- 1Chongqing Key Laboratory of Conservation and Utilization of Freshwater Fishes, Animal Biology Key Laboratory of Chongqing Education Commission of China, College of Life Sciences, Chongqing Normal University, Chongqing, China
- 2Laboratory of Water Ecological Health and Environmental Safety, College of Life Sciences, Chongqing Normal University, Chongqing, China
Anxiety is a multifaceted emotional response exhibited by animals when confronted with potential threats. Among most vertebrates, including mammals and fish, there is a pronounced sexual dimorphism in anxiety responses, with females typically demonstrating higher anxiety levels than males. Concurrently, endogenous estrogen levels, specifically 17β-estradiol (E2), are significantly higher in females compared to males. This suggests a potential positive regulatory role of E2 on anxiety, contributing to sexually dimorphic anxiety in fish. To elucidate the role of E2 in mediating sexually dimorphic anxiety responses, male zebrafish (Danio rerio) were administered E2 (E2-M), while females were treated with letrozole (LET, an aromatase inhibitor that reduces E2 synthesis, LET-F) for 60 days, and plasma and brain levels of E2 were detected and anxiety response was evaluated by a novel tank diving test. Females (C-F) showed significantly higher anxiety responses, along with elevated E2 and cortisol levels in plasma and brain, and reduced brain serotonin (5-HT) and dopamine (DA) levels compared to males (C-M). Treatment with LET significantly decreased E2 levels in the plasma and brain of female zebrafish, which corresponded with reduced anxiety responses, lower plasma cortisol levels, and increased brain 5-HT and DA content. Additionally, the expression of genes associated with E2, cortisol, 5- HT, and DA pathways was relevantly altered. Conversely, E2 treatment in males (E2-M) increased E2 levels and anxiety responses, elevated plasma cortisol levels, and decreased brain 5-HT and DA content, with corresponding changes in gene expression. These findings strongly suggest that E2 positively regulates sexually dimorphic anxiety responses possibly by modulating plasma cortisol levels and the synthesis and action of 5-HT/DA in the brain.
1 Introduction
Anxiety is a physiological, psychological, and behavioral stress response elicited by actual or perceived distress or existential threats, typically triggered in individuals confronted with threatening stimuli or potential danger (Zarrindast and Khakpai, 2015; Russart and Nelson, 2018). Appropriate levels of anxiety enable animals to maintain vigilance, keenly perceive environmental changes, and evade predators, thereby reducing the risk of predation (Perrot-Minnot et al., 2017); however, altered anxiety may disturb feeding and social interactions in animals and affect their social status and reproductive competitiveness, ultimately being detrimental to the survival of individuals and perpetuation of the populations (Kavaliers and Choleris, 2001; Perrot-Minnot et al., 2017).
The endocrine system, with its array of hormones, plays a pivotal role in the modulation of stress responses in animals, and cortisol is central to the regulation of these stress reactions (Ghisleni et al., 2012; Duboué et al., 2017). In zebrafish (Danio rerio), the hypothalamus-pituitary-interrenal (HPI) axis governs cortisol production and secretion. Upon stimulation, the hypothalamus produces corticotropin-releasing hormone (CRH; encoded by crha and crhb), which stimulates the release of pro-opiomelanocortin through the receptors CRHR1 and CRHR2 (encoded by crhr1 and crhr2) (Alderman and Bernier, 2009; Herman et al., 2016; Oh et al., 2020). Under the action of prohormone convertases 1 and 2 (encoded by pc1 and pc2, respectively), adrenocorticotropic hormone (ACTH; encoded by actha and acthb) is produced and released, which leads to the synthesis and secretion of cortisol in the interrenal tissue (Alderman and Bernier, 2009; Herman et al., 2016; Oh et al., 2020). Moreover, CRH-binding protein (encoded by crhbp) inhibits ACTH secretion and reduces cortisol production by competitively binding to CRH (Shontz et al., 2018).
Additionally, extensive research has confirmed that the inhibitory neurotransmitter serotonin (5-hydroxytryptamine; 5-HT) and excitatory neurotransmitter dopamine (DA) exert anxiolytic effects in a range of vertebrate species, including fish (Kalueff et al., 2012; Jia and Pittman, 2015; Tu et al., 2020). In zebrafish, 5-HT in the brain is synthesized by tryptophan hydroxylase (encoded by tph1 and tph2) and regulated by the transcription factor Pet-1 (encoded by pet1) (Bellipanni et al., 2002; Lillesaar, 2011). When transported into synaptic vesicles by the vesicular monoamine transporter (VMAT2; encoded by slc18a2), 5-HT is released into the synaptic cleft and is subsequently reabsorbed by the serotonin transporter (encoded by slc6a4a and slc6a4b), which is responsible for 5-HT recycling from the synaptic cleft (Norton et al., 2008). 5-HT exerts its effects by binding to specific receptors (such as 5-HT1A and 5-HT1B) on effector neurons and is subsequently degraded by monoamine oxidase (encoded by mao) (Norton et al., 2008; Albert et al., 2014; Maximino et al., 2015).
Similar to 5-HT, the synthesis of DA in the brain is mediated by tyrosine hydroxylase (encoded by th1 and th2), transported by VMAT2, and released into the synaptic cleft, where it is reabsorbed by the DA transporter (encoded by slc6a3), which is responsible for the reuptake of free DA from the synaptic cleft back into the DAergic neuron (Kaslin and Panula, 2001; Shontz et al., 2018; Tang et al., 2019). DA binds to Drd1 and Drd2 receptors to elicit excitatory neurotransmission and is subsequently metabolized and inactivated by catechol-O-methyltransferase (encoded by comta and comtb) (Kaslin and Panula, 2001; Shontz et al., 2018; Tang et al., 2019). Changes in the expression of these genes are believed to affect the levels of 5-HT and DA and their effects on the brain, thereby disrupting the anxiety response in zebrafish.
Among vertebrates, including higher mammals and more primitive fish, anxiety (a stress response) exhibits sexual dimorphism (Guo et al., 2004; Ossenkopp et al., 2005; Weil et al., 2007; de Abreu et al., 2021). In fish and rodents, females appear to be more prone to anxiety and exhibit heightened perceptivity and vigilance in response to various environmental stressors (Ossenkopp et al., 2005; Weil et al., 2007; Woodward et al., 2023).
Sex hormones, such as estrogen, which regulate a broad range of physiological functions, are considered the most important causes of various behaviors and physiological sexual dimorphisms (Gegenhuber et al., 2022; Zhang et al., 2022). Among these estrogens, 17β-estradiol (E2) is the primary estrogen during reproductive stages and a major regulator of sexual differentiation in vertebrates (Lagranha et al., 2018; Saito and Cui, 2018). An extensive body of evidence suggests that estrogen levels and functions exhibit sexual dimorphism, including differences within the neuroendocrine system (Che et al., 2019; Gegenhuber et al., 2022). E2 is considered to play a significant role in regulating physiological functions and cognitive abilities in female individuals (Hwang et al., 2022; Iqbal et al., 2024). Fluctuations and changes in the levels of endogenous estrogens, such as E2, are generally assumed to susceptibility to anxiety, particularly in humans (Borrow and Handa, 2017).
Based on the positive correlation between E2 levels and anxiety responses as well as research on sex-related differences in neurotransmitters and hormones, several studies have focused on the key role of E2 in regulating anxiety responses in rodents, alongside 5-HT, DA, and the hypothalamus-pituitary-adrenal (HPA) axes (Songtachalert et al., 2018; Yin et al., 2022). However, the research on fish remains limited. Interestingly, brain regions and related neurotransmitter systems associated with anxiety responses also exhibit sexual dimorphisms in vertebrates, including fish. Previous studies have shown that various neurotransmitters (such as 5-HT) in the hippocampus display distinct sexual dimorphisms (Jones and Lucki, 2005; Gressier et al., 2016). In the DAergic system, DA receptors exhibit different expression patterns in brain regions between the sexes, and the distribution and number of neurons also differ between male and female brain regions (Orendain-Jaime et al., 2016; Zachry et al., 2021). In mammals, E2 promotes neurogenesis and prevents neuronal damage, and on the other hand, regulates the neuronal activity and neurotransmitter release, thereby affecting synaptic function (Sherwin, 2003; Genazzani et al., 2007). Furthermore, the HPA/HPI axis is more active in females, with cortisol levels significantly higher than in males (Asher et al., 2017; Wang et al., 2024). This evidence suggests that E2 may play a role in regulating vertebrate mood by regulating the above-mentioned factors (Sharma et al., 2021; He et al., 2024).
Although preliminary studies have revealed the role of E2 in anxiety responses in rodents, whereas such research in fish is very limited (Sharma et al., 2021; He et al., 2024). In fact, the mechanisms by which E2 may regulate sexually dimorphic anxiety responses in animals remain largely unknown. The effects and sexually dimorphic regulatory mechanisms of E2 in anxiety in vertebrates, particularly in fish, remain to be explored (Ossenkopp et al., 2005; Ellis et al., 2023).
Zebrafish is a widely used model animal, findings in zebrafish are usually highly representative among fish species (Muralidharan et al., 2024). Consequently, the role of endogenous E2 in the regulation of anxiety responses in fish and the possible regulatory pattern of endogenous E2 in sexually dimorphic anxiety responses were studied using zebrafish as the model in this study.
To understand the role of endogenous E2 in the regulation of anxiety responses in fish and the possible regulatory pattern of endogenous E2 in sexually dimorphic anxiety responses, adult male and female zebrafish with artificially disturbed endogenous E2 levels were subjected to a novel tank diving test to assess their anxiety responses. Subsequently, plasma cortisol and E2 levels, as well as brain E2, 5-HT, and DA levels were measured using enzyme-linked immunosorbent assays (ELISA). The mRNA levels of key genes associated with E2 synthesis and action (cyp19a1a, cyp19a1b, foxl2, esr1, esr2a, esr2b, and gper1), HPI/cortisol axis (crha, crhb, crhbp, crhr1, crhr2, actha, acthb, pc1, and pc2), and 5-HT (tph2, tph1b, mao, pet1, slc6a4a, slc6a4b, htr1aa, htr1ab, and htr1b)/DA (th1, comta, comtb, slc6a3, drd1b, drd2a, drd2b, and slc18a2) pathways were further investigated by quantitative reverse-transcription PCR (qRT-PCR).
2 Materials and methods
2.1 Animals
Adult zebrafish (AB strain, 6 months of age) were purchased from the China Zebrafish Resource Center and were housed in a thermostatic recirculating aquaculture system before the experiment (temperature: 28 ± 0.5°C; lighting: 14 h; average illuminance: 800 ± 350 lx; dissolved oxygen: 6.2–7.5 mg/L; pH: 7.3–7.6). The zebrafish were fed a microparticle-based compound diet (Shengsuo, Co. Ltd., Shandong, China) three times per day, i.e., at 9:00 am, 3:00 pm, and 9:00 pm. To avoid intraspecific stress, the average rearing density of the zebrafish was maintained at 0.8 individuals/L. All animal experiments were performed in accordance with the Guide for the Care and Use of Laboratory Animals and were approved by the Committee of Laboratory Animal Experimentation of Chongqing Normal University (Approval No. CKLCUFF20240701-02).
2.2 Drug treatment
Ninety female and ninety male fish were randomly selected from the culture system and placed in 12 parallel tanks (50× 25× 30 cm with 30 L water). Four groups with three replicates (15 fish per tank) were established: control-female (solvent, acetone; C-F), control-male (solvent, acetone; C-M), letrozole-female (6 mg/g letrozole; LET-F), and E2-male (200 ng/g E2; E2-M). For pharmacological treatment, E2 (200 ng/g) and letrozole (LET; an aromatase inhibitor used to reduce E2 synthesis; 6 mg/g) were dissolved in acetone. The LET-F and E2-M diets were moistened with acetone containing the respective drugs, whereas the C-F and C-M diets were moistened with an equal volume of drug-free acetone. The treated diets were thoroughly dried at room temperature before use. Zebrafish of each group were fed the respective diet three times per day (at 9:00 am, 3:00 pm, and 9:00 pm) for 60 days. The daily amount of feed was 1.5%–2% of the fish body weight.
