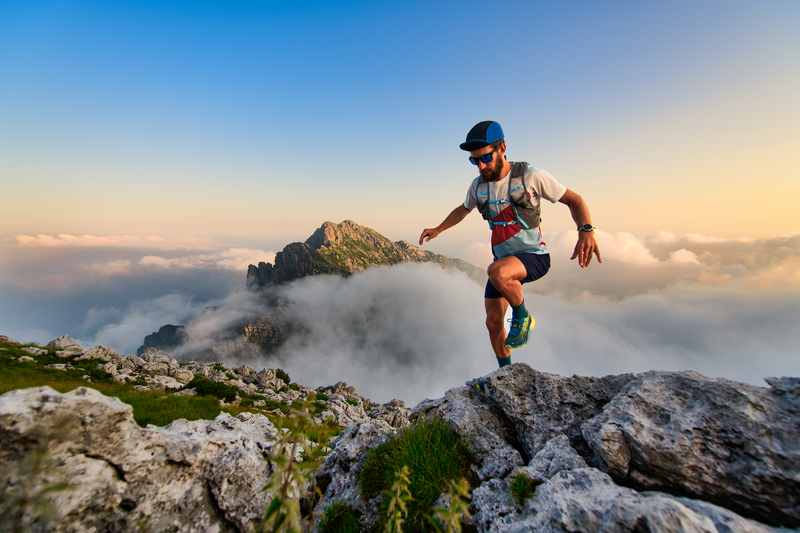
95% of researchers rate our articles as excellent or good
Learn more about the work of our research integrity team to safeguard the quality of each article we publish.
Find out more
ORIGINAL RESEARCH article
Front. Mar. Sci. , 14 March 2025
Sec. Marine Pollution
Volume 12 - 2025 | https://doi.org/10.3389/fmars.2025.1545131
Introduction: Microplastics (MPs) and tetracycline (TC) are pervasive contaminants in marine ecosystems, yet their combined effects on benthic organisms, such as Aurelia aurita polyps, remain poorly understood. This study investigates the mechanisms of cellular apoptosis, oxidative stress, and metabolic responses induced by single and combined exposures to MPs and TC.
Methods: Three experimental phases were conducted: (1) A 7-day exposure to MPs (1 mg/L) and TC (5 mg/L) to assess apoptosis via TUNEL assay; (2) Short-term high-concentration exposure (MPs: 10 mg/L, TC: 5 mg/L) for 72 hours, followed by a 288-hour recovery period, with antioxidant indicators (CAT, GSH, SOD, MDA, etc.) measured at intervals; (3) Long-term exposure (185 days) to environmentally relevant concentrations of MPs (0–1 mg/L) and TC (0–5 mg/L), with metabolomic profiling via LC-MS and pathway analysis. Polyp cultures were maintained under controlled conditions, and statistical analyses included two-way ANOVA and multivariate models (PCA, OPLS-DA).
Results: The TUNEL assay revealed significantly higher apoptosis rates in the MPs+TC group compared to controls or single-pollutant groups (P < 0.05). Antioxidant capacity tests indicated persistent oxidative damage in the MPs group even after 288 hours of recovery. Metabolomics identified distinct physiological strategies for MPs and TC, with altered pathways (e.g., ABC transporters, protein digestion) and disrupted metabolites (antioxidants, neurotransmitters). Notably, TC exhibited non-linear toxicity, with high concentrations not consistently exacerbating harm.
Discussion: Combined exposure to MPs and TC induced synergistic stress in Aurelia aurita polyps, elevating apoptosis and causing irreversible oxidative damage (e.g., sustained T-AOC decline, MDA accumulation). TC paradoxically mitigated oxidative stress in co-exposure groups, likely via antibacterial effects. Long-term exposure disrupted metabolic pathways (e.g., ABC transporters, arachidonic acid) and neurotransmitter levels, impairing stress resilience and intercellular communication. MPs also altered retinoic acid and indole derivatives, potentially interfering with life-history transitions. While A. aurita exhibited tolerance, persistent damage underscores risks for less resilient benthic species. These findings highlight the need to address cumulative ecological impacts of marine pollutants through enhanced regulation and mechanistic studies.
Plastic products degrade over time through aging, weathering, and various physical and chemical processes, decomposing into fragments. Microplastics (MPs) defined as fragments smaller than 5 mm, can enter ecosystems and are widely dispersed globally through ocean currents, runoff, and human activity (Talang et al., 2024). The extensive distribution of MPs has resulted in considerable ecological pollution challenging to address and irreversible. MPs particle size decreases annually, yet their quantity increases exponentially (Hale et al., 2020). The smaller size of MP particles increases their relative surface area and mobility within the environment while also enhancing their ecotoxicity. Studies of tilapia (Oreochromis niloticus) exposed to varying MP sizes have indicated that smaller particles cause more severe damage (Wu et al., 2024). MPs are similar to certain plankton in size and density, making them easy prey for aquatic organisms like zooplankton. MPs reduce antioxidant defenses in various species such as fish, microalgae, and corals, leading to oxidative damage and increased reactive oxygen species (ROS) within the body. These particles accumulate in tissues and organs, including the gills, intestines, and liver, resulting in reduced growth, reproductive decline, and even mortality (Andrady, 2011). MPs are also known to induce cellular apoptosis (Mai et al., 2023). Accumulated MPs can move progressively up the food chain, ultimately affecting human health (Kahane-Rapport et al., 2022). Pathological examination has identified MPs in human tissues, including the placenta (Ragusa et al., 2021), lungs (Amato-Lourenço et al., 2021), and arteries (Liu et al., 2024). This evidence highlights the urgent and concerning levels of harmful effects of MPs.
MPs also act as carriers, adsorbing and compounding like plastic additives (Da Costa et al., 2023), heavy metal ions (Godoy et al., 2019), polycyclic aromatic hydrocarbons, and polychlorinated biphenyls (Sharma et al., 2020). When microplastics absorb various substances, their density increases, causing them to sink and be taken up by organisms living on the ocean floor (Bour et al., 2018). MPs with lower density can move along the surface currents of the ocean and accumulate in oceanic vortices (Law et al., 2010). Meanwhile, MPs with higher density may be transported and moved by deep ocean currents during the sinking process. This variability in density makes microplastics detectable at nearly every level of the food chain (Engler, 2012). Researchers have found that pollutants transported by microplastics accumulate in the tissues of the organisms they invade, and in some instances, these pollutants are later released—much like the behavior of viral particles (Zuo, 2019; Yang and Huang, 2019). Harmful bacteria can also adhere to MPs and travel through ocean currents. For example, the coral pathogen (Halofolliculina spp.), which is typically found in the Indian and Southern Oceans, has been observed on plastic debris in the North Pacific (Goldstein et al., 2014).
As an affordable antibiotic, tetracycline is extensively used in animal husbandry and aquaculture, ultimately enters marine environments. MPs can act as carriers for antibiotics, adsorbing them into the environment and partially inhibiting their degradation, thereby increasing the risk of antibiotic-resistant gene proliferation. Experiments on microalgae (Coelastrella sp.) demonstrated that tetracycline significantly reduced growth rates and activated a response to eliminate ROS (Tong et al., 2020). Another major ecological concern of tetracycline is its role in promoting the accumulation of antibiotic resistance genes (ARGs). Tetracycline resistance genes are now prevalent in many polluted water bodies (Antos et al., 2024), minimum inducing concentration (MIC) of these genes is as low as 1-10 μg/L (Lundström et al., 2016). Moreover, interactions with MPs facilitate ARG accumulation, leading to an increase in antimicrobial-resistant bacteria (ARB) in contaminated areas.
The genus Aurelia is widely distributed across oceans and plays a crucial role in marine ecosystems. Since the 1990s, it has been implicated in numerous jellyfish blooms, leading to disruptions in coastal ecosystems and economic development. When Aurelia reaches high abundance, it significantly affects coastal plankton communities (Lucas, 2001) and damages coastal aquaculture, including fish gill injuries (Baxter et al., 2011) and sea cucumber stress responses (Dong, 2019). Known for its wide distribution, it demonstrates remarkable adaptability and resilience to various pollutants, including elevated carbon dioxide levels (Algueró-Muñiz et al., 2016) and radiation environments (Chen, 2021). The complex life cycle of A. aurita, with stages such as planula, ephyrae, and medusa classified as planktonic, and strobila and polyp stages as benthic, enables it to thrive across diverse marine environments. Researchers have identified A. aurita as a potential indicator organism for assessing marine ecological toxicity (Echols et al., 2016). The polyp stage is crucial for Aurelia bloom formation. As benthic organisms, A. aurita polyps use tentacles to capture small planktons as food. In turn, they serve as prey for species such as Hyastenus diacanthus, Sakuraeolis enosimensis, and various manatees, thereby entering the food chain (Takao et al., 2014). Residual toxins in A. aurita polyps may accumulate, posing a potential ecological risk.
