). The most comprehensive barcoding study using COI to assess ophiuroid diversity was carried out by Christodoulou et al. (2020), analyzing 543 specimens from five contract areas (UKSRL, BGR, IFREMER, GSR, IOM), APEI3 as well as the DISCOL area in the Peru basin. This assessment resulted in 43 species of which 19 were restricted to a single sampling area. Some of these were shown to belong to ancient deep-sea lineages (Christodoulou et al., 2019). The different contract areas shared on average six species ranging from three (IOM and IFREMER) to 14 (BGR and UKSRL) while even the most distant sites (e.g., IFREMER and BGR, IFREMER and UKSRL) shared at least four species. Faunistic assemblages of the contractor areas were surprisingly similar, especially when compared to APEI3 which shared only up to two species with the contractor areas and none with the DISCOL area. The most common species of the study were Ophioleucidae sp., Silax daleus (Lyman, 1879) (reported as Amphioplus daleus in Christodoulou et al., 2020) and Ophiosphalma glabrum (Lütken & Mortensen, 1899), which were present in all areas except APEI3. Ophiosphalma glabrum likely possess lecithotrophic larvae which would allow for long-range larval dispersal independent of resource availability (Laming et al., 2021). One of the species has been described as Ophiotholia saskia by Eichsteller et al. (2023) and has since then been collected in the IOM, BGR, UKSRL and NORI contract areas. Ophiotholia supplicans (Lyman, 1880) was analyzed and collected from UKSRL, NORI, BGR and OMS contract areas and was also reported from APEI3 and DISCOL. Molecular species delimitation, however, indicated high molecular diversity in O. supplicans, resulting in the division of several closely related MOTUs. Thus, it is unclear whether this is one widely distributed species with high genetic variability or several different, locally restricted species.
Copepods are small crustaceans which make up a significant part of the meiofauna with most of them belonging to the group Harpacticoida. In the CCZ they represent around four to ten percent of the metazoan meiofauna in terms of abundance when including nauplii (Hauquier et al., 2019; Pape et al., 2021; Tong et al., 2022). Despite their high abundance, the diversity and connectivity of benthic copepods in the CCZ is not well understood. Morphological data from Pape et al. (2021) compared copepod species composition and diversity between three stations in the GSR contract area, one nodule-free and two nodule-rich, finding that no copepod species occurred in all three sites, 89% was confined to one site, 63% of all species were singletons, and 12% were doubletons. Very little genetic data were available until a recent study by Rossel et al. (2022) published 1296 COI barcodes. However, this study focused only on the BGR contract area. Even within this dataset, MOTUs were represented by only a few specimens. Irrespective of the applied delimitation method, diversity was high with species numbers ranging from 718 to 794. Notably, over a third of the specimens belonged to singleton MOTUs. Only nine MOTUs were represented by ten or more specimens. Comparing the aforementioned DNA sequences to genetic material from the CCZ that have been published in earlier phylogenetic studies (Khodami et al., 2017, 2019) and species descriptions (Mercado-Salas et al., 2019) revealed only one potential species of hyperbenthic cyclopoids, Euryte sp., with a distribution beyond the BGR contract area. It was found in both the BGR and IFREMER contract areas, hundreds of km apart (Khodami et al., 2019). In another study on meroplankton that included genetic analyses from the OMS and UKSRL contract areas using COI, 18S Small Sub-Unit (SSU) V1-V2 and V7-V8 markers, six MOTUs with distributions extending beyond these areas were found (Kersten et al., 2019). Of these, one occurred in the OMS, UKSRL, and BGR areas; another in the OMS and BGR areas; one from the UKSRL area was also found in the BGR area; and another in the IFREMER area. Interestingly, one V1-V2 sequence from the OMS and UKSRL areas matched a sequence from the Kuril Kamchatka trench with 99.72% identity. A V7-V8 sequence from the UKSRL area had 100% identity with Cyclopina agilis from the western Atlantic coast. However, the accuracy of species-level identification using the V7-V8 marker for copepods is questionable (Wu et al., 2015). It may only be reliable for distinguishing genera or families, suggesting the need for further investigation using alternative genetic markers.
The species description of Siphonis ruehlemanni (Mercado-Salas et al., 2019), included records from the eastern BGR area, and the IFREMER area. The species Cerviniopsis longicaudata, originally described from deep waters off Norway, was also found in the CCZ and genetically analyzed by Khodami et al. (2017) from the BGR area. Another specimen with a matching COI barcode was found in the same region years later (Rossel et al., 2022). One new species described by Gheerardyn and George (2019) from the GSR contract area was also in the Porcupine Abyssal Plain in the eastern Atlantic Ocean. Other benthic copepod species described from the CCZ (Republic of Korea area) by Cho et al., 2016 have not been reported elsewhere. Because specimens of new deep-sea species are rare, DNA analyses are sometimes neglected in taxonomic descriptions in order to maintain an intact specimen which would otherwise be consumed during DNA extraction.
Few tentative conclusions can be made regarding the connectivity of benthic copepod species within the CCZ based on DNA barcoding or morphological data. Certain species were shared between distant contract areas, suggesting that the distribution of these microscopic animals across large expanses of abyssal plains is possible, indicative of long range connectivity. Nevertheless, it is currently impossible to conclude on any abiotic factors or life history traits influencing the degree of connectivity. In general, looking at smaller spatial scales, species are rare yet intensified and extended sampling may reveal connectivity to be more extensive.
Nematodes are the most abundant metazoans in the CCZ, constituting over 80% of the infaunal assemblages (Hauquier et al., 2019; Pape et al., 2021; Pape et al., 2017). While a number of morphological studies of meiofauna have been conducted in the area (Tong et al., 2022; Min et al., 2018; Renaud-Mornant and Gourault, 1990; Uhlenkott et al., 2020) these typically do not provide taxonomic information beyond phylum-level for nematodes. When nematodes are the specific group of study, the resolution is commonly restricted to genus-level (Radziejewska et al., 2001) due to paucity of diagnostic characters and phenotypic plasticity (De Ley et al., 2005; Derycke et al., 2010), with just five studies providing (morpho)species-level identifications (Lambshead and Boucher, 2003; Hauquier et al., 2019; Pape at al., 2017; Pape et al., 2021; Miljutin et al., 2011; Miljutina et al., 2010). Due to the time-consuming process of mounting specimens on microscope slides, typically around 100 nematodes from each sample are chosen at random for genus and species identification. Lambshead and Boucher, (2003) sorted 3321 specimens into 200 morphospecies. From these, 63% (12/19) of the dominant species (>1% relative abundance) were found across their five sampling stations, spanning a latitudinal gradient from the equator up to 23° North, suggesting that the Clarion and Clipperton fractures do not pose an impediment for connectivity. Miljutina et al. (2010) identified 2114 specimens into 325 morphospecies and reported significant differences between nodule-bearing and nodule-free sites of the IFREMER contract area. Even so, a substantial fraction of morphospecies (44%, 144/325) were shared between the two, meaning that connectivity exists between areas with and without nodules. Similarly, Pape et al. (2021) observed that 6 out of 11 species of the genus Halalaimus were shared between nodule-free and nodule-bearing sediments in the GSR contract area. Hauquier et al. (2019) also focused on species of the common deep-sea genus Halalaimus which typically represents between 3-11% of the nematode community. Out of 143 specimens, 24 species were identified of which five were found in the APEI3, IFREMER, GSR, IOM, and BGR contract areas. Although this would suggest that connectivity does exist across such large distances, these taxa were represented in low numbers (~ 6 specimens/species) raising the question whether these areas could function as a source of population replenishment following disturbance. Additionally, six of the 14 Halalaimus species identified from the GSR contract area had been reported before for the DISCOL area (Pape et al., 2017), indicative of potential connectivity. A consistent finding across all studies was that Acantholaimus, Desmoscolex, Halalaimus and Thalassomonhystera were the most widespread and species-rich genera. As in the Peru Basin (Thiel et al., 1993; Bussau et al., 1995), nematodes dominated the metazoan meiofauna inhabiting the crevices of polymetallic nodules of the GSR contract area (Pape et al., 2021). The same dominant taxa were found in both nodule crevices and sediments (i.e., Monhystrella/Thalassomonhystera), though genus composition differed significantly. About 25% of the genera were unique to either substrate, but none were endemic to nodule crevices. These differences in genus composition corresponded to differential feeding strategies as inferred from buccal morphology, with more tooth-bearing nematodes in the nodule crevices. The divergence between meiofauna communities increased with higher taxonomic resolution (higher taxon → nematode genus), hinting at even greater differences between substrates at the species level, but this remains to be investigated.
The vast majority of deep-sea nematode species are undescribed, leading to significant deficits of taxonomically informed reference sequences in public databases such as GenBank. Resultantly, DNA-based investigations of CCZ nematodes are few and do not resolve beyond phylum- (Laroche et al., 2020; Lejzerowicz et al., 2021) or genus-level (Macheriotou et al., 2020) and tend to have a large proportion of taxonomically unassigned Operational Taxonomic Units (OTUs) or Amplicon Sequence Variants (ASVs), (Callahan et al., 2017). Macheriotou et al. (2020) targeted the 18S rRNA locus (V1-V2) to generate ASVs; the majority were unique to each area and two (Acantholaimus_sp & Daptonema_sp) were shared between the BGR, IOM, GSR, IFREMER and APEI3 while 15% (300/1981) were shared by at least two areas.
Generally, the morphological data point to a degree of connectivity which exceeds that of DNA-based assessments, however one can only speculate whether this would increase or decrease if all specimens in a sample were identified rather than a subset. Moreover, cryptic speciation known for (deep-sea) nematodes (e.g., Smith et al., 2008) may be resulting in an overestimation of shared species between areas while intraspecific variability in morphological characters would result in the reverse (Miljutin and Miljutina, 2016). Cosmopolitanism has been suggested for some nematode species (Bik et al., 2010; Miljutin et al., 2010; Zeppilli et al., 2011); thus high connectivity within the CCZ alone may not be implausible. Similarly, the metabarcoding analyses showed that identical genetic material, most likely belonging to the same species, was found at distances hundreds of km apart, hinting at long range connectivity.