2.3 Novel tank diving test
To date, the novel tank diving test has proven to be effective in studying anxiety in zebrafish and is currently one of the most commonly used behavioral test models for fish (Levin et al., 2007; Kysil et al., 2017). The novel tank diving test was conducted according to the method described by Tu et al. (2020). Briefly, dechlorinated tap water was added to a novel tank (15 × 20 × 25 cm) to a height of 10 cm, and a horizontal line was drawn 5 cm from the waterline, dividing the water evenly into upper and lower layers (Figure 1). A high-definition camera (SONY FDR-AX60) was placed approximately 40 cm in front of each novel tank to record the behavior of the experimental fish. After individually placing 45 fish from each group in the novel tank, behavioral parameters were recorded for 5 min (N = 45). Generally, once a fish enters a new or strange environment (e.g., a novel tank) that triggers an anxiety response, it instinctively dives to the bottom of the waterbody to seek shelter. Consequently, when introduced to a novel tank, zebrafish immediately descend to the bottom of the tank in search of shelter and only begin to explore upward when they perceive the environment as safe. Based on previous research including our study (Egan et al., 2009; Tu et al., 2020), the following behavioral parameters were selected: 1) the latency to enter upper layer (the onset at which the zebrafish initially explored the upper strata of the water), and 2) the total duration spent in lower layer (the total duration that zebrafish spent in the lower water layer) (Cachat et al., 2011; Tu et al., 2020).
2.4 Sampling
After the novel tank diving test, fish were returned to their treatment tanks for recovery. After 24 h of recovery, MS-222 at a concentration of 0.1% was carefully administered to the corner of the tank using a pipette to anesthetize all experimental fish, which were subsequently dissected on ice. Post-anesthesia, blood was collected from 15 fish (4 µL per fish) using heparinized capillary tubes to compile one sample (N = 1), with each group having three biological replicates (N = 3). These blood samples were used to determine plasma E2 and cortisol hormone levels. After blood collection, the fish were dissected to retrieve their brains, which were allocated as follows: six brains per biological sample (N = 1), with three brain samples (N = 3) per group for the detection of brain E2 levels; three brains per biological sample (N = 1), with three brain samples (N = 3) per group for the detection of brain 5-HT levels; three brains per biological sample (N = 1), with three brain samples (N = 3) per group for the detection of brain DA levels; and three brains per biological sample (N = 1), with three brain samples (N = 3) per group for total RNA extraction. For the brains intended for total RNA extraction, each biological sample was placed in an RNA-free tube containing 1 mL TRIzol (RNAiso Plus, Takara, Dalian, China) and was homogenized. After thorough grinding in a high-throughput tissue grinder (Scientz-48, Ningbo, China), the homogenate was stored at -80°C until total RNA extraction.
2.5 Measurements of E2, cortisol, 5-HT, and DA levels
Following the method described by Tu et al. (2020), the plasma E2 and cortisol levels were measured using specific E2 and cortisol ELISA kits (Cayman, Ann Arbor, MI, USA). Briefly, the blood samples were centrifuged at 5000 × g and 4°C for 10 min, and the supernatant was collected to obtain plasma. The obtained plasma was aliquoted and diluted 40-fold (for E2 of females), 20-fold (for E2 of males), and 150-fold (for cortisol) to determine the plasma E2 and cortisol levels according to the kit manufacturer’s instructions (Cayman).
For the estimation of brain E2 levels, the brain samples were rinsed with physiological saline (0.86%) at 4°C and accurately weighed. Pre-chilled physiological saline was added to each sample at a ratio of 9:1 (mL/g). The brains were homogenized in a high-throughput tissue grinder and centrifuged at 5000 × g and 4°C for 5 min. The supernatant was collected, and E2 concentrations were measured using an E2 ELISA kit (Cayman) according to the kit manufacturer’s instructions.
The levels of 5-HT and DA in the brain were quantified using 5-HT and DA ELISA kits (SINOBESTIO, Shanghai, China). The procedure involved rinsing the brain samples designated for the 5-HT and DA assays with ice-cold physiological saline, followed by precise recording of their weights. Subsequently, each sample was mixed with pre-chilled physiological saline at a ratio of 9:1 (mL/g). Following homogenization, the samples were centrifuged at 5000 × g and 4°C for 5 min. The resulting supernatant was used to determine the concentrations of 5-HT and DA, according to the manufacturer’s instructions.
2.6 qRT-PCR
Total RNA extraction and qRT-PCR were performed as previously described (Wang et al., 2019). Briefly, brain tissues were homogenized in 1 mL of TRIzol reagent (Invitrogen, NY, USA) to isolate total RNA. RNA quality was verified by agarose gel electrophoresis, and RNA concentration was determined using a NanoDrop ND-2000 spectrophotometer (Thermo Electron Corporation, Waltham, MA, USA). RNA integrity was confirmed using agarose gel electrophoresis. DNase I was applied to eliminate genomic DNA contamination, and cDNA synthesis was performed from 1 μg RNA using a TakaRa Reverse Transcription Kit (TaKaRa, Dalian, China). Gene-specific primers for each target gene and the internal reference genes (rpl13a and ef1a) was prepared as published previously (Table 1), and their specificity were confirmed by observing a single band of the expected size in the PCR products derived from the cDNA of C-F fish. The internal reference genes rpl13a and ef1a were selected because of their relatively constant expression levels across various tissues in zebrafish, and their expression levels remained unchanged after drug treatment. Following the method described previously (Wang et al., 2019), primer fit and stability of the reference gene across all groups were validated. qRT-PCR was performed using a SYBR® Premix Ex TaqTM kit (TaKaRa) on a CFX96TM Real-time PCR Detection system (Bio-Rad, Hercules, CA, USA). All samples were assayed in triplicates in 96-well PCR plates (Axygen Biosciences, Union City, CA, USA). qRT-PCR thermal cycling conditions were as follows: 95°C for 30 s, followed by 40 cycles of 95°C for 5 s, 60°C for 30 s and 72°C for 10 s. To confirm that each primer pair produced a single PCR product and to avoid contamination, dissociation curve analysis and non-template controls were conducted for each PCR reaction. Using a series of cDNA dilutions and their corresponding Ct values, the amplification efficiencies of all genes were calculated to be between 94.8% and 103.8%. As previously described, the expression of each target gene was quantified relative to the average value of rpl13a and ef1a using the 2-ΔΔCT method (Livak and Schmittgen, 2001).
2.7 Statistical analysis
The data are expressed as means ± standard error of the mean. The normality and homogeneity of variance of the original data were analyzed using Shapiro–Wilk and Levene’s tests, respectively. Given that the behavioral data did not conform to a normal distribution, a generalized linear model was used to analyze the relationship between the different treatments and anxiety responses. In this analysis process, a 95% confidence interval level was established, and the “Wald” Chi-squared statistic was used, followed by pairwise comparisons of each treatment group. One-way ANOVA was used to analyze other indicators (hormones, neurotransmitters, and gene expression), followed by post-hoc testing using Tukey’s test. Statistical analysis was performed using SPSS 27.0 (Chicago, IL, USA), and the statistics results were statistically significant when P < 0.05. GraphPad Prism 8.0 (San Diego, CA, USA) was used for graphing purposes, and R software (v.4.2.2) with the packages “ggpubr” (v0.4.0) and “ggplot2” (v3.4.2) through Hiplot Pro (https://hiplot.com.cn/, a comprehensive web service for biomedical data analysis and visualization) was utilized to generate figures (Villanueva and Chen, 2019; Kassambara, 2020).
3 Results
3.1 Plasma and brain E2 levels and expressions of genes for E2 synthesis and action
The plasma and brain E2 levels of C-F fish were significantly higher than those of C-M fish (Figure 2A, P (plasma E2) < 0.001; Figure 2B, P (brain E2) < 0.001). Moreover, the mRNA levels of cyp19a1a, foxl2, esr1, and gper1 in the brains of C-F fish were significantly higher than those in C-M fish brains (Figure 2C, P (cyp19a1a) < 0.001, P (foxl2) = 0.045, P (esr1) = 0.008, P (gper1) = 0.002).
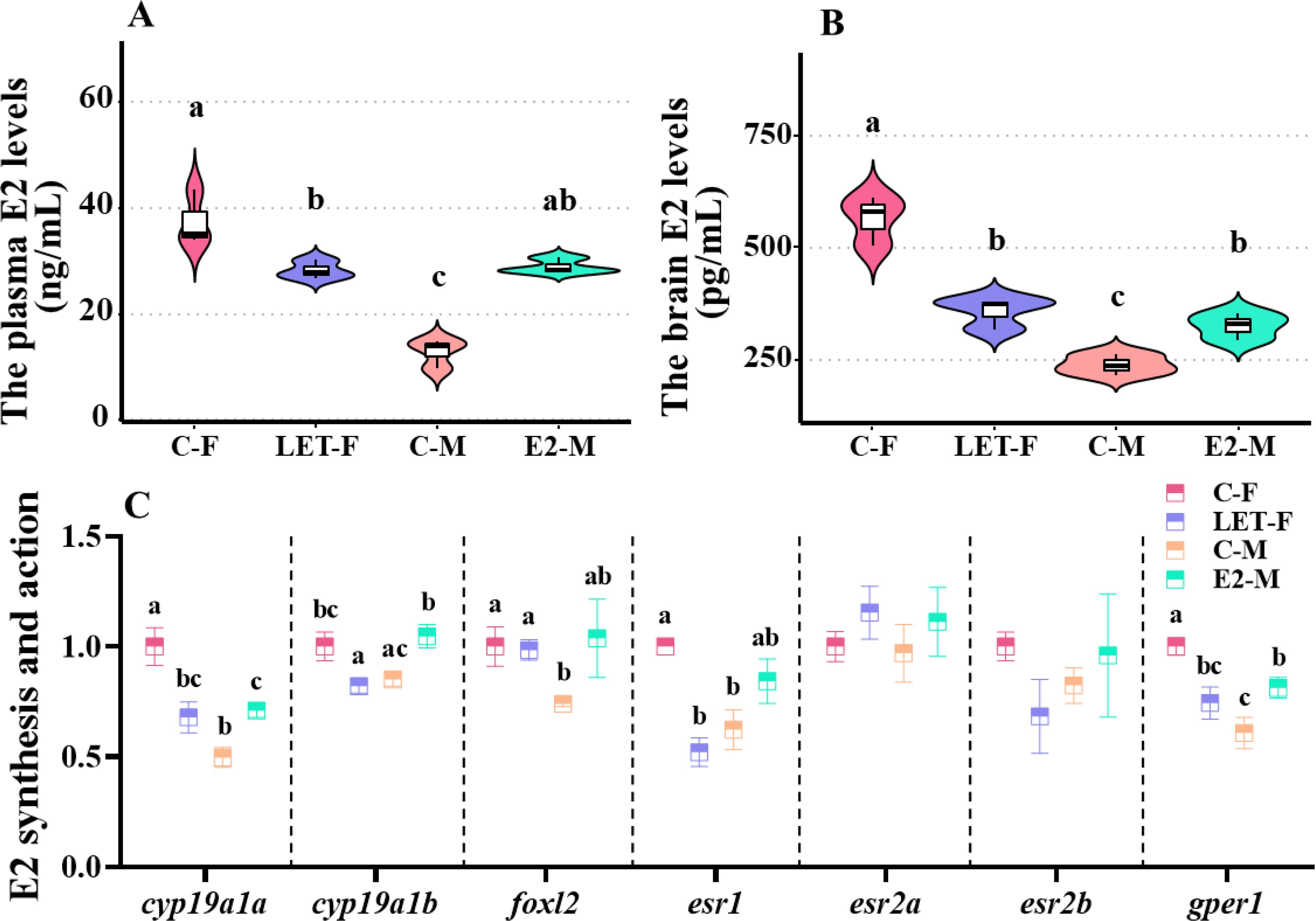
Figure 2. Plasma E2 levels and expressions of related genes in the brain. C-F, control-female (solvent, acetone); C-M, control-male (solvent, acetone); LET-F, letrozole-female (6 mg/g Letrozole); E2-M, E2-male (200 ng/g E2). (A) plasma E2 levels; (B) brain E2 levels; (C) expressions of genes responsible for E2 synthesis and action. Different letters on boxplots in each figure represent significant differences between groups (a, b, and c; P < 0.05). N = 3.