The effect of MPs on A. aurita has been documented in several studies. A. aurita polyps can attach to plastic substrates in natural environments (Feng et al., 2017). Newborn ephyrae (day 0 post-strobilation) were exposed to PE MPs of 1–4 μm to assess toxicity with immobility as the endpoint. The results indicated the acute toxicity from MPs, with a 24-hour EC50 of 1.36 mg/L in static exposure and 4.36 mg/L in semi-dynamic exposure (Costa et al., 2020). Even at low concentrations (below 0.01 mg/L), MPs significantly affected the pulsing frequency, whereas particle size did not notably influence the activities in this study. After the recovery experiment, only a few MPs adhered to the mouthparts of the disc-shaped bodies. Juvenile A. aurita medusae ingested MPs solely through predatory behavior under laboratory conditions (Romero-Kutzner et al., 2022). MPs could disperse throughout bodies of larger adults of A. aurita upon exposure, while showing no evident physiological toxicity (Sucharitakul et al., 2020). Aurelia exhibits different physiological capacities across life stages, its response to MPs varies (Costa et al., 2020). High concentrations of tetracycline can negatively affect the statolith and nematocyst of A. aurita ephyrae, reducing statolith size and causing defects in nematocyst clusters (Spangenberg and Beck, 1972).
The combined stress effects of MPs and tetracycline on A. aurita remain unclear. Based on prior studies, we hypothesized that short- and long-term treatment with MPs, with and without tetracycline as a co-contaminant, would exert varying ecotoxic and biological effects on A. aurita. In this study, the short-term effects were assessed by measuring antioxidant-related molecules and apoptosis rate test in A. aurita polyps. Additionally, metabolomic changes in polyps following long-term exposure (185 d) to MPs and tetracycline were analyzed. The experimental design sought to determine whether short-term exposure could result in physiological damage that was challenging to reverse within a short timeframe, and to evaluate whether pollutant removal through water changes could mitigate this damage. Long-term experiments focused on alterations in metabolic profiles and the impact on the metabolic pathways of A. aurita when exposed to a polluted environment for a long time.
The MPs used in the experiment were spherical polystyrene (PS) particles (80 nm in size) sourced from Tianjin Bessler Chromatography Technology Development Center (Tianjin, China). Tetracycline hydrochloride was obtained from Shanghai McLean Biochemical Technology Co., Ltd. (Shanghai, China).
A. aurita polyps were obtained from the Hongyuan breeding base in Beihai, and the parent medusae were collected from the coast of Hong Kong. Joint phylogenetic analysis of the mt-COI and 16S gene fragments confirmed the species to be Aurelia aurita (Jian-Yan et al., 2013).
Prior to the experiment, the polyps were cultured in flat cylindrical containers (diameter: 134 mm, depth: 83.4 mm) containing 500 mL of artificial seawater with a salinity of 30 PSU and maintained at 25°C in the dark. Artificial seawater was prepared by mixing Tropic Marin® Sea Salt (Germany) with ultrapure water. The polyps were fed ad libitum with newly hatched Artemia sp. nauplii for 3–4 h once or twice weekly, followed by a change in artificial seawater.
Before the experiment, 12 healthy polyps of similar sizes were selected. Each polyp was carefully detached from its attachment point using a dissecting needle and gently transferred to a new rectangular container (80 mm × 80 mm × 54 mm) containing 50 mL of artificial seawater. The polyps were kept in the dark for 7-10 d until they were fully attached. Once attached, they were fed as previously described.
To assess whether MPs, with or without tetracycline hydrochloride, induced cellular apoptosis, a two-factor, two-level orthogonal experiment was conducted with stimulation concentrations reflecting environmental pollution levels (Figure 1A). Three polyp replicates were prepared for each group for a total of 12 polyps. To simulate the natural oceanic environment, polyp culture containers were placed on a shaker (Shanghai Binglin Electronic Technology Co., Ltd.) at a speed of 30 rpm to mimic current movement. The experiment was conducted over seven days, with polyps preserved in darkness and without food.
Figure 1. Diagram of experimental method design. (A) TUNEL assay process; (B) antioxidant assay process; (C) Metabolomics experiment design process. ASW, artificial seawater control group; MPs, single microplastic treatment group; TC, single tetracycline treatment group; CO, combined pollutant treatment group; LTC, low concentration tetracycline treatment group; HTC, high concentration tetracycline treatment group; LMPs, low concentration microplastics treatment group; LTLP, low concentration tetracycline combined with low concentration microplastic treatment group; HTLP, high concentration tetracycline combined with low concentration microplastic treatment group; HMPs, high concentration microplastic treatment group; LTHP, low concentration tetracycline combined with high concentration microplastic treatment group; HTHP, low concentration tetracycline combined with high concentration microplastic treatment group.
After the experiment, the polyps were rinsed 2–3 times with a pre-cooled PBS solution and then fixed with 4% paraformaldehyde. Samples were carefully detached from the container using a dissecting needle and processed into paraffin sections. TUNEL assay (Servicebio®, Wuhan, China) was performed according to the manufacturer’s instructions.
The A. aurita polyps were exposed to MPs and MP-tetracycline hydrochloride composites for 72 h, followed by a recovery period (Figure 1B). The MPs and tetracycline hydrochloride concentrations matched those used in the TUNEL assay. In the MP-only treatment group, the microplastic concentration was 10 mg/L. In the combined treatment, the tetracycline hydrochloride concentration was 5 mg/L, whereas the microplastic concentration remained at 10 mg/L. Polyps were not fed during the culture period, and the temperature was maintained at 25°C to stabilize the polyp stage by placing into incubators away from light. Water was refreshed every 24 h to ensure the concentration of environmental pollutants and avoid their degradation. After 72 h, all groups were transferred to artificial seawater for recovery. Each treatment was replicated 3–5 times.
Sampling was performed 0, 1, 6, 24, 96, 144, and 288 h after exposure to pollutants. At each sampling time point, more than 150 polyps were collected for each replicate from each group to meet the minimum sampling requirements for subsequent tissue homogenization and kit assays. Using a dissecting needle, the polyps were detached from their attachment points and transferred into a small Petri dish containing 4°C sterilized PBS, where they were gently rinsed 2–3 times. Excess PBS was allowed to drain naturally by positioning the polyps on the petri dish wall. The polyps were then transferred to sterilized centrifuge tubes, and their weights were recorded. Four times the polyp weight in physiological saline was then added using a pipette, and the tissue homogenate was prepared using a mechanical wall disruption method on ice, followed by centrifugation.
Centrifugation conditions were adjusted according to the manufacturer’s instructions for subsequent experiments. Kits for total antioxidant capacity (T-AOC), superoxide dismutase (SOD), glutathione peroxidase (GSH-Px), glutathione (GSH), catalase (CAT), malondialdehyde (MDA), and total protein (TPN) were sourced from the Nanjing Jiancheng Bioengineering Institute (Nanjing, China). These physiological indices were measured as markers of oxidative damage and biological activity using procedures and calculations performed according to the manufacturer’s guidelines. Significant differences were analyzed using two-way ANOVA and Tukey’s multiple comparisons test, both the analysis and graphical results were generated using GraphPad Prism 9.5.1.
A two-factor orthogonal experiment was designed to investigate the effects of prolonged exposure to various concentrations of MPs and tetracycline hydrochloride on A. aurita polyps to simulate environmental pollution levels (Figure 1C). The MP concentrations were set at three levels (0, 0.1, and 1 mg/L) and tetracycline hydrochloride at three levels (0, 0.5, and 5 mg/L), resulting in nine treatment combinations. Each group included approximately 100 polyps with three replicates. The experiment was conducted at 25°C in darkness, with polyps fed weekly and water changed 1–2 h post-feeding. After 185-day exposure, the polyps were collected using a dissecting needle, rinsed twice in PBS, then in ultrapure water, and rapidly frozen in liquid nitrogen.
An appropriate amount of sample was added to a 2 mL centrifuge tube, followed by the addition of 1000 μL of tissue extract (75% [9:1 methanol: chloroform]: 25% H2O) and steel balls. The sample was placed in a tissue grinder and ground at 50 Hz for 60 s, which was repeated twice. Ultrasound treatment was performed at room temperature for 30 min, followed by an ice bath for 30 min. The sample was centrifuged at 12,000 rpm and 4°C for 10 min, and the supernatant was collected and transferred to a new 2 mL centrifuge tube. The supernatant was concentrated and dried, and 200 μL of a 50% acetonitrile solution prepared with 2-chloro-l-phenylalanine (4 ppm) was added to re-dissolve the sample. The supernatant was filtered through a 0.22 μm PTFE membrane and transferred to a detection vial for LC-MS analysis (Warren et al., 2017).
LC analysis was conducted using a Vanquish UHPLC System (Thermo Fisher Scientific, USA) with an ACQUITY UPLC® HSS T3 column (2.1 × 100 mm, 1.8 μm) (Waters, Milford, MA, USA). The separation and parameter settings followed reference standards (Zelena et al., 2009).
Metabolite detection was performed using an Orbitrap Exploris 120 mass spectrometer (Thermo Fisher Scientific, USA) with an ESI ion source, following the parameter settings outlined in the literature (Want et al., 2013).
Raw data were first converted to the mzXML format using MSConvert in the ProteoWizard software package (v3.0.8789) (Rasmussen et al., 2022) and processed with R XCMS (v3.12.0) for feature detection (Navarro-Reig et al., 2015). The metabolites were identified using accurate mass and MS/MS data and matched against HMDB (http://www.hmdb.ca) (Ds, 2007), MassBank (http://www.massbank.jp/) (Horai et al., 2010), KEGG (https://www.genome.jp/kegg/) (Ogata et al., 1999), LipidMaps (http://www.lipidmaps.org) (Sud et al., 2007), mzcloud (https://www.mzcloud.org) (Abdelrazig et al., 2020), and the metabolite database developed by Panomix Biomedical Tech Co., Ltd. (Shuzhou, China).