More than 50% of meiofaunal biomass and abundance in abyssal plains is represented by protists (Gooday et al., 2021) with benthic foraminifera being a major contributor. Extensive research over the last decades in the central North Pacific has revealed the striking diversity of this group (Gooday et al., 1992, 2017, 2021). In the different CCZ areas (Figure 1; UK1, OMS, BGR, GSR, IOM, IFREMER, YUZH, APEI1, 3, 4, 6 and 7) on average 75% of foraminiferal species are represented by monothalamiids, characterized by slow growth and metabolic rates. These play an important role in carbon cycling as they feed on refractory organic matter, making it available for higher trophic levels. The ability to feed on sparsely distributed food sources such as bacteria and degraded organic matter is considered to be one of the reasons why these soft-shelled forms are so successful in oligotrophic environments (Gooday et al., 2001). However, rarity, combined with under-sampling, precludes any statements regarding endemicity of monothalamiids in the CCZ (Goineau and Gooday, 2019). Xenophyophores are also an abundant component of CCZ megafauna (Gooday et al., 2017; Simon-Lledó et al., 2019) with many species being represented by 1-2 specimens and are known from a single site, rendering any inferences of connectivity impossible (Gooday et al., 2020). Nonetheless, shared occurrences across 3800 km (APEI4 to UK-1/OMS) have been confirmed using barcoding for Aschemonella monile and Moanammina semicircularis (Gooday et al., 2020). Sessile Foraminifera, especially encrusting forms, are very abundant on polymetallic nodules (Veillette et al., 2007; Gooday et al., 2015) with up to 75 morphospecies reported to occur on just seven nodules (Gooday et al., 2015). Whether these mat-like Foraminifera occur in different CCZ areas with common genetic patterns has not yet been explored, due their complex taxonomy. Most benthic Foraminifera are widely distributed at regional scales (i.e., UK1, OMS, BGR, GSR, IOM, IFREMER, Yuzhmorgeologiya, APEI1, 3, 4, 6 and 7) but not necessarily at a global scale. Both morphological and DNA-based approaches highlight rarity with singletons often representing up to 30% of the community (Goineau and Gooday, 2017). Their occurrence seems to be positively correlated to nodule abundance and size. Foraminiferal dispersal is driven by propagules which are easily transported by bottom currents after death. Consequently, benthic foraminiferal taxa could hypothetically be transported globally, resulting in cosmopolitan species (Gooday et al., 2021). Distribution similarities at regional scale is often observed despite a large number of singletons. Even though particular taxa have wide ranges, there seems to be a gradual shift in the community composition in relation to environmental gradients, such as productivity and nodule abundance (Gooday et al., 2021). The carbonate compensation depth (CCD) also plays a major role in defining foraminiferal distribution, restricting calcareous species. The CCD is situated at 4500 m in the southern and north Pacific, deepening to ~5000 m near the equator, yet shifting upward in the water column due to global warming (Sulpis et al., 2018), resulting in high dominance of non-calcareous Foraminifera in the CCZ (Gooday et al., 2021). Finally, the ability of Foraminifera to reproduce asexually may allow them to persist at very low abundance without the need for propagules to be introduced from elsewhere. As such, and considering potential patchiness of communities, the observed singletons could reflect pseudo-endemism due to under-sampling (Gooday et al., 2021).
Microbial connectivity in the CCZ remains poorly understood, with most studies focusing on microbial communities in seawater, sediments, and polymetallic nodules at local to regional scales (Tully and Heidelberg, 2013; Wu et al., 2013; Shulse et al., 2016; Lindh et al., 2017; Hollingsworth et al., 2021). Larger-scale syntheses have analyzed datasets spanning 10 to 4000 km, revealing substrate-specific microbial compositions (Wear et al., 2021; Orcutt et al., 2024). Different physical properties of nodules and sediments foster distinct habitats; thus, connectivity between these substrates likely results from passive transfer rather than taxon establishment. Given these complexities, the microbial assemblages associated with sediments and nodules are reviewed separately.
Microbial diversity in CCZ sediments has been studied to establish baselines for mining impact assessments, however, microbial biogeography and connectivity remains poorly understood. Most data come from local to regional scales (10-1500 km) in the eastern CCZ (Shulse et al., 2016; Lindh et al., 2017; Hollingsworth et al., 2021). Their findings suggest high dispersal rates and low compositional turnover, consistent with minimal physical barriers and low sedimentation rates reducing microbial isolation (Washburn et al., 2021a). Community composition showed little variation with distance and was not significantly influenced by environmental selection, suggesting dispersal mitigates effects of mutation and drift (Hanson et al., 2012). Rare taxa drive community shifts at local scale, indicating spatial and temporal variability in ecological processes (dispersal, drift, selection, mutation) for taxa with different population sizes (Molari, unpublished data). Rare taxa may result from stochastic fluctuations or niche specificity, contributing to microbial adaptiveness (Jousset et al., 2017). Understanding their connectivity and function is key to assessing sediment microbe resilience in polymetallic nodule ecosystems.
Wear et al. (2021) examined benthic samples across 10-4000 km in CCZ, combining new (western CCZ) and existing data. Despite being the most comprehensive study, limitations remain due to differing sampling methods, PCR primers, sequencing techniques, and the absence of central CCZ data. Geographical distance had a weak effect on microbial beta diversity, with shifts mainly in relative taxon abundance rather than composition. The CCZ is characterized by a gradient in primary productivity, organic carbon flux, and sediment organic content decreasing southeastward (Pennington et al., 2006; Lutz et al., 2007; Volz et al., 2018). Microbial abundance shifts may reflect preferential growth under varying trophic regimes. Potential heterotrophs (Woeseiaceae, Dehalococcoidaceae, Acidimicrobiaceae) and nitrifiers (Nitrosomonadaceae, Nitrosopumilaceae, Nitrospinaceae) exhibited habitat preference across the CCZ trophic regimes (Hollingsworth et al., 2021; Wear et al., 2021). Additionally, local microbial community shifts have been observed in presence of different seafloor topographic features (ridges, troughs, deep plains) and nodule coverage (Hollingsworth et al., 2021; Molari, unpublished data), indicating that habitat heterogeneity, alongside trophic regimes at large spatial scale, shapes community structure at local scale.
Polymetallic nodules harbor distinct microbial communities composed of taxa enriched from surrounding seawater and sediments rather than unique species (Wu et al., 2013; Tully and Heidelberg, 2013; Blöthe et al., 2015; Shulse et al., 2017; Lindh et al., 2017; Molari et al., 2020; Wear et al., 2021; Orcutt et al., 2024). Some manganese-oxidizing and -reducing bacteria, potentially involved in nodule formation, have been proposed as core microbiota (Wu et al., 2013; Blöthe et al., 2015), but their presence is inconsistent across studies (Tully and Heidelberg, 2013; Shulse et al., 2017; Lindh et al., 2017; Molari et al., 2020; Wear et al., 2021; Orcutt et al., 2024). It should be noted that this discrepancy might be due to the lack of standards in the study of microbial community associated to the nodules (e.g., part of the nodule investigated, DNA isolation procedure, and sequencing depth). Nodules exhibit higher beta diversity than sediments (e.g., Molari et al., 2020; Wear et al., 2021), but community composition is influenced more by nodule microhabitats than geographic location or time (Tully and Heidelberg, 2013; Blöthe et al., 2015; Wear et al., 2021; Molari, unpublished data). The upper surface, exposed to bottom water and fauna (Vanreusel et al., 2016), differs from the lower side embedded in the seafloor, where interactions with sediment biogeochemistry and infauna occur. This habitat heterogeneity is likely an important driver for beta diversity. Additionally, the nodules internal structure, mineral composition, and complex pore system (up to 120 m2 g-1) provide diverse microhabitats (Blöthe et al., 2015). Microbial cell abundance decreases up to two orders of magnitude from exterior to interior (Shiraishi et al., 2016), further supporting nodule zonation. Thus, it is reasonable to hypothesize that the interplay of ecological processes controlling microbial connectivity may vary across different nodule regions and act at different spatial and temporal scales. For example, microbes on the upper surface of the nodule could follow the biogeographical patterns of sediment communities influenced by trophic regimes, potentially explaining shifts in nitrifying taxa (Nitrosococcaceae, Nitrosomonadaceae, Nitrospinaceae, Nitrospiraceae, Nitrosopumilaceae) (Molari et al., 2020; Wear et al., 2021; Molari, unpublished data). In contrast, variations in potential metal-cycling bacteria (Hyphomicrobiaceae, Magnetospiraceae, Methyloligellaceae, Kiloniellaceae) (Molari et al., 2020; Molari, unpublished data) may be linked to nodule mineralogy, suggesting biogeographical patterns are shaped by nodule formation processes.
As mentioned previously (Section 2), deep-sea research specifically aimed at assessing connectivity is a challenging endeavor requiring a dedicated investment of time and resources. Here we outline the information that would be required to complete the “idealized connectivity study” for fauna and microbes (Table 1).
Even though the deep sea has not escaped the breadth of human impacts (e.g., oil and gas exploration, litter, radioactive waste disposal, pollution), it remains a largely pristine ecosystem of which only a very small fraction has been thoroughly explored (Mengerink et al., 2014). For it to remain as such and to maintain its ecosystem functions, the deep sea needs to be protected; and in order to be protected, it needs to be understood. This understanding is crucial for marine spatial planning through the designation of areas that will be spared from deep seabed mining and the adoption of a layout of mining operations that reduces harmful effects. Thus, addressing the gaps of knowledge relating to marine connectivity in the CCZ is timely and of paramount importance.