The plasma and brain E2 levels in LET-F fish were significantly lower than those in C-F fish (Figure 2A, P (plasma E2) < 0.001; Figure 2B, P (brain E2) = 0.007) but were still significantly higher than those in C-M fish (Figure 2A, P (plasma E2) < 0.001; Figure 2B, P (brain E2) = 0.005). Furthermore, compared with the C-F fish, the expression levels of cyp19a1a, cyp19a1b, esr1, and gper1 in the brain were significantly downregulated (P (cyp19a1a) = 0.006, P (cyp19a1b) = 0.028, P (esr1) = 0.002, P (gper1) = 0.016), whereas the expression levels of foxl2, esr2a, and esr2b did not show significant changes (Figure 2C, P > 0.05).
The plasma and brain levels of E2 in E2-M fish were significantly higher than those in C-M fish (Figure 2A, P (plasma E2) < 0.001; Figure 2B, P (brain E2) = 0.021). Moreover, the plasma E2 levels in E2-M fish did not show significant changes compared with those in C-F fish (Figure 2A, P > 0.05), whereas the brain E2 levels in E2-M fish were significantly lower than those in C-F fish (Figure 2B, P = 0.01). In comparison to the C-M fish, apart from the significant upregulation of cyp19a1a (P = 0.041), cyp19a1b (P = 0.02) and gper1 (P = 0.041), the mRNA levels of the other genes investigated in the E2-M fish were not significantly different (Figure 2C, P > 0.05).
3.2 Effects of drug treatments on anxiety responses in zebrafish
Compared with C-F fish, the latency to enter the upper layer (Figure 3A, P < 0.001) and the total duration spent in the lower layer were significantly lower in C-M fish (Figure B, P < 0.001).
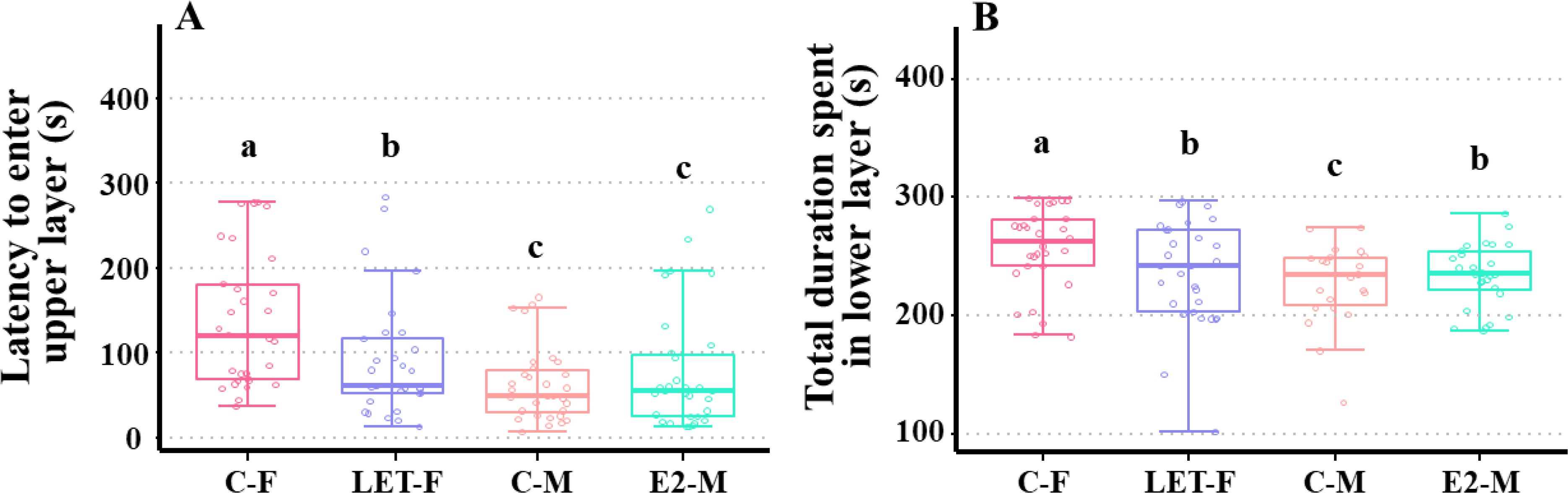
Figure 3. Effects of drug treatments on anxiety response in zebrafish. C-F, control-female (solvent, acetone); C-M, control-male (solvent, acetone); LET-F, letrozole-female (6 mg/g Letrozole); E2-M, E2-male (200 ng/g E2). (A) latency to enter upper layer; (B) total duration spent in lower layer. Different letters on boxplots in each figure represent significant differences between groups (a, b, and c; P < 0.05). N = 45.
Compared with C-F fish, LET-F fish showed significantly decreased latency before entering the upper layer (P = 0.003), but this duration was still significantly higher than that of the C-M fish (Figure 3A, P = 0.012). Similarly, the total duration spent in the lower layer was significantly reduced in LET-F fish (P = 0.026) compared with that of C-F fish, and the duration time was significantly longer than that of C-M fish (Figure 3B, P = 0.002).
After E2 treatment, the total duration spent in the lower layer was significantly increased in E2-M fish compared with C-M fish (P = 0.006), and it was significantly shorter than that of C-F fish (Figure 3B, P = 0.012). There was no significant change in the latency to enter the upper layer between E2-M and C-M fish (Figure 3A, P > 0.05).
3.3 Plasma cortisol levels and expressions of genes of the HPI/cortisol pathway
Plasma cortisol levels were significantly higher in C-F fish than in C-M fish (P < 0.001). Compared with C-F fish, the plasma cortisol levels were significantly lower in the LET-F fish (P < 0.001), with no significant difference compared with those in C-M fish (P > 0.05). In contrast, the plasma cortisol levels in E2-M fish were significantly higher than those in C-M fish (P = 0.02) and remained significantly lower than those in C-F fish (Figure 4A, P = 0.005).
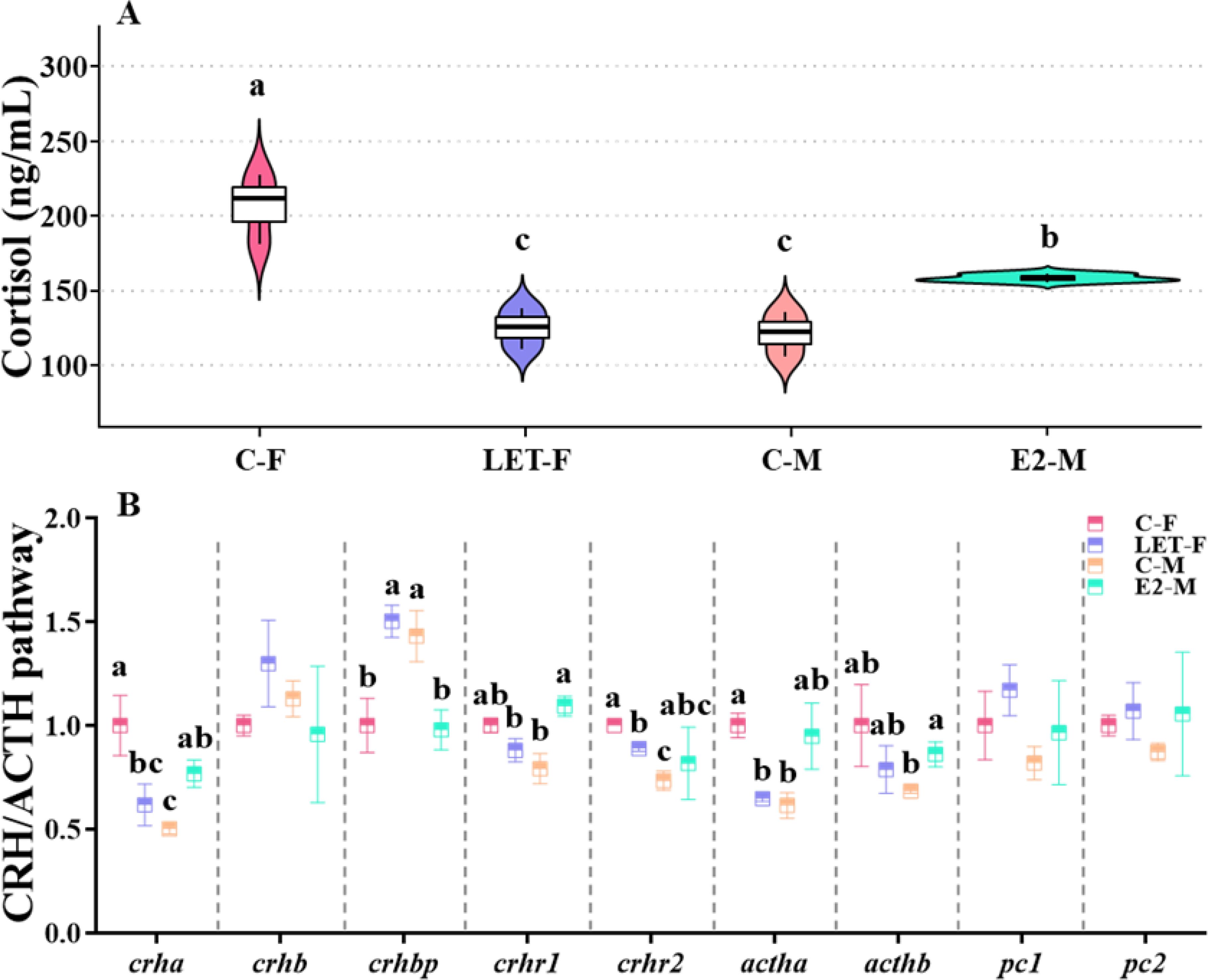
Figure 4. Effects of drug treatments on the plasma cortisol levels and relative mRNA levels of genes in CRH/ACTH pathway of zebrafish brain. C-F, control-female (solvent, acetone); C-M, control-male (solvent, acetone); LET-F, letrozole-female (6 mg/g Letrozole); E2-M, E2-male (200 ng/g E2). (A) plasma cortisol levels; (B) relative mRNA levels of genes in CRH/ACTH pathway. Different letters on boxplots in each figure represent significant differences between groups (a, b, and c; P < 0.05). N = 3.
The expression of crha, crhr2, and actha was significantly upregulated in the brains of C-F fish compared to that in C-M fish (P (crha) = 0.006, P (crhr2) = 0.008, P (actha) = 0.017), whereas the expression of crhbp was significantly downregulated (P = 0.023). Compared to C-F fish, the expression levels of crha, crhr2, and actha were significantly suppressed in LET-F fish (P (crha) = 0.022, P (crhr2) = 0.027, P (actha) = 0.024), whereas the expression of crhbp was significantly upregulated (P = 0.011). In contrast, compared to the C-M fish, the mRNA levels of crha, crhr1, and acthb were significantly increased in E2-M fish (P (crha) = 0.02, P (crhr1) = 0.004, P (acthb) = 0.043), whereas the expression of crhbp was significantly decreased (Figure 4B, P = 0.019).
3.4 Brain 5-HT levels and expressions of genes of the 5-HT pathway
Brain 5-HT levels in C-F fish were significantly lower than those in C-M fish (P = 0.004). Compared with C-F fish, brain 5-HT levels in LET-F fish were significantly higher (P = 0.006) and were not significantly different from those in C-M fish (P > 0.05). In contrast, brain 5-HT levels in E2-M fish were significantly lower than those in C-M fish (P = 0.024), with no significant difference compared with those in C-F fish (Figure 5A, P > 0.05).
