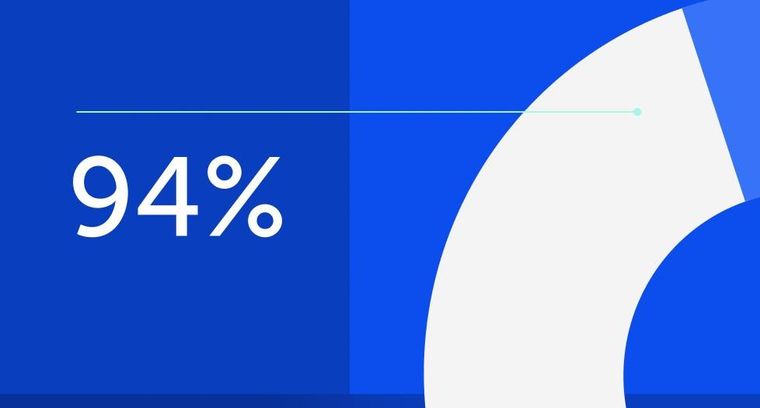
94% of researchers rate our articles as excellent or good
Learn more about the work of our research integrity team to safeguard the quality of each article we publish.
Find out more
ORIGINAL RESEARCH article
Front. Mar. Sci., 25 March 2025
Sec. Marine Biotechnology and Bioproducts
Volume 12 - 2025 | https://doi.org/10.3389/fmars.2025.1544737
This study investigated the biodiversity of macroalgae along the southern Atlantic coast of Morocco and explored the relationship between their distribution and the physicochemical properties of seawater. The study identified a total of 21 macroalgal species, classified into 19 genera, 14 families, and 9 orders. The distribution of these species along the coast was analyzed, revealing 8 dominant species that collectively made up approximately 64.7% of the macroalgal flora. These dominant species belonged predominantly to the Florideophyceae (75%) and Phaeophyceae (25%) families. Gracilariaceae and Rhodomelaceae families exhibited high species diversity, accounting for 28.57% of all observed species. The equitability index indicated variations in species distribution and dominance among different sites. Chemical characterization indicated that the concentrations of available phosphorus in the seawater ranged from 0.2 to 0.6 mg/L, while the chlorophyll-a content in the macroalgae varied between 1.2 and 2.8 mg/g. Additionally, the total phenol content in the algae ranged from 2 to 5 mg/g. Gas chromatography-mass spectrometry (GC-MS) analysis of macroalgae revealed the presence of diverse compounds such as fatty acid, phytyl-eicosanoate, and sterols. These findings highlighted the interactions between the marine environment’s characteristics and algal diversity, while exploring some key parameters of algal growth and composition. The obtained results could help the scientific community to identify several strategies for both algal biodiversity and biorefinery.
Marine algae are a diverse and significant group within aquatic ecosystems, playing vital ecological roles. With an estimated 25,000-50,000 species, including approximately 12,000 macroalgae or seaweeds, they exhibit a wide range of sizes, forms, pigments, and functional compounds (Kulshreshtha et al., 2020). Marine algae contribute extensively to various biological systems, such as nutrient circulation, primary production, sediment stabilization, and atmospheric oxygen supply. Their ecological significance has been widely recognized (Kumar et al., 2016; Pereira, 2016). The global annual harvest of macroalgae surpasses 36 million metric tons, with a market value of approximately $6 billion USD for several commercial applications (Kulshreshtha et al., 2020).
The Moroccan coast boasts a rich diversity of marine algae, with more than 500 species, including green, brown, and red algae (Grina et al., 2020). Among these, red algae, specifically Gelidium sesquipedale, are the main species exploited for economic purposes in Morocco. The favorable salinity and hydro-climatic conditions along the Moroccan coast have led to the development of a seaweed valorization industry since the 1950s (Ouahid et al., 2021). This sector employs over 500 people full-time, provides seasonal employment for 9,000 individuals, and generates a turnover of nearly 170 million MAD (Ouahid et al., 2021).
Seaweed harvesting is driven by the high demand from foreign buyers who procure it at a price of 15 MAD/Kg, significantly higher than the local processing industries’ offering of 8 MAD/Kg (Ouahid et al., 2021). Gelidium sesquipedale, a red seaweed abundant along the Moroccan Atlantic coast, is considered an excellent raw material for agar-agar extraction. Other species, such as Gigartina, Pterocladia, and Gracilaria, also contribute to the local seaweed industry (Cai and Y., 2021).
Algae producers selectively cultivate specific strains to harness valuable compounds found in algal biomass. These compounds include lipids for biofuel production, proteins for food, feed, and nutraceuticals, and starches and carbohydrates with diverse applications (Connelly et al., 2015). The valorization of algal biomass represents an intriguing international program in marine resource exploitation, with significant economic potential. Seaweeds have already gained recognition for their commercial value in various sectors, including food industry, agriculture, pharmaceuticals, and biotechnology (Armeli Minicante et al., 2022; Cai and Y., 2021).
There are several economic fields that use algae. They are currently a nutritional source and a product of increasing value, especially in Asia where they are used directly as food, or indirectly, especially by the phycocolloids industry (agars and alginates) (Msuya et al., 2022). They are used in agriculture as fertilizer, in the food and pharmaceutical industries, in textiles, and in many other fields (Cai and Y., 2021).
The southern Atlantic coast of Morocco presents unique environmental conditions, such as salinity, water temperature, marine currents, and sunlight, which can influence the diversity of macroalgae species in the region (Bahammou et al., 2021; Derhy et al., 2022). However, the current state of knowledge regarding the occurrence and distribution of seaweed along the south Atlantic coast is very limited. The scarcity of information hinders a comprehensive understanding of the region’s seaweed richness. The objective of this study is to investigate, for the first time, the biodiversity of macroalgae along the Moroccan Atlantic coast, specifically focusing on the southern region. By examining the physicochemical characteristics of seawater and their relationship to algal biodiversity parameters, such as species richness, abundance, and distribution, we aim to gain insights into the state of macroalgal diversity in this area. Consequently, the outcomes of the present study assume great significance as they serve to bridge this knowledge gap. By shedding light on the seaweed’s presence and spatial distribution, the study significantly contributes to advancing our comprehension of the seaweed diversity encompassing the south Atlantic coast.
To conduct a comprehensive assessment of the coastal environment, a systematic sampling approach was employed to collect seawater, sediment, and seaweed samples during optimal exploration periods in 2022. A total of 19 selected sites (S1, S2, S3, …., S19) from Tarfaya to Laayoune city, Morocco, were visited, ensuring an extensive coverage of the coastal surface for thorough investigation.
The collected samples were stored at 4°C during the transportation and all valuable information in each sampling point were recorded (GPS location, presence/absence of seaweed, habitat description, identification of seaweed species, level of attachment to the rock, …etc.). Based on recorded coordinates (latitude, longitude), geographical mapping was conducted in a satellite image as showed in the Figure 1.
The pH and EC were measured using an aqueous extract of the seaweed at room temperature (1 g/10 mL of distilled water) after 30 min of agitation and directly in 10 mL of seawater.
Total organic carbon (TOC) was determined after oxidation using dichromate of potassium according to the Anne’s method as described by (Aubert, 1978).
Available phosphorus was performed using Olsen method. The extraction was done with a solution of sodium bicarbonate (NaHCO3). After agitation and filtration, the filtrate was determined by a spectrophotometer at 840 nm.
COD was determined by the Standard Methods for the Examination of Water and Wastewater (APHA, 1998). The appropriate amount of water samples was diluted, and two milliliters of diluted sample was introduced into a lab-prepared digestion solution containing sulfuric acid. potassium dichromate and mercuric sulfate. The mixture was then incubated for 120 min at 150°C in a COD reactor and the COD concentration was measured calorimetrically at 600 nm.
The flame photometer was calibrated using the standard stock solution of sodium, potassium, and calcium using 30 ppm concentration. 100 ml of water from each collected sample was used to perform analysis using the flame photometer (Banerjee and Prasad, 2020).
Sample identification was conducted based on the morphological characters such as the color (pigment), the nature of the thallus, the shape, and the amplitude of the ramification of the thallus. In this work. the identification was based on the observation of morphological characters by comparing our data to some previous works (Gueye et al., 2019; Yang et al., 2021). The seaweed samples were carefully examined using a digital microscope. By leveraging these comparative analyses, we aimed to classify the seaweed samples based on their morphological attributes.
The study of the spatial dynamic and biodiversity of the seaweed species, several indicators were calculated (specific richness, Abundance, Shannon and equitability indices, the similarity coefficient, and Sørensen’s β index).
The species richness (S) is the total number of species present on a study site at a given time (Dajoz, 1985).
The Shannon index (also called the Shannon-Wiener index) is used to express diversity by considering the number of species and the abundance of individuals within each of these species (Coppejans et al., 2010).
Generally, Shannon diversity indices range from 0 to 4.5 (Ortiz-Burgos, 2016).
Where:
■ pi = the proportional abundance or percent abundance of a species present (pi = ni/N).
■ ni = the number of individuals of one species in the sample.
■ N = the total number of individuals of all species in the sample.
■ S = the total or cardinal number of the list of species present.
The base of the logarithm used is base 2.