Two multivariate statistical analysis models, unsupervised and supervised (PCA, PLS-DA, and OPLS-DA), were applied to distinguish between groups using the R package ropls (v1.22.0) (Thévenot et al., 2015). Differential metabolites were analyzed for pathway involvement using MetaboAnalyst (Xia and Wishart, 2011).
The TUNEL apoptosis in situ hybridization detection experiment (Figure 2; Supplementary Figure S1) revealed that microplastic composite pollutants induced apoptosis in A. aurita polyps, with most apoptotic sites concentrated in the outer skin and a few within the mesoglea, where plastic contact occurs. Although TUNEL results indicated that MPs alone did not significantly affect apoptosis in A. aurita polyps, the apoptosis rate increased markedly when MPs were combined with tetracycline (P < 0.05) (Figure 3).
Figure 2. Apoptosis of Aurelia aurita polyps after 7 d of exposure to MPs/tetracycline contaminants. Cell nuclei stained with DAPI were blue under ultraviolet excitation, the TUNEL kit was labeled with the CY3 fluorescent element, and the positive apoptotic cell nuclei were red. Overlap between blue and red indicates the point of apoptosis. ASW, artificial seawater control group; MPs, single microplastic treatment group; TC, single tetracycline treatment group; CO, combined pollutant treatment group. Scale bar: 50 μm.
Figure 3. Apoptosis rate of Aurelia aurita polyps after exposure to MPs/tetracycline pollutants for 7 d. ASW, artificial seawater control group; MPs, single microplastic treatment group; TC, single tetracycline treatment group; CO, combined pollutant treatment group. Means with different letters are significantly different. Horizontal bars: SD.
In the first hour following microplastic exposure, the total protein (TP) level (Figure 4A) in the microplastic-treated group (MP) increased significantly higher than that in the control group (P < 0.01). After 6 h, the TP levels dropped significantly below those in the control group (P < 0.01). Between 6 and 72 h, TP gradually increased, with levels at 6, 24, and 72 h significantly higher than those in the control group. Following the water change, TP levels declined from 72 to 144 h and remained significantly elevated compared to the control group at 72 and 96 h. At 144 h and 288 h, the TP values were similar to those in the control group, showing no significant differences.
Figure 4. Changes in enzyme activities in Aurelia aurita polyps exposed to MPs and MPs/tetracycline mixed pollutants at 1, 6, and 24 h, and the change in enzyme activities in polyps at 24, 72, and 216 h after 72 h of replacing microplastics and MPs/tetracycline mixed pollutants with artificial seawater. In order to clearly show the trend of various indicators after the body is stimulated in a short period of time, the 0-6 hours are partially enlarged. The dotted line at 72 h indicates the time point of water change. (A) TP; (B) GSH; (C) GSH-PX; (D) SOD; (E) MDA; (F) CAT; (G) T-AOC.
In the MP+TCHCl treatment group, TP levels decreased significantly compared to the control group after the initial treatment and continued to decline over the first 6 h. The TP levels gradually increased from 6 to 72 h. At 24 h, there was no significant difference between the MP+TCHCl and control groups, whereas at 72 h, the TP levels were significantly higher than those in the control group. Similar to the single MP treatment group, the TP levels in the MP+TCHCl group decreased shortly after exposure and then gradually increased from 6 to 72 h. Following a water change, TP levels gradually declined, with the 288-hour group showing higher levels than the 144-hour group. The primary difference between the MP and MP+TCHCl groups was observed within 1 h of exposure. TP levels in the single MP group increased significantly, whereas those in the tetracycline hydrochloride group significantly decreased. With the exception of the 6-hour and 72-hour points (P > 0.05), significant differences were noted between the two treatments (P < 0.05).
In the MP group, CAT secretion (Figure 4B) significantly decreased 1 h after exposure compared to that in the control group (P < 0.01) and then increased markedly at 6 h (P < 0.005), displaying a fluctuating pattern. Between 6 and 72 h, CAT secretion gradually decreased in the MP group. Following a water change, the CAT levels gradually increased from 72 to 144 h, with statistically significant increases at 72 and 96 h compared to the control group. From 144 to 288 h, no significant differences were observed between the MP and control groups.
The CAT secretion trend in the MP+TCHCl group mirrored that in the MP group. The CAT levels significantly decreased 1 h after exposure, followed by an increase at 6 h. From 6 to 72 h, CAT secretion gradually declined, with no significant differences observed at 24 and 72 h compared with the MP group. After a water change, CAT secretion increased at 96 h but then gradually declined from 96 to 288 h relative to the control group. A notable increase in CAT secretion was observed at 6 h, with significant differences at 1, 24, 72, and 96 h, indicating a general downregulating trend.
In the MP group, GSH levels (Figure 4C) increased slightly 1 h after exposure, with values from 6 to 288 h remaining below those of the control group. The MP+TCHCl group followed a similar trend, with a larger increase at the 1-hour mark. No significant differences in GSH secretion were observed between the MP and MP+TCHCl groups compared with the control group across various time points (P > 0.05), except for a significant difference between the MP and MP+TCHCl groups at 24 h (P < 0.05). No significant differences were found between the MP and MP+TCHCl groups (P > 0.05).
In the MP group, GSH-Px levels (Figure 4D) decreased 1 h after MP exposure and exhibited a brief increase at 6 h, whereas neither change was significant compared to the control group. From 6 to 72 h, GSH-Px levels continued to decline, with a significant decrease observed between 24 and 72 h (P < 0.05). The levels then gradually increased from 96 to 288 h, with no significant difference from the control group.
In the MP+TCHCl group, GSH-Px levels increased significantly between 1 and 6 h after exposure, with a notable statistical difference compared to the control group (P < 0.001). The levels then gradually declined from 6 to 72 h and remained significantly higher than that of the control at 24 h. After 72 h, the difference from the control was no longer significant (P > 0.05). After a water change, the GSH-Px levels at 96 h were slightly lower than the control, but not significantly. At 144 h, the secretion levels increased significantly, exceeding those in both the control and MP groups (P < 0.001). At 288 h, the levels decreased but remained significantly higher than those in the control (P < 0.05), with no significant difference from the MP group.
In the MP group, MDA levels (Figure 4E) initially decreased within the first 6 h post-exposure, increased at 24 h, gradually declined from 24 to 144 h, and then rose again at 288 h. Significant differences from the control group were observed only at 24 and 288 h.
The MP+TCHCl group demonstrated no significant difference from the control group but differed significantly from the MP group at 24, 72, and 288 h.
The SOD activity (Figure 4F) in the MP group decreased significantly compared to the control group within the first hour of exposure (P < 0.005), increased after 6 h, although not significantly (P > 0.05), and then dropped sharply at 24 h (P < 0.0001). At 72 h, SOD levels significantly increased above those of the control group (P < 0.0001) but decreased again at 96 h (P < 0.0001). At 144 h, the levels increased slightly without a significant difference from the control group and then dropped at 288 h to a significantly lower level. Overall, SOD activity in the MP group exhibited marked fluctuations.
Following MP+TCHCl exposure, SOD activity increased slightly in the first hour, decreased at 6 h, and increased again at 24 h, without significant differences from the control group at these times. However, significant differences were observed compared to the MP group. At 72 h, the SOD activity increased slightly, demonstrating a significant difference from both the control and MP groups (P < 0.0001). At 96 h, SOD decreased significantly compared to the control group (P < 0.05), with no significant difference from the MP group (P > 0.05). An increase was observed at 144 h, with no significant difference observed between the control and MP groups (P > 0.05). At 288 h, SOD levels decreased significantly below those in both the control group (P < 0.0001) and the MP group (P < 0.0005).
Following treatment in the MP group, T-AOC levels (Figure 4G) initially decreased in the first hour and were not significantly different from those in the control group (P > 0.05). At 6 h, T-AOC significantly increased (P < 0.005) but then steadily declined from 6 to 72 h, with statistically significant differences from the control group (P < 0.05) at all time points except 24 h. After 72 and 96 h, the T-AOC levels decreased to nearly zero. After replacing the water with ASW at 72 h, T-AOC levels at 96, 144, and 288 h were significantly lower than those in the control group (P < 0.005).
Following MP+TCHCl exposure, the T-AOC levels increased after 1 h, with no significant difference from the control group (P > 0.05) but a significant difference from the MP group (P < 0.05). Levels decreased at 6 h, showing a significant difference from those in the MP group (P < 0.0001), but not in the control group. T-AOC increased at 24 h, remaining non-significant compared to the control group (P > 0.05) but significantly different from the MP group. At 72 h, T-AOC dropped sharply to nearly zero, aligning with the MP group trend and indicating no significant difference. After the water change, T-AOC gradually recovered from 96 to 288 h, with no significant difference from the control group (P > 0.05) but was significantly different from the MP group (P < 0.05).