The International Union for the Conservation of Nature (IUCN) has called for a moratorium on deep seabed mining unless and until certain conditions have been met such as conducting rigorous and transparent impact assessments, the projected impacts being understood, livelihoods of interested parties being considered, implementation of the precautionary principle and ensuring the effective protection of the marine environment (IUCN, https://iucn.org/, Resolution 122). While a large fraction of the research devoted to MPA design has been derived from fisheries sciences and thus focused on fish species or coral reefs (e.g., Krueck et al., 2017; Grorud-Colvert et al., 2021), the guidelines and recommendations originating from these works can be used to inform spatial management of the CCZ. The APEIs in this context are surrogates to MPAs.
Within this context, we urge scientists and stakeholders to address the aforementioned gaps of knowledge and most importantly, to distribute the insights gained.
• Marine connectivity must be included in spatial management of the CCZ.
Connectivity within and between individual APEIs as well as between APEIs and mined sites should be verified. The size and spatial arrangement of the mined sites should be such that they do not enclose (larval) source areas and do not intercept dispersal routes in order to allow post-mining recolonization of fauna. Likewise, the planning of mining operations should be guided by the timing of spawning or migration events of taxa if known.
• An appropriate sampling design must be applied.
To comprehensively assess connectivity, it is necessary to overcome the limitations that have marred previous studies: (i) restricted geographical range and limited number of samples from each site; (ii) lack of characterization of the relationship between biodiversity and habitat heterogeneity on continuous spatial scales; (iii) absence of time series covering seasonal and interannual variability. The distribution of samples must be widespread enough to incorporate habitat heterogeneity and the number of samples must be informed by statistical analysis to determine the number of replicates required to achieve the desired level of statistical power. Environmental parameters should be derived from the same samples as the biological data. Finally, the same sites should be re-visited across seasons and years to capture temporal variability. These requirements must be included in the ISA mining code to ensure license holders compliance.
• Detailed habitat characterization is needed.
A comprehensive understanding of the CCZ environment in combination with species occurrences is needed to predict species distribution based on habitat suitability models. This requires high-resolution bathymetric maps, including seafloor topography and nodule density to resolve local spatial heterogeneity, as well as particulate organic carbon (POC) flux to characterize the trophic regime. Bottom water-current data need to be mapped to identify where biota and sediment plumes from mining activities will be transported to. Time-series observations of currents and fluxes will be required to capture temporal variability.
• Efficient, state-of-the art sampling methods must be used.
The boxcorer and multiple corer are the most widely used tools to sample macrofauna and meiofauna respectively, and the only ones recommended by the ISA (ISBA/25/LTC/6/Rev.1). ROVs allow for targeted and opportunistic sampling of mainly megafauna, while Epi-Benthic Sleds (EBS) are needed in order to capture macrofauna in large numbers as well as various life-stages, especially those which are under-sampled. Moreover, the EBS can sample benthic boundary layer communities impacted by the sediment plumes, which are not sampled by corers. Where possible, minimally invasive methods shall be employed (e.g., by combining imaging methods with sample-based validation). A disadvantage of imaging methods is that the small faunal elements caught by EBS are not visible in the image data. Thus, even though it leaves a long-lasting impact on the seafloor, the EBS is the only gear that provides a deeper insight into the “sediment secrets”, the macrofauna not visible by naked eye.
• Genetic tools should be comprehensively applied.
Both nuclear (e.g., 18s rRNA) and mitochondrial genes (e.g., COI) should be applied when investigating population structure in order to be most informative. Although the methodologies described herein do not represent an exhaustive list of what can be used to investigate connectivity, we can provide suggestions as to which strategies would serve this objective, such as barcoding, metabarcoding and Restriction site associated DNA markers (RADseq). In order for sequence data to be interoperable in time and between different institutions requires that the laboratory and bioinformatic workflows are reported in sufficient detail to be reproducible. Given the rapid development of novel methods, archiving following FAIR principles (Wilkinson et al., 2016) is needed for samples to be suited for future re-analysis (Mayer et al., 2021).
• Ensure the accuracy of DNA-based methods.
It is of the utmost importance to validate and standardize (environmental) DNA extraction methods in order to ensure the comparability and reliability of DNA-based diversity data. The presence of dormant or deceased organisms represents a challenge for DNA-based approaches, which are unable to discriminate between the presence of an individual and successful establishment or between one that is living or dead. Consequently, the application of methods (e.g., based on RNA) for determining the active versus dormant fractions is crucial to better resolve the mechanisms controlling connectivity in CCZ, including the ecological role of rare taxa.
• Distribution of knowledge and data sharing.
Data (e.g., species inventories, genetic sequences, image catalogues), metadata, and protocols must be shared amongst researchers, contractors and the public domain following FAIR principles in order to facilitate comprehensive investigations and robust, reproducible inferences (e.g., consistent morphospecies designations). A good example is the CCZ atlas for megafauna identification (Simon-Lledó et al., 2023) and the Clarion-Clipperton Zone Species Checklist embedded in the World Register of Marine Species (https://www.marinespecies.org/deepsea/CCZ/).
Despite their large size, megafaunal taxa in the CCZ remain among the most challenging groups to investigate due to low abundances as well as constraints associated with deep water sampling. Unlike smaller fauna, which can be collected in large numbers during a single expedition, ROV or EBS-based surveys typically retrieve fewer than ~50 megafaunal specimens per cruise, making it difficult to conduct robust genetic analyses or build comprehensive reference databases. As a result, our current understanding of connectivity at large spatial scales relies primarily on image-based numerical ecology. Such investigations have revealed the presence of a strong biogeographic boundary across the CCZ likely driven by the carbonate compensation depth and that life history is linked to dispersal potential. To rigorously assess population structure and dispersal dynamics, it is critical to expand molecular analyses on specimens, integrating barcoding and genomic tools with image-based approaches. This will help determine the extent to which observed distributional patterns correspond to real genetic connectivity and advance our understanding of the evolutionary and ecological processes shaping megafaunal populations across the CCZ.
Macrofaunal taxa exhibited a wide range of connectivity patterns, which may be partly driven by dispersal potential as brooding isopods tend to have the narrowest distribution range with 86% of species unique to a contract area, while the most widespread species tend to be those with the highest adult motility. Polychaetes and ophiuroids whose varied reproductive traits and larval forms are generally unknown or at best inferred for CCZ taxa, reported 65% and 56% of species unique to a contract area, respectively. Many of the species that were unique to a contract area occurred as singletons, suggesting either under-sampling of small local populations or the importance of source-sink dynamics in the abyss. Few species were abundant and widespread enough to investigate genetic connectivity; our results nonetheless suggest the absence of genetic structure across the CCZ. However, mitochondrial genes do not have the resolution needed to assess contemporary genetic connectivity, and highly polymorphic markers such as microsatellites, SNPs or RAD sequencing would be better suited.
The available data for meiofaunal taxa (primarily Nematoda and Copepoda) in the CCZ thus far suggest that connectivity does exist between geographically distant regions with an apparent lack of “endemic” taxa. Whether this would remain true given exhaustive sampling remains an open question. Concurrently, there is evidence that contract areas in the eastern CCZ have higher connectivity between them than with nearby APEIs which may be harboring distinct assemblages. To comprehensively study connectivity in nematodes will require the time-consuming effort of firstly sorting specimens into species using morphology prior to any further molecular investigation; unfortunately, this action cannot be by-passed. From this point onward, the application of state-of-the-art methods such as SNPs and RAD-sequencing would be possible to obtain detailed population structure. Moreover, such an approach will enable the discovery of species-specific population structures. Comparative studies between metabarcoding and morphological identifications in copepods suggest that the use of COI can match morphological species occurrences; thus, connectivity can in fact be studied using metabarcoding. However, only a short fragment of the usual barcoding region was used, meaning that this would need to be expanded to allow the assessment of ongoing geneflow. The highest resolution would be provided by the aforementioned SNP or RAD-sequencing approaches.
Studies of microbial beta-diversity in the CCZ suggest high dispersal capacity and low compositional turnover in sediment communities, likely due to minimal physical barriers and low sedimentation rates. Geographical distance exerts a weak effect, with community shifts primarily driven by trophic regimes at large spatial scales (>1000 km) and by seafloor heterogeneity, resource partitioning, and nodule coverage at finer spatial scales (<30 km). Rare taxa contribute to community shifts at local scales, emphasizing the need to understand their role in microbial resilience within polymetallic nodule ecosystems. Nodules host distinct microbial communities enriched from surrounding sediments rather than unique species, with higher beta diversity than sediments due to microhabitat heterogeneity. Ecological processes controlling microbial connectivity likely interact and operate across multiple spatial and temporal scales within different parts of the nodule. Microbial diversity assessments predominantly rely on genetic markers such as the 16S rRNA gene, with taxa defined either as unique sequences or clustered units based on arbitrary similarity thresholds. While higher taxonomic resolution enhances connectivity assessments, lower-resolution clustering facilitates ecological interpretation but risks biasing evolutionary and ecological distances. The harmonization and standardization of sampling and molecular methods are essential to improve cross-study comparisons, mitigate methodological biases, and enhance the robustness of microbial biogeography assessments.