Diversity is low when H< 3 bits. medium if H is between 3 and 4 bits. and then high when H ≥ 4 bits. The environment is low in diversity when H is low and relatively diverse in species when H is high (Djego et al., 2012).
To better discuss the Shannon index, it is often accompanied by Piélou’s equitability index (J) or equirrepartition index (E). This index measures the distribution of individuals within species, independently of the specific richness (Wang et al., 2016). These values vary between 0 and 1. A high value of this index indicates that the population is homogeneous, or that the individuals are equitably distributed between the various species. On the other hand. when its value is low (i.e. close to 0), the stand is considered to be dominated by one or a few species. The measure of equitability corresponds to the Shannon-Weaver index or real diversity on the value of the maximum theoretical diversity (Hmax) and is realized according to the following formula:
■
■
■
Equitability is low when E< 0.6; medium when E is between 0.6 and 0.8 and high if E ≥ 0.8. If H and E are low. then the medium is homogeneous and specialized. If H and E are high. then the medium is isotropic (Djego et al., 2012).
The β index measures the similarity in species between two habitats. The index ranges from 0 when there are no common species between two habitats (Rombouts et al., 2019). Sørensen’s index gives significant weight to the presence of a species over its absence. Sørensen’s index is given by the following formula:
where:
■ a: represents the number of common species between two habitats.
■ S1: represents the number of species for habitat 1.
■ S2: represents the number of species for habitat 2.
The dried macroalgae material was grounded to powder using a laboratory mechanical grinder. The different materials were extracted using n-hexane in a Soxhlet apparatus, then absolute ethanol was used for 48h to form ethanolic extracts. After complete extraction, each algae residue was reextracted by distillated water using ultrasound assisted extraction (UAS) to form an aqueous extract. Dried extracts were stored in glass vials at 4 C until use.
500 mg of seaweed was kept in a pestle and mortar with 10 ml of 80% acetone and it was ground well, and the homogenate was centrifuged at 3000 rpm for 15 minutes and the supernatant was stored (Adhoni et al., 2016; Mohy El-Din and El-Ahwany, 2016). The pellet was re-extracted by repeated washing with 5 ml of 80% acetone till it became colorless. All the extracts were pooled and utilized for pigment quantification. The process was followed for all the samples.
The amount of chlorophyll present in the algae was estimated by the method of Arnon (1949). Absorbance was measured at 645 nm and 663 nm in a spectrophotometer. The chlorophyll content was determined by using the following formula Arnon’s (1949) equations:
■
■
■
Where, A = Absorbance at respective wavelength = Volume of extract (mL). W = Fresh weight of the sample (g).
The amount of Carotenoid was estimated by the method of Kirk and Allen (1965).The same chlorophyll extract was measured at 480 nm in spectrophotometer to estimate the carotenoid content.
■
Where, A = Absorbance at respective wave length (Vimala and Poonghuzhali, 2015).
The total sugar was determined by the phenol-sulfuric acid method with some modifications (Dubois et al., 1956). The color reaction was initiated by mixing 1 mL of ethanolic extract with 0.5 mL of a 5% phenol solution and 2.5 mL of concentrated sulfuric acid, and the reaction mixture was incubated in a boiling water bath for 15 min. After cooling to room temperature, the absorbance was determined at 490 nm and the total carbohydrate content was calculated with D-glucose as a standard. The proteins are assessed by the Bradford method at 595 nm (Bradford, 1976). Bovine serum albumin (BSA) was used as a reference protein to establish the standard curve. The mixture consisted of 200 µL of Bio-Rad reagent and 800 µL of 96% of ethanolic extract of each sample. The optical density reading was carried out via spectrophotometer at 595 nm after 10 minutes against a control without protein.
The total phenol content (TPC) was determined spectrophotometrically using Folin-Ciocalteu reagent as described by Matanjun et al. (2008). The results were expressed as mg Gallic acid/g of dry matter (DM).
The flavonoid content was assessed following the method of (Woisky and Salatino, 1998) with slight modifications. The absorbance of the mixture was taken at 415 nm. Quercetin was used as standard. The flavonoid content was expressed as mg of quercetin equivalence (QE)/g of DM.
The GC–MS analyses were carried out using GC Shimadzu, Nexis 2030 instrument attached with TQ8040 NX, equipped with a RTx-5MS capillary column (30 m × 0.25 mm i.d., 0.25 μm film thickness). The chromatographic conditions were as follows: initial temperature: 150°C for 2 min; final temperature: 320°C for 10 min; injector temperature: 150°C; split ratio: 1:00. The MS was operated in the electron impact mode with an electron impact energy of 70 eV and data collected at a rate of full scan over a range of m/z of 50–500. The ion source was maintained at 200°C. Compounds were identified by comparing their mass spectra with the equipment mass spectral library (Wiley-NIST Mass Spectral Library).
The 2,2-diphenyl-1-picrylhydrazyl radical scavenging activity was analyzed using the method described by (Ben Bakrim et al., 2022). DPPH (0.1 mM) solution was mixed with ethanol and stored in dark. Before measuring the absorbance at 517 nm, the solution was kept incubated in dark at 24°C for 30 min. Quercetin was used as positive control at 0.5 mg/mL.
The evaluation of ABTS radical scavenging activity was carried out following the approach described by (Re et al., 1999). To prepare the ABTS+ working solution, a 7 mM ABTS+ stock solution and a 2.45 mM K2S2O8 solution were added and then incubated for 12 hours at 24°C. Dilution with ethanol was performed and the absorbance was measured at 734 nm. To measure the absorbance at 534 nm, approximately one milliliter of ABTS+ solution at 30°C was mixed with 3 ml Trolox for 30 minutes at different concentrations ranging from 10 to 80 mg/ml. The formula for calculating ABTS+ radical scavenging activity is given below. Vitamin was used as positive control at 0.5 mg/mL.
The ability of the sample to inhibit tyrosinase activity was measured according to (Bakrim et al., 2022). And as follows: 40 μL of the sample was mixed with 40 μL of tyrosinase enzyme and 80 μL of phosphate buffer, and then incubated for 10 minutes at 25 °C. To start the reaction, 40 μL of 5 mM L-DOPA substrate was added. After another 30 minutes of incubation, the absorbance was measured at 475 nm using a microplate reader. The percent inhibition was calculated using the formula: Inhibition (%) = (A0 - A1)/A0 x 100, where A0 is the absorbance of the control tube and A1 is the absorbance of the reaction mixture with the sample. Kojic acid was used as positive control at 0.5 mg/mL.
The activity of substances that inhibit elastase was assessed using a method described by (Abdelfattah et al., 2022) where a sample was mixed with elastase solution and Tris-HCl buffer, then incubated for 15 minutes. Next, a substrate solution was added, and the mixture was incubated for a further 10 minutes before measuring absorbance at 405 nm. A blank control without N-succinyl-Ala-Ala-Ala-p-nitroanilide was included for each sample. The percentage of enzyme inhibition was calculated using the following formula: Inhibition (%) = (A0 - A1)/A0 x 100, where A0 is the absorbance of the control tube and A1 is the absorbance of the reaction mixture with the sample. Kojic acid was used as positive control at 0.5 mg/mL.
The effectiveness of the assay was evaluated using Abnova’s lipoxygenase (LO) inhibitor screening assay kit in accordance with the manufacturer’s instructions. The assay was conducted at a temperature of 25 C. At the outset, the extracts were placed in an assay buffer consisting of 0.1 M tris-HCL with a pH of 7.4. Then, 90 µL of lipoxygenase enzyme and 1mM linoleic acid were added, followed by the addition of potassium hydroxide (0.1 M). After 5 minutes of shaking the mixture, 100 µL chromogen was added to arrest enzyme catalysis and generate the reaction. Finally, the absorbance was measured at 490-500 nm with a plate reader. Diclofenac sodium was used as positive control at 0.5 mg/mL.
The pH value of seawater samples in the study area ranged from 6.7 to 7.9. Site 14 recorded the minimum pH value (6.7), and the maximum pH value was recorded at sites 2, 6, and 17 (Table 1).
The low pH variations recorded in these areas are related to the alkalizing action of seawater (Amazzal et al., 2020). Indeed. the pH of aquatic ecosystems is used as a proxy parameter to represent the complex relationships between water chemistry and biological effects (Diamantini et al., 2018).The presence of organic acids, biological processes (photosynthesis and respiration), and physical processes (turbulence and aeration), which can modify the concentration of dissolved carbon dioxide affect the pH level in the sea (Ingrosso et al., 2016). The pH value measured in site 14, which is lower than the pH values of seawater which varies between 7.5 and 8.4, could be explained by the geochemistry of the area while suspecting the existence of agro-industrial effluents. Similar results were obtained by Hassoun et al. (2014) and Chaouay et al. (2016) reported that organic waste causes a decrease in seawater pH. For the conductivity, the values recorded ranged from 49.9 mS/cm in site 8 to 51.8 mS/cm in site 1 (Figure 2). The conductivity of seawater is very high because of the high concentration of dissolved salts (Chaouay et al., 2016). Moreover, the seawater is most abundant water resource on the earth surface (~71%) and having various mineral contents (25–35 g/L) leading to a good electric conductivity (~50 mS/cm) (Tyler et al., 2017; Visbeck, 2018).