The validation parameters of the PCA combined with OPLS-DA model can be seen in Table 1. The PCA score plot (Figures 5A, C) indicated a clear separation trend of the ASW group from the other groups in both positive and negative ion modes, while the composite pollutants showed some overlap. The PCA results for both ion modes revealed similar sample characteristics and stable experimental data, demonstrating the successful modeling of this experiment.
Figure 5. PCA and OPLS-DA score plots of the 27 samples. The groups are listed on the right-hand side of the figure. (A) PCA Score plot (+); (B) OPLS-DA Score Plot (+); (C) PCA Score plot (-); (D) OPLS-DA Score plot (-). ASW, artificial seawater treatment control group; LTC, low concentration tetracycline treatment group; HTC, high concentration tetracycline treatment group; LMPs, low concentration microplastics treatment group; LTLP, low concentration tetracycline combined with low concentration microplastic treatment group; HTLP, high concentration tetracycline combined with low concentration microplastic treatment group; HMPs, high concentration microplastic treatment group; LTHP, low concentration tetracycline combined with high concentration microplastic treatment group; HTHP, low concentration tetracycline combined with high concentration microplastic treatment group.
In the OPLS-DA score plot (Figures 5B, D), the R2Y model was determined for the single microplastic pollution group, where R2Y represents the model’s explanatory power for the Y matrix and Q reflects the predictive ability. In the single microplastic treatment in the positive ion mode. R2Y reached 1, and Q2 reached 0.985. In negative ion mode, R2Y and Q2 were 0.993 and 0.929, respectively. Model verification across all samples yielded R2Y values of 0.992 and 0.998 for the positive and negative ion modes, respectively, indicating high model reliability. Both the OPLS-DA and PCA results confirmed that sample quality was appropriate for further metabolomic analysis.
The total metabolite graph (Supplementary Figure S2) reveals distinct patterns of up- and downregulated metabolites in the composite MP and tetracycline hydrochloride groups compared to the single microplastic or tetracycline treatment groups. This suggested that composite pollutants had a unique impact on A. aurita polyps, which differed from those of individual pollutants.
Among the primary metabolites, several bioactive compounds involved in the life history transition and morphological changes of A. aurita were also affected, notably including two main categories: indole and its derivatives and retinol (Figure 6).
Figure 6. Regulation-related metabolite cluster heat map. The columns represent samples, the rows represent metabolites, the cluster tree on the left is the differential metabolite cluster tree, and the top is the sample cluster tree. The gradient color represents the size of the quantitative values. The redder the color, the higher the expression; the bluer the color, the lower the expression. ASW, artificial seawater treatment control group; LTC, low concentration tetracycline treatment group; HTC, high concentration tetracycline treatment group; LMPs, low concentration microplastics treatment group; LTLP, low concentration tetracycline combined with low concentration microplastic treatment group; HTLP, high concentration tetracycline combined with low concentration microplastic treatment group; HMPs, high concentration microplastic treatment group; LTHP, low concentration tetracycline combined with high concentration microplastic treatment group; HTHP, low concentration tetracycline combined with high concentration microplastic treatment group.
The secondary metabolite difference diagram (Figure 7) illustrates that following MP stimulation, the body expressed various antioxidant substances. The secretion levels of some antioxidants were higher at low concentrations, with no significant differences observed at higher concentrations. This suggested that antioxidant substances were preferentially released in response to low-concentration stimulation.
Figure 7. Secondary differential metabolite heat map. The columns represent samples, the rows represent metabolites, the cluster tree on the left is the differential metabolite cluster tree, and the top is the sample cluster tree. The gradient color represents the size of the quantitative values. The redder the color, the higher the expression; the bluer the color, the lower the expression. ASW, artificial seawater treatment control group; LMPs, low concentration microplastics treatment group; HMPs, high concentration microplastic treatment group.
Following stimulation, various neurotransmitters, excitatory stimulators, and inhibitors demonstrated the significant up- and downregulation, including choline, amino acid neurotransmitters, and arachidonic acid metabolism-related neurotransmitters. Microplastic exposure notably decreased choline neurotransmitter levels and expression of the neuroexcitability inhibitor 11,12-EET. In addition to antioxidants and neurotransmitters, body proteins were also affected by MPs.
Following tetracycline treatment, the concentrations of certain substances decreased with higher tetracycline levels, including antioxidants such as trans-cinnamate and alpha-linolenic acid, prostaglandin precursors such as androstenedione, neurotransmitter nicotine, linoleic and bovinic acids, progesterone intermediate 21-deoxycortisol (Barnard et al., 2017), and N-acetyl-L-2-amino-6-oxopimelate. In contrast, substances that increased with higher tetracycline concentrations were mainly metabolic intermediates, such as isocitrate, glyceric acid, arachidonic acid metabolite 19(S)-HETE, L-cystine, and dehypoxanthine futalosine.
The correlation difference diagram (Supplementary Figure S3) indicates that in the microplastic treatment group, 12-keto-leukotriene, phthalic acid, and nonadecanoic acid were predominantly negatively correlated with other metabolites. Phthalic acid is a plastic additive that is not synthesized by organisms and exhibits a negative correlation with various antioxidant substances. This suggested that microplastic additives could stimulate oxidative damage. Additionally, 12-keto-leukotriene, an oxidative inflammatory factor in the arachidonic acid metabolic pathway, is negatively correlated with multiple antioxidants in this pathway.
KEGG pathway analysis (Figures 8, 9) demonstrated that MPs significantly affected the ABC transporter metabolic pathway, followed by beta-alanine metabolism and taste transduction, when compared to the control group. Tetracycline has a higher impact on kegg pathways such as protein digestion and absortion compared to single MPs treated groups. After compounding with two pollutants, the P values of some neurotransmission synthesis pathways and substance metabolism pathways increased, such as GABAergic synpase and central carbon metabolism in cancer.
Figure 8. Top 20 metabolic pathway enrichment maps. (A) MPs related metabolic pathway enrichment map (groups ASW, LMPs, HMPs); (B) TCHCl related metabolic pathway enrichment map (groups ASW, LTC, HTC); (C) Metabolic pathway enrichment map of all samples (groups ASW, LTC, HTC, LMPs, LTLP, HTLP, HMPs, LTHP, HTHP); The X-axis represents the KEGG enrichment degree of the substance, the Y-axis represents the enriched KEGG pathway, and the color on the right indicates the P value (Pvalue) of the hypergeometric distribution test of KEGG enrichment.The numbers on the right side of the bar graph are the number of substances related to the pathway. ASW, artificial seawater treatment control group; LTC, low concentration tetracycline treatment group; HTC, high concentration tetracycline treatment group; LMPs, low concentration microplastics treatment group; LTLP, low concentration tetracycline combined with low concentration microplastic treatment group; HTLP, high concentration tetracycline combined with low concentration microplastic treatment group; HMPs, high concentration microplastic treatment group; LTHP, low concentration tetracycline combined with high concentration microplastic treatment group; HTHP, low concentration tetracycline combined with high concentration microplastic treatment group.
Figure 9. Top 20 metabolic network diagrams. (A) MPs related metabolic pathway enrichment map (groups ASW, LMPs, HMPs); (B) TCHCl related metabolic pathway enrichment map (groups ASW, LTC, HTC); (C) Metabolic pathway enrichment map of all samples (groups ASW, LTC, HTC, LMPs, LTLP, HTLP, HMPs, LTHP, HTHP); The blue dots represent pathways, their locations represent metabolites, and the sizes of the pathway dots represent the metabolites connected to them. The more connections there are, the larger the dots. ASW, artificial seawater treatment control group; LTC, low concentration tetracycline treatment group; HTC, high concentration tetracycline treatment group; LMPs, low concentration microplastics treatment group; LTLP, low concentration tetracycline combined with low concentration microplastic treatment group; HTLP, high concentration tetracycline combined with low concentration microplastic treatment group; HMPs, high concentration microplastic treatment group; LTHP, low concentration tetracycline combined with high concentration microplastic treatment group; HTHP, low concentration tetracycline combined with high concentration microplastic treatment group.
MPs significantly affected A. aurita cell membrane functions, particularly transmembrane transport and signal transmission. They also affected protein and purine metabolism and had a pronounced influence on certain hormones and hormone-like signal transduction molecules.
The natural concentration of microplastics in water varies, typically ranging from 0-0.1 mg/L (Koelmans et al., 2015). However, in sediment pore water, the concentration could be higher, speculated reaching as high as 162 mg/L (Besseling et al., 2014). MPs take up 3.3% weight of some sediment (Auta et al., 2017). As for tetracycline, the concentration often varies from 5 ng/L to 2500 ng/L in seawater, highest values could reach up to 12 mg/L found at sewage treatment plants (Xu et al., 2021). Tetracycline is also adsorbed on the sediment, and more is adsorbed as organic carbon increases (Xu and Li, 2010).
As a benthic organism, A. aurita polyps predominantly encounters MPs from sediments. Our experiment selected environmentally relevant concentrations to simulate and observe the actual damage caused to the polyps by the real marine ecological environment. In the short-term experiment of antioxidant activity test, we selected 10 mg/L of microplastics and 5 mg/L of tetracycline, which are relatively high environmentally relevant concentrations. The intention was to observe whether high concentration treatment in a short period of time would cause damage to a level that is difficult to recover. In the long-term experiment, we chose both environmentally relevant normal and higher concentration to mimic a wider range and observe the effects of concentration differences on the polyps. More than 150 individuals were selected for each repetition, which can better simulate the regulatory substances of the group. The experiment showed that even at a smaller concentration, microplastics would affect the chemicals related to the regulation of the life history of A. aurita. But we still cannot draw a conclusion on it, and describe microplastics as to promote or inhibit their strobilation.