Overall, the benthic communities of the CCZ are characterized by high diversity, low abundance with predominantly undescribed species. By default, connectivity studies focus on abundant and widespread taxa, leaving knowledge gaps regarding the rare and localized fauna. Across the taxa examined (except for Foraminifera), a core set of species exhibits a wide spatial distribution, whereas the majority appear to have restricted geographic ranges, often dominated by singletons and doubletons. This pattern is not unique to the CCZ but is commonly observed in the deep sea, however, the extent to which it is an artefact of under-sampling remains unknown. Spatial variability in community composition across benthic size classes is at least partially driven by environmental gradients, including nodule abundance, seafloor topography, and food availability while the carbonate compensation depth plays a major role for taxa with a calcareous skeleton. Taxa inhabiting both nodules and sediments, such as microbes and nematodes, exhibit clear segregation in community composition between the two substrates, likely driven by the differing physical properties and ecological conditions. Life-history traits, such as mobility and reproductive strategy, which are more readily assessed for mega- and macrobenthic taxa, play a crucial role in determining connectivity. Even though many studies rely on physical samples or seafloor imagery for morphological identifications, genetic data are necessitated by the presence of cryptic speciation in deep-sea taxa. Concurrently, the main impediment to using metabarcoding in connectivity research is lack of taxonomically informed DNA reference sequences. Finally, this review is of course not exhaustive; firstly it mainly includes data from the eastern CCZ while a comprehensive understanding of connectivity in this extensive region requires data from the central and western areas. Secondly, not all benthic taxa are included herein (e.g., Porifera, Tanaidacea, Anthozoa, Crinoidea, Holothuria and others); nonetheless, we hereby provide the current state-of-the-art for at least a subset of the CCZ benthic fauna.
LMa: Conceptualization, Investigation, Writing – original draft, Writing – review & editing, Methodology. SR: Conceptualization, Visualization, Writing – original draft, Writing – review & editing. MM: Conceptualization, Methodology, Writing – original draft, Writing – review & editing. PM: Funding acquisition, Writing – review & editing, Project administration, Resources, Conceptualization. SB: Visualization, Writing – original draft, Writing – review & editing. MC: Visualization, Writing – original draft, Writing – review & editing. PD: Writing – original draft, Writing – review & editing. AH: Conceptualization, Writing – original draft, Writing – review & editing. FJ: Funding acquisition, Project administration, Writing – review & editing. ES: Conceptualization, Visualization, Writing – original draft, Writing – review & editing. LMe: Funding acquisition, Project administration, Writing – review & editing. EP: Writing – original draft, Writing – review & editing. GR: Writing – original draft, Writing – review & editing. AV: Conceptualization, Funding acquisition, Project administration, Resources, Writing – original draft, Writing – review & editing.
The author(s) declare that financial support was received for the research and/or publication of this article. This research is part of the DEEP REST project that was funded through the 2020-2021 Biodiversa and Water JPI joint call for research projects, under the BiodivRestore ERA-NET Cofund (GA N°101003777), with the EU and the following funding organizations: Agence Nationale de la Recherche (ANR-21-BIRE-0003), France, Ministry of Agriculture, Nature and Food Quality (LNV), Netherlands, Research Foundation – Flanders (FWO), Belgium, German Federal Ministry of Research (BMBF) through VDI/VDE-IT, Germany, Environmental Protection Agency (EPA), Ireland, Fundação para a Ciência e a Tecnologia (FCT), Portugal, Fundo Regional para a Ciência e Tecnologia (FRCT), Portugal-Azores and State Research Agency (AEI), Spain. Lenaick Menot and Pierre-Antoine Dessandier were supported by the French National Research Agency under France 2030 (reference ANR-22-MAFM-0001) Ana Hilario was further supported by funds from FCT/MCTES granted to CESAM (UID Centro de Estudos do Ambiente e Mar + LA/P/0094/2020).
The authors declare that the research was conducted in the absence of any commercial or financial relationships that could be construed as a potential conflict of interest.
The author(s) declared that they were an editorial board member of Frontiers, at the time of submission. This had no impact on the peer review process and the final decision.
The author(s) declare that no Generative AI was used in the creation of this manuscript.
All claims expressed in this article are solely those of the authors and do not necessarily represent those of their affiliated organizations, or those of the publisher, the editors and the reviewers. Any product that may be evaluated in this article, or claim that may be made by its manufacturer, is not guaranteed or endorsed by the publisher.
Aleynik D., Inall M. E., Dale A., Vink A. (2017). Impact of remotely generated eddies on plume dispersion at abyssal mining sites in the Pacific. Sci. Rep. 7 (1). doi: 10.1038/s41598-017-16912-2
Amon D., Hilario A., Arbizu P., Smith C. (2017a). Observations of organic falls from the abyssal Clarion-Clipperton Zone in the tropical eastern Pacific Ocean. Mar. Biodiversity 47, 311–321. doi: 10.1007/s12526-016-0572-4
Amon D., Ziegler A., Dahlgren T., Glover A., Goineau A., Gooday A., et al. (2016). Insights into the abundance and diversity of abyssal megafauna in a polymetallic-nodule region in the eastern Clarion-Clipperton Zone. Sci. Rep. 6, 30492. doi: 10.1038/srep30492
Amon D., Ziegler A., Drazen J., Grischenko A., Leitner A., Lindsay D., et al. (2017b). Megafauna of the UKSRL exploration contract area and eastern Clarion-Clipperton Zone in the Pacific Ocean: Annelida, Arthropoda, Bryozoa, Chordata, Ctenophora, Mollusca. Biodiversity Data J. 5. doi: 10.3897/BDJ.5.e14598
Anderson R., Sogin M., Baross J. (2015). Biogeography and ecology of the rare and abundant microbial lineages in deep-sea hydrothermal vents. FEMS Microbiol. Ecol. 91, 1–11. doi: 10.1093/femsec/fiu016
Baas Becking L. G. M. (1934). Geobiologie of inleiding tot de milieukunde (The Hague, the Netherlands: W.P. Van Stockum & Zoon (in Dutch).
Baco A., Etter R., Ribeiro P., Heyden S., Beerli P., Kinlan B. (2016). A synthesis of genetic connectivity in deep-sea fauna and implications for marine reserve design. Mol. Ecol. 25, 3276–3298. doi: 10.1111/mec.2016.25.issue-14
Balbar A., Metaxas A. (2019). The current application of ecological connectivity in the design of marine protected areas. Global Ecol. Conserv. 17, e00569. doi: 10.1016/j.gecco.2019.e00569
Berglund M., Nilsson Jacobi M., Jonsson P. (2012). Optimal selection of marine protected areas based on connectivity and habitat quality. Ecol. Model. 240, 105–112. doi: 10.1016/j.ecolmodel.2012.04.011
Bienhold C., Boetius A., Ramette A. (2012). The energy-diversity relationship of complex bacterial communities in Arctic deep-sea sediments. ISME J. 6, 724–732. doi: 10.1038/ismej.2011.140
Bienhold C., Zinger L., Boetius A., Ramette A. (2016). Diversity and biogeography of bathyal and abyssal seafloor bacteria. PloS One 11, e0148016. doi: 10.1371/journal.pone.0148016
Bik H., Thomas W., Lunt D., Lambshead P. (2010). Low endemism, continued deep-shallow interchanges, and evidence for cosmopolitan distributions in free-living marine nematodes (order Enoplida). BMC Evolutionary Biol. 10, 1–10. doi: 10.1186/1471-2148-10-389
Blöthe M., Wegorzewski A., Müller C., Simon F., Kuhn T., Schippers A. (2015). Manganese-cycling microbial communities inside deep-sea manganese nodules. Environ. Sci. Technol. 49, 7692–7700. doi: 10.1021/es504930v
Bober S. (2018). Abyssal Barriers-Phylogeography, distribution and natural history of Asellota (Crustacea) in the deep sea. (Doctoral dissertation). Staats-und Universitätsbibliothek Hamburg Carl von Ossietzky.
Boetius A., Haeckel M. (2018). Mind the seafloor. Science 359 (6371), 34–36. doi: 10.1126/science.aap7301
Bonifácio P., Martínez Arbizu P., Menot L. (2020). Alpha and beta diversity patterns of polychaete assemblages across the nodule province of the eastern Clarion-Clipperton Fracture Zone (equatorial Pacific). Biogeosciences 17, 865–886. doi: 10.5194/bg-17-865-2020
Bonifácio P., Menot L. (2018). New genera and species from the Equatorial Pacific provide phylogenetic insights into deep-sea Polynoidae (Annelida). Zoological J. Linn. Soc. 185, 555–635. doi: 10.1093/zoolinnean/zly063
Bonifácio P., Neal L., Menot L. (2021). Diversity of deep-sea scale-worms (Annelida, polynoidae) in the clarion-clipperton fracture zone. Front. Mar. Sci. 8. doi: 10.3389/fmars.2021.656899
Bribiesca-Contreras G., Dahlgren T., Amon D., Cairns S., Drennan R., Durden J., et al. (2022). Benthic megafauna of the western Clarion-Clipperton Zone, Pacific Ocean. ZooKeys 1113, 1. doi: 10.3897/zookeys.1113.82172
Brix S., Osborn K., Kaiser S., Truskey S., Schnurr S., Brenke N., et al. (2020). Adult life strategy affects distribution patterns in abyssal isopods–implications for conservation in Pacific nodule areas. Biogeosciences 17, 6163–6184. doi: 10.5194/bg-17-6163-2020
Bussau C., Schriever G., Thiel H. (1995). Evaluation of abyssal metazoan meiofauna from a manganese nodule area of the eastern South Pacific. Vie Milieu/Life Environ. 45, 39–48.
Callahan B., McMurdie P., Holmes S. (2017). Exact sequence variants should replace operational taxonomic units in marker-gene data analysis. ISME J. 11, 2639–2643. doi: 10.1038/ismej.2017.119
Cho D., Wi J., Suh H. L. (2016). Two new species of the deep-sea genus Parameiropsis (Copepoda: Harpacticoida) from the eastern central Pacific. Zootaxa 4132. doi: 10.11646/zootaxa.4132.4.4
Christodoulou M., O’hara T., Hugall A., Arbizu P. (2019). Dark ophiuroid biodiversity in a prospective abyssal mine field. Curr. Biol. 29, 3909–3912. doi: 10.1016/j.cub.2019.09.012
Christodoulou M., O’Hara T., Hugall A., Khodami S., Rodrigues C., Hilario A., et al. (2020). Unexpected high abyssal ophiuroid diversity in polymetallic nodule fields of the northeast Pacific Ocean and implications for conservation. Biogeosciences 17, 1845–1876. doi: 10.5194/bg-17-1845-2020
Convention on Biological Diversity (2009). Technical series no. 19 (Montreal, Canada: Secretariat of the Convention on Biological Diversity).