Figure 2. Proteins content of seaweed species. Gro, Gelidium robustum; AM, Algae Mixture; Cga, Codium galeatum; Hin, Halopithys incurve; Pca, Plocamium Cartilagineum; Nla, Neorhodomela larix; Ccr, Chondrus crispus; Hsc, Halopteris scoparia; Hmu, Hypnea musciformis; Ggr, Gracilaria gracilis; Gmu, Gracilaria multipartite; Smu, Sargassum muticum; Pca, Pterocladiella capillacea; Hdi, Halidrys dioica; Ldi, Laminaria digitata.
Total organic carbon (TOC) concentrations are very heterogeneous within the study area. They range from 0.12% to 0.87% (Table 1). The highest TOC levels in seawater were noted in samples from sites 1, 6, 7, 12, and 16, while the lowest ones were observed in sites 8 and 11. The main factors influencing the concentration of TOC in seawater are human activity and biological processes (Wang et al., 2022). Indeed, the elevated TOC concentrations could be excreted by healthy algae, high zooplanktonic activity, and the potential presence of external source of organic matter (Sempéré et al., 2000). Algal blooms are dominated (pico- and microalgae) is also associated to a significant increase in TOC concentration, which could exceed 15 mg/L in some cases (Desormeaux et al., 2011).
Table 1 shows the distribution of available phosphorus in the study area. Mean values range from 0.76 mg/L at site 11 to 7372.23 mg/L at site 6. The concentration of phosphorus was lower in seawater at the majority of the studied sites. relatively high values were recorded at sites 6. 7. 8. and 19. In general, slight variations were observed in the assimilable phosphorus content. The high level of available phosphorus is primarily due to diffusion and migration of phosphorus from the sediment (Ding et al., 2015). However, a large fraction of P is transported in suspension bound to sediment particles (Yin et al., 2022). In general. water movement and resuspension of sediments in the study area could be responsible of high levels of phosphorus compounds, especially in water at sites 6, 7, 8, and 19. On the other hand, nutrients such as phosphates are brought into coastal waters by rivers, agricultural and aquaculture runoff, industrial and household waste (Wu et al., 2017). Table 1 explains the variation of COD concentrations in the study area. The results showed a slight variation depending on the sites The lowest chemical oxygen demand (COD) concentration value was recorded in site 6 (0.82 g/L), while the maximum value (8.47 g/L) was detected in site 9. Compared to freshwater systems, COD measurement in seawater is complicated due to high salt content, pH variations, and the presence of various organic and inorganic contaminants (Jin et al., 2010). High COD values, site 9 for example, are potentially due to the human or/and industrial activity.
As indicated in the Table 1, sodium levels range from 371 ppm at site 6 to 517 ppm at site 1, while potassium levels range from 27.37 ppm at site 17 to 315 ppm at site 9. For calcium, the observed levels indicated range values from 83.73 ppm at site 10 to 126 ppm at site 3.
The obtained results are in accordance with the several previous studies indicating that seawater is a complex medium containing the majority characterized by the presence of major ion i.e chloride (55.03%), sodium (30.65%), sulfate (7.71%), and magnesium (3.65%), while boron (2.4%), calcium (1.17%), and potassium (1.13%) are minor ions (Millero et al., 2008) (Kang et al., 2014). Kang et al. (2014) mentioned that anion in electrolyte solution will be a stronger inhibitor than cation in gas hydrate formation because of its strong interaction, which is kept with the ambient water molecule cluster even after being hydrated.
Table 2 represents the global list of species encountered during this study. Each species is defined by its presence and its systematic position. This list allowed us to establish the structure of the macroalgal flora of the Atlantic coast from Tarfaya to Laayoune city.
During this study, 21 species of macroalgae belonging to 19 genera and 14 families and 9 orders were recorded in the study area (Table 2). These species belong to the phyla Rhodophyta, Ochrophyta, and Chlorophyta. They are distributed in three classes (Florideophyceae, Phaeophyceae and Ulvophyceae) with a dominance of Florideophyceae containing 55.56% of orders, 71.43% of families, 68.42% of genera, and 71.43% of species; followed by the class of Phaeophyceae which contains 33.33% of orders, 21.43% of families, 26.32% of genera and 23.81% of species. The class Ulvophyceae is the least represented with 11.11% of orders, 7.14% of families, 5.26% of genera and 4.76% of species (Table 3).
Table 4 provides information on the relative importance of the different families in the study area.
From 21 species, 8 of them are clearly dominant and represent about 64.7% of the macroalgal flora. These species are: Chondrus crispus, Plocamium Cartilagineum, Gelidium robustum, Gracilaria chilensis, Gracilaria gracilis, Halopithys incurva, Halopteris scoparia, and Laminaria digitata. They represent respectively the families of Florideophyceae (75%) and Phaeophyceae (25%).
This study showed that the macroalgal flora of the Atlantic coast from Tarafaya to Laayoune is characterized by 21 species distributed in 19 genera and 14 families. It was also observed that the Florideophyceae (55.56%) are more represented than the Phaeophyceae (33.33%) and the Ulvophyceae (11.11%). Gracilariaceae and Rhodomelaceae represent the most diverse families with 28.57% among all observed species. The dominance of Floridaophyceae seems to be already observed in the coastal marine waters of the Gulf of Guinea, Ivory coast, and Ghana according (Guiry and Guiry, 2022) with a flora composed of 192 Rhodophytes, 62 Chlorophytes, and 47 Phaeophytes. The same results were found by Gueye et al. (2019) in three marine sites of Dakar with 14.23% of the listed species and (Gueye et al., 2020) in the islands of Dakar with 13.15% of the species encountered (Dictyotaceae).
In Table 5, the results indicate a low diversity in the study area, as evidenced by the Shannon index values being less than 3 for most sites, except for site 6 and site 7. This suggests that the algal community in the study area is not highly diverse overall. However, it is worth noting that site 6 shows the highest diversity index (H’), indicating a relatively higher algal diversity in that specific location.
Additionally, the equitability index, which measures the evenness of species distribution, ranges from 0.23 to 0.76 as shown in Table 4. The highest equitability index is recorded at site 3, indicating a more balanced distribution of individuals among different species at that site. In contrast, site 3 also exhibits the lowest equitability index of 0.23, suggesting the dominance of two species, namely Gracilaria chilensis and Gracilaria gracilis.
For the remaining sites, the equitability index indicates a relatively higher diversity at sites 6, 7, and 9, as reflected by their higher equitability values compared to other sites listed in Table 4. This implies a more even distribution of individuals across different species in those locations.
Concerning the species distribution, 10 species were collected at site 6, 9 species at site 7, 7 species were collected at site 9, 6 species at site 15, 4 species at sites 2,11, 13 and 16. 2 species at site 3 and 1 species at site 5.
Comparison of intra- and inter-habitat diversity (Table 6) showed that similarity coefficients ranged from 0 to 1. This demonstrates that there are sites that are similar and others that have no similarity.
Regarding the species distribution, the observed results could be partly related to the geomorphology of the sampling area where the nature of the substrate plays a very important role on algae distribution. Indeed, Mensi et al. (2014) reported that macroalgae live mainly attached to a solid substrate except for the case of Sargassum which floats on the water surface, and Gracilaria which can live in sand. In addition, the macroalgal distribution could differ according to the climatological variations (Robuchon, 2014). The anthropic aspect must be also considered to explain the distribution variation since the study area is characterized by a strong presence of fishermen.
The pH of macroalgal species varies between 6.49 and 7.87 while the conductivity values vary between 2.81 mS/cm and 23.81 mS/cm (Table 7). Indeed, these values depend on water composition in terms mineral components. Furthermore, the observed value of pH and conductivity depend on several factors such as: seaweed species, natural habitat, and other environmental conditions (Seminara et al., 2008).
The results in Table 7 show that the solid matter varies between 5.95% and 35.3%, while the volatile matter range between 25.77% and 82.01. Indeed, these contents vary according to the species of algae. For example, Gracilaria gracilis is characterized by a high DM of about 19.69%. This value is higher than that reported one by other studies on Gracilaria gracilis (8% to 15.4%) (Rodrigues et al., 2015). Gracilaria gracilis consists of 82.01% volatile matter and 17.99% ash. These values are comparable to those reported by other authors for this alga (17.8% to 34%) (Marinho-Soriano et al., 2006; Sfriso et al., 1994).
According to the Table 7, results show that the algae contain high percentages of TOC (from 17.76% to 39.66%). Indeed, organic carbon, is a good indicator of productivity and CO2 adsorption (Zonneveld et al., 2010).
The results of available phosphorus and sugars of the algae are presented in Table 7. The highest value of available phosphorus is noticed for the species Halopithys incurve with a content of 23.06 mg/g DM, while the species Chondrus crispus contains the lowest content of 5.65 mg/g of DM.
Seaweeds contain significant amounts of sugars ranging from 0.45 mg/g DM to 8.81 mg/g DM. Seaweeds have cell walls composed of soluble parietal carbohydrates (from the extracellular matrix) and insoluble parietal carbohydrates (fixing cellulose fibers) called phycocolloids (Pereira, 2016), known by its high interest in food industry (Rodrigues-Souza et al., 2022).
Results for the major photosynthetic pigments studied in seaweeds are usually presented as content of total chlorophylls a and b, and carotenoids (expressed as µg/100 g dry weight (DW)). The values for total carotenoids, chlorophyll a, and chlorophyll b content are shown in Table 7.