After the combination of microplastics and tetracycline, different patterns appeared in the metabolites than those of single pollutants. In natural habitats, benthic organisms will be exposed to much more pollutants. And the related impacts would be stronger. A. aurita has strong vitality. In our preliminary experiments, the polyps could survive for more than a month without feeding in a tetracycline concentration far exceeding the environmental concentration (100 mg/L). However, the results of metabolomics and antioxidant activity experiments showed that A. aurita were still affected internally. Benthic organisms those are not as adaptable and resilient as A. aurita would suffer greater damage, which undoubtedly makes us pay more attention to the problem of marine pollution.
A. aurita polyps were relatively small in size, and for many organisms, the smaller the particle size of microplastics, the greater the ecological toxicity. Based on the size of polyp and the influence of particle size on biological organisms (Zhao et al., 2021; Wu et al., 2024), particles with smaller size, specifically with a diameter of 80 nm, were chosen to better assess their impact. A previous study shown the ecotoxicology of MPs on the ephyrae of A. aurita was not significantly affected by size. However, due to different life history stages, we believe that the effects in polyps need to be re-evaluated.
As persistent pollutants, microplastics and tetracyclines would have a profound impact on the long-term sustainability of ecosystems, and a large part of microplastics would be deposited on the seabed with ocean currents. A. aurita is particularly suitable as an indicator species for water quality and environmental pollution, especially the polyp stage as a model for benthic organisms for strong vitality and wide distribution. At the same time, jellyfish are an important link in the marine food chain. Therefore, by studying the performance of A. aurita in microplastic and tetracycline polluted environments, we can reflect the impact of these pollutants on the health of marine ecosystem.
The in situ cell apoptosis experiment showed a significant increase in apoptosis rates after MPs were combined with tetracycline, with no notable difference observed prior to compounding. TUNEL imaging revealed that most apoptotic sites were concentrated on the lower contact surface (Supplementary Figure S1), suggesting that exposure to complex pollutants enabled these substances to penetrate the epidermal cells of A. aurita, leading to localized cell apoptosis (Kyrylkova et al., 2012). In the experiment, the lack of feeding may also be the reason why the oral area and tentacles were not severely damaged. Research on marine medaka Oryzias melastigma has revealed that tetracycline-MP combinations have a greater effect on the gut microbial community than on the gill microbiota (Liao et al., 2023). This may indicate that different parts of the organism have different tolerance to microplastics and tetracycline. The TUNEL experiment used 12 individuals, so there may be certain errors. The interaction between microplastics and tetracycline is still unclear, and it is difficult to directly show that microplastics act as carriers to carry tetracycline into the polyp of the A. aurita and cause damage. Possible reasons also include microplastics damaging the surface on the polyp, and therefore allowed high concentrations of tetracycline invaded into the polyp body.
Measurements of antioxidant molecules indicated that the effects of tetracycline on other indicators did not consistently demonstrate increased damage. This experiment utilized various oxidative metabolism-related enzymes and marker molecules to assess the damage caused by MPs and MP-tetracycline combinations in A. aurita. In the microplastic treatment group, the total oxidative capacity initially increased shortly after exposure, followed by a sharp decline, with no significant recovery observed after the recovery phase, suggesting irreversible oxidative damage in the short term (Guan et al., 2005). Enzymes such as SOD, GSH-Px, and CAT exhibited similar patterns after exposure. After the water change, stress secretion remained briefly elevated, with the enzyme activity rising before gradually stabilizing to a steady state. Activity levels per milligram of protein were higher than those in the control group. After the water change, the body could continue to secrete certain anti-oxidative enzymes. However, reduced glutathione levels exhibited no recovery trend and continued to decrease after recovery. Malondialdehyde (MDA) levels rose after initial stimulation, briefly decreased, then increased again following the water change. MDA, often analyzed alongside SOD, serves as an indicator of cellular damage from free radicals (Del Rio et al., 2005), whereas SOD activity reflects the body’s ability to scavenge oxygen free radicals (Ifeanyi, 2018). The persistently high levels of MDA and declining GSH suggest ongoing free radical damage even after recovery, indicating that the body maintained some secretion under sustained stress. The total antioxidant capacity encompasses both enzymatic and non-enzymatic antioxidants (Bartosz, 2003). The total oxidative capacity and enzymes such as SOD, GSH, GSH-Px, and CAT collectively exhibited a decrease in total oxidative capacity, whereas some antioxidative enzymes sustained their activity. Experimental results indicate that, in the body of the Aurelia polyp, small molecule non-enzymatic antioxidant active substances are depleted, leading to a significant reduction in total antioxidant capacity. Simultaneously, while the activity of certain antioxidant enzymes has shown some recovery, the total antioxidant capacity remained reduced. This suggests that the antioxidant enzymes that retain some activities following water change may not be adequately targeting the oxidative damage the organism was experiencing. Thus, the body’s mechanism for coping with oxidative stress appeared insufficient to fully mitigate the substantial oxidative damage caused by microplastics. The decline in total antioxidant capacity, coupled with the incomplete recovery of certain antioxidant enzyme activities, points to a prolonged state of stress, suggesting the presence of additional oxidative stress factors within the organism.
Under the combined stress from MPs and tetracycline, the total antioxidant capacity of A. aurita polyps suffered damage that was difficult to recover within a short period, with most indicators demonstrating higher activity levels than those in the MP-only group, except for CAT. This suggests that tetracycline combined treatment may cause more peroxide damage to the body. The accumulation of MDA indicated ongoing oxidative damage. Although the SOD and CAT levels did not return to baseline after the water change, the MDA index remained low, suggesting that while oxidative damage had not reached a critical level, the polyp oxidative defense, particularly in scavenging oxygen free radicals, was impaired. Compared to MP-only treatment, MDA levels were lower in the co-stress group, the total antioxidant capacity of the body was alleviated, indicating that tetracycline somewhat mitigated the oxidative damage caused by MPs. We deduced that MPs may make A. aurita more susceptible to harmful microorganisms, whereas tetracycline, an antibiotic that kills certain bacteria, reduced microbial damage post-treatment. Some studies have reported that low concentrations of tetracycline can promote fish and algae growth (Liu et al., 2022; Guan et al., 2015). Conversely, studies have reported a positive correlation between high concentrations of tetracycline exposure and MDA production in Selenastrum capricornutum and Microcystis aeruginosa (Jiang et al., 2010; Wang et al., 2019). This suggests that different organisms have different tolerance levels to environmental pollutants. The effect of tetracycline on the body is relatively complex and does not always manifest as oxidative damage or antioxidant, and we cannot definitively determine its specific role in the polyp stage of Aurelia.
Compared with the experiment of ephyrae (Costa et al., 2020), the MP concentration we used in antioxidant test was way higher than the 24-hour EC50 of ephyrae. During our experiment, the polyps still maintained a high activity to external stimuli. However, the indicators inside of the polyp did not fully recover after 288 h. In an article on Aurelia coerulea (Song et al., 2024), the tentacles of the polyps were reduced in length after exposure to microplastics, and the results also showed a decrease in T-AOC and an increase in MDA secretion levels. Even under different environmental concentrations and treatment durations, some enzyme activities still showed the same similar trends. However, further experiments are required to elucidate the specific mechanisms involved.
Long-term exposure revealed that metabolomic changes in A. aurtia caused by complex pollutants differed from those induced by single pollutants. Notably, the variations in primary metabolites included substances that influenced various life stages, primarily indomethacin and retinoic acid (RA) compounds. RA compounds, such as 9-cis-retinoic acid and RA itself, promote strobilation, whereas indomethacin, within the indole and its derivatives category, can facilitate the transition of A. aurita polyps from asexual to sexual life. In contrast, indole-3-acrylic acid (IAA) inhibits strobilation (Wang, 2017). Pollutant stimulation led to varying degrees of retinal reduction, which is a dehydrogenation product of retinol. Indomethacin, after single microplastic treatment, initially decreased and then increased in concentration, indicating a nonlinear effect of MPs. Tetracycline exposure increased 9-cis-retinoic acid levels, whereas MP exposure decreased these levels. MPs reduced both inhibitors and promoters of strobilation in A. aurita polyps, with some bioactive compounds initially decreasing and then increasing. Thus, it remains uncertain whether these effects make A. aurita polyps more or less prone to strobilation at different temperatures or environmental conditions.
As with other aquatic organisms, MPs can affect antioxidant concentrations in A. aurita polyps, likely causing ROS-related damage. When combined with tetracycline, the primary metabolites of the affected antioxidant substances can also shift, suggesting a change in the response sequence of the antioxidants.
In addition to changes in antioxidant concentrations, various amino acids in A. aurtia polyps also fluctuated, ultimately affecting protein metabolism and synthesis. The ABC transporter pathway is among the most affected pathways because MPs and composite pollutants influence multiple transmembrane transport proteins.