Cuvelier D., Ribeiro P., Ramalho S., Kersken D., Martinez Arbizu P., Colaço A. (2020). Are seamounts refuge areas for fauna from polymetallic nodule fields? Biogeosciences 17, 2657–2680. doi: 10.5194/bg-17-2657-2020
Dahlgren T., Wiklund H., Rabone M., Amon D., Ikebe C., Watling L., et al. (2016). Abyssal fauna of the UK-1 polymetallic nodule exploration area, Clarion-Clipperton Zone, central Pacific Ocean: Cnidaria. Biodiversity Data J. 4. doi: 10.3897/BDJ.4.e9277
Danovaro R., Fanelli E., Aguzzi J., Billett D., Carugati L., Corinaldesi C., et al. (2020). Ecological variables for developing a global deep-ocean monitoring and conservation strategy. Nat. Ecol. Evol. 4, 181–192. doi: 10.1038/s41559-019-1091-z
De Ley P., De Ley I. T., Morris K., Abebe E., Mundo-Ocampo M., Yoder M., et al. (2005). An integrated approach to fast and informative morphological vouchering of nematodes for applications in molecular barcoding. Philos. Trans. R. Soc. B: Biol. Sci. 360, 1945–1958. doi: 10.1098/rstb.2005.1726
Derycke S., Vanaverbeke J., Rigaux A., Backeljau T., Moens T. (2010). Exploring the use of cytochrome oxidase c subunit 1 (COI) for DNA barcoding of free-living marine nematodes. PloS One 5, e13716. doi: 10.1371/journal.pone.0013716
Deutsch C., Penn J. L., Lucey N. (2024). Climate, oxygen, and the future of marine biodiversity. Annu. Rev. Mar. Sci. 16 (1), 217–245. doi: 10.1146/annurev-marine-040323-095231
Ditlevsen P., Ditlevsen S. (2023). Warning of a forthcoming collapse of the Atlantic meridional overturning circulation. Nat. Commun. 14, 4254. doi: 10.1038/s41467-023-39810-w
Drazen J. C., Smith C. R., Gjerde K. M., Haddock S. H., Carter G. S., Choy C. A., et al. (2020). Midwater ecosystems must be considered when evaluating environmental risks of deep-sea mining. Proc. Natl. Acad. Sci. U.S.A. 117 (30), 17455–17460. doi: 10.1073/pnas.2011914117
Durden J., Putts M., Bingo S., Leitner A., Drazen J., Gooday A., et al. (2021). Megafaunal ecology of the western Clarion Clipperton Zone. Front. Mar. Sci. 8, 671062. doi: 10.3389/fmars.2021.671062
Dutkiewicz A., Judge A., Müller R. D. (2020). Environmental predictors of deep-sea polymetallic nodule occurrence in the global ocean. Geology 48 (3), 293–297. doi: 10.1130/G46836.1
Eichsteller A., Martynov A., O’Hara T., Christodoulou M., Korshunova T., Bribiesca-Contreras G., et al. (2023). Ophiotholia (Echinodermata: Ophiuroidea): A little-known deep-sea genus present in polymetallic nodule fields with the description of a new species. Front. Mar. Sci. 10, 1056282. doi: 10.3389/fmars.2023.1056282
Gheerardyn H., George K. (2019). Description of a new species of Neoargestes Drzycimski 1967 (Copepoda, Harpacticoida, Argestidae) from the Clarion Clipperton Fracture Zone (Pacific Ocean), with remarks on the systematics of the genus. Mar. Biodiv 49, 1891–1912. doi: 10.1007/s12526-019-00951-1
Goineau A., Gooday A. (2017). Novel benthic foraminifera are abundant and diverse in an area of the abyssal equatorial Pacific licensed for polymetallic nodule exploration. Sci. Rep. 7, 45288. doi: 10.1038/srep45288
Goineau A., Gooday A. (2019). Diversity and spatial patterns of foraminiferal assemblages in the eastern Clarion–Clipperton zone (abyssal eastern equatorial Pacific). Deep-Sea Res. Part I 149, 103036. doi: 10.1016/j.dsr.2019.04.014
Gollner S., Kaiser S., Menzel L., Jones D., Brown A., Mestre N., et al. (2017). Resilience of benthic deep-sea fauna to mining activities. Mar. Environ. Res. 129, 76–101. doi: 10.1016/j.marenvres.2017.04.010
Gooday A., Durden J., Holzmann M., Pawlowski J., Smith C. (2020). Xenophyophores (Rhizaria, Foraminifera), including four new species and two new genera, from the western Clarion-Clipperton Zone (abyssal equatorial Pacific). Eur. J. protistology 75, 125715. doi: 10.1016/j.ejop.2020.125715
Gooday A., Goineau A., Voltski I. (2015). Abyssal foraminifera attached to polymetallic nodules from the eastern Clarion Clipperton Fracture Zone: a preliminary description and comparison with North Atlantic dropstone assemblages. Mar. Biodiversity 45, 391–412. doi: 10.1007/s12526-014-0301-9
Gooday A., Holzmann M., Caulle C., Goineau A., Kamenskaya O., Weber A., et al. (2017). Giant protists (xenophyophores, Foraminifera) are exceptionally diverse in parts of the abyssal eastern Pacific licensed for polymetallic nodule exploration. Biol. Conserv. 207, 106–116. doi: 10.1016/j.biocon.2017.01.006
Gooday A., Kitazato H., Hori S., Toyofuku T. (2001). Monothalamous soft-shelled foraminifera at an abyssal site in the North Pacific: a preliminary report. J. Oceanography 57, 377–384. doi: 10.1023/A:1012447015771
Gooday A., Lejzerowicz F., Goineau A., Holzmann M., Kamenskaya O., Kitazato H., et al. (2021). The biodiversity and distribution of abyssal benthic foraminifera and their possible ecological roles: a synthesis across the Clarion-Clipperton Zone. Front. Mar. Sci. 8, 634726. doi: 10.3389/fmars.2021.634726
Gooday A., Levin L., Linke P., Heeger T. (1992). The role of benthic foraminifera in deep-sea food webs and carbon cycling. Deep-sea Food chains Global carbon Cycle 360, 63–91.
Grorud-Colvert K., Sullivan-Stack J., Roberts C., Constant V., Horta e Costa B., Pike E. P., et al. (2021). The MPA Guide: A framework to achieve global goals for the ocean. Science 373, eabf0861. doi: 10.1126/science.abf0861
Hanson C. A., Fuhrman J. A., Horner-Devine M. C., Martiny J. B. (2012). Beyond biogeographic patterns: processes shaping the microbial landscape. Nat. Rev. Microbiol. 10 (7), 497–506. doi: 10.1038/nrmicro2795
Harris P., Westerveld L., Zhao Q., Costello M. (2023). Rising snow line: Ocean acidification and the submergence of seafloor geomorphic features beneath a rising carbonate compensation depth. Mar. Geology 463, 107121. doi: 10.1016/j.margeo.2023.107121
Hauquier F., Macheriotou L., Bezerra T. N., Egho G., Martínez Arbizu P., Vanreusel A. (2019). Distribution of free-living marine nematodes in the Clarion–Clipperton Zone: implications for future deep-sea mining scenarios. Biogeosciences 16, 3475–3489. doi: 10.5194/bg-16-3475-2019
Hein J. R., Koschinsky A., Kuhn T. (2020). Deep-ocean polymetallic nodules as a resource for critical materials. Nat. Rev. Earth Environ. 1 (3), 158–169. doi: 10.1038/s43017-020-0027-0
Hessler R., Jumars P. (1974). Abyssal community analysis from replicate box cores in the central North Pacific. Deep-Sea Res. 21, 185–209. doi: 10.1016/0011-7471(74)90058-8
Hilário A., Metaxas A., Gaudron S., Howell K., Mercier A., Mestre N., et al. (2015). Estimating dispersal distance in the deep sea: challenges and applications to marine reserves. Front. Mar. Sci. 2, 6. doi: 10.3389/fmars.2015.00006
Hogg C. J. (2024). Translating genomic advances into biodiversity conservation. Nat. Rev. Genet. 25, 362–373. doi: 10.1038/s41576-023-00671-0
Hollingsworth A., Jones D., Young C. (2021). Spatial variability of abyssal nitrifying microbes in the north-eastern clarion-clipperton zone. Front. Mar. Sci. 8, 663420. doi: 10.3389/fmars.2021.663420
Horton T., Marsh L., Bett B., Gates A., Jones D., Benoist N., et al. (2021). Recommendations for the standardisation of open taxonomic nomenclature for image-based identifications. Front. Mar. Sci. 8, 620702. doi: 10.3389/fmars.2021.620702
Horton T., Rabone M., Ahyong S. T., Bieler R., Boyko C., N Brandão S., et al. (2024). Tackling Temporary Names: Interim Solutions for the Taxonomic Impediment. doi: 10.2139/ssrn.4917749
Hughes A., Orr M., Ma K., Costello M., Waller J., Provoost P., et al. (2021). Sampling biases shape our view of the natural world. Ecography 44, 1259–1269. doi: 10.1111/ecog.2021.v44.i9
ISA (2021). Review of the Implementation of the Environmental Management Plan for the Clarion-Clipperton Zone Report and Recommendations of the Legal and Technical Commission (Kingston, Jamaica: ISA).