From the Table 7, it may be observed that the concentration of total carotenoids measured varies between 0.92 µg/100 g DW and 81.03 µg/100 g DW, whereas chlorophylls contents measured 28.62 µg/100 g DW and 222.51 µg/100 g DW for chlorophyll a, and 0.37 µg/100 g DW and 92.27 µg/100 g DW for chlorophyll b, respectively. Indeed, photosynthetic pigments are composed of chlorophylls, carotenoids, and phycobilliproteins. Given their powerful antioxidant effect, they have been shown to participate in the prevention of cardiovascular diseases, cancers, ophthalmic diseases, as well as in chronic diseases involving oxidative stress phenomena (Holdt and Kraan, 2011). It was reported that chlorophyll a and b in seaweeds ranged from 68 to 162 ug/g and from 25 to 46 µg/g respectively. For the case of Gracilaria spp., it was reported that chlorophyll a was high with 577.89 µg/g. Chlorophyll content was higher in Gracilaria edulis than G. corticata while carotenoids were higher in G. corticata (Rosemary et al., 2019).
The protein content of each alga was expressed as µg of BSA/g of extract (Figure 2). The different algae presented varied content in protein where Saragasum muticum, Pterocladiella capillacea, and Gracilaria multipartita presented the highest content. Furthermore, total carbohydrate, total protein, fat, were found to be 55.82 ± 3.72 mg/g, 12.15 ± 1.34 mg/g, 0.61 ± 0.08 mg/g, respectively (Balamurugan et al., 2013). In 20% MeOH, Halopithys incurva extracts showed the highest percentage of total carbohydrates among the algae studied whereas levels higher than 6% were detected in Gelidium pusillu (Álvarez-Gómez et al., 2016). Proteins from seaweeds can have antibacterial antioxidant and anti-inflammatory activities.
Regarding total phenolic contents (Figure 3), ethanolic and aqueous extract of all the algae demonstrated a high content of polyphenol ranging between 10-25 mg gallic acid/g of extract except for the ethanolic extract of Gracilaria gracilis where the quantity was less than 10. Thus, Neorhodomela larix, Saragasum muticum and Pterocladiella capillacea exhibited the highest content especially in water extracts. The same results were obtained in total flavonoid content (Figure 4) with Gelidium robustum and Pterocladiella capillacea demonstrating the highest content with more than 4 mg of quercetin/g of extract.
Figure 3. Phenolic compounds of seaweed species. Gro, Gelidium robustum; AM, Algae Mixture; Cga, Codium galeatum; Hin, Halopithys incurve; Pca, Plocamium Cartilagineum; Nla, Neorhodomela larix; Ccr, Chondrus crispus; Hsc, Halopteris scoparia; Hmu, Hypnea musciformis; Ggr, Gracilaria gracilis; Gmu, Gracilaria multipartite; Smu, Sargassum muticum; Pca, Pterocladiella capillacea; Hdi, Halidrys dioica; Ldi, Laminaria digitata.
Figure 4. Flavonoid content of seaweed species. Gro, Gelidium robustum; AM, Algae Mixture; Cga, Codium galeatum; Hin, Halopithys incurve; Pca, Plocamium Cartilagineum; Nla, Neorhodomela larix; Ccr, Chondrus crispus; Hsc, Halopteris scoparia; Hmu, Hypnea musciformis; Ggr, Gracilaria gracilis; Gmu, Gracilaria multipartite; Smu, Sargassum muticum; Pca, Pterocladiella capillacea; Hdi, Halidrys dioica; Ldi, Laminaria digitata.
Many studies studied the content of total phenolic and total flavonoid in different extract of different algae species (Álvarez-Gómez et al., 2016). demonstrated that the highest values of phenolic compounds were obtained for Gelidium pusillum with 6 mg of GA/g DW followed by Halopithys incurva with 4.5 mg GA/g DW. Moreover, saragasum muticum viscozyme extract contain 6.4% of polyphenol per dry weight (Puspita et al., 2017).
The total phenolic content of Hypnea musciformis was 0.61 mg GA/g DW (Balamurugan et al., 2013) while the total phenolic content present in the polyphenol compound H. valentiae 70.08% (Mahendran et al., 2021).
Macroalgae have been used in both food and non-food industries due to their unique properties and characteristic biological activity. Regarding the biological activity of the different species, all the species possess an antioxidant activity with a percentage high than 50% especially in the water extracts. The same results were obtained in the ABTS bioassay (Figure 5), where Hypnea musciformis and Gracilaria multipartita possessed good antiradical activity.
Figure 5. Antioxidant activity (ABTS) of seaweed species. Gro, Gelidium robustum; AM, Algae Mixture; Cga, Codium galeatum; Hin, Halopithys incurve; Pca, Plocamium Cartilagineum; Nla, Neorhodomela larix; Ccr, Chondrus crispus; Hsc, Halopteris scoparia; Hmu, Hypnea musciformis; Ggr, Gracilaria gracilis; Gmu, Gracilaria multipartite; Smu, Sargassum muticum; Pca, Pterocladiella capillacea; Hdi, Halidrys dioica; Ldi, Laminaria digitata; VE, Vitamine E.
The same results were obtained in different studies. The study performed by Alvarez (Álvarez-Gómez et al., 2016) demonstrated that the antioxidant capacity in Gelidium pusillum was correlated with the lipid content in the ABTS assay and with the total carbohydrate content. Also, the phenolic content correlated positively with the antioxidant capacity. However, in the case of Gelidium corneum, in the ABTS assay, the antioxidant capacity was related to only total phenolic content. Moreover, Sargassum angustifolium showed the highest antioxidant activity with an IC50 = 0.231 related to the high content of total phenolics (0.061 mg/g DW) (Mehdinezhad et al., 2016).
Furthermore. The DPPH radical scavenging assay (Figure 6) for the G. edulisis 74.16% and H. valentiae was 61.41%. Also, DPPH radicals scavenging activities in the methanolic extracts of red seaweeds G. corticata (44.32%), G. dura (33.03%), G. debilis (53.34%), G. fergusonii (23.99%) and G. salicornia (53.43%) (Kumar et al., 2011; Mahendran et al., 2021).
Figure 6. Antioxidant activity (DPPH) of seaweed species. Gro, Gelidium robustum; AM, Algae Mixture; Cga, Codium galeatum; Hin, Halopithys incurve; Pca, Plocamium Cartilagineum; Nla, Neorhodomela larix; Ccr, Chondrus crispus; Hsc, Halopteris scoparia; Hmu, Hypnea musciformis; Ggr, Gracilaria gracilis; Gmu, Gracilaria multipartite; Smu, Sargassum muticum; Pca, Pterocladiella capillacea; Hdi, Halidrys dioica; Ldi, Laminaria digitata; Q, Quercetin.
Likewise (Arulkumar et al., 2018), reported that G. edulis showed significantly higher ABTS free radical scavenging activity (40.24%) than G. corticata (32.65%) and exhibited higher nitric oxide radical scavenging activity (36.78%) than G. edulis 35.25%. Thus, the total antioxidant capacity of H. valentiae was found to be 78.12% (Mahendran et al., 2021).
Further the hexanoic extract of the different species demonstrated good activity especially for Codium galeatum; Halopithys incurve; Neorhodomela larix; Pterocladiella capillacea; Ericaria selaginoides; Halidrys dioica and Laminaria digitata. Similar results were obtained in different studies.
The hexanoic extract of Halopithys incurva has been studied for its antioxidant capacity and other bioactive properties. the exttact exhibited the highest antioxidant capacity compared to other solvent extracts, as determined by assays such as FRAP, metal chelating, and lipid peroxidation inhibition tests. This suggests that the hexanic extract is rich in compounds that can effectively scavenge free radicals and protect against oxidative stress (Torres et al., 2022).
Moreover, The hexanoic extract of Codium galeatum has demonstrated antioxidant properties, which are essential for combating oxidative stress in biological systems by scavenging free radicals in different antioxidant assays such as DPPH and ABTS. Anatomical and phytochemical properties of Codium, a marine macroalga. Available at SSRN 4706287.
The hexanoic extract of Halidrys dioica has demonstrated significant antioxidant properties, as evidenced by its ability to scavenge free radicals in assays such as the DPPH radical scavenging test.
The hexanoic extract of Laminaria digitata has demonstrated significant antioxidant properties, which are essential for combating oxidative stress in biological systems. This activity is attributed to the presence of various phenolic compounds and fatty acids that scavenge free radicals (de Alencar et al., 2016).
Moreover, The hexanoic extract of Codium galeatum has demonstrated antioxidant properties, which are essential for combating oxidative stress in biological systems by scavenging free radicals in different antioxidant assays such as DPPH and ABTS. Anatomical and phytochemical properties of Codium, a marine macroalga. Available at SSRN 4706287.
The hexanoic extract of Halidrys dioica has demonstrated significant antioxidant properties, as evidenced by its ability to scavenge free radicals in assays such as the DPPH radical scavenging test.
The hexanoic extract of Laminaria digitata has demonstrated significant antioxidant properties, which are essential for combating oxidative stress in biological systems. This activity is attributed to the presence of various phenolic compounds and fatty acids that scavenge free radicals.