After long-term exposure, metabolite analysis revealed significant effects on the arachidonic acid metabolic pathway in A. aurtia (Figure 10), with multiple related pathways being regulated following stimulation. In aquatic organisms, the arachidonic acid pathway is involved in osmotic regulation and secretion of chemical defenses (Bundy, 1985; Shinzato et al., 2011). In Hydrozoa jellyfish, it influences tentacle development (Di Marzo et al., 1993), whereas the role in Aurelia remains unexplored. Considering the changes in other metabolites, it can be inferred that arachidonic acid metabolism plays a regulatory role in the oxidative damage response and stress resilience of polyps.
Figure 10. Box plot of arachidonic acid metabolism-related metabolites in the polyp of A. aurita affected by microplastics. ASW, artificial seawater treatment control group; LMPs, low concentration microplastics treatment group; HMPs, high concentration microplastic treatment group. *p<0.05; **p<0.01; ***p<0.001; ****p<0.0001.
MPs affect neurotransmitter levels in A. aurtia and reduce the concentrations of various neurotransmitters. Coupled with their effect on ABC transporters, it is hypothesized that MPs can weaken intercellular communication efficiency in A. aurtia polyps following stimulation. Studies have indicated that PS MPs can influence cells via extracellular vesicle-mediated communication (Kim et al., 2024), suggesting that A. aurita regulates intracellular and intercellular communication to mitigate MP-induced damage (Trianni et al., 2024).
A study on Daphnia demonstrated that MPs significantly affected ABC transporter activity (Ali et al., 2024). Similarly, research on human liver cells has shown that long-term PS MP exposure can inhibit ABC efflux transporter activity, suggesting a chemosensitizing effect (Chen et al., 2024). These findings indicate that MP-induced damage and impact share commonalities among different organisms.
MPs could affect the signal transduction, metabolism, and oxidative stress systems of Aurelia, with the combined pollutants leading to more severe outcomes such as apoptosis. Short-term exposure to MPs, with or without tetracycline, impeded some antioxidant indicators from returning to baseline even after water restoration. Long-term experiments revealed more intricate changes, including potential stress pathways, such as arachidonic acid metabolism. Despite A. aurita’s typically high tolerance as a benthic organism, it demonstrated notable damage from MP exposure, suggesting that other benthic organisms may experience even greater impacts from MPs and combined pollutants. Limitations in experimental equipment and funding could prevent in situ hybridization labeling of pollutants, making it challenging to visually confirm the increased pollutant entry into polyps when combined with MPs. Microplastics and tetracyclines significantly affect marine ecosystems, and studying the moon jellyfish A. aurita, especially in its polyp stage, can serve as an indicator of the impact of these pollutants on marine health. Our experimental design can be combined with phenotype-related experimental observations to fully explore the impact of experimental conditions on organisms. Future research trends will focus more on collaborative analysis between omics, promote the linkage application of multi-omics data, and strive to build complex marine ecological models that consider the interaction of multiple factors, so as to more accurately reveal the dynamic changes of marine ecosystems and their driving mechanisms.
The raw data supporting the conclusions of this article will be made available by the authors, without undue reservation.
Ethical approval was not required for the studies involving animals in accordance with the local legislation and institutional requirements because The experimental animal used is the moon jellyfish Aurelia aurita, which is an invertebrate. Written informed consent was obtained from the owners for the participation of their animals in this study.
XW: Data curation, Methodology, Software, Supervision, Visualization, Writing – original draft, Writing – review & editing. XZ: Writing – review & editing, Resources, Supervision. HL: Writing – review & editing, Formal analysis, Supervision. JG: Writing – review & editing, Formal analysis. ZM: Writing – review & editing, Resources. ZF: Funding acquisition, Methodology, Writing – review & editing, Project administration, Supervision, Resources.
The author(s) declare that financial support was received for the research and/or publication of this article. This research was supported by the Natural Science Foundation of Guangxi Province, China (2022GXNSFBA035473), Guangdong Natural Science Foundation of China (2023A1515011859), Guangxi Minzu University Research Fund (2021KJQD18), the Basic Research Fund of the Guangxi Academy of Sciences (2024YWF2112), and Opening Project of Guangxi Key Laboratory of Marine Natural Products and Combinatorial Biosynthesis Chemistry (GXMNPC2020005).
The authors sincerely thank Beihai Hongyuan breeding base for providing us Aurelia aurita polyps.
The authors declare that the research was conducted in the absence of any commercial or financial relationships that could be construed as a potential conflict of interest.
The author(s) declare that no Generative AI was used in the creation of this manuscript.
All claims expressed in this article are solely those of the authors and do not necessarily represent those of their affiliated organizations, or those of the publisher, the editors and the reviewers. Any product that may be evaluated in this article, or claim that may be made by its manufacturer, is not guaranteed or endorsed by the publisher.
The Supplementary Material for this article can be found online at: https://www.frontiersin.org/articles/10.3389/fmars.2025.1545131/full#supplementary-material
Supplementary Figure 1 | TUNEL assay results for short-term exposure. Representative images of each group are shown. ASW, artificial seawater control group; MPs, single microplastic treatment group; TC, single tetracycline treatment group; CO, combined pollutant treatment group. Scale bar: 200 μm.
Supplementary Figure 2 | Total metabolite cluster heat map. The columns represent samples, the rows represent metabolites, the cluster tree on the left is the differential metabolite cluster tree, and the top is the sample cluster tree. The gradient color represents the size of the quantitative values. The redder the color, the higher the expression; the bluer the color, the lower the expression. ASW, artificial seawater treatment control group; LTC, low concentration tetracycline treatment group; HTC, high concentration tetracycline treatment group; LMPs, low concentration microplastics treatment group; LTLP, low concentration tetracycline combined with low concentration microplastic treatment group; HTLP, high concentration tetracycline combined with low concentration microplastic treatment group; HMPs, high concentration microplastic treatment group; LTHP, low concentration tetracycline combined with high concentration microplastic treatment group; HTHP, low concentration tetracycline combined with high concentration microplastic treatment group.
Supplementary Figure 3 | Secondary metabolite correlation molecular heat map. There is a synergistic or mutually exclusive relationship between different metabolites. For example, if a certain type of metabolite has the same change trend, it means that the relative content changes of this type of metabolite are positively correlated; if the trend is opposite, it is a negative correlation. The purpose of differential metabolite correlation analysis is to check the consistency of change trends between metabolites, and to analyze the correlation between each metabolite by calculating the Pearson correlation coefficient between pairs of differential metabolites. When the linear relationship between two metabolites is enhanced, it tends to 1 when there is a positive correlation and to -1 when there is a negative correlation. At the same time, statistical test analysis was performed on the significance of metabolite correlation, and a significant P value < 0.05 was selected as a significant correlation. (A) Microplastic related; (B) Tetracycline related; (C) All samples.
Abdelrazig S., Safo L., Rance G. A., Fay M. W., Theodosiou E., Topham P. D., et al. (2020). Metabolic characterisation of Magnetospirillum gryphiswaldense MSR-1 using LC-MS-based metabolite profiling. RSC Adv. 10, 32548–32560. doi: 10.1039/D0RA05326K
Algueró-Muñiz M., Meunier C. L., Holst S., Alvarez-Fernandez S., Boersma M. (2016). Withstanding multiple stressors: ephyrae of the moon jellyfish (Aurelia aurita, Scyphozoa) in a high-temperature, high-CO2 and low-oxygen environment. Mar. Biol. 163, 186. doi: 10.1007/s00227-016-2958-z
Ali W., Jeong H., Tisné M. L., Favrelle-Huret A., Thielemans W., Zinck P., et al. (2024). The comparative toxicity of biobased, modified biobased, biodegradable, and petrochemical-based microplastics on the brackish water flea Diaphanosoma celebensis. Sci. Total Environ. 944, 173747. doi: 10.1016/j.scitotenv.2024.173747
Amato-Lourenço L. F., Carvalho-Oliveira R., Júnior G. R., dos Santos Galvão L., Ando R. A., Mauad T. (2021). Presence of airborne microplastics in human lung tissue. J. Hazardous Mater 416, 126124. doi: 10.1016/j.jhazmat.2021.126124
Andrady A. L. (2011). Microplastics in the marine environment. Mar. pollut. Bull. 62, 1596–1605. doi: 10.1016/j.marpolbul.2011.05.030
Antos J., Piosik M., Ginter-Kramarczyk D., Zembrzuska J., Kruszelnicka I. (2024). Tetracyclines contamination in European aquatic environments: A comprehensive review of occurrence, fate, and removal techniques. Chemosphere 353, 141519. doi: 10.1016/j.chemosphere.2024.141519
Auta H. S., Emenike C. U., Fauziah S. H. (2017). Distribution and importance of microplastics in the marine environment: a review of the sources, fate, effects, and potential solutions. Environ. Int. 102, 165–176. doi: 10.1016/j.envint.2017.02.013
Barnard L., Gent R., Van Rooyen D., Swart A. C. (2017). Adrenal C11-oxy C21 steroids contribute to the C11-oxy C19 steroid pool via the backdoor pathway in the biosynthesis and metabolism of 21-deoxycortisol and 21-deoxycortisone. J. Steroid Biochem. Mol. Biol. 174, 86–95. doi: 10.1016/j.jsbmb.2017.07.034
Bartosz G. (2003). Total antioxidant capacity. Adv. Clin. Chem. 37, 219–292. doi: 10.1016/S0065-2423(03)37010-6
Baxter E. J., Sturt M. M., Ruane N. M., Doyle T. K., Mcallen R., Harman L., et al. (2011). Gill damage to Atlantic salmon (Salmo salar) caused by the common jellyfish (Aurelia aurita) under experimental challenge. PloS One 6, e18529. doi: 10.1371/journal.pone.0018529
Besseling E., Wang B., Lurling M., Koelmans A. A. (2014). Nanoplastic affects growth of S. obliquus and reproduction of D. magna. Environ. Sci. Technol. 48, 12336–12343. doi: 10.1021/es503001d
Bour A., Avio C. G., Gorbi S., Regoli F., Hylland K. (2018). Presence of microplastics in benthic and epibenthic organisms: Influence of habitat, feeding mode and trophic level. Environ. pollut. 243, 1217–1225. doi: 10.1016/j.envpol.2018.09.115
Bundy G. (1985). Nonmammalian sources of eicosanoids. Adv. Prostaglandin Thromboxane Leukotriene Res. 14, 229–262.