Janssen A., Kaiser S., Meissner K., Brenke N., Menot L., Martínez Arbizu P. (2015). A reverse taxonomic approach to assess macrofaunal distribution patterns in abyssal Pacific polymetallic nodule fields. PloS One 10, e0117790. doi: 10.1371/journal.pone.0117790
Janssen A., Stuckas H., Vink A., Arbizu P. (2019). Biogeography and population structure of predominant macrofaunal taxa (Annelida and Isopoda) in abyssal polymetallic nodule fields: implications for conservation and management. Mar. Biodiversity 49, 2641–2658. doi: 10.1007/s12526-019-00997-1
Jones D., Simon-Lledó E., Amon D., Bett B., Caulle C., Clément L., et al. (2021). Environment, ecology, and potential effectiveness of an area protected from deep-sea mining (Clarion Clipperton Zone, abyssal Pacific). Prog. Oceanography 197, 102653. doi: 10.1016/j.pocean.2021.102653
Jones D., Yool A., Wei C., Henson S., Ruhl H., Watson R., et al. (2014). Global reductions in seafloor biomass in response to climate change. Global Change Biol. 20, 1861–1872. doi: 10.1111/gcb.2014.20.issue-6
Jousset A., Bienhold C., Chatzinotas A., Gallien L., Gobet A., Kurm V., et al. (2017). Where less may be more: how the rare biosphere pulls ecosystems strings. ISME J. 11, 853–862. doi: 10.1038/ismej.2016.174
Kaiser S., Bonifácio P., Kihara T., Menot L., Vink A., Wessels A., et al. (2024). Effects of environmental and climatic drivers on abyssal macrobenthic infaunal communities from the NE Pacific nodule province. Mar. Biodiversity 54, 35. doi: 10.1007/s12526-024-01427-7
Kaiser S., Christodoulou M., Janssen A., Kihara T., Mohrbeck I., Pasotti F., et al. (2023). Diversity, distribution and composition of abyssal benthic Isopoda in a region proposed for deep-seafloor mining of polymetallic nodules: a synthesis. Mar. Biodiversity 53, 30. doi: 10.1007/s12526-023-01335-2
Kaiser S., Kihara T., Brix S., Mohrbeck I., Janssen A., Jennings R. (2021). Species boundaries and phylogeographic patterns in new species of Nannoniscus (Janiroidea: Nannoniscidae) from the equatorial Pacific nodule province inferred from mtDNA and morphology. Zoological J. Linn. Soc. 193, 1020–1071. doi: 10.1093/zoolinnean/zlaa174
Kersken D., Janussen D., Arbizu P. M. (2019). Deep-sea glass sponges (Hexactinellida) from polymetallic nodule fields in the Clarion-Clipperton Fracture Zone (CCFZ), northeastern Pacific: Part II—Hexasterophora. Mar. Biodivers. 49, 947–987.
Kersten O., Vetter E., Jungbluth M., Smith C., Goetze E. (2019). Larval assemblages over the abyssal plain in the Pacific are highly diverse and spatially patchy. PeerJ 7, e7691. doi: 10.7717/peerj.7691
Khodami S., McArthur J., Blanco-Bercial L., Martinez Arbizu P. (2017). RETRACTED ARTICLE: molecular phylogeny and revision of copepod orders (Crustacea: Copepoda). Sci. Rep. 7, 9164. doi: 10.1038/s41598-017-06656-4
Khodami S., Mercado-Salas N., Tang D., Arbizu P. (2019). Molecular evidence for the retention of the Thaumatopsyllidae in the order Cyclopoida (Copepoda) and establishment of four suborders and two families within the Cyclopoida. Mol. Phylogenet. Evol. 138, 43–52. doi: 10.1016/j.ympev.2019.05.019
Krueck N. C., Ahmadia G. N., Green A., Jones G. P., Possingham H. P., Riginos C., et al. (2017). Incorporating larval dispersal into MPA design for both conservation and fisheries. Ecol. Appl. 27, 925–941. doi: 10.1002/eap.2017.27.issue-3
Lambshead P. J. D., Boucher G. (2003). Marine nematode deep-sea biodiversity - Hyperdiverse or hype? J. Biogeogr. 30 (4), 475–485. doi: 10.1046/j.1365-2699.2003.00843.x
Laroche O., Kersten O., Smith C. R., Goetze E. (2020). Environmental DNA surveys detect distinct metazoan communities across abyssal plains and seamounts in the western Clarion Clipperton Zone. Mol. Ecol. 29 (23), 4588–4604. doi: 10.1111/mec.15484
Lagabrielle E., Crochelet E., Andrello M., Schill S., Arnaud-Haond S., Alloncle N., et al. (2014). Connecting MPAs–eight challenges for science and management. Aquat. Conservation: Mar. Freshw. Ecosyst. 24, 94–110. doi: 10.1002/aqc.2500
Laming S., Christodoulou M., Martinez Arbizu P., Hilário A. (2021). Comparative reproductive biology of deep-sea ophiuroids inhabiting polymetallic-nodule fields in the Clarion-Clipperton Fracture Zone. Front. Mar. Sci. 8, 663798. doi: 10.3389/fmars.2021.663798
Lejzerowicz F., Gooday A. J., Barrenechea Angeles I., Cordier T., Morard R., Apothéloz-Perret-Gentil L., et al. (2021). Eukaryotic biodiversity and spatial patterns in the clarion-clipperton zone and other abyssal regions: insights from sediment DNA and RNA metabarcoding. Front. Mar. Sci. 8. doi: 10.3389/fmars.2021.671033
Levin L. A. (2017). Manifestation, drivers, and emergence of open ocean deoxygenation. Annu. Rev. Mar. Sci. 10 (1), 229–260. doi: 10.1146/annurev-marine-121916-063359
Levin L. A., Bris N. L. (2015). The deep ocean under climate change. Science. Am. Assoc. Advancement Sci. 350, 766–768. doi: 10.1126/science.aad0126
Lindh M., Maillot B., Shulse C., Gooday A., Amon D., Smith C., et al. (2017). From the surface to the deep-sea: bacterial distributions across polymetallic nodule fields in the Clarion-Clipperton zone of the Pacific Ocean. Front. Microbiol. 8, 1696. doi: 10.3389/fmicb.2017.01696
Lins L., Zeppilli D., Menot L., Michel L., Bonifácio P., Brandt M., et al. (2021). Toward a reliable assessment of potential ecological impacts of deep-sea polymetallic nodule mining on abyssal infauna. Limnology Oceanography: Methods 19, 626–650. doi: 10.1002/lom3.10448
Lutz M., Caldeira K., Dunbar R., Behrenfeld M. (2007). Seasonal rhythms of net primary production and particulate organic carbon flux to depth describe the efficiency of biological pump in the global ocean. J. Geophysical Research: Oceans 112. doi: 10.1029/2006JC003706
Macheriotou L., Rigaux A., Derycke S., Vanreusel A. (2020). Phylogenetic clustering and rarity imply risk of local species extinction in prospective deep-sea mining areas of the Clarion–Clipperton Fracture Zone. Proc. R. Soc. B: Biol. Sci. 287 (1924). doi: 10.1098/rspb.2019.2666
Malinsky M., Challis R. J., Tyers A. M., Schiffels S., Terai Y., Ngatunga B. P., et al. (2015). Genomic islands of speciation separate cichlid ecomorphs in an East African crater lake. Science 350, 1493–1498. doi: 10.1126/science.aac9927
Malyutina M. V., Frutos I., Brandt A. (2018). Diversity and distribution of the deep-sea Atlantic Acanthocope (Crustacea, Isopoda, Munnopsidae), with description of two new species. Deep Sea Res. Part II: Topical Stud. Oceanography 148, 130–150. doi: 10.1016/j.dsr2.2017.11.003
Manel S., Loiseau N., Andrello M., Fietz K., Goñi R., Forcada A., et al. (2019). Long-distance benefits of marine reserves: myth or reality? Trends Ecol. Evol. 34, 342–354. doi: 10.1016/j.tree.2019.01.002
Mayer G., Müller W., Schork K., Uszkoreit J., Weidemann A., Wittig U., et al. (2021). Implementing FAIR data management within the German Network for Bioinformatics Infrastructure (de. NBI) exemplified by selected use cases. Briefings Bioinf. 22, bbab010. doi: 10.1093/bib/bbab010
McQuaid K., Attrill M., Clark M., Cobley A., Glover A., Smith C., et al. (2020). Using habitat classification to assess representativity of a protected area network in a large, data-poor area targeted for deep-sea mining. Front. Mar. Sci. 7, 558860. doi: 10.3389/fmars.2020.558860
Mejía-Saenz A., Simon-Lledó E., Partridge L. S., Xavier J. R., Jones D. O. (2023). Rock outcrops enhance abyssal benthic biodiversity. Deep-Sea Res. Part I: Oceanogr. Res. Papers 195. doi: 10.1016/j.dsr.2023.103999
Mengerink K. J., Van Dover C. L., Ardron J., Baker M., Escobar-Briones E., Gjerde K., et al. (2014). A call for deep-ocean stewardship. Science 344 (6185), 696–698.