Regarding tyrosinase and elastase inhibition assay (Figures 7, 8), ethanolic extract of Gracilaria multipartita, Pterocladiella capillacea, Laminaria digitata, Sargassum muticum, and Gracilaria multipartita demonstrated an inhibition of more than 60% in tyrosinase. The same results were obtained by (Puspita et al., 2017) Where S. muticum Neutrase extract demonstrated 41.3% of tyrosinase inhibition activity compared to other enzymes. Moreover, S. muticum shearzyme extract demonstrated (32.8%) of elastase inhibition activity.
Figure 7. Anti-age activity (tyrosine) of seaweed species. Gro, Gelidium robustum; AM, Algae Mixture; Cga, Codium galeatum; Hin, Halopithys incurve; Pca, Plocamium Cartilagineum; Nla, Neorhodomela larix; Ccr, Chondrus crispus; Hsc, Halopteris scoparia; Hmu, Hypnea musciformis; Ggr, Gracilaria gracilis; Gmu, Gracilaria multipartite; Smu, Sargassum muticum; Pca, Pterocladiella capillacea; Hdi, Halidrys dioica; Ldi, Laminaria digitata; KA, Kojic acid.
Figure 8. Anti-age activity (elastase) of seaweed species. Gro, Gelidium robustum; AM, Algae Mixture; Cga, Codium galeatum; Hin, Halopithys incurve; Pca, Plocamium Cartilagineum; Nla, Neorhodomela larix; Ccr, Chondrus crispus; Hsc, Halopteris scoparia; Hmu, Hypnea musciformis; Ggr, Gracilaria gracilis; Gmu, Gracilaria multipartite; Smu, Sargassum muticum; Pca, Pterocladiella capillacea; Hdi, Halidrys dioica; Ldi, Laminaria digitata; Q, Kojic acid.
In fact, the hexanoic extract of Halidrys dioica has not been extensively documented. The extract may have applications in dermatological and cosmetic products, particularly for skin care due to its potential skin-whitening properties and ability to inhibit melanin synthesis, like findings in related species like Halidrys siliquosa. The results have shown that the extract of brown algae of Halidrys, alone or in combination with other active agent could be utilized for preparing a cosmetic/dermatological product for topical application to the skin, mucous membranes and/or dander (Reshma et al., 2022).
Regarding anti-inflammatory activities (Figure 9), Hypnea musciformis, Gracilaria gracilis, multipartita, Gracilaria multipartita and Pterocladiella capillacea demonstrated a good lipoxygenase inhibition higher than 40%.
Figure 9. Anti-inflammatory activity of seaweed species. Gro, Gelidium robustum; AM, Algae Mixture; Cga, Codium galeatum; Hin, Halopithys incurve; Pca, Plocamium Cartilagineum; Nla, Neorhodomela larix; Ccr, Chondrus crispus; Hsc, Halopteris scoparia; Hmu, Hypnea musciformis; Ggr, Gracilaria gracilis; Gmu, Gracilaria multipartite; Smu, Sargassum muticum; Pca, Pterocladiella capillacea; Hdi, Halidrys dioica; Ldi, Laminaria digitata; DS, Diclofenac sodium.
Previous studies revealed that the pharmacological functions of Sargassum fusiforme, such as anti-oxidation, anti-tumor, anti-bacteria, anti-virus, hypoglycemic effect, anti-aging and enhancing immunity, are closely related to the molecular weight, the sulfate content, and location of fucoidan (Zhang et al., 2018). Half of the studies on anti-inflammatory activities in Gracilaria species were performed with aqueous extracts or sulfated agarans. In general, these studies showed a good anti-inflammatory potential (Makkar and Chakraborty, 2017; Paramsivam et al., 2016). In contrast, the results with organic extracts showed moderate to no activity (Shu et al., 2013). Other studies disclosed the anti-inflammatory and antinociceptive properties of Pterocladiella capillacea. (Silva et al., 2010) Revealed that P. capillacea did not present significant antinociceptive effects in the hot plate test when compared to morphine at (72.9 mg/kg). It was also observed that P. capillacea (8.1 mg/kg) significantly reduced neutrophil migration by 84%, as compared to untreated animals, suggesting inhibition of inflammatory mediators. Beyond fatty acids, research has indicated that extracts from Pterocladiella capillacea may possess other bioactive compounds with potential health benefits, including anti-inflammatory properties (Wang et al., 2023).
The GC-MS analysis was performed on different hexanoic extracts of different species of algae to determine the chemical constituents. The results were presented in Table 8, where different compounds were identified and categorized into different chemical groups. These groups include ketones, terpenes, fatty acids, fatty alcohols, esters, hydrocarbons, steroids, and others. Table 8 lists the identified compounds with their common names, retention time, and % peak area, grouped by their chemical categories.
Table 8. Lists of the identified compounds with their common names, retention time, and % peak area.
The number of identified compounds varied among the different crude extracts of the algae. A phytol ester named as phytyl-eicosanoate was detected in Plocamium Cartilagineum with 1.52% as shown in Table 6. This compound has been identified in Papyrus (Cyperus papyrus L.) biomass (Rosado et al., 2022). Phytol, a compound commonly utilized in fragrances, cosmetics, shampoos, toilet soaps, household cleaners, and detergents, has been shown to possess antimicrobial, anticancer, and antidiuretic properties. In addition, research has found that phytol serves as a precursor for both vitamin E and vitamin K. This information is supported by studies (Abdel Latef et al., 2017).
Regarding hydrocarbons, from all the algae, the hydrocarbons within the range of C10 to C29, with the odd-numbered carbon atoms (C13, C15, C19, C21, C25, C27, and C29) being the most prevalent. Most of the hydrocarbons identified were alkane. Alkane Eicosane was identified in the different species of seaweeds (Adesalu et al., 2016; Moustafa, 2008).
Macroalgae-derived hydrocarbons have been identified as a promising source of hydrocarbon fuels. There is speculation that hydrocarbons from macroalgae may have played a role in the formation of oil reserves across the globe. Hexacontane and hexatriacontane have been identified in the different species of algae. Other compounds as heptasiloxane, hexadecamethyl was identified in N. oculate, T. suecica, and Chlorella sp. different extracts (Hussein et al., 2020).
Previously, it was discovered that sterols and their derivatives had a significant impact on reducing LDL cholesterol levels in vivo, as noted in a study by (Francavilla et al., 2010). Moreover, phytosterols, which include C28 and C29 sterols, are crucial precursors to the synthesis of various compounds like D vitamins, as observed in research by (Kametani and Furuyama, 1987).
In the studied algae, 3-β-Cholest-5-en-3-ol and 3-β,24Z Stigmasta-5,24(28)-dien-3-ol were the most identified sterols with more than 20% in Laminaria digitata and Plocamium Cartilagineum. In S. longata, seven distinct steroid compounds represented the phytosterols. The sterol profile of the studied algae was consistent with the findings reported by Omer and Attar, 2013. In the various crude extracts of the studied algae, different ester compounds were identified. All these compounds were derived from fatty acid as shown in Table 8. The Phthalic acid, ethyl pentadecyl ester was only detected in the Chondrus crispus, with a peak area percentage of 0.85%. Undec-10-ynoic acid, tetradecyl ester was identified in Chondrus crispus and Halopithys incurve.
In a study conducted by Rzama et al., 1995, different volatile compounds have been identified of two green algae, Scenedesmus sp. and Chlorella vulgaris. The main components in the oil of both algae species include palmitic acid, phytol and 2-butyloctanol, methyl palmitate, methyl oleate, methyl-linoleate, and methy-stearate in the ester fraction, and heptadecane and 1-heptadecene in the hydrocarbon fraction. Minor amounts of ketones and lactones were also detected.
Moreover, the hexanoic extract of Codium galeatum is rich in various bioactive compounds, including fatty acids, sterols, and carotenoids. These compounds contribute to the antioxidant and anti-inflammatory properties of the extract, making it a subject of interest for pharmacological studies (Meinita et al., 2022).
In conclusion, this study explored the biodiversity of macroalgae along the southern Atlantic coast of Morocco and investigated the physicochemical characteristics of seawater in relation to macroalgal diversity parameters. The results revealed variations in pH values, conductivity, total organic carbon, available phosphorus, and chemical oxygen demand across the study area. The macroalgal flora consisted of 21 species belonging to 19 genera and 14 families, with Florideophyceae exhibiting the highest diversity. The identified macroalgae species included Chondrus crispus, Plocamium Cartilagineum, Gelidium robustum, Gracilaria chilensis, Gracilaria gracilis, Halopithys incurva, Halopteris scoparia, and Laminaria digitata. The presence of these macroalgae species indicates the potential economic value of the region’s marine resources. Additionally, gas chromatography-mass spectrometry (GC-MS) analysis revealed the presence of various compounds with potential biological activities, such as lipids, proteins, carbohydrates, and other functional compounds. These compounds hold significant economic potential and find applications in various industries, including food, agriculture, pharmaceuticals, and biotechnology.
Overall, this study contributes to the understanding of macroalgal diversity and the physicochemical factors influencing their distribution along the Moroccan Atlantic coast. The findings have implications for the sustainable exploitation of marine resources and highlight the importance of preserving and managing the biodiversity of macroalgae ecosystems. Further research is warranted to explore the full potential of these macroalgae species and their bioactive compounds for various applications.