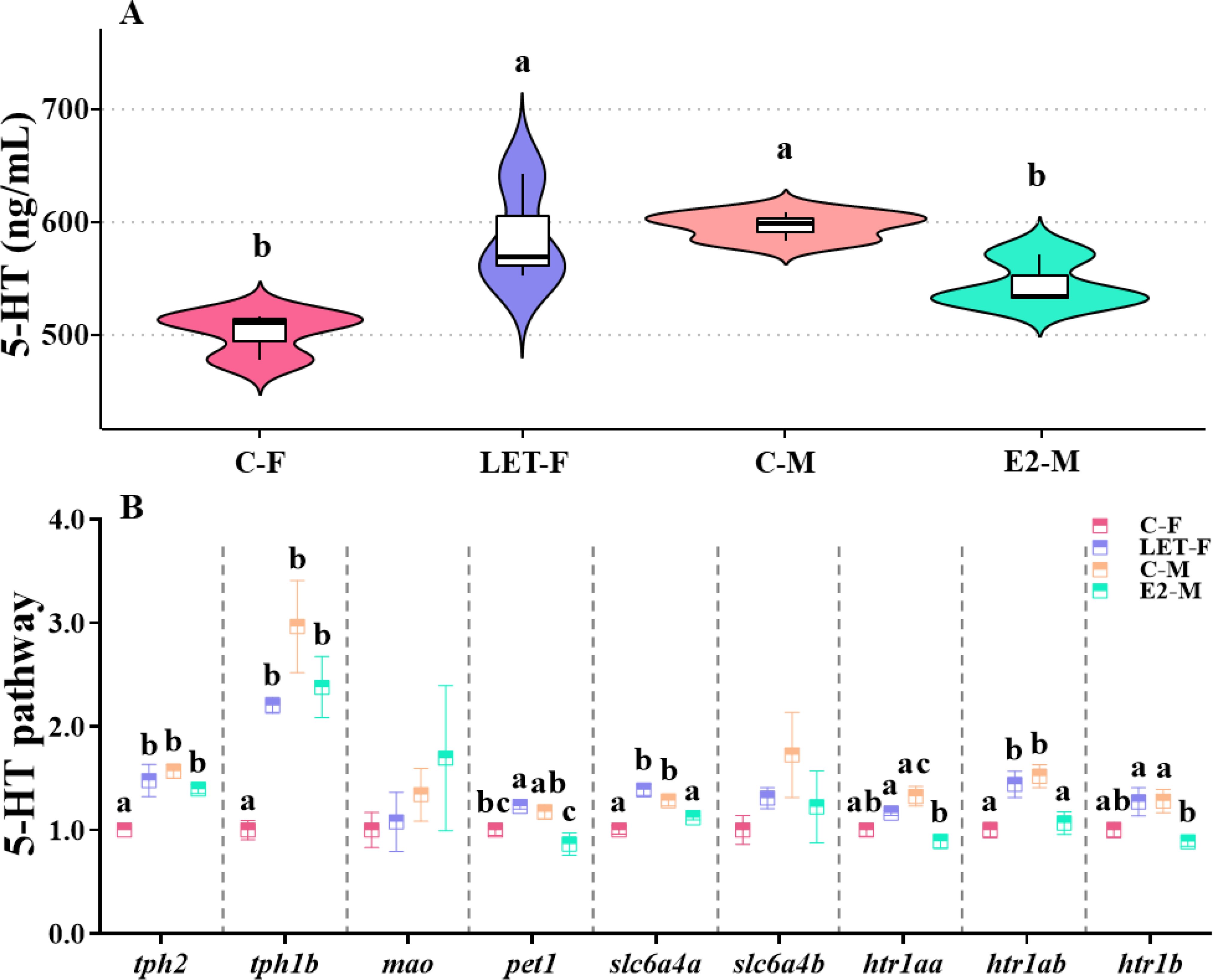
Figure 5. Effects of drug treatments on the 5-HT levels and relative mRNA levels of genes in 5-HT pathway of zebrafish brain. C-F, control-female (solvent, acetone); C-M, control-male (solvent, acetone); LET-F, letrozole-female (6 mg/g Letrozole); E2-M, E2-male (200 ng/g E2). (A) brain 5-HT levels; (B) relative mRNA levels of genes in 5-HT pathway. Different letters on boxplots in each figure represent significant differences between groups (a, b, and c; P < 0.05). N = 3.
Moreover, the mRNA levels of tph2, tph1b, slc6a4a, and htr1ab in the brains of C-F fish were significantly lower than those in the brains of C-M fish (P (tph2) = 0.002, P (tph1b) < 0.01, P (slc6a4a) = 0.002, P (htr1ab) = 0.009). Compared with C-F fish, the mRNA levels of tph2, tph1b, pet1, slc6a4a, and htr1ab were significantly upregulated in LET-F fish (P (tph2) = 0.005, P (tph1b) = 0.014, P (pet1) = 0.047, P (slc6a4a) < 0.001, P (htr1ab) = 0.019). In contrast, compared with C-M fish, the expression levels of pet1, slc6a4a, htr1aa, htr1ab, and htr1b in the brains of E2-M fish were significantly down-regulated (Figure 5B, P (pet1) = 0.013, P (slc6a4a) = 0.03, P (htr1aa) = 0.002, P (htr1ab) = 0.017, P (htr1b) = 0.022).
3.5 Brain DA levels and expressions of genes of the DA axis pathway
Brain DA levels in C-F fish were significantly lower than those in C-M fish (P = 0.002). Compared to C-M fish, brain DA levels in LET-F fish were significantly higher (P = 0.012), but were not significantly different from those in C-M fish (P > 0.05). In contrast, compared to C-M fish, the brain DA levels in E2-M fish were significantly inhibited (P = 0.045), but remained significantly higher than those of the C-F fish (Figure 6A, P = 0.013).
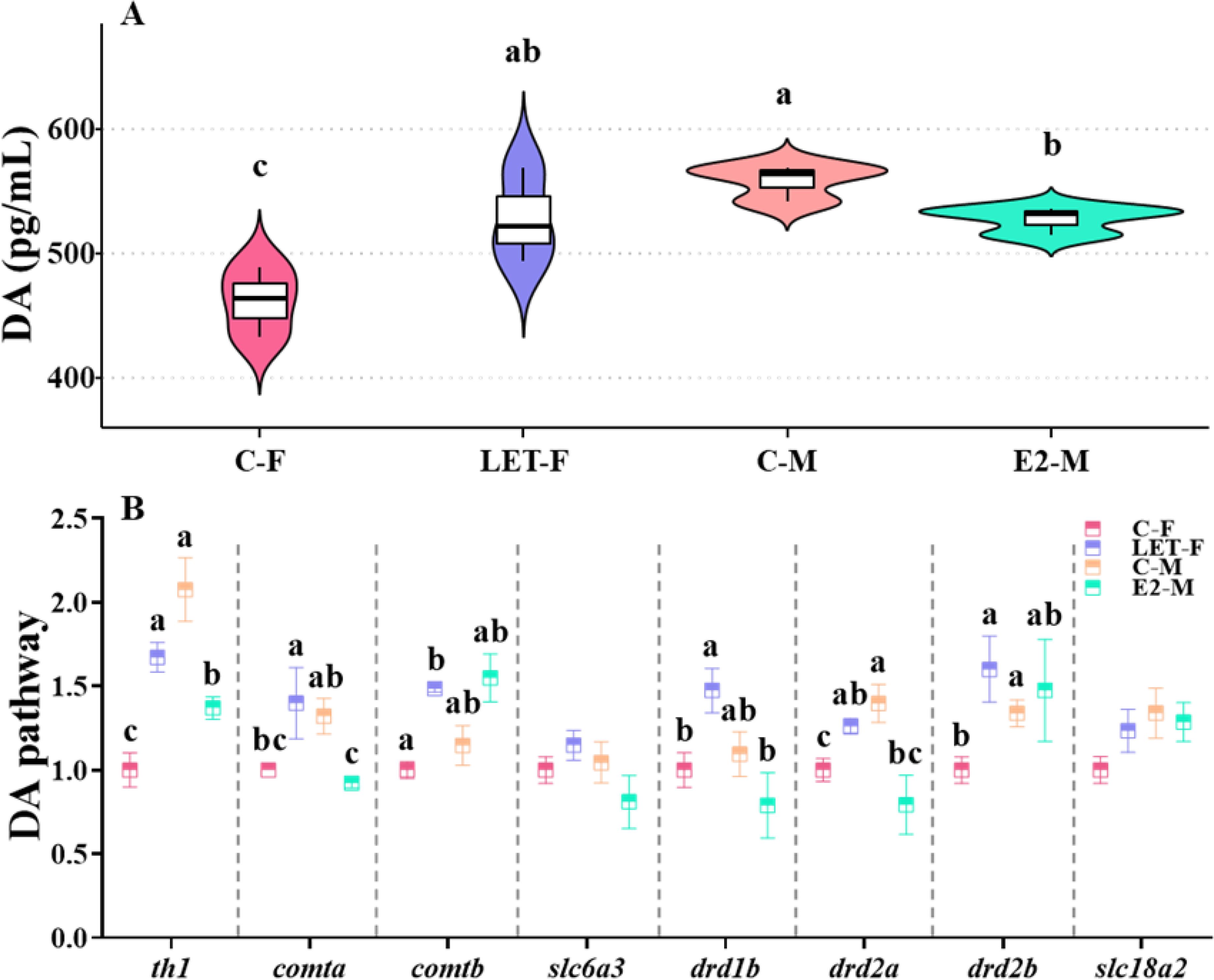
Figure 6. Effects of drug treatments on the DA levels and relative mRNA levels of genes in DA pathway of zebrafish brain. C-F, control-female (solvent, acetone); C-M, control-male (solvent, acetone); LET-F, letrozole-female (6 mg/g Letrozole); E2-M, E2-male (200 ng/g E2). (A) brain DA levels; (B) relative mRNA levels of genes in DA pathway. Different letters on boxplots in each figure represent significant differences between groups (a, b, and c; P < 0.05). N = 3.
Furthermore, the mRNA levels of th1, drd2a, and drd2b were significantly lower in the C-F fish than in the C-M fish (P (th1) < 0.001, P (drd2a) = 0.037, P (drd2b) = 0.04). Compared to C-F fish, the mRNA levels of th1, comta, comtb, drd1b, drd2a and drd2b were significantly increased in LET-F fish (P (th1) = 0.004, P (comta) = 0.046, P (comtb) = 0.008, P (drd1b) = 0.049, P (drd2a) = 0.033, P (drd2b) = 0.048). In contrast, compared with C-M fish, the expression of th1, comta, and drd2a in the brains of E2-M fish was significantly lower (Figure 6B, P (th1) = 0.03, P (comta) = 0.046, P (drd2a) = 0.005).
4 Discussion
4.1 The role of endogenous E2 in anxiety responses of zebrafish
Based on our results, there was a significant sexual dimorphism in anxiety responses in zebrafish, with females exhibiting a more intense anxiety response than males. Furthermore, reducing endogenous E2 levels in females decreased anxiety, whereas increasing endogenous E2 levels in males exacerbated anxiety. These findings suggest that endogenous E2 plays a pivotal role in the anxiety response in zebrafish.
Anxiety is a stress reaction regulated by the neuroendocrine system, which is important for animal survival (Berridge and Kringelbach, 2008; Russart and Nelson, 2018). Previous research has elucidated pronounced universal sexual dimorphic anxiety responses in vertebrates (Bland et al., 2005; Weiser et al., 2008; Ter Horst et al., 2012; Wallace et al., 2020; Loughery et al., 2021). This sexually dimorphic anxiety response typically manifests as more intense anxiety reactions or a greater susceptibility to anxiety in females than in males. In the present study, female zebrafish exhibited a more intense anxiety response than male zebrafish, which is consistent with previous study results (Amar and Ramachandran, 2023; Xiang et al., 2023). Accordingly, the neuroendocrine system that regulates anxiety responses also showed distinct sexual dimorphism, with higher plasma cortisol levels and lower brain 5-HT/DA levels in females, and the expression levels of related pathway genes followed a similar trend. These findings suggest that sexual dimorphic anxiety responses are highly conserved among vertebrates, indicating the pivotal ecological significance of this dimorphism in vertebrates.
In zebrafish, adult females are typically larger than males because of differences in gamete size and nutritional requirements (Yang et al., 2016), which increases their visibility to predators and leads to higher alertness to ensure survival rates (Moretz et al., 2007). In contrast, male zebrafish are smaller and dominate reproductive activities, often being more exploratory and aggressive in discovering, contesting, and defending spawning areas favored by females (Paull et al., 2010; Baatrup and Henriksen, 2015; Conradsen and McGuigan, 2015; Shontz et al., 2018). This may explain why male zebrafish exhibit lower levels of anxiety than females. Although wild-type zebrafish are highly domesticated laboratory strains, our results are consistent with wild-caught males being “bolder” than females (Dahlbom et al., 2011). More intense anxiety responses in female zebrafish can help them remain vigilant and enhance survival and reproductive success rates, whereas lower anxiety levels in male zebrafish can aid them in exploring new environments and improving reproductive success. This sexual dimorphism in the anxiety response of zebrafish is beneficial for the stability and continuation of the population.