Mercado-Salas N., Khodami S., Arbizu P. (2019). Convergent evolution of mouthparts morphology between Siphonostomatoida and a new genus of deep-sea Aegisthidae Giesbrecht 1893 (Copepoda: Harpacticoida). Mar. Biodiversity 49, 1–21. doi: 10.1007/s12526-018-0932-3
Mestre N., Sousa V., Rocha T., Bebianno M. (2019). Ecotoxicity of rare earths in the marine mussel Mytilus galloprovincialis and a preliminary approach to assess environmental risk. Ecotoxicology 28, 294–301. doi: 10.1007/s10646-019-02022-4
Miljutin D., Gad G., Miljutina M., Mokievsky V., Fonseca-Genevois V., Esteves A. (2010). The state of knowledge on deep-sea nematode taxonomy: how many valid species are known down there? Mar. Biodiversity 40, 143–159. doi: 10.1007/s12526-010-0041-4
Miljutin D., Miljutina M. (2016). Intraspecific variability of morphological characters in the species-rich deep-sea genus Acantholaimus Allgén 1933 (Nematoda: Chromadoridae). Nematology 18, 455–473. doi: 10.1163/15685411-00002970
Miljutin D. M., Miljutina M. A., Arbizu P. M., Galeron J. (2011). Deep-sea nematode assemblage has not recovered 26 years after experimental mining of polymetallic nodules (Clarion-Clipperton Fracture Zone, Tropical Eastern Pacific). Deep-Sea Res. Part I: Oceanogr. Res. Papers 58 (8), 885–897. doi: 10.1016/j.dsr.2011.06.003
Miljutina M. A., Miljutin D. M., Mahatma R., Galéron J. (2010). Deep-sea nematode assemblages of the Clarion-Clipperton Nodule Province (tropical north-eastern Pacific). Mar. Biodiversity 40, 1–15. doi: 10.1007/s12526-009-0029-0
Miller K. J., Gunasekera R. M. (2017). A comparison of genetic connectivity in two deep sea corals to examine whether seamounts are isolated islands or stepping stones for dispersal. Sci. Rep. 7, 46103. doi: 10.1038/srep46103
Min W. G., Kim D., Rho H. S., Chi S. B., Son S. K. (2018). Distribution and variability of the meiobenthic assemblages near the korean polymetallic nodule claim area of the clarion-clipperton fracture zone (Subequatorial NE Pacific). Ocean Sci. J. 53 (2), 315–336. doi: 10.1007/s12601-018-0027-x
Molari M., Janssen F., Vonnahme T., Wenzhöfer F., Boetius A. (2020). The contribution of microbial communities in polymetallic nodules to the diversity of the deep-sea microbiome of the Peru Basin, (4130–4198 m depth). Biogeosciences 17, 3203–3222. doi: 10.5194/bg-17-3203-2020
Nekola J., White P. (1999). The distance decay of similarity in biogeography and ecology. J. Biogeography 26, 867–878. doi: 10.1046/j.1365-2699.1999.00305.x
O’Leary B. C., Roberts C. M. (2018). Ecological connectivity across ocean depths: Implications for protected area design. Global Ecol. Conserv. 15 (2018), e00431. doi: 10.1016/j.gecco.2018.e00431
Orcutt B., Bolton E., Erazo N., Bowman T., Gajigan A., Harris M., et al. (2024). Impacts of deep-sea mining on microbial ecosystem services. Limnol. Oceanogr. 65 (7), 1489–1510. doi: 10.1002/lno.11403
Orcutt B., D’Angelo T., Wheat C., Trembath-Reichert E. (2021). Microbe-mineral biogeography from multi-year incubations in oceanic crust at North Pond, Mid-Atlantic Ridge. Environ. Microbiol. 23, 3923–3936. doi: 10.1111/1462-2920.15366
Pape E., Bezerra T. N., Hauquier F., Vanreusel A. (2017). Limited spatial and temporal variability in meiofauna and nematode communities at distant but environmentally similar sites in an area of interest for deep-sea mining. Front. Mar. Sci. 4. doi: 10.3389/fmars.2017.00205
Pape E., Bezerra T. N., Gheerardyn H., Buydens M., Kieswetter A., Vanreusel A. (2021). Potential impacts of polymetallic nodule removal on deep-sea meiofauna. Sci. Rep. 11, 19996. doi: 10.1038/s41598-021-99441-3
Pechenik J. (1999). On the advantages and disadvantages of larval stages in benthic marine invertebrate life cycles. Mar. Ecol. Prog. Ser. 177, 269–297. doi: 10.3354/meps177269
Pennington J., Mahoney K., Kuwahara V., Kolber D., Calienes R., Chavez F. (2006). Primary production in the eastern tropical Pacific: A review. Prog. oceanography 69, 285–317. doi: 10.1016/j.pocean.2006.03.012
Pulliam H. R. (1988). Sources, Sinks, and Population Regulation. Am. Nat. 132 (5), 652–661. doi: 10.1086/284880
Rabone M., Wiethase J., Simon-Lledó E., Emery A., Jones D., Dahlgren T., et al. (2023). How many metazoan species live in the world’s largest mineral exploration region? Curr. Biol. 33, 2383–2396. doi: 10.1016/j.cub.2023.04.052
Radziejewska T., Drzycimski I., Galtsova V., Kulangieva L., Stoyanova V. (2001). “Changes in genus-level diversity of meiobenthic free-living nematodes (Nematoda) and harpacticoids (Copepoda Harpacticoida) at an abyssal site following experimental sediment disturbance,” in ISOPE Ocean Mining and Gas Hydrates Symposium (ISOPE), ISOPE–ISOPM.
Ramírez G., Garber A., Lecoeuvre A., D’Angelo T., Wheat C., Orcutt B. (2019). Ecology of subseafloor crustal biofilms. Front. Microbiol. 10, 1983. doi: 10.3389/fmicb.2019.01983
Ramirez-Llodra E., Brandt A., Danovaro R., De Mol B., Escobar E., German C. R., et al. (2010). Deep, diverse and definitely different: unique attributes of the world's largest ecosystem. Biogeosciences 7 (9), 2851–2899. doi: 10.5194/bg-7-2851-2010
Renaud-Mornant J., Gourbault N. (1990). Evaluation of abyssal meiobenthos in the eastern central Pacific (Clarion-Clipperton fracture zone). Prog. Oceanogr. 24 (1–4), 317–329. doi: 10.1016/0079-6611(90)90041-Y
Rex M., Etter R., Morris J., Crouse J., McClain C., Johnson N., et al. (2006). Global bathymetric patterns of standing stock and body size in the deep-sea benthos. Mar. Ecol. Prog. Ser. 317, 1–8. doi: 10.3354/meps317001
Riehl T., Smet B. (2020). Macrostylis metallicola spec. nov.–an isopod with geographically clustered genetic variability from a polymetallic-nodule area in the Clarion-Clipperton Fracture Zone. PeerJ 8, e8621. doi: 10.7717/peerj.8621
Rossel S., Uhlenkott K., Peters J., Vink A., Arbizu P. (2022). Evaluating species richness using proteomic fingerprinting and DNA barcoding–a case study on meiobenthic copepods from the Clarion Clipperton Fracture Zone. Mar. Biodiversity 52, 67. doi: 10.1007/s12526-022-01307-y
Ruff S., Biddle J., Teske A., Knittel K., Boetius A., Ramette A. (2015). Global dispersion and local diversification of the methane seep microbiome. Proc. Natl. Acad. Sci. 112, 4015–4020. doi: 10.1073/pnas.1421865112
Schauer R., Bienhold C., Ramette A., Harder J. (2010). Bacterial diversity and biogeography in deep-sea surface sediments of the South Atlantic Ocean. ISME J. 4, 159–170. doi: 10.1038/ismej.2009.106
Selkoe K. A., Aloia C. C., Crandall E. D., Iacchei M., Liggins L., Puritz J. B., et al. (2016). A decade of seascape genetics: contributions to basic and applied marine connectivity. Mar. Ecol. Prog. Ser. 554, 1–19. doi: 10.3354/meps11792
Shiraishi F., Mitsunobu S., Suzuki K., Hoshino T., Morono Y., Inagaki F. (2016). Dense microbial community on a ferromanganese nodule from the ultra-oligotrophic South Pacific Gyre: Implications for biogeochemical cycles. Earth Planetary Sci. Lett. 447, 10–20. doi: 10.1016/j.epsl.2016.04.021
Shulse C. N., Maillot B., Smith C. R., Church M. J. (2016). Polymetallic nodules, sediments, and deep waters in the equatorial North Pacific exhibit highly diverse and distinct bacterial, archaeal, and microeukaryotic communities. MicrobiologyOpen 6 (2), e00428. doi: 10.1002/mbo3.428
Shulse C., Maillot B., Smith C., Church M. (2017). Polymetallic nodules, sediments, and deep waters in the equatorial North Pacific exhibit highly diverse and distinct bacterial, archaeal, and microeukaryotic communities. MicrobiologyOpen 6, e00428. doi: 10.1002/mbo3.2017.6.issue-2
Simon-Lledó E., Baselga A., Gómez-Rodríguez C., Metaxas A., Amon D. J., Bribiesca-Contreras G., et al. (2025). Marked variability in distance-decay patterns suggests contrasting dispersal ability in abyssal taxa. Global Ecol. Biogeogr. 34 (1), e13956.
Simon-Lledó E., Amon D., Bribiesca-Contreras G., Cuvelier D., Durden J., Ramalho S., et al. (2023). Carbonate compensation depth drives abyssal biogeography in the northeast Pacific. Nat. Ecol. Evol. 7, 1388–1397. doi: 10.1038/s41559-023-02122-9
Simon-Lledó E., Bett B., Huvenne V., Schoening T., Benoist N., Jeffreys R., et al. (2019). Megafaunal variation in the abyssal landscape of the Clarion Clipperton Zone. Prog. oceanography 170, 119–133. doi: 10.1016/j.pocean.2018.11.003
Simon-Lledó E., Pomee C., Ahokava A., Drazen J., Leitner A., Flynn A., et al. (2020). Multi-scale variations in invertebrate and fish megafauna in the mid-eastern Clarion Clipperton Zone. Prog. Oceanography 187, 102405. doi: 10.1016/j.pocean.2020.102405
Smith C., Paterson G., Lambshead J., Glover A., Rogers A., Gooday A., et al. (2008). “Biodiversity, species ranges, and gene flow in the abyssal Pacific nodule province: predicting and managing the impacts of deep seabed mining,” in ISA Technical Study, 3 (International Seabed Authority, Kingston, Jamaica).