The original contributions presented in the study are included in the article/supplementary material. Further inquiries can be directed to the corresponding author.
AB: Formal Analysis, Investigation, Methodology, Writing – original draft, Writing – review & editing. MA: Formal Analysis, Investigation, Methodology, Writing – original draft, Writing – review & editing. WB: Writing – original draft, Writing – review & editing. AM: Writing – original draft, Writing – review & editing. YK: Writing – original draft, Writing – review & editing. HE: Writing – original draft, Writing – review & editing. YO: Writing – original draft, Writing – review & editing. MH: Writing – original draft, Writing – review & editing. LK: Writing – original draft, Writing – review & editing. AE: Formal Analysis, Funding acquisition, Investigation, Methodology, Writing – original draft, Writing – review & editing.
The author(s) declare that financial support was received for the research and/or publication of this article.
We would like to thank OCP and Phosboucraa foundation for the technical assistance and financial support.
The authors declare that the research was conducted in the absence of any commercial or financial relationships that could be construed as a potential conflict of interest.
The author(s) declare that no Generative AI was used in the creation of this manuscript.
All claims expressed in this article are solely those of the authors and do not necessarily represent those of their affiliated organizations, or those of the publisher, the editors and the reviewers. Any product that may be evaluated in this article, or claim that may be made by its manufacturer, is not guaranteed or endorsed by the publisher.
Abdelfattah M. A., Dmirieh M., Bakrim W., Mouhtady O., Ghareeb M. A., Wink M., et al. (2022). Antioxidant and anti-aging effects of Warburgia salutaris bark aqueous extract: Evidences from in silico, in vitro and in vivo studies. J. Ethnopharmacol. 292, 115187. doi: 10.1016/j.jep.2022.115187
Abdel Latef A. A. H., Srivastava A. K., Saber H., Alwaleed E. A., Tran L.-S. P. (2017). Sargassum muticum and Jania rubens regulate amino acid metabolism to improve growth and alleviate salinity in chickpea. Sci. Rep. 7, 1–12.
Adesalu T., Temenu T., Julius M. (2016). Molecular characterization, lipid analysis and GC-MS determination of bioactive compounds identified in a West African strain of the green alga Oedogonium (Chlorophyta). J. Pharmacogn. Phytochem. 5, 01–06.
Adhoni S., Shivasharana C., Kaliwal B. (2016). Identification and characterisation of chlorella vulgaris for biodiesel production. Int. J. Sci. Res. Eng. Stud. 3, 7–15.
Álvarez-Gómez F., Korbee N., Figueroa F. L. (2016). Analysis of antioxidant capacity and bioactive compounds in marine macroalgal and lichenic extracts using different solvents and evaluation methods. Cienc. Mar. 42, 271–288. doi: 10.7773/cm.v42i4.2677
Amazzal A., Ait-Talborjt E., Hermas J., Hafidi N. (2020). Importance of hydrological parameters in the distribution of planktonic eggs and larvae in an upwelling zone (Imessouane Bay, Moroccan Atlantic Coast). Casp. J. Environ. Sci. 18, 1–12.
APHA, AWWA, WEF (1998). Standard methods for the examination of water and wastewater (Washington, DC, USA: American Public Health Association, American Water Works Association and Water Environment Federation). Available at: https://doi.org/30M11/98. (Accessed June 1, 2024)
Armeli Minicante S., Bongiorni L., De Lazzari A. (2022). Bio-based products from mediterranean seaweeds: italian opportunities and challenges for a sustainable blue economy. Sustain 14. doi: 10.3390/su14095634
Arnon D. I. (1949). Copper enzymes in isolated chloroplasts. Polyphenoloxidase Beta vulgaris. Plant Physiol. 24, 1.
Arulkumar A., Rosemary T., Paramasivam S., Rajendran R. B. (2018). Phytochemical composition, in vitro antioxidant, antibacterial potential and GC-MS analysis of red seaweeds (Gracilaria corticata and Gracilaria edulis) from Palk Bay, India. Biocatal. Agric. Biotechnol. 15, 63–71. doi: 10.1016/j.bcab.2018.05.008
Aubert G. (1978). Méthodes d’analyses des sols, 2ème Edn. Marseille: Centre régional de Documentation Pédagogique, 191.
Bahammou N., Cherifi O., Bouamama H., Rezzoum N., Sabri H., Boundir Y. (2021). Checklist of rhodophyceae and the first report of Aglaothamnion tripinnatum and Gaillona gallica in the Moroccan coastline. Egypt. J. Aquat. Res. 47, 101–107. doi: 10.1016/j.ejar.2021.04.007
Balamurugan M., Selvam G. G., Thinakaran T., Sivakumar K. (2013). Biochemical study and GC-MS analysis of Hypnea musciformis (Wulf.) Lamouroux. Am. J. Sci. Res. 8, 117–123.
Banerjee P., Prasad B. (2020). Determination of concentration of total sodium and potassium in surface and ground water using a flame photometer. Appl. Water Sci. 10, 1–7. doi: 10.1007/s13201-020-01188-1
Ben Bakrim W., Aghraz A., Hriouch F., Larhsini M., Markouk M., Bekkouche K., et al. (2022). Phytochemical study and antioxidant activity of the most used medicinal and aromatic plants in Morocco. J. Essent. Oil Res. 34, 131–142. doi: 10.1080/10412905.2022.2029777
Ben Bakrim W., Nurcahyanti A. D. R., Dmirieh M., Mahdi I., Elgamal A. M., El Raey M. A., et al. (2022). Phytochemical Profiling of the Leaf Extract of Ximenia americana var. caffra and Its Antioxidant, Antibacterial, and Antiaging Activities In Vitro and in Caenorhabditis elegans: A Cosmeceutical and Dermatological Approach. Oxid. Med. Cell. Longev.
Bradford M. M. (1976). A rapid and sensitive method for the quantitation of microgram quantities of protein utilizing the principle of protein-dye binding. Anal. Biochem. 72, 248–254. doi: 10.1016/0003-2697(76)90527-3
Cai J. L.A. A.-M.J. C.L. D.L. D.A. D.S. G.G.E. G.J. H.A. L.D. M.G. M.W. P.P. P.C. R.M. R.R. T.M., Y. X. (2021). Seaweeds and microalgae: an overview for unlocking their potential in global aquaculture development. Seaweeds microalgae: an overview unlocking their potential Global aquaculture Dev. doi: 10.4060/cb5670en
Chaouay A., Okhrib R., Hilali M., Bazzi L., Chahid A., Khiri F. (2016). Contribution to the study of physico-chemical analysis and metal contamination of coastal Agadir seawater (South Morocco). J. Mater. Environ. Sci. 7, 2748–2759.
Connelly E. B., Colosi L. M., Clarens A. F., Lambert J. H. (2015). Life cycle assessment of biofuels from algae hydrothermal liquefaction: The upstream and downstream factors affecting regulatory compliance. Energy Fuels 29, 1653–1661. doi: 10.1021/ef502100f
Coppejans E., Prathep A., Leliaert F., Lewmanomont K., De Clerck O. (2010). Khrōngkān Phatthanā ʿOngkhwāmrū læ Sưksā Nayōbāi Kānčhatkān Sapphayākō̜n Chīwaphāp nai Prathēt Thai (Seaweeds of Mu Ko Tha Lae Tai (SE Thailand) : methodologies and field guide to the dominant species).
Dajoz R. (1985). Linné, un précurseur de l’écologie. Publ. la Société Linnéenne Lyon 54, 53–64. doi: 10.3406/linly.1985.10711
de Alencar D. B., de Carvalho F. C. T., Rebouças R. H., Dos Santos D. R., dos-Santos-Pires-Cavalcante K. M., de Lima R. L., et al. (2016). Bioactive extracts of red seaweeds Pterocladiella capillacea and Osmundaria obtusiloba (Floridophyceae: Rhodophyta) with antioxidant and bacterial agglutination potential. Asian Pacific J. Trop. Med. 9, 372–379. doi: 10.1016/j.apjtm.2016.03.015
Derhy G., Macías D., Elkalay K., Khalil K., Rincón M. M. (2022). Stochastic modelling to assess external environmental drivers of atlantic chub mackerel population dynamics. Sustain. 14, 1–19. doi: 10.3390/su14159211
Desormeaux E. D., Meyerhofer P. F., Luckenbach H. R., Kudela R. M. (2011). Pilot-testing multiple pretreatment systems for seawater desalination. IDA J. Desalin. Water Reuse 3, 42–52. doi: 10.1179/ida.2011.3.1.42
Diamantini E., Lutz S. R., Mallucci S., Majone B., Merz R., Bellin A. (2018). Driver detection of water quality trends in three large European river basins. Sci. Total Environ. 612, 49–62. doi: 10.1016/j.scitotenv.2017.08.172
Ding S., Han C., Wang Y., Yao L., Wang Y., Xu D., et al. (2015). In situ, high-resolution imaging of labile phosphorus in sediments of a large eutrophic lake. Water Res. 74, 100–109. doi: 10.1016/j.watres.2015.02.008
Djego J., Gibigaye M., Tente B., Sinsin B. (2012). Analyses écologique et structurale de la forêt communautaire de Kaodji au Bénin. Int. J. Biol. Chem. Sci. 6, 705–713. doi: 10.4314/ijbcs.v6i2.14
Dubois M., Gilles K. A., Hamilton J. K., Rebers P. A., Smith F. (1956). Colorimetric method for determination of sugars and related substances. Anal. Chem. 28, 350–356. doi: 10.1021/ac60111a017
Francavilla M., Trotta P., Luque R. (2010). Phytosterols from Dunaliella tertiolecta and Dunaliella salina: a potentially novel industrial application. Bioresour. Technol. 101, 4144–4150. doi: 10.1016/j.biortech.2009.12.139
Grina F., Ullah Z., Kaplaner E., Moujahid A., Eddoha R., Nasser B., et al. (2020). In vitro enzyme inhibitory properties, antioxidant activities, and phytochemical fingerprints of five Moroccan seaweeds. South Afr. J. Bot. 128, 152–160. doi: 10.1016/j.sajb.2019.10.021
Gueye M. F., Bodian M. Y., Mbaye M. S., Sene G., Noba K. (2019). Analyse de la flore des macroalgues de trois sites marins de Dakar (PNIM, Soumbédioune et Terrou-bi) au Sénégal. Int. J. Biol. Chem. Sci. 13, 634. doi: 10.4314/ijbcs.v13i2.5
Gueye M. F., Mbaye M. S., Dieme N. A., Gueye F. K., Diouf N., Noba K. (2020). Structure et répartition des macroalgues de la côte Nord du Sénégal (Yoff, Kayar, Mboro, Loumpoul et Saint Louis). J. Appl. Biosci. 153, 15798–15806.