Chen X.-T. (2021). Establishment of 60Co-γ-ray Radiation Model of Aurita coerulea Polyps and Screening of Related Genes. Master degree (China: The Second Military Medical University).
Chen Z., Li Y., Xia H., Wang Y., Pang S., Ma C., et al. (2024). Chronic exposure to polystyrene microplastics increased the chemosensitivity of normal human liver cells via ABC transporter inhibition. Sci. Total Environ. 912, 169050. doi: 10.1016/j.ecoenv.2019.109983
Costa E., Gambardella C., Piazza V., Vassalli M., Sbrana F., Lavorano S., et al. (2020). Microplastics ingestion in the ephyra stage of Aurelia sp. triggers acute and behavioral responses. Ecotoxicol Environ. Saf. 189, 109983. doi: 10.1016/j.ecoenv.2019.109983
Da Costa J. P., Avellan A., Mouneyrac C., Duarte A., Rocha-Santos T. (2023). Plastic additives and microplastics as emerging contaminants: Mechanisms and analytical assessment. TrAC Trends Analytical Chem. 158, 116898. doi: 10.1016/j.trac.2022.116898
Del Rio D., Stewart A. J., Pellegrini N. (2005). A review of recent studies on malondialdehyde as toxic molecule and biological marker of oxidative stress. Nutrition Metab. Cardiovasc. Dis. 15, 316–328. doi: 10.1016/j.numecd.2005.05.003
Di Marzo V., De Petrocellis L., Gianfrani C., Cimino G. (1993). Biosynthesis, structure and biological activity of hydroxyeicosatetraenoic acids in Hydra vulgaris. Biochem. J. 295, 23–29. doi: 10.1042/bj2950023
Dong Z. (2019). Blooms of the moon jellyfish Aurelia: causes, consequences and controls. World Seas: An Environmental Evaluation (Second Edition) Volume III: Ecological Issues and Environmental Impacts 2019, 163–171. doi: 10.1016/B978-0-12-805052-1.00008-5
Ds W. (2007). HMDB: the human metabolome database. Nucleic Acids Res. 35, D521–D526. doi: 10.1093/nar/gkl923
Echols B., Smith A., Gardinali P., Rand G. (2016). The use of ephyrae of a scyphozoan jellyfish, Aurelia aurita, in the aquatic toxicological assessment of Macondo oils from the Deepwater Horizon incident. Chemosphere 144, 1893–1900. doi: 10.1016/j.chemosphere.2015.10.082
Engler R. E. (2012). The complex interaction between marine debris and toxic chemicals in the ocean. Environ. Sci. Technol. 46, 12302–12315. doi: 10.1021/es3027105
Feng S., Lin J., Sun S., Zhang F. (2017). Artificial substrates preference for proliferation and immigration in Aurelia aurita (sl) polyps. Chin. J. Oceanol Limnol 35, 153–162. doi: 10.1007/s00343-016-5230-y
Godoy V., Blázquez G., Calero M., Quesada L., Martín-Lara M. (2019). The potential of microplastics as carriers of metals. Environ. pollut. 255, 113363. doi: 10.1016/j.envpol.2019.113363
Goldstein M. C., Carson H. S., Eriksen M. (2014). Relationship of diversity and habitat area in North Pacific plastic-associated rafting communities. Mar. Biol. 161, 1441–1453. doi: 10.1007/s00227-014-2432-8
Guan Y.-H., Li H.-W., Lv M. (2015). Effects of norfloxacin and tetracycline on microcystis aeruginosa. SiChuan Enviroment 34, 25–30. doi: 10.14034/j.cnki.schj.2015.05.005
Guan C., Zhang Y., Yao Z., Li R., Yu P. (2005). Association of general level of total anti-oxidation capacity, superoxide dismutase, nitric oxide synthase activity in serum with the lifestyle of residents of Bin County of Heilongjiang Province. Chin. J. Tissue Eng. Res., 198–201.
Hale R. C., Seeley M. E., La Guardia M. J., Mai L., Zeng E. Y. (2020). A global perspective on microplastics. J. Geophysical Rese: Oceans 125, e2018JC014719. doi: 10.1029/2018JC014719
Horai H., Arita M., Kanaya S., Nihei Y., Ikeda T., Suwa K., et al. (2010). MassBank: a public repository for sharing mass spectral data for life sciences. J. Mass Spectrometry 45, 703–714. doi: 10.1002/jms.1777
Ifeanyi O. E. (2018). A review on free radicals and antioxidants. Int. J. Curr. Res. Med. Sci. 4, 123–133. doi: 10.22192/ijcrms.2018.04.02.019
Jiang L., Shu-Yi C., Yin D.-Q. (2010). Effects of tetracycline on photosynthesis and antioxidant enzymes of Microcystis aeruginosa. J. Ecol. Rural Environ. 26, 564–567. doi: 10.3969/j.issn.1673-4831.2010.06.010
Jian-Yan W., Yu Z., Guo-Shan W., Tie-Zhu M., Zhi-Gang Y. (2013). Molecular identification and detection of moon jellyfish (Aurelia sp.) based on partial sequencing of mitochondrial 16S rDNA and COI. Chin. J. Appl. Ecol. 24, 847–852. doi: 10.13287/j.1001-9332.2013.0239
Kahane-Rapport S., Czapanskiy M., Fahlbusch J., Friedlaender A., Calambokidis J., Hazen E., et al. (2022). Field measurements reveal exposure risk to microplastic ingestion by filter-feeding megafauna. Nat. Commun. 13, 6327. doi: 10.1038/s41467-022-33334-5
Kim N., Park J. H., Lee I., Jung G. S., Lee J. H., Lee M. J., et al. (2024). Investigation of cell-to-cell transfer of polystyrene microplastics through extracellular vesicle-mediated communication. Biochem. Biophys. Res. Commun. 734, 150719. doi: 10.1016/j.bbrc.2024.150719
Koelmans A. A., Besseling E., Shim W. J. (2015). Nanoplastics in the aquatic environment. Critical review. Mar. Anthropogenic Litter, 325–340. doi: 10.1007/978-3-319-16510-3_12
Kyrylkova K., Kyryachenko S., Leid M., Kioussi C. (2012). Detection of apoptosis by TUNEL assay. Odontogenesis: Methods Protoc. 887, 41–47. doi: 10.1007/978-1-61779-860-3_5
Law K. L., Morét-Ferguson S., Maximenko N. A., Proskurowski G., Peacock E. E., Hafner J., et al. (2010). Plastic accumulation in the North Atlantic subtropical gyre. Science 329, 1185–1188. doi: 10.1126/science.119232
Liao X., Zhao P., Hou L., Adyari B., Xu E. G., Huang Q., et al. (2023). Network analysis reveals significant joint effects of microplastics and tetracycline on the gut than the gill microbiome of marine medaka. J. Hazardous Mater 442, 129996. doi: 10.1016/j.jhazmat.2022.129996
Liu S., Wang C., Yang Y., Du Z., Li L., Zhang M., et al. (2024). Microplastics in three types of human arteries detected by pyrolysis-gas chromatography/mass spectrometry (Py-GC/MS). J. Hazardous Mater 469, 133855. doi: 10.1016/j.jhazmat.2024.133855
Liu G., Xu Y., Wei X., Zhong C., Liu X., Luo Z. (2022). Long-term environmental-related tetracycline exposure on growth performance, hepatic lipid metabolism and antioxidant responses in gift tilapia (Oreochromis niloticus). Acta Hydrobiol Sin. 46, 1642–1648. doi: 10.7541/2022.2021.0251
Lucas C. H. (2001). Reproduction and life history strategies of the common jellyfish, Aurelia aurita, in relation to its ambient environment (Netherlands: Springer), 229–246. doi: 10.1007/978-94-010-0722-1_19
Lundström S. V., Östman M., Bengtsson-Palme J., Rutgersson C., Thoudal M., Sircar T., et al. (2016). Minimal selective concentrations of tetracycline in complex aquatic bacterial biofilms. Sci. Total Environ. 553, 587–595. doi: 10.1016/j.scitotenv.2016.02.103
Mai N. T. Q., Batjargal U., Kim W.-S., Kim J.-H., Park J.-W., Kwak I.-S., et al. (2023). Microplastic induces mitochondrial pathway mediated cellular apoptosis in mussel (Mytilus galloprovincialis) via inhibition of the AKT and ERK signaling pathway. Cell Death Discovery 9, 442. doi: 10.1038/s41420-023-01740-3
Navarro-Reig M., Jaumot J., García-Reiriz A., Tauler R. (2015). Evaluation of changes induced in rice metabolome by Cd and Cu exposure using LC-MS with XCMS and MCR-ALS data analysis strategies. Analytical Bioanalytical Chem. 407, 8835–8847. doi: 10.1007/s00216-015-9042-2
Ogata H., Goto S., Sato K., Fujibuchi W., Bono H., Kanehisa M. (1999). KEGG: Kyoto encyclopedia of genes and genomes. Nucleic Acids Res. 27, 29–34. doi: 10.1093/nar/27.1.29