Numerous studies have confirmed that sexual dimorphisms in animal phenotypes largely attributed to differences in sex hormones (such as estrogens and androgens) (Gegenhuber et al., 2022; Zhang et al., 2022). Thus, the sexual dimorphic anxiety response in zebrafish is speculated to be closely related to differences in sex hormone levels in both the nervous and endocrine systems (Gegenhuber et al., 2022), especially with regard to E2 (Diotel et al., 2013). Endogenous E2 in zebrafish is regulated by aromatases and is associated with the expression of cyp19a1a, in gonads, and cyp19a1b, in radial glial cells of the brain (Pellegrini et al., 2005; Diotel et al., 2010). Our data showed that both the plasma and brain E2 levels were significantly higher in female than in male zebrafish. Compared to males, the expression of cyp19a1a and foxl2 (positive regulatory factors of aromatase) was markedly higher in female fish, whereas the expression of cyp19a1b and other receptors did not differ. Additionally, the expression levels of estrogen receptors (esr1 and gper1) were higher in the female brain, suggesting a greater estrogenic effect in females. Previous studies investigated sexual dimorphism in the expression of aromatase during the developmental and reproductive periods in zebrafish, and was suggested that this sexual dimorphism influences sex differentiation and a range of sexually dimorphic traits in zebrafish (Kallivretaki et al., 2007; Santos et al., 2008). In the present study, no significant differences in the expression of cyp19a1b were observed between the brains of adult male and female zebrafish, which may be related to their reproductive maturity. Additionally, an intriguing study suggested that androgens may upregulate the expression of cyp19a1b, potentially explaining the lack of significant sexual dimorphism in cyp19a1b expression in the zebrafish brain (Mouriec et al., 2009). Based on these results, E2 levels may be closely associated with sexually dimorphic anxiety responses in zebrafish.
Previous studies have found that changes in endogenous E2 levels are closely associated with alterations in anxiety responses. However, these studies have primarily focused on rodents, with limited research on fish (Ossenkopp et al., 2005; Ellis et al., 2023). In the present study, LET treatment significantly reduced E2 levels in the plasma and brain of female zebrafish, concurrently downregulating the expression of genes associated with E2 synthesis (cyp19a1a and cyp19a1b) and estrogen receptor genes (esr1 and gper1) in the brain. As an aromatase inhibitor, the inhibitory effect of LET on zebrafish E2 levels was highly consistent with previous findings (Adhikari et al., 2024; Zhang et al., 2024). In contrast, E2 treatment significantly increased E2 levels in the brain and plasma of male zebrafish, thereby upregulating the expression of cyp19a1a, cyp19a1b, and gper1. Interestingly, the anxiety response in LET-F fish significantly diminished, whereas that in E2-M fish was significantly enhanced. These results imply a pronounced positive correlation of E2 levels with anxiety in zebrafish, consistent with the findings of previous rodent studies (Flores et al., 2020). Fluctuations in E2 levels may induce changes in the expression levels of estrogen receptors, thereby maintaining homeostasis. This effect is generally considered to be a form of negative regulatory mechanism (Christian et al., 2008; Shi et al., 2013). However, the expression of estrogen receptors (primarily esr1 and gper1), has been shown to be stimulated by E2, suggesting a possible positive correlation between E2 and estrogen receptors (Menuet et al., 2004; Liu et al., 2017). Furthermore, E2 signaling occurs via both genomic and non-genomic pathways, with esr1, esr2a, and esr2b participating in the genomic pathway, while gper1 is involved in the non-genomic pathways (Shi et al., 2013; Liu and Shi, 2015). Combining previous research with our results, endogenous E2 may participate in the regulation of anxiety responses in zebrafish through genomic and non-genomic pathways via a potential positive regulatory mechanism (Liu and Shi, 2015).
The close relationship observed between endogenous E2 levels and anxiety responses in zebrafish and mammals suggests that the role of E2 in anxiety responses may be conserved among vertebrates to some extent (Altemus et al., 2014; de Abreu et al., 2021; Ellis et al., 2023).
4.2 The pathways through which endogenous E2 regulates anxiety responses in zebrafish
A substantial body of previous research indicates that anxiety responses in fish are regulated by a range of neuroendocrine systems, including the 5-HT and DA systems, as well as the HPI axis (Wommack and Delville, 2007; Berridge and Kringelbach, 2008; Kalueff et al., 2012; Jia and Pittman, 2015; Oh et al., 2020; Tu et al., 2020). Concurrently, E2 is synthesized primarily through two pathways in vertebrates: release by the hypothalamic-pituitary-gonadal axis and local release in the brain, indicating that E2 signaling is regulated by both neuronal and endocrine systems (Hamilton et al., 2017). A number of studies have been focusing on the interactions between E2 and the neuroendocrine system, such as its effects on the HPA axis and the serotoninergic system (Weiser and Handa, 2009; Ulhaq and Kishida, 2018). Some evidence suggests that E2 may cause changes in animal emotional activities by affecting or modulating the neuroendocrine system (Ter Horst et al., 2009; Hernández-Hernández et al., 2019).
Cortisol, synthesized under the influence of the HPA/HPI axis, plays a crucial role in the modulation of stress responses in animals (Berridge and Kringelbach, 2008; Ghisleni et al., 2012; Duboué et al., 2017). Interestingly, the plasma cortisol levels in many animal species exhibit significant sexual dimorphism, typically characterized by higher levels in females compared to males (Bangasser and Valentino, 2014; Culbert et al., 2021). This sexual dimorphism in cortisol levels corresponds with the sexual dimorphism observed in anxiety and E2 levels. Numerous studies have revealed the positive regulatory effect of E2 on cortisol production in animals (Weiser et al., 2008; Weiser and Handa, 2009). In the current study, compared with C-F fish, LET-F fish showed a significant decrease in plasma cortisol levels, corresponding to a reduction in anxiety levels. In contrast, E2-M fish exhibited a sharp increase in plasma cortisol levels compared with C-M fish, which corresponded to an exacerbation of anxiety responses. Changes in plasma cortisol levels positively correlated with changes in E2 levels in the plasma and brain. In addition, the expression of a series of genes involved in the regulation of cortisol synthesis and metabolism in the HPI axis of male and female fish treated with the drugs exhibited corresponding changes. These changes were consistent with alterations in plasma cortisol levels and anxiety responses observed in male and female fish after treatment. Gonadal steroid hormones play an essential role in regulating the function of the HPI/HPA axis (Suzuki et al., 2001; Zhang et al., 2009). In rodents, E2 supplementation can counteract the decrease in corticosterone and ACTH levels induced by stress in ovariectomized females (Heck and Handa, 2019). Additionally, E2 therapy in gonadectomized male rats increased stress-induced levels of c-fos mRNA, CRH hnRNA, and corticosterone, suggesting that E2 regulates anxiety by stimulating the HPA axis (Weiser and Handa, 2009). Our results, combined with these previous findings, strongly suggest that E2 regulates anxiety responses in zebrafish via the HPI/cortisol axis and that this action may be conserved in vertebrates (Weiser and Handa, 2009; Heck and Handa, 2019).
As an important inhibitory neurotransmitter, 5-HT alleviates anxiety responses in fish (Kalueff et al., 2012; Tu et al., 2020). Notably, the 5-HTergic system exhibits a distinct sexual dimorphism in vertebrates, characterized by a higher rate of 5-HTergic synthesis in males compared to females (Jones and Lucki, 2005; Gressier et al., 2016). In our results, compared with C-F fish, the brain 5-HT levels of LET-F fish were significantly higher, whereas those of E2-M fish were significantly lower than those of C-M fish. Furthermore, the expression of genes related to the 5-HT pathway (including its synthesis, action, and degradation) also changed. These alterations corresponded with the changes in anxiety responses observed in both female and male fish post-treatment and were negatively correlated with changes in E2 levels (in the plasma and/or brain). Similarly, a significant negative correlation between E2 and 5-HT levels has been observed in tilapia (Oreochromis mossambicus) and zebrafish larvae (Tsai et al., 2000; Ulhaq and Kishida, 2018). In the present study, the expression levels of the receptors for the two pathways of E2 action (esr1 and gper1) exhibited a significant inverse correlation with the levels of 5-HT. A close association between estrogen receptors and 5-HTergic neurons in zebrafish has been postulated (Lillesaar, 2011). E2 may regulate the 5-HT system in the brain by interacting with estrogen response elements or other transcription factors, thereby modulating the mood (Hernández-Hernández et al., 2019). These findings imply that higher levels of anxiety in females are closely associated with the interaction between endogenous E2 and 5-HT, with estrogen receptors potentially playing a key role in modulating this interaction, thereby suppressing 5-HT levels in the brain and stimulating a stronger anxiety response.
Similarly, DA, a neurotransmitter associated with the reward mechanism, is also closely related to the regulation of anxiety responses in fish (Jia and Pittman, 2015), and the DAergic system in males tends to be more active than that in females (Orendain-Jaime et al., 2016; Zachry et al., 2021), which is negatively correlated with sexual dimorphism in anxiety levels and E2 concentrations.
Consistent with 5-HT, DA exhibits a complex inter-regulatory relationship with E2 in mammals (Dluzen, 2005; Petersen et al., 2021; Bendis et al., 2024). In the present study, the brain DA levels of LET-F fish increased dramatically compared to C-F fish, whereas the brain DA levels in E2-M fish decreased significantly compared with those in C-M fish. These changes were also negatively correlated with changes in E2 (in the plasma and/or brain), suggesting that endogenous E2 may negatively regulate DA levels in the zebrafish brain. Moreover, the expression of genes related to the DA pathway (including synthesis, action, and degradation) in male and female fish treated with the drugs showed corresponding changes. These changes were consistent with the alterations in plasma DA levels and anxiety responses observed in male and female fish after treatment. Similar to our results, an inhibitory effect of E2 on the DA pathway has been observed in rainbow trout (Oncorhynchus mykiss), where a decrease in the expression of the DA receptor DR2A was correlated with elevated plasma E2 levels (Crago and Schlenk, 2015; Magnuson et al., 2021). These results complicate the mode of action of E2 on DA pathways. Nonetheless, our results provide a new perspective on the action of E2 on DAergic neurons, suggesting that E2 elicits anxiety responses by inhibiting the DAergic pathway.
Interestingly, the activation of the HPI axis has been demonstrated to be closely associated with the activity of 5-HT and DA neurons, and the 5-HTergic and DAergic systems also play significant roles in the function of the HPI/HPA axis (Contesse et al., 2000; Porter et al., 2004; McArthur et al., 2005; Fink and Göthert, 2007). These findings illustrate the complex relationship between the HPI axis and 5-HTergic/DAergic systems. Endogenous E2 may participate in the regulation of anxiety responses in zebrafish via complex interactions between the HPI axis and the 5-HT and DA systems.
In summary, this study confirmed that zebrafish exhibit a sexually dimorphic anxiety response, with females showing higher levels of anxiety than males. The anxiety responses elicited by artificially altering endogenous E2 levels, along with the associated changes in the HPI/cortisol axis and the 5-HT/DA system, suggest that endogenous E2 influences the anxiety of zebrafish by positively modulating the HPI/cortisol axis and negatively modulating the 5-HT/DA pathways (Figure 7). The present study revealed how E2 modulates anxiety and the associated neurochemical pathways in fish, offering new insights into the impact of sex hormones on anxiety in animals.