Guiry M. D., Guiry G. M. (2022). AlgaeBase (National University of Ireland, Galway: World-Wide Electronic Publication). Available online at: http://www.algaebase.org.
Hassoun M., Salhi G., Bouksir H., Moussa H., Riadi H., Kazzaz M. (2014). Codium tomentosum var. mucronatum et son epiphyte Aglaothamnion pseudobyssoides, deux nouvelles espèces d¿ algues benthiques pour la phycoflore du Maroc. Acta Bot. Malacit., 37–44.
Holdt S. L., Kraan S. (2011). Bioactive compounds in seaweed: functional food applications and legislation. J. Appl. Phycol. 23, 543–597. doi: 10.1007/s10811-010-9632-5
Hussein H. A., Maulidiani M., Abdullah M. A. (2020). Microalgal metabolites as anti-cancer/anti-oxidant agents reduce cytotoxicity of elevated silver nanoparticle levels against non-cancerous vero cells. Heliyon 6, e05263. doi: 10.1016/j.heliyon.2020.e05263
Ingrosso G., Giani M., Cibic T., Karuza A., Kralj M., Del Negro P. (2016). Carbonate chemistry dynamics and biological processes along a river–sea gradient (Gulf of Trieste, northern Adriatic Sea). J. Mar. Syst. 155, 35–49. doi: 10.1016/j.jmarsys.2015.10.013
Jin X. L., Jing M., Chen X., Zhuang Z. X., Wang X. R., Lee F. S. C. (2010). A study on the relationship between BOD5and COD in a coastal seawater environment with a rapid BOD measurement system. Water Sci. Technol. 61, 1499–1503. doi: 10.2166/wst.2010.810
Kametani T., Furuyama H. (1987). Synthesis of vitamin D3 and related compounds. Med. Res. Rev. 7, 147–171. doi: 10.1002/med.2610070202
Kang K. C., Linga P., Park K., Choi S. J., Lee J. D. (2014). Seawater desalination by gas hydrate process and removal characteristics of dissolved ions (Na+, K+, Mg2+, Ca2+, B3+, Cl-, SO42-). Desalination 353, 84–90. doi: 10.1016/j.desal.2014.09.007
Kirk J. T. O., Allen R. L. (1965). Dependence of chloroplast pigment synthesis on protein synthesis: effect of actidione. Biochem. Biophys. Res. Commun. 21, 523–530. doi: 10.1016/0006-291X(65)90516-4
Kulshreshtha G., Hincke M. T., Prithiviraj B., Critchley A. (2020). A review of the varied uses of macroalgae as dietary supplements in selected poultry with special reference to laying hen and broiler chickens. J. Mar. Sci. Eng. 8. doi: 10.3390/JMSE8070536
Kumar M., Kumari P., Trivedi N., Shukla M. K., Gupta V., Reddy C., et al. (2011). Minerals, PUFAs and antioxidant properties of some tropical seaweeds from Saurashtra coast of India. J. Appl. Phycol. 23, 797–810. doi: 10.1007/s10811-010-9578-7
Kumar V., Zozaya-Valdes E., Kjelleberg S., Thomas T., Egan S. (2016). Multiple opportunistic pathogens can cause a bleaching disease in the red seaweed Delisea pulchra. Environ. Microbiol. 18, 3962–3975. doi: 10.1111/emi.2016.18.issue-11
Mahendran S., Maheswari P., Sasikala V., Pandiarajan J. (2021). In vitro antioxidant study of polyphenol from red seaweeds dichotomously branched gracilaria Gracilaria edulis and robust sea moss Hypnea valentiae. Toxicol. Rep. 8, 1404–1411. doi: 10.1016/j.toxrep.2021.07.006
Makkar F., Chakraborty K. (2017). Antidiabetic and anti-inflammatory potential of sulphated polygalactans from red seaweeds Kappaphycus alvarezii and Gracilaria opuntia. Int. J. Food Prop. 20, 1326–1337. doi: 10.1080/10942912.2016.1209216
Marinho-Soriano E., Fonseca P. C., Carneiro M. A. A., Moreira W. S. C. (2006). Seasonal variation in the chemical composition of two tropical seaweeds. Bioresour. Technol. 97, 2402–2406. doi: 10.1016/j.biortech.2005.10.014
Matanjun P., Mohamed S., Mustapha N. M., et al. (2008). Antioxidant activities and phenolics content of eight species of seaweeds from north Borneo. J Appl Phycol. 20, 367–373. doi: 10.1007/s10811-007-9264-6
Mehdinezhad N., Ghannadi A., Yegdaneh A. (2016). Phytochemical and biological evaluation of some Sargassum species from Persian Gulf. Res. Pharm. Sci. 11, 243.
Meinita M. D. N., Harwanto D., Choi J. S. (2022). A concise review of the bioactivity and pharmacological properties of the genus Codium (Bryopsidales, Chlorophyta). J. Appl. Phycology 34, 2827–2845. doi: 10.1007/s10811-022-02842-8
Mensi F., Ksouri J., Hammami W., Romdhane M. S. (2014). Etat des connaissances et perspectives de recherches sur la culture de Gracilaria (Gracilaria et Gracilariopsis): application à la lagune de Bizerte. INSTM Bulletin: Marine and Freshwater Sciences 41, 101–119.
Millero F. J., Feistel R., Wright D. G., McDougall T. J. (2008). The composition of Standard Seawater and the definition of the Reference-Composition Salinity Scale. Deep Sea Res. Part I Oceanogr. Res. Pap. 55, 50–72. doi: 10.1016/j.dsr.2007.10.001
Mohy-El-Din S. M., El-Ahwany A. M. D. (2016). Bioactivity and phytochemical constituents of marine red seaweeds (Jania rubens, Corallina mediterranea and Pterocladia capillacea). J. Taibah Univ. Sci. 10, 471–484. doi: 10.1016/j.jtusci.2015.06.004
Moustafa A. M. Y. (2008). Comparative phycochemical investigation of hydrocarbons content on some marine seaweeds algae’Amal M. Youssef moustafa,“Gehan A. El-shoubaky and ”Essam abd E. Salem" Department of chemistry, faculty of science, suez canal university. Res. J. Phytochem. 2, 10–17.
Msuya F. E., Bolton J., Pascal F., Narrain K., Nyonje B., Cottier-Cook E. J. (2022). Seaweed farming in Africa: current status and future potential. J. Appl. Phycol. 34, 985–1005. doi: 10.1007/s10811-021-02676-w
Omer T. A., Attar T. (2013). Isolation and identification of some chemical constituents in two different types of fresh water macro algae in Bestansur village in Suleiman City Kurdistan Region (North Iraq) by Hplc technique. IOSR J. Appl. Chem., 2278–5736.