Ragusa A., Svelato A., Santacroce C., Catalano P., Notarstefano V., Carnevali O., et al. (2021). Plasticenta: First evidence of microplastics in human placenta. Environ. Int. 146, 106274. doi: 10.1016/j.envint.2020.106274
Rasmussen J. A., Villumsen K. R., Ernst M., Hansen M., Forberg T., Gopalakrishnan S., et al. (2022). A multi-omics approach unravels metagenomic and metabolic alterations of a probiotic and synbiotic additive in rainbow trout (Oncorhynchus mykiss). Microbiome 10, 21. doi: 10.1186/s40168-021-01221-8
Romero-Kutzner V., Tarí J., Herrera A., Martínez I., Bondyale-Juez D., Gómez M. (2022). Ingestion of polyethylene microspheres occur only in presence of prey in the jellyfish Aurelia aurita. Mar. pollut. Bull. 175, 113269. doi: 10.1016/j.marpolbul.2021.113269
Sharma M. D., Elanjickal A. I., Mankar J. S., Krupadam R. J. (2020). Assessment of cancer risk of microplastics enriched with polycyclic aromatic hydrocarbons. J. Hazardous Mater 398, 122994. doi: 10.1016/j.jhazmat.2020.122994
Shinzato C., Shoguchi E., Kawashima T., Hamada M., Hisata K., Tanaka M., et al. (2011). Using the Acropora digitifera genome to understand coral responses to environmental change. Nature 476, 320–323. doi: 10.1038/nature10249
Song M., Zhu Y., Wei Z., Li X., Chen X., Liu X., et al. (2024). Oxidative damage induced by ps-nps in aurelia aurita polyps and bv2 cells through the mapk pathway. SSRN. doi: 10.2139/ssrn.4954480
Spangenberg D., Beck C. (1972). Tetracycline effects on statolith and nematocyst differentiation in Aurelia. Calcified Tissue Res. 9, 122–130. doi: 10.1007/BF02061950
Sucharitakul P., Pitt K. A., Welsh D. T. (2020). Limited ingestion, rapid egestion and no detectable impacts of microbeads on the moon jellyfish, Aurelia aurita. Mar. pollut. Bull. 156, 111208. doi: 10.1016/j.marpolbul.2020.111208
Sud M., Fahy E., Cotter D., Brown A., Dennis E. A., Glass C. K., et al. (2007). Lmsd: Lipid maps structure database. Nucleic Acids Res. 35, D527–D532. doi: 10.1093/nar/gkl838
Takao M., Okawachi H., Uye S.-I. (2014). Natural predators of polyps of Aurelia aurita sl (Cnidaria: Scyphozoa: Semaeostomeae) and their predation rates. Plankton Benthos Res. 9, 105–113. doi: 10.3800/pbr.9.105
Talang R. P. N., Polruang S., Sirivithayapakorn S. (2024). Influencing factors of microplastic generation and microplastic contamination in urban freshwater. Heliyon 10. doi: 10.1016/j.heliyon.2024.e30021
Thévenot E. A., Roux A., Xu Y., Ezan E., Junot C. (2015). Analysis of the human adult urinary metabolome variations with age, body mass index, and gender by implementing a comprehensive workflow for univariate and OPLS statistical analyses. J. Proteome Res. 14, 3322–3335. doi: 10.1021/acs.jproteome.5b00354
Tong M., Li X., Luo Q., Yang C., Lou W., Liu H., et al. (2020). Effects of humic acids on biotoxicity of tetracycline to microalgae Coelastrella sp. Algal Res. 50, 101962. doi: 10.1016/j.algal.2020.101962
Trianni A., Brossa A., Catalano F., Saraceni A., Bongiovanni S., Merlo G., et al. (2024). Unraveling the molecular mechanisms of microplastics internalization and their intracellular impact. Eur. J. Histochem. 68, 47–48. doi: 10.4081/ejh.2024.4071
Wang W. (2017). Regulation of metamorphosis and the evolution of life cycles: insights from the common moon jelly Aurelia aurita: Universitätsbibliothek Kiel (Germany: Christian-Albrechts-Universität zu Kiel / Kiel University).
Wang Y., Wu P., Shen H. (2019). Effects of tetracycline on growth and antioxidant system of Selenastrum capricornutum. J. Hebei Univ. Sci. Technol. 40 (6), 548–554. doi: 10.7535/hbkd.2019yx06013
Want E. J., Masson P., Michopoulos F., Wilson I. D., Theodoridis G., Plumb R. S., et al. (2013). Global metabolic profiling of animal and human tissues via UPLC-MS. Nat. Protoc. 8, 17–32. doi: 10.1038/nprot.2012.135
Warren C. R., O’sullivan J. F., Friesen M., Becker C. E., Zhang X., Liu P., et al. (2017). Induced pluripotent stem cell differentiation enables functional validation of GWAS variants in metabolic disease. Cell Stem Cell 20, 547–557, e547. doi: 10.1016/j.stem.2017.01.010
Wu D., Lu X., Dong L.-X., Tian J., Deng J., Wei L., et al. (2024). Nano polystyrene microplastics could accumulate in Nile tilapia (Oreochromis niloticus): Negatively impacts on the intestinal and liver health through water exposure. J. Environ. Sci. 137, 604–614. doi: 10.1016/j.jes.2023.02.018
Xia J., Wishart D. S. (2011). Web-based inference of biological patterns, functions and pathways from metabolomic data using MetaboAnalyst. Nat. Protoc. 6, 743–760. doi: 10.1038/nprot.2011.319
Xu X.-R., Li X.-Y. (2010). Sorption and desorption of antibiotic tetracycline on marine sediments. Chemosphere 78, 430–436. doi: 10.1016/j.chemosphere.2009.10.045
Xu L., Zhang H., Xiong P., Zhu Q., Liao C., Jiang G. (2021). Occurrence, fate, and risk assessment of typical tetracycline antibiotics in the aquatic environment: A review. Sci. Total Environ. 753, 141975. doi: 10.1016/j.scitotenv.2020.141975
Yang B., Huang H. (2019). Effect of microplastics on antioxidant enzyme system in juvenile red crucian carp. Environ. Sci. Technol. 42, 23–27. doi: 10.19672/j.cnki.1003-6504.2019.12.004
Zelena E., Dunn W. B., Broadhurst D., Francis-Mcintyre S., Carroll K. M., Begley P., et al. (2009). Development of a robust and repeatable UPLC– MS method for the long-term metabolomic study of human serum. Analytical Chem. 81, 1357–1364. doi: 10.1021/ac8019366
Zhao J., Rao B.-Q., Guo X.-M., Gao J.-Y. (2021). Effects of microplastics on embryo hatching and intestinal accumulation in larval zebrafish Danio rerio. Environ. Sci. 42, 485–491. doi: 10.13227/j.hjkx.202003199
Keywords: Aurelia aurita, microplastics, tetracycline, TUNEL, oxidative damage, metabolome
Citation: Wu X, Zhang X, Liao H, Guo J, Ma Z and Fu Z (2025) Microplastics and tetracycline affecting apoptosis, enzyme activities and metabolism processes in the Aurelia aurita polyps: insights into combined pollutant effects. Front. Mar. Sci. 12:1545131. doi: 10.3389/fmars.2025.1545131
Received: 14 December 2024; Accepted: 26 February 2025;
Published: 14 March 2025.
Edited by:
Carlos Gravato, University of Lisbon, PortugalReviewed by:
Sedat Gundogdu, Çukurova University, TürkiyeCopyright © 2025 Wu, Zhang, Liao, Guo, Ma and Fu. This is an open-access article distributed under the terms of the Creative Commons Attribution License (CC BY). The use, distribution or reproduction in other forums is permitted, provided the original author(s) and the copyright owner(s) are credited and that the original publication in this journal is cited, in accordance with accepted academic practice. No use, distribution or reproduction is permitted which does not comply with these terms.
*Correspondence: Zhilu Fu, ZnV6aGlsdUBneG16dS5lZHUuY24=
Disclaimer: All claims expressed in this article are solely those of the authors and do not necessarily represent those of their affiliated organizations, or those of the publisher, the editors and the reviewers. Any product that may be evaluated in this article or claim that may be made by its manufacturer is not guaranteed or endorsed by the publisher.
Research integrity at Frontiers
Learn more about the work of our research integrity team to safeguard the quality of each article we publish.