Smith K. Jr., Ruhl H., Bett B., Billett D., Lampitt R., Kaufmann R. (2009). Climate, carbon cycling, and deep-ocean ecosystems. Proc. Natl. Acad. Sci. 106, 19211–19218. doi: 10.1073/pnas.0908322106
Stewart E., Bribiesca-Contreras G., Taboada S., Wiklund H., Ravara A., Pape E., et al. (2023). Biodiversity, biogeography, and connectivity of polychaetes in the world’s largest marine minerals exploration frontier. Diversity Distributions 29, 727–747. doi: 10.1111/ddi.13690
Sulpis O., Boudreau B. P., Mucci A., Jenkins C., Trossman D. S., Arbic B. K., et al. (2018). Current CaCO3 dissolution at the seafloor caused by anthropogenic CO2. Proc. Natl. Acad. Sci. 115, 11700–11705. doi: 10.1073/pnas.1804250115
Swearer S. E., Treml E. A., Shima J. S. (2019). A review of biophysical models of marine larval dispersal. Oceanogr. Mar. Biol. doi: 10.1201/9780429026379-7
Sweetman A., Thurber A., Smith C., Levin L., Mora C., Wei C., et al. (2017). Major impacts of climate change on deep-sea benthic ecosystems. Elem Sci. Anth 5, 4. doi: 10.1525/elementa.203
Thiel H., Schriever G., Bussau C., Borowski C. (1993).Manganese nodule crevice fauna (Accessed 18 Dec 2024).
Thiel H., Schriever G., Foell E. J. (2005). Polymetallic nodule mining, waste disposal, and species extinction at the abyssal seafloorMarine Georesources and Geotechnology 23, 3, 209–220. doi: 10.1080/10641190500192292
Thurber A. R., Sweetman A. K., Narayanaswamy B. E., Jones D. O. B., Ingels J., Hansman R. L. (2014). Ecosystem function and services provided by the deep sea. Biogeosciences 11, 3941–3963. doi: 10.5194/bg-11-3941-2014
Tong S. J. W., Gan B. Q., Tan K. S. (2022). Community structure of deep-sea benthic metazoan meiofauna in the polymetallic nodule fields in the eastern Clarion-Clipperton Fracture Zone, Pacific Ocean. Deep Sea Res. Part I: Oceanographic Res. Papers 188. doi: 10.1016/j.dsr.2022.103847
Tully B., Heidelberg J. (2013). Microbial communities associated with ferromanganese nodules and the surrounding sediments. Front. Microbiol. 4. doi: 10.3389/fmicb.2013.00161
Uhlenkott K., Vink A., Kuhn T., Martinez Arbizu P. (2020). Predicting meiofauna abundance to define preservation and impact zones in a deep-sea mining context using random forest modelling. J. Appl. Ecol. 57 (7), 1210–1221. doi: 10.1111/1365-2664.13621
Vanreusel A., Hilario A., Ribeiro P., Menot L., Arbizu P. (2016). Threatened by mining, polymetallic nodules are required to preserve abyssal epifauna. Sci. Rep. 6, 26808. doi: 10.1038/srep26808
Varliero G., Bienhold C., Schmid F., Boetius A., Molari M. (2019). Microbial diversity and connectivity in deep-sea sediments of the South Atlantic polar front. Front. Microbiol. 10, 665. doi: 10.3389/fmicb.2019.00665
Veillette J., Sarrazin J., Gooday A., Galéron J., Caprais J., Vangriesheim A., et al. (2007).Ferromanganese nodule fauna in the Tropical North Pacific Ocean: Species richness, faunal cover and spatial distribution (Accessed 18 Dec 2024).
Verlaan P., Cronan D. (2022). Origin and variability of resource-grade marine ferromanganese nodules and crusts in the Pacific Ocean: A review of biogeochemical and physical controls. Geochemistry 82, 125741. doi: 10.1016/j.chemer.2021.125741
Viegas C., Juliano M., Colaço A. (2024). Larval dispersal and physical connectivity of Pheronema carpenteri populations in the Azores. Front. 11. doi: 10.3389/fmars.2024.1393385
Volkmann S. E., Lehnen F. (2018). Production key figures for planning the mining of manganese nodules. Mar. Georesources Geotechnol. 36 (3), 360–375. doi: 10.1080/1064119X.2017.1319448
Volz J., Mogollón J., Geibert W., Arbizu P., Koschinsky A., Kasten S. (2018).Natural spatial variability of depositional conditions, biogeochemical processes and element fluxes in sediments of the eastern Clarion-Clipperton Zone, Pacific Ocean (Accessed 18 Dec 2024).
Vrijenhoek R. C. (1997). Gene flow and genetic diversity in naturally fragmented metapopulations of deep-sea hydrothermal vent animals. J. Heredity 88 (4), 285–293. doi: 10.1093/oxfordjournals.jhered.a023106
Washburn T., Jones D., Wei C., Smith C. (2021a). Environmental heterogeneity throughout the Clarion-Clipperton Zone and the potential representativity of the APEI network. Front. Mar. Sci. 8, 661685. doi: 10.3389/fmars.2021.661685
Washburn T., Menot L., Bonifácio P., Pape E., Błażewicz M., Bribiesca-Contreras G., et al. (2021b). Patterns of macrofaunal biodiversity across the Clarion-Clipperton Zone: an area targeted for seabed mining. Front. Mar. Sci. 8, 626571. doi: 10.3389/fmars.2021.626571
Wear E., Church M., Orcutt B., Shulse C., Lindh M., Smith C. (2021). Bacterial and archaeal communities in polymetallic nodules, sediments, and bottom waters of the abyssal clarion-Clipperton zone: emerging patterns and future monitoring considerations. Front. Mar. Sci. 8, 634803. doi: 10.3389/fmars.2021.634803
Webb T., Vanden Berghe E., O’Dor R. (2010). Biodiversity’s big wet secret: the global distribution of marine biological records reveals chronic under-exploration of the deep pelagic ocean. PloS One 5, e10223. doi: 10.1371/journal.pone.0010223
Wedding L. M., Friedlander A. M., Kittinger J. N., Watling L., Gaines S. D., Bennett M., et al. (2013). From principles to practice: A spatial approach to systematic conservation planning in the deep sea. Proc. R. Soc. B: Biol. Sci. 280 (1773). doi: 10.1098/rspb.2013.1684
Wedding L. M., Reiter S. M., Smith C. R., Gjerde K. M., Kittinger J. N., et al. (2015). Managing mining of the deep seabed. Science 349 (6244), 144–145. doi: 10.1126/science.aac6647
Whittaker R. H. (1960). Vegetation of the Siskiyou mountains, Oregon and California. Ecol. Monogr. 30 (3), 279–338.
Wilkinson M. D., Dumontier M., Aalbersberg I. J., Appleton G., Axton M., Baak A., et al. (2016). The FAIR Guiding Principles for scientific data management and stewardship. Sci. Data 3, 1–9. doi: 10.1038/sdata.2016.18
Wilson G. D. F. (2017). Macrofauna abundance, species diversity and turnover at three sites in the Clipperton-Clarion Fracture Zone. Mar. Biodiv. 47 (2), 323–347. doi: 10.1007/s12526-016-0609-8
Winston J. E. (2012). Dispersal in marine organisms without a pelagic larval phase. Integr. Comp. Biol. 447–457. doi: 10.1093/icb/ics040
Wu Y., Liao L., Wang C., Ma W., Meng F., Wu M., et al. (2013).A comparison of microbial communities in deep-sea polymetallic nodules and the surrounding sediments in the Pacific Ocean (Accessed 18 Dec 2024).
Wu S., Xiong J., Yu Y. (2015). Taxonomic resolutions based on 18S rRNA genes: A case study of subclass copepoda. PloS One 10. doi: 10.1371/journal.pone.0131498
Young C. M., He R., Emlet R. B., Li Y., Qian H., Arellano S. M., et al. (2012). Dispersal of deep-sea larvae from the intra-American seas: simulations of trajectories using ocean models. Integrative and Comparative Biology. 52 (4), 483–496. doi: 10.1093/icb/ics090
Zeppilli D., Vanreusel A., Danovaro R. (2011). Cosmopolitanism and biogeography of the genus Manganonema (Nematoda: Monhysterida) in the deep sea. Animals 1, 291–305. doi: 10.3390/ani1030291
Zinger L., Amaral-Zettler L., Fuhrman J., Horner-Devine M., Huse S., Welch D., et al. (2011). Global patterns of bacterial beta-diversity in seafloor and seawater ecosystems. PloS One 6, e24570. doi: 10.1371/journal.pone.0024570
Keywords: Clarion and Clipperton Fracture Zone, megafauna, macrofauna, meiofauna, microbes, connectivity, review
Citation: Macheriotou L, Rossel S, Molari M, Martinez Arbizu P, Brix S, Christodoulou M, Dessandier P-A, Hilário A, Janssen F, Simon-Lledó E, Menot L, Pape E, Ramírez GA and Vanreusel A (2025) Connectivity in the Clarion-Clipperton Zone: a review. Front. Mar. Sci. 12:1547803. doi: 10.3389/fmars.2025.1547803
Received: 18 December 2024; Accepted: 10 March 2025;
Published: 14 April 2025.
Edited by:
Elva G. Escobar-Briones, National Autonomous University of Mexico, MexicoReviewed by:
Sandor Mulsow, Austral University of Chile, ChileCopyright © 2025 Macheriotou, Rossel, Molari, Martinez Arbizu, Brix, Christodoulou, Dessandier, Hilário, Janssen, Simon-Lledó, Menot, Pape, Ramírez and Vanreusel. This is an open-access article distributed under the terms of the Creative Commons Attribution License (CC BY). The use, distribution or reproduction in other forums is permitted, provided the original author(s) and the copyright owner(s) are credited and that the original publication in this journal is cited, in accordance with accepted academic practice. No use, distribution or reproduction is permitted which does not comply with these terms.
*Correspondence: Lara Macheriotou, bGFyYS5tYWNoZXJpb3RvdUB1Z2VudC5iZQ==
Disclaimer: All claims expressed in this article are solely those of the authors and do not necessarily represent those of their affiliated organizations, or those of the publisher, the editors and the reviewers. Any product that may be evaluated in this article or claim that may be made by its manufacturer is not guaranteed or endorsed by the publisher.
Research integrity at Frontiers
Learn more about the work of our research integrity team to safeguard the quality of each article we publish.