Ortiz-Burgos S. (2016). “Shannon-weaver diversity index BT - encyclopedia of estuaries,”. Ed. Kennish M. J. (Springer Netherlands, Dordrecht), 572–573. doi: 10.1007/978-94-017-8801-4_233
Ouahid E. A., Mohamed R., Soufiane F. (2021). Green seaweed polysaccharides inventory of nador lagoon in north east Morocco. Polysaccharides Prop. Appl., 163–175. doi: 10.1002/9781119711414.ch8
Paramsivam R., Sudevan S., Sundar S., Ramasamy V. (2016). Study on metabolic compounds of Gracilaria salicornia against anti-inflammatory activity. Int. J. Curr. Microbiol. Appl. Sci. 5, 202–211. doi: 10.20546/ijcmas.2016.504.025
Pereira L. (2016). Edible seaweeds of the world, edible seaweeds of the world. CRC Press (Taylor and Francis Group). doi: 10.1201/b19970
Puspita M., Déniel M., Widowati I., Radjasa O. K., Douzenel P., Marty C., et al. (2017). Total phenolic content and biological activities of enzymatic extracts from Sargassum muticum (Yendo) Fensholt. J. Appl. Phycol. 29, 2521–2537. doi: 10.1007/s10811-017-1086-6
Re R., Pellegrini N., Proteggente A., Pannala A., Yang M., Rice-Evans C. (1999). Antioxidant activity applying an improved ABTS radical cation decolorization assay. Free Radic. Biol. Med. 26, 1231–1237. doi: 10.1016/S0891-5849(98)00315-3
Reshma B. S., Aavula T., Narasimman V., Ramachandran S., Essa M. M., Qoronfleh M. W. (2022). Antioxidant and antiaging properties of agar obtained from brown seaweed Laminaria digitata (Hudson) in D-galactose-induced swiss albino mice. Evidence-Based Complementary Altern. Med. 2022, 7736378. doi: 10.1155/2022/7736378
Robuchon M. (2014). Etude spatio-temporelle de la biodiversité des forêts de laminaires des côtes bretonnes par une approche intégrée de génétique des populations et d’écologie des communautés. Thèse de doctorat. Museum national d'histoire naturelle-MNHN PARIS.
Rodrigues D., Freitas A. C., Pereira L., Rocha-Santos T. A. P., Vasconcelos M. W., Roriz M., et al. (2015). Chemical composition of red, brown and green macroalgae from Buarcos bay in Central West Coast of Portugal. Food Chem. 183, 197–207. doi: 10.1016/j.foodchem.2015.03.057
Rodrigues-Souza I., Pessatti J. B. K., da Silva L. R., de Lima Bellan D., de Souza I. R., Cestari M. M., et al. (2022). Protective potential of sulfated polysaccharides from tropical seaweeds against alkylating- and oxidizing-induced genotoxicity. Int. J. Biol. Macromol. 211, 524–534. doi: 10.1016/j.ijbiomac.2022.05.077
Rombouts I., Simon N., Aubert A., Cariou T., Feunteun E., Guérin L., et al. (2019). Changes in marine phytoplankton diversity: Assessment under the Marine Strategy Framework Directive. Ecol. Indic. 102, 265–277. doi: 10.1016/j.ecolind.2019.02.009
Rosado M. J., Marques G., Rencoret J., Gutiérrez A., Bausch F., Rosenau T., et al. (2022). Chemical composition of the lipophilic compounds from the rind and pith of papyrus (Cyperus papyrus L.) stems. Front. Plant Sci. 13, 5378. doi: 10.3389/fpls.2022.1097866
Rosemary T., Arulkumar A., Paramasivam S., Mondragon-Portocarrero A., Miranda J. M. (2019). Biochemical, micronutrient and physicochemical properties of the dried red seaweeds gracilaria edulis and gracilaria corticata. Molecules 24, 1–14. doi: 10.3390/molecules24122225
Rzama A., Benharref A., Arreguy B., Dufourc E. (1995). Volatile compounds of green microalgae grown on reused waste water. Phytochemistry 38, 1375–1379. doi: 10.1016/0031-9422(94)00835-H
Seminara M., Vagaggini D., Margaritora F. G. (2008). Differential responses of zooplankton assemblages to environmental variation in temporary and permanent ponds: zooplankton of temporary and permanent ponds. Aquat. Ecol. 42, 129–140. doi: 10.1007/s10452-007-9088-0
Sempéré R., Yoro S. C., Van Wambeke F., Charrière B. (2000). Microbial decomposition of large organic particles in the northwestern Mediterranean Sea: an experimental approach. Mar. Ecol. Prog. Ser. 198, 61–72.
Sfriso A., Marcomini A., Pavoni B. (1994). Gracilaria distribution, production and composition in the lagoon of Venice. Bioresour. Technol. 50, 165–173. doi: 10.1016/0960-8524(94)90069-8
Shu M.-H., Appleton D., Zandi K., AbuBakar S. (2013). Anti-inflammatory, gastroprotective and anti-ulcerogenic effects of red algae Gracilaria changii (Gracilariales, Rhodophyta) extract. BMC Complement. Altern. Med. 13, 1–13. doi: 10.1186/1472-6882-13-61
Silva L. M. C. M., Lima V., Holanda M. L., Pinheiro P. G., Rodrigues J. A. G., Lima M. E. P., et al. (2010). Antinociceptive and anti-inflammatory activities of lectin from marine red alga Pterocladiella capillacea. Biol. Pharm. Bull. 33, 830–835. doi: 10.1248/bpb.33.830
Torres P., Chow F., dos Santos D. Y. A. C. (2022). A chemical investigation of the antioxidant capacity of extracts from red macroalga Gracilaria domingensis. Phycology 2, 332–343. doi: 10.3390/phycology2030018
Tyler R. H., Boyer T. P., Minami T., Zweng M. M., Reagan J. R. (2017). Electrical conductivity of the global ocean. Earth Planets Sp. 69, 1–10. doi: 10.1186/s40623-017-0739-7
Vimala T., Poonghuzhali T. V. (2015). Estimation of pigments from seaweeds by using acetone and DMSO. Int. J. Sci. Res. 4, 1850–1854.
Visbeck M. (2018). Ocean science research is key for a sustainable future. Nat. Commun. 9, 690. doi: 10.1038/s41467-018-03158-3
Wang C., Ding Y., Guo Z., Lin H., Wu J. (2022). Spatial–temporal distribution of total organic carbon and its transportation in the Jiulong River Estuary. Sci. Rep. 12, 1–15. doi: 10.1038/s41598-022-13268-0
Wang X., Wiegand T., Kraft N. J. B., Swenson N. G., Davies S. J., Hao Z., et al. (2016). Stochastic dilution effects weaken deterministic effects of nichebased processes in species rich forests. Ecology 97, 347–360. doi: 10.1890/14-2357.1
Wang Y., Zhou L., Chen M., Liu Y., Yang Y., Lu T., et al. (2023). Mining xanthine oxidase inhibitors from an edible seaweed Pterocladiella capillacea by using in vitro bioassays, affinity ultrafiltration LC-MS/MS, metabolomics tools, and in silico prediction. Mar. Drugs 21, 502. doi: 10.3390/md21100502
Woisky R. G., Salatino A. (1998). Analysis of propolis: some parameters and procedures for chemical quality control. J. Apic. Res. 37, 99–105. doi: 10.1080/00218839.1998.11100961
Wu Q., Qi J., Xia X. (2017). Long-term variations in sediment heavy metals of a reservoir with changing trophic states: Implications for the impact of climate change. Sci. Total Environ. 609, 242–250. doi: 10.1016/j.scitotenv.2017.04.041
Yang Y., Zhang M., Alalawy A. I., Almutairi F. M., Al-Duais M. A., Wang J., et al. (2021). Identification and characterization of marine seaweeds for biocompounds production. Environ. Technol. Innov. 24, 101848. doi: 10.1016/j.eti.2021.101848
Yin H., Zhang M., Yin P., Li J. (2022). Characterization of internal phosphorus loading in the sediment of a large eutrophic lake (Lake Taihu, China). Water Res. 225, 119125. doi: 10.1016/j.watres.2022.119125
Zhang J., Zhu L., Li F., Liu C., Qiu Z., Xiao M., et al. (2018). Comparison of toxic metal distribution characteristics and health risk between cultured and wild fish captured from Honghu city, China. . Int. J. Environ. Res. Public Health 15, 334. doi: 10.3390/ijerph15020334
Keywords: seaweed, biodiversity, secondary metabolites, biological activities, biorefinery
Citation: Boutafda A, Akkari M, Ben Bakrim W, Mazar A, El Kharrassi Y, Elarroussi H, Ouhdouch Y, Hafidi M, Kouisni L and Ezzariai A (2025) Holistic investigation of macroalgal species richness along the Southern Atlantic Coast of Morocco. Front. Mar. Sci. 12:1544737. doi: 10.3389/fmars.2025.1544737
Received: 13 December 2024; Accepted: 26 February 2025;
Published: 25 March 2025.
Edited by:
Santhiyagu Prakash, Tamil Nadu Fisheries University, IndiaReviewed by:
Ramasamy Ramasubburayan, Saveetha University, IndiaCopyright © 2025 Boutafda, Akkari, Ben Bakrim, Mazar, El Kharrassi, Elarroussi, Ouhdouch, Hafidi, Kouisni and Ezzariai. This is an open-access article distributed under the terms of the Creative Commons Attribution License (CC BY). The use, distribution or reproduction in other forums is permitted, provided the original author(s) and the copyright owner(s) are credited and that the original publication in this journal is cited, in accordance with accepted academic practice. No use, distribution or reproduction is permitted which does not comply with these terms.
*Correspondence: Amine Ezzariai, YW1pbmUuZXp6YXJpYWlAdW02cC5tYQ==
Disclaimer: All claims expressed in this article are solely those of the authors and do not necessarily represent those of their affiliated organizations, or those of the publisher, the editors and the reviewers. Any product that may be evaluated in this article or claim that may be made by its manufacturer is not guaranteed or endorsed by the publisher.
Research integrity at Frontiers
Learn more about the work of our research integrity team to safeguard the quality of each article we publish.