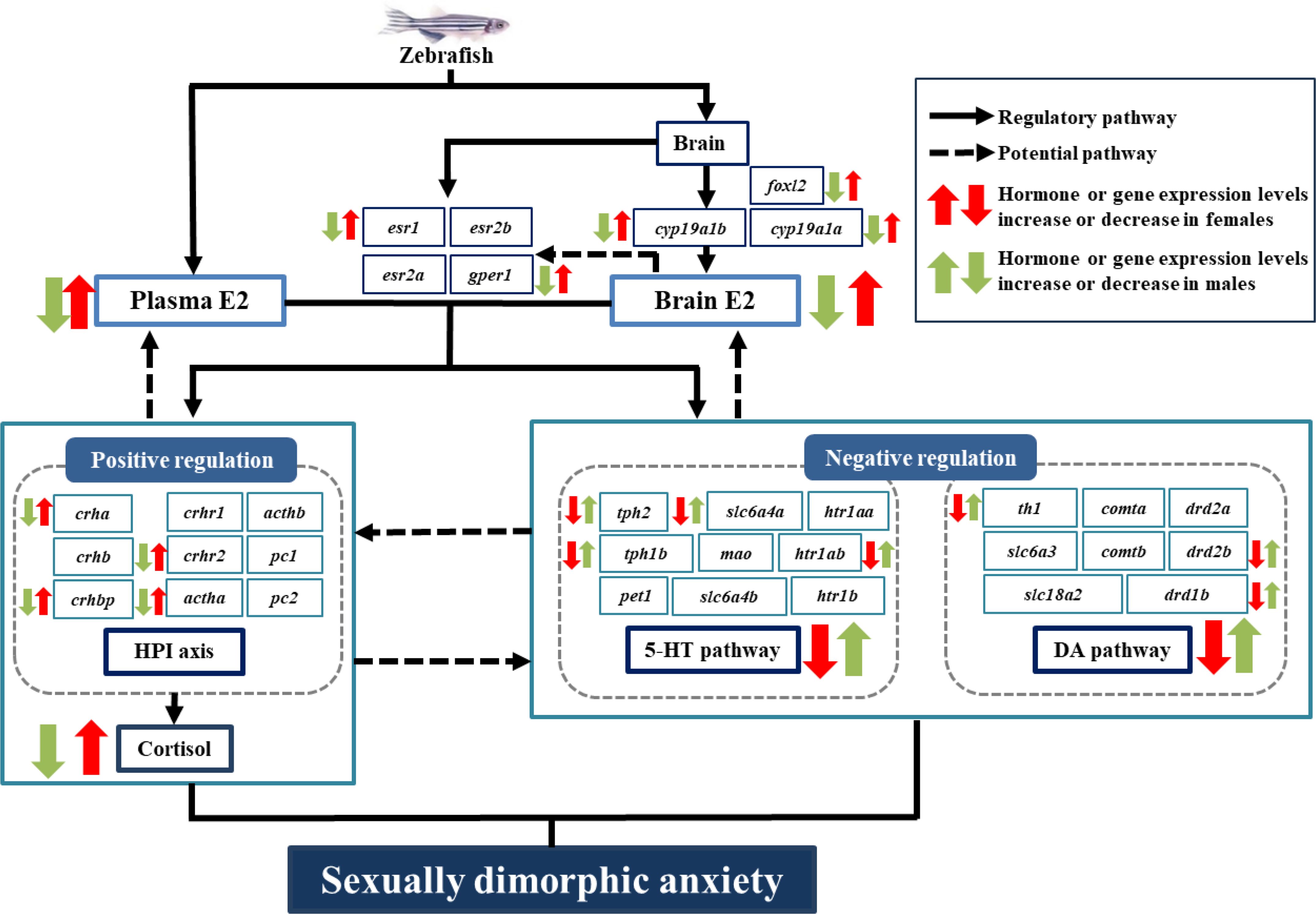
Figure 7. Schematic diagram of endogenous E2 regulating sexually dimorphic anxiety responses in zebrafish via the HPI axis and 5-HT/DA pathways. Black arrows, regulatory pathways that E2 regulates sexually dimorphic anxiety of zebrafish via modulating HPI/cortisol axis and 5-HT/DA systems respectively, as demonstrated in this study; dashed arrows, potential pathways that HPI/cortisol axis and 5-HT/DA systems may act on the effect of E2 through negative feedback pathways and act on each other respectively, as revealed by previous studies.
Data availability statement
The original contributions presented in the study are included in the article/supplementary material. Further inquiries can be directed to the corresponding author.
Ethics statement
The animal study was approved by the ethics committee of Chongqing Normal University (Approval No. CKLCUFF20240701-02). The study was conducted in accordance with the local legislation and institutional requirements.
Author contributions
HT: Data curation, Investigation, Methodology, Writing – original draft. YZ: Data curation, Investigation, Methodology, Writing – original draft. YS: Data curation, Writing – review & editing. QC: Conceptualization, Methodology, Writing – review & editing. ZL: Conceptualization, Funding acquisition, Project administration, Supervision, Writing – review & editing.
Funding
The author(s) declare financial support was received for the research, authorship, and/or publication of this article. This work was funded by the Chongqing Research Program of Basic Research and Frontier Technology (cstc2020jcyj-msxmX0805, 2020); The Enhanced Project of scientific research platform in Fuyang Normal University (FSKFKT021D,2022).
Acknowledgments
We appreciate the pilot work conducted by Xin Tu, Cheng-Ting Xie and Mei-Ling Tan for this study.
Conflict of interest
The authors declare that the research was conducted in the absence of any commercial or financial relationships that could be construed as a potential conflict of interest.
Generative AI statement
The author(s) declare that no Generative AI was used in the creation of this manuscript.
Publisher’s note
All claims expressed in this article are solely those of the authors and do not necessarily represent those of their affiliated organizations, or those of the publisher, the editors and the reviewers. Any product that may be evaluated in this article, or claim that may be made by its manufacturer, is not guaranteed or endorsed by the publisher.
References
Adhikari M., Biswas C., Mazumdar P., Sarkar S., Pramanick K. (2024). Evaluating the potential of daily intake of polystyrene microplastics via drinking water in inducing PCOS and its ovarian fibrosis progression using female zebrafish. NanoImpact 34, 100507. doi: 10.1016/j.impact.2024.100507
Albert P. R., Vahid-Ansari F., Luckhart C. (2014). Serotonin-prefrontal cortical circuitry in anxiety and depression phenotypes: pivotal role of pre-and post-synaptic 5-HT1A receptor expression. Front. Behav. Neurosci. 8, 199. doi: 10.3389/fnbeh.2014.00199
Alderman S. L., Bernier N. J. (2009). Ontogeny of the corticotropin-releasing factor system in zebrafish. Gen. Comp. Endocrinol. 164, 61–69. doi: 10.1016/j.ygcen.2009.04.007
Altemus M., Sarvaiya N., Epperson C. N. (2014). Sex differences in anxiety and depression clinical perspectives. Front. Neuroendocrinol. 35, 320–330. doi: 10.1016/j.yfrne.2014.05.004
Amar A., Ramachandran B. (2023). Environmental stressors differentially modulate anxiety-like behavior in male and female zebrafish. Behav. Brain Res. 450, 114470. doi: 10.1016/j.bbr.2023.114470
Asher M., Asnaani A., Aderka I. M. (2017). Gender differences in social anxiety disorder: A review. Clin. Psychol. Rev. 56, 1–12. doi: 10.1016/j.cpr.2017.05.004
Baatrup E., Henriksen P. G. (2015). Disrupted reproductive behavior in unexposed female zebrafish (Danio rerio) paired with males exposed to low concentrations of 17α-ethinylestradiol (EE2). Aquat. Toxicol. 160, 197–204. doi: 10.1016/j.aquatox.2015.01.020
Bangasser D. A., Valentino R. J. (2014). Sex differences in stress-related psychiatric disorders: neurobiological perspectives. Front. Neuroendocrinol. 35, 303–319. doi: 10.1016/j.yfrne.2014.03.008
Bellipanni G., Rink E., Bally-Cuif L. (2002). Cloning of two tryptophanhydroxylase genes expressed in the diencephalon of the developing zebrafish brain. Mech. Dev. 119, 215–220. doi: 10.1016/S0925-4773(03)00119-9
Bendis P. C., Zimmerman S., Onisiforou A., Zanos P., Georgiou P. (2024). The impact of estradiol on serotonin, glutamate, and dopamine systems. Front. Neurosci. 18, 1348551. doi: 10.3389/fnins.2024.1348551
Berridge K. C., Kringelbach M. L. (2008). Affective neuroscience of pleasure: reward in humans and animals. Psychopharmacology 199, 457–480. doi: 10.1007/s00213-008-1099-6
Bland S. T., Schmid M. J., Der-Avakian A., Watkins L. R., Spencer R. L., Maier S. F. (2005). Expression of c-fos and BDNF mRNA in subregions of the prefrontal cortex of male and female rats after acute uncontrollable stress. Brain Res. 1051, 90–99. doi: 10.1016/j.brainres.2005.05.065
Borrow A., Handa R. J. (2017). Estrogen receptors modulation of anxiety-like behavior. Vitamins hormones 103, 27–52. doi: 10.1016/bs.vh.2016.08.004
Cachat J. M., Canavello P. R., Elkhayat S. I., Bartels B. K., Hart P. C., Elegante M. F., et al. (2011). Video-aided analysis of zebrafish locomotion and anxiety-related behavioral responses. In: Kalueff A. V., Cachat J. M. editor. Zebrafish neurobehavioral Protoc. New York: Humana Press, 1–14.
Che W., Yang M., Cheng Y., Wu M., Lan Y., Zhang H. (2019). Arsenic induces gender difference of estrogen receptor in AECII cells from ICR fetal mice. Toxicol. Vitro 56, 133–140. doi: 10.1016/j.tiv.2019.01.014
Christian C. A., Glidewell-Kenney C., Jameson J. L., Moenter S. M. (2008). Classical estrogen receptor α signaling mediates negative and positive feedback on gonadotropin-releasing hormone neuron firing. Endocrinology 149, 5328–5334. doi: 10.1210/en.2008-0520
Conradsen C., McGuigan K. (2015). Sexually dimorphic morphology and swimming performance relationships in wild-type zebrafish Danio rerio. J. Fish Biol. 87, 1219–1233. doi: 10.1111/jfb.2015.87.issue-5
Contesse V., Lefebvre H., Lenglet S., Kuhn J.-M., Delarue C., Vaudry H. (2000). Role of 5-HT in the regulation of the brain-pituitary-adrenal axis: effects of 5-HT on adrenocortical cells. Can. J. Physiol. Pharmacol. 78, 967–983. doi: 10.1139/y00-098
Crago J., Schlenk D. (2015). The effect of bifenthrin on the dopaminergic pathway in juvenile rainbow trout (Oncorhynchus mykiss). Aquat. Toxicol. 162, 66–72. doi: 10.1016/j.aquatox.2015.03.005
Culbert B. M., Ligocki I. Y., Salena M. G., Wong M. Y., Hamilton I. M., Aubin-Horth N., et al. (2021). Rank-and sex-specific differences in the neuroendocrine regulation of glucocorticoids in a wild group-living fish. Hormones Behav. 136, 105079. doi: 10.1016/j.yhbeh.2021.105079
Dahlbom S. J., Lagman D., Lundstedt-Enkel K., Sundström L. F., Winberg S. (2011). Boldness predicts social status in zebrafish (Danio rerio). PloS One 6, e23565. doi: 10.1371/journal.pone.0023565
de Abreu M. S., Demin K. A., Giacomini A. C., Amstislavskaya T. G., Strekalova T., Maslov G. O., et al. (2021). Understanding how stress responses and stress-related behaviors have evolved in zebrafish and mammals. Neurobiol. Stress 15, 100405. doi: 10.1016/j.ynstr.2021.100405
Diotel N., Le Page Y., Mouriec K., Tong S.-K., Pellegrini E., Vaillant C., et al. (2010). Aromatase in the brain of teleost fish: expression, regulation and putative functions. Front. Neuroendocrinol. 31, 172–192. doi: 10.1016/j.yfrne.2010.01.003
Diotel N., Vaillant C., Gabbero C., Mironov S., Fostier A., Gueguen M.-M., et al. (2013). Effects of estradiol in adult neurogenesis and brain repair in zebrafish. Hormones Behav. 63, 193–207. doi: 10.1016/j.yhbeh.2012.04.003
Dluzen D. E. (2005). Unconventional effects of estrogen uncovered. Trends Pharmacol. Sci. 26, 485–487. doi: 10.1016/j.tips.2005.08.001
Duboué E. R., Hong E., Eldred K. C., Halpern M. E. (2017). Left habenular activity attenuates fear responses in larval zebrafish. Curr. Biol. 27, 2154–2162.e2153. doi: 10.1016/j.cub.2017.06.017
Egan R. J., Bergner C. L., Hart P. C., Cachat J. M., Canavello P. R., Elegante M. F., et al. (2009). Understanding behavioral and physiological phenotypes of stress and anxiety in zebrafish. Behav. Brain Res. 205, 38–44. doi: 10.1016/j.bbr.2009.06.022
Ellis M. N., Wright M. D., Dennis T. S. (2023). Estradiol administration increases anxiety-like behavior following chronic escalating morphine administration in hormone-replaced ovariectomized female rats. Pharmacol. Biochem. Behav. 227, 173582. doi: 10.1016/j.pbb.2023.173582
Fink K. B., Göthert M. (2007). 5-HT receptor regulation of neurotransmitter release. Pharmacol. Rev. 59, 360–417. doi: 10.1124/pr.59.07103
Flores R. J., Cruz B., Uribe K. P., Correa V. L., Arreguin M. C., Carcoba L. M., et al. (2020). Estradiol promotes and progesterone reduces anxiety-like behavior produced by nicotine withdrawal in female rats. Psychoneuroendocrinology 119, 104694. doi: 10.1016/j.psyneuen.2020.104694
Fuzzen M. L. M., Glen V. D. K., Bernier N. J. (2010). Stirring up new ideas about the regulation of the hypothalamic-pituitary-interrenal axis in zebrafish (Danio rerio). Zebrafish 7, 349–58. doi: 10.1089/zeb.2010.0662
Gegenhuber B., Wu M., Bronstein R., Tollkuhn J. (2022). Gene regulation by gonadal hormone receptors underlies brain sex differences. Nature 606, 153–159. doi: 10.1038/s41586-022-04686-1
Genazzani A. R., Pluchino N., Luisi S., Luisi M. (2007). Estrogen, cognition and female ageing. Hum. Reprod. Update 13, 175–187. doi: 10.1093/humupd/dml042
Ghisleni G., Capiotti K. M., Da Silva R. S., Oses J. P., Piato ÂL, Soares V., et al. (2012). The role of CRH in behavioral responses to acute restraint stress in zebrafish. Prog. Neuropsychopharmacol. Biol. Psychiatry 36, 176–182. doi: 10.1016/j.pnpbp.2011.08.016
Gressier F., Calati R., Serretti A. (2016). 5-HTTLPR and gender differences in affective disorders: a systematic review. J. Affect. Disord. 190, 193–207. doi: 10.1016/j.jad.2015.09.027
Guo M., Wu C. F., Liu W., Yang J. Y., Chen D. (2004). Sex difference in psychological behavior changes induced by long-term social isolation in mice. Prog. Neuropsychopharmacol. Biol. Psychiatry 28, 115–121. doi: 10.1016/j.pnpbp.2003.09.027
Hamilton K. J., Hewitt S. C., Arao Y., Korach K. S. (2017). Estrogen hormone biology. Curr. topics Dev. Biol. 125, 109–146. doi: 10.1016/bs.ctdb.2016.12.005
He W., Zhang S., Qi Z., Liu W. (2024). Unveiling the potential of estrogen: exploring its role in neuropsychiatric disorders and exercise intervention. Pharmacol. Res. 240, 107201. doi: 10.1016/j.phrs.2024.107201
Heck A. L., Handa R. J. (2019). Sex differences in the hypothalamic–pituitary–adrenal axis’ response to stress: an important role for gonadal hormones. Neuropsychopharmacology 44, 45–58. doi: 10.1038/s41386-018-0167-9
Herman J. P., McKlveen J. M., Ghosal S., Kopp B., Wulsin A., Makinson R., et al. (2016). Regulation of the hypothalamic-pituitary-adrenocortical stress response. Compr. Physiol. 6, 603. doi: 10.1002/cphy.c150015
Hernández-Hernández O. T., Martínez-Mota L., Herrera-Pérez J. J., Jiménez-Rubio G. (2019). Role of estradiol in the expression of genes involved in serotonin neurotransmission: implications for female depression. Curr. Neuropharmacology 17, 459–471. doi: 10.2174/1570159X16666180628165107
Hwang J.-A., Park J., Kim J. E., Lee J.-H., Kim H. S. (2022). Estradiol-17 β levels as a tool for sex determination in Farmed Anguilla japonica. Biochem. Biophys. Res. Commun. 634, 108–113. doi: 10.1016/j.bbrc.2022.10.020
Iqbal J., Huang G.-D., Xue Y.-X., Yang M., Jia X.-J. (2024). Role of estrogen in sex differences in memory, emotion and neuropsychiatric disorders. Mol. Biol. Rep. 51, 415. doi: 10.1007/s11033-024-09374-z
Jia M., Pittman J. (2015). Deficits in striatal dopamine and hippocampal serotonin following induction of anxiety/depressive-like behaviors by bisphenol A. Arch. Neurosci. 2, e18555. doi: 10.5812/archneurosci.18555
Jones M. D., Lucki I. (2005). Sex differences in the regulation of serotonergic transmission and behavior in 5-HT receptor knockout mice. Neuropsychopharmacology 30, 1039–1047. doi: 10.1038/sj.npp.1300664
Kallivretaki E., Eggen R. I., Neuhauss S. C., Kah O., Segner H. (2007). The zebrafish, brain-specific, aromatase cyp19a2 is neither expressed nor distributed in a sexually dimorphic manner during sexual differentiation. Dev. Dynamics 236, 3155–3166. doi: 10.1002/dvdy.v236:11
Kalueff A. V., Stewart A. M., Kyzar E. J., Cachat J., Gebhardt M., Landsman S., et al. (2012). Time to recognize zebrafish ‘affective’behavior. Behavior 149, 1019–1036. doi: 10.1163/1568539X-00003030
Kaslin J., Panula P. (2001). Comparative anatomy of the histaminergic and other aminergic systems in zebrafish (Danio rerio). J. Comp. Neurol. 440, 342–377. doi: 10.1002/cne.v440:4
Kassambara A. (2020). ggpubr: 'ggplot2' Based Publication Ready Plots. R package version 0.1. Available at: https://kassambara.r-universe.dev/ggpubr/ggpubr.pdf (Accessed 2025-02-01 february 02, 2025).
Kavaliers M., Choleris E. (2001). Antipredator responses and defensive behavior: ecological and ethological approaches for the neurosciences. Neurosci. Biobehav. Rev. 25, 577–586. doi: 10.1016/S0149-7634(01)00042-2
Kysil E. V., Meshalkina D. A., Frick E. E., Echevarria D. J., Rosemberg D. B., Maximino C., et al. (2017). Comparative analyses of zebrafish anxiety-like behavior using conflict-based novelty tests. Zebrafish 14, 197–208. doi: 10.1089/zeb.2016.1415
Lagranha C. J., Silva T. L. A., Silva S. C. A., Braz G. R. F., da Silva A. I., Fernandes M. P., et al. (2018). Protective effects of estrogen against cardiovascular disease mediated via oxidative stress in the brain. Life Sci. 192, 190–198. doi: 10.1016/j.lfs.2017.11.043
Levin E. D., Bencan Z., Cerutti D. T. (2007). Anxiolytic effects of nicotine in zebrafish. Physiol. Behav. 90, 54–58. doi: 10.1016/j.physbeh.2006.08.026
Li R., Guo W., Lei L., Zhang L., Liu Y., Han J., et al. (2020). Early-life exposure to the organophosphorus flame-retardant tris (1,3-dichloro-2-propyl) phosphate induces delayed neurotoxicity associated with DNA methylation in adult zebrafish. Environ. Int. 134, 105293. doi: 10.1016/j.envint.2019.105293
Lillesaar C. (2011). The serotonergic system in fish. J. Chem. Neuroanat. 41, 294–308. doi: 10.1016/j.jchemneu.2011.05.009
Liu S., Zhang Y., Wang L., Li H., Zhou X. (2020). Carbofuran induces increased anxiety-like behaviors in female zebrafish (Danio rerio) through disturbing dopaminergic/norepinephrinergic system. Chemosphere 253, 126635. doi: 10.1016/j.chemosphere.2020.126635
Liu K.-C., Lau S.-W., Ge W. (2017). Spatiotemporal expression analysis of nuclear estrogen receptors in the zebrafish ovary and their regulation in vitro by endocrine hormones and paracrine factors. Gen. Comp. Endocrinol. 246, 218–225. doi: 10.1016/j.ygcen.2016.12.011
Liu X., Shi H. (2015). Regulation of estrogen receptor α expression in the hypothalamus by sex steroids: implication in the regulation of energy homeostasis. Int. J. Endocrinol. 2015, 949085. doi: 10.1155/2015/949085
Livak K. J., Schmittgen T. D. (2001). Analysis of relative gene expression data using real-time quantitative PCR and the 2– ΔΔCT method. methods 25, 402–408. doi: 10.1006/meth.2001.1262
Loughery J. R., Crowley E., Kidd K. A., Martyniuk C. J. (2021). Behavioral and hypothalamic transcriptome analyses reveal sex-specific responses to phenanthrene exposure in the fathead minnow (Pimephales promelas). Comp. Biochem. Physiol. Part D: Genomics Proteomics 40, 100905. doi: 10.1016/j.cbd.2021.100905
Magnuson J. T., Huff Hartz K. E., Fulton C. A., Lydy M. J., Schlenk D. (2021). Transcriptomic and histopathological effects of bifenthrin to the brain of juvenile rainbow trout (Oncorhynchus mykiss). Toxics 9, 48. doi: 10.3390/toxics9030048
Maximino C., Lima M. G., Batista E., Oliveira K. R. H. M., Herculano A. M. (2015). Interaction between 5-HT1B receptors and nitric oxide in zebrafish responses to novelty. Neurosci. Lett. 588, 54–56. doi: 10.1016/j.neulet.2014.12.049
McArthur S., McHale E., Dalley J., Buckingham J., Gillies G. (2005). Altered mesencephalic dopaminergic populations in adulthood as a consequence of brief perinatal glucocorticoid exposure. J. Neuroendocrinol. 17, 475–482. doi: 10.1111/j.1365-2826.2005.01331.x
Menuet A., Le Page Y., Torres O., Kern L., Kah O., Pakdel F. (2004). Analysis of the estrogen regulation of the zebrafish estrogen receptor (ER) reveals distinct effects of ERalpha, ERbeta1 and ERbeta2. J. Mol. Endocrinol. 32, 975–986. doi: 10.1677/jme.0.0320975
Moretz J. A., Martins E. P., Robison B. D. (2007). Behavioral syndromes and the evolution of correlated behavior in zebrafish. Behav. Ecol. 18, 556–562. doi: 10.1093/beheco/arm011
Mouriec K., Gueguen M.-M., Manuel C., Percevault F., Thieulant M.-L., Pakdel F., et al. (2009). Androgens upregulate cyp19a1b (aromatase B) gene expression in the brain of zebrafish (Danio rerio) through estrogen receptors. Biol. Reprod. 80, 889–896. doi: 10.1095/biolreprod.108.073643
Muralidharan A., Swaminathan A., Poulose A. (2024). Deep learning dives: Predicting anxiety in zebrafish through novel tank assay analysis. Physiol. Behav. 287, 114696. doi: 10.1016/j.physbeh.2024.114696
Norton W. H., Folchert A., Bally-Cuif L. (2008). Comparative analysis of serotonin receptor (HTR1A/HTR1B families) and transporter (slc6a4a/b) gene expression in the zebrafish brain. J. Comp. Neurol. 511, 521–542. doi: 10.1002/cne.v511:4
Oh J., Kim D. H., Kim G.-y., Park E.-J., Ryu J. H., Jung J. W., et al. (2020). Hydrangeae dulcis folium attenuates physical stress by suppressing ACTH-induced cortisol in zebrafish. Chin. J. Integr. Med. 26, 130–137. doi: 10.1007/s11655-019-3204-6
Orendain-Jaime E. N., Ortega-Ibarra J. M., López-Pérez S. J. (2016). Evidence of sexual dimorphism in D1 and D2 dopaminergic receptors expression in frontal cortex and striatum of young rats. Neurochemistry Int. 100, 62–66. doi: 10.1016/j.neuint.2016.09.001
Ossenkopp K.-P., Van Anders S. M., Engeland C. G., Kavaliers M. (2005). Influence of photoperiod and sex on locomotor behavior of meadow voles (Microtus pennsylvanicus) in an automated light–dark ‘anxiety’test. Psychoneuroendocrinology 30, 869–879. doi: 10.1016/j.psyneuen.2005.05.001
Paull G. C., Filby A. L., Giddins H. G., Coe T. S., Hamilton P. B., Tyler C. R. (2010). Dominance hierarchies in zebrafish (Danio rerio) and their relationship with reproductive success. Zebrafish 7, 109–117. doi: 10.1089/zeb.2009.0618
Pellegrini E., Menuet A., Lethimonier C., Adrio F., Gueguen M.-M., Tascon C., et al. (2005). Relationships between aromatase and estrogen receptors in the brain of teleost fish. Gen. Comp. Endocrinol. 142, 60–66. doi: 10.1016/j.ygcen.2004.12.003
Perrot-Minnot M.-J., Banchetry L., Cézilly F. (2017). Anxiety-like behavior increases safety from fish predation in an amphipod crustacea. R. Soc. Open Sci. 4, 171558. doi: 10.1098/rsos.171558
Petersen N., Rapkin A. J., Okita K., Kinney K. R., Mizuno T., Mandelkern M. A., et al. (2021). Striatal dopamine D2-type receptor availability and peripheral 17 β-estradiol. Mol. Psychiatry 26, 2038–2047. doi: 10.1038/s41380-020-01000-1
Porter R. J., Gallagher P., Watson S., Young A. H. (2004). Corticosteroid-serotonin interactions in depression: a review of the human evidence. Psychopharmacology 173, 1–17. doi: 10.1007/s00213-004-1774-1
Russart K. L., Nelson R. J. (2018). Artificial light at night alters behavior in laboratory and wild animals. J. Exp. Zoology Part A: Ecol. Integr. Physiol. 329, 401–408. doi: 10.1002/jez.2173
Saito K., Cui H. (2018). Emerging roles of estrogen-related receptors in the brain: potential interactions with estrogen signaling. Int. J. Mol. Sci. 19, 1091. doi: 10.3390/ijms19041091
Santos E. M., Kille P., Workman V. L., Paull G. C., Tyler C. R. (2008). Sexually dimorphic gene expression in the brains of mature zebrafish. Comp. Biochem. Physiol. Part A: Mol. Integr. Physiol. 149, 314–324. doi: 10.1016/j.cbpa.2008.01.010
Sharma R., Cameron A., Fang Z., Ismail N., Smith A. (2021). The regulatory roles of progesterone and estradiol on emotion processing in women. Cognitive Affective Behav. Neurosci. 21, 1026–1038. doi: 10.3758/s13415-021-00908-7
Sherwin B. B. (2003). Estrogen and cognitive functioning in women. Endocrine Rev. 24, 133–151. doi: 10.1210/er.2001-0016
Shi H., Kumar S. P. D. S., Liu X. (2013). G protein-coupled estrogen receptor in energy homeostasis and obesity pathogenesis. Prog. Mol. Biol. Trans. Sci. 114, 193–250. doi: 10.1016/B978-0-12-386933-3.00006-6
Shontz E., Souders C., Schmidt J., Martyniuk C. (2018). Domperidone upregulates dopamine receptor expression and stimulates locomotor activity in larval zebrafish (Danio rerio). Genes Brain Behav. 17, e12460. doi: 10.1111/gbb.2018.17.issue-4
Songtachalert T., Roomruangwong C., Carvalho A. F., Bourin M and Maes M. (2018). Anxiety disorders: sex differences in serotonin and tryptophan metabolism. Curr. Topics Medicinal Chem. 18, 1704–1715. doi: 10.2174/1568026618666181115093136
Suzuki S., Lund T. D., Price R., Handa R. J. (2001). Sex differences in the hypothalamo-pituitary-adrenal axis: novel roles for androgen and estrogen receptors. In: Pandalai S. G. editor. Recent Research Developments in Endocrinology Vol. 2 Part I. United Kingdom: CABI Publishing. p. 69–86.
Tan M. L., Shen Y. J., Chen Q. L., Wu F. R., Liu Z. H. (2024). Environmentally relevant estrogens impaired spermatogenesis and sexual behaviors in male and female zebrafish. Aquatic Toxicology 275, 107008. doi: 10.1016/j.aquatox.2024.107008
Tang Y.-Q., Li Z.-R., Zhang S.-Z., Mi P., Chen D.-Y., Feng X.-Z. (2019). Venlafaxine plus melatonin ameliorate reserpine-induced depression-like behavior in zebrafish. Neurotoxicology Teratology 76, 106835. doi: 10.1016/j.ntt.2019.106835
Ter Horst J. P., de Kloet E. R., Schächinger H., Oitzl M. (2012). Relevance of stress and female sex hormones for emotion and cognition. Cell. Mol. Neurobiol. 32, 725–735. doi: 10.1007/s10571-011-9774-2
Ter Horst G. J., Wichmann R., Gerrits M., Westenbroek C., Lin Y. (2009). Sex differences in stress responses: focus on ovarian hormones. Physiol. Behav. 97, 239–249. doi: 10.1016/j.physbeh.2009.02.036
Tsai C. L., Wang L. H., Chang C. F., Kao C. C. (2000). Effects of gonadal steroids on brain serotonergic and aromatase activity during the critical period of sexual differentiation in tilapia, Oreochromis mossambicus. J. Neuroendocrinol. 12, 894–898. doi: 10.1046/j.1365-2826.2000.00536.x
Tu X., Li Y.-W., Chen Q.-L., Shen Y.-J., Liu Z.-H. (2020). Tributyltin enhanced anxiety of adult male zebrafish through elevating cortisol level and disruption in serotonin, dopamine and gamma-aminobutyric acid neurotransmitter pathways. Ecotoxicology Environ. Saf. 203, 111014. doi: 10.1016/j.ecoenv.2020.111014
Ulhaq Z. S., Kishida M. (2018). Brain aromatase modulates serotonergic neuron by regulating serotonin levels in zebrafish embryos and larvae. Front. Endocrinol. 9, 230. doi: 10.3389/fendo.2018.00230
Villanueva R. A. M., Chen Z. J. (2019). ggplot2: elegant graphics for data analysis (2nd ed.). MEAS-INTERDISCIP RES. 17, 160–167. doi: 10.1080/15366367.2019.1565254
Wallace K. J., Rausch R. T., Ramsey M. E., Cummings M. E. (2020). Sex differences in cognitive performance and style across domains in mosquitofish (Gambusia affinis). Anim. Cogn. 23, 655–669. doi: 10.1007/s10071-020-01367-2
Wang R., Kogler L., Derntl B. (2024). Sex differences in cortisol levels in depression: A systematic review and meta-analysis. Front. Neuroendocrinol. 72, 101118. doi: 10.1016/j.yfrne.2023.101118
Wang Y. Q., Li Y. W., Chen Q. L., Liu Z. H. (2019). Long-term exposure of xenoestrogens with environmental relevant concentrations disrupted spermatogenesis of zebrafish through altering sex hormone balance, stimulating germ cell proliferation, meiosis and enhancing apoptosis. Environ. pollut. 244, 486–494. doi: 10.1016/j.envpol.2018.10.079
Weil Z. M., Bowers S. L., Nelson R. J. (2007). Photoperiod alters affective responses in collared lemmings. Behav. Brain Res. 179, 305–309. doi: 10.1016/j.bbr.2007.02.003
Weiser M. J., Foradori C. D., Handa R. J. (2008). Estrogen receptor beta in the brain: from form to function. Brain Res. Rev. 57, 309–320. doi: 10.1016/j.brainresrev.2007.05.013
Weiser M., Handa R. J. (2009). Estrogen impairs glucocorticoid dependent negative feedback on the hypothalamic–pituitary–adrenal axis via estrogen receptor alpha within the hypothalamus. Neuroscience 159, 883–895. doi: 10.1016/j.neuroscience.2008.12.058
Wommack J. C., Delville Y. (2007). Stress, aggression, and puberty: neuroendocrine correlates of the development of agonistic behavior in golden hamsters. Brain Behav. Evol. 70, 267–273. doi: 10.1159/000105490
Woodward E., Rangel-Barajas C., Ringland A., Logrip M. L., Coutellier L. (2023). Sex-specific timelines for adaptations of prefrontal parvalbumin neurons in response to stress and changes in anxiety-and depressive-like behaviors. Eneuro 10, 0300–0322. doi: 10.1523/ENEURO.0300-22.2023
Xiang J., Guo R.-y., Wang T., Zhang N., Chen X.-r., Li E.-c., et al. (2023). Brain metabolite profiles provide insight into mechanisms for behavior sexual dimorphisms in zebrafish (Danio rerio). Physiol. Behav. 263, 114132. doi: 10.1016/j.physbeh.2023.114132
Yang L., Zhang Z., He S. (2016). Both male-biased and female-biased genes evolve faster in fish genomes. Genome Biol. Evol. 8, 3433–3445. doi: 10.1093/gbe/evw239
Yin W., Swanson S. P., Biltz R. G., Goodman E. J., Gallagher N. R., Sheridan J. F., et al. (2022). Unique brain endothelial profiles activated by social stress promote cell adhesion, prostaglandin E2 signaling, hypothalamic–pituitary–adrenal axis modulation, and anxiety. Neuropsychopharmacology 47, 2271–2282. doi: 10.1038/s41386-022-01434-x
Zachry J. E., Nolan S. O., Brady L. J., Kelly S. J., Siciliano C. A., Calipari E. S. (2021). Sex differences in dopamine release regulation in the striatum. Neuropsychopharmacology 46, 491–499. doi: 10.1038/s41386-020-00915-1
Zarrindast M.-R., Khakpai F. (2015). The modulatory role of dopamine in anxiety-like behavior. Arch. Iranian Med. 18, 0–0.
Zhang Y., Leung D. Y., Nordeen S. K., Goleva E. (2009). Estrogen inhibits glucocorticoid action via protein phosphatase 5 (PP5)-mediated glucocorticoid receptor dephosphorylation. J. Biol. Chem. 284, 24542–24552. doi: 10.1074/jbc.M109.021469
Zhang F., Tang C., Wang J., Lin T., Ge W., He C., et al. (2024). Letrozole induced a polycystic ovary syndrome model in zebrafish by interfering with the hypothalamic-pituitary-gonadal axis. Environ. pollut. 347, 123723. doi: 10.1016/j.envpol.2024.123723
Zhang M., Yang Q., Shi R., Wang J., Zhang Z., Yang Y., et al. (2022). Effects of long-term sex steroid hormones (estradiol and testosterone)–supplemented feeds on the growth performance of Chinese tongue sole (Cynoglossus semilaevis). Fish Physiol. And Biochem. 48, 1365–1375. doi: 10.1007/s10695-022-01125-w
Keywords: sexually dimorphic anxiety, zebrafish, 17β-estradiol, cortisol, serotonin, dopamine
Citation: Tao H, Zhang Y–Y, Shen Y–J, Chen Q–L and Liu Z–H (2025) Endogenous 17β-estradiol regulates sexually dimorphic anxiety responses in zebrafish via the HPI axis and 5-HT/DA pathways. Front. Mar. Sci. 12:1541351. doi: 10.3389/fmars.2025.1541351
Received: 07 December 2024; Accepted: 22 January 2025;
Published: 07 February 2025.
Edited by:
Melissa Diogo Faria, Leitat Technological Center, SpainReviewed by:
Victoria P. Connaughton, American University, United StatesSofia Priyadarsani Das, National Taiwan Ocean University, Taiwan
Copyright © 2025 Tao, Zhang, Shen, Chen and Liu. This is an open-access article distributed under the terms of the Creative Commons Attribution License (CC BY). The use, distribution or reproduction in other forums is permitted, provided the original author(s) and the copyright owner(s) are credited and that the original publication in this journal is cited, in accordance with accepted academic practice. No use, distribution or reproduction is permitted which does not comply with these terms.
*Correspondence: Zhi–Hao Liu, bWluZW51dEAxNjMuY29t
†These authors have contributed equally to this work