- 1Physical Oceanography Division, National Oceanic and Atmospheric Administration (NOAA) Atlantic Oceanographic and Meteorological Laboratory, Miami, FL, United States
- 2Climate, Atmospheric Science, and Physical Oceanography Division, Scripps Institution of Oceanography, University of California, San Diego, La Jolla, CA, United States
- 3Atmosphere-Ocean Processes and Predictability Division, NOAA Physical Sciences Laboratory, Boulder, CO, United States
- 4Cooperative Institute for Research in Environmental Sciences, Boulder, CO, United States
- 5Université de Toulouse, LEGOS (Institut de Recherche pour le Developpement (IRD), Centre National D'Etudes Spatiales (CNES), Centre National de la Recherche Scientifique (CNRS), Universite Toulouse-III (UT3)), Toulouse, France
- 6IRD Center, Noumea, New Caledonia
- 7Department of Physical Oceanography, GEOMAR Helmholtz Centre for Ocean Research Kiel, Kiel, Germany
- 8Faculty of Mathematics and Natural Sciences, Kiel University, Kiel, Germany
- 9National Oceanic and Atmospheric Administration (NOAA) Pacific Marine Environmental Laboratory, Seattle, WA, United States
- 10Egagasini Node, South African Environmental Observation Network (SAEON), Cape Town, South Africa
- 11Department of Oceanography, University of Cape Town, Cape Town, South Africa
- 12Integrated Marine Observing System (IMOS) Animal Tagging, Sydney Institute of Marine Science, Sydney, NSW, Australia
- 13Cooperative Institute for Marine and Atmospheric Studies, University of Miami, Miami, FL, United States
- 14Rosenstiel School of Marine, Atmospheric, and Earth Science, University of Miami, Miami, FL, United States
- 15Centre for Climate Change Research, Indian Institute of Tropical Meteorology, Ministry of Earth Sciences, Pune, India
- 16Key Laboratory of Ocean Observation and Forecasting, Institute of Oceanology, Chinese Academy of Sciences, Qingdao, China
- 17College of Ocean and Earth Science, State Key Laboratory of Marine Environmental Science, Xiamen University, Fujian, China
- 18Department of Physical Oceanography, Federal University of Santa Catarina, Florianopolis, Brazil
- 19Department of Physics of the Earth and Astrophysics, Complutense University of Madrid, Madrid, Spain
- 20Geosciences Institute Complutense University - The Geosciences Institute (UCM-IGEO), Madrid, Spain
- 21Department of Atmospheric and Oceanic Sciences, University of Colorado Boulder, Boulder, CO, United States
- 22NOAA Global Ocean Monitoring and Observing, Silver Spring, MD, United States
- 23U.S. Integrated Ocean Observing System, NOAA, Silver Spring, MD, United States
- 24School of Atmospheric Sciences, Sun Yat-sen University, Guangzhou, Guangdong, China
Global climate is regulated by the ocean, which stores, releases, and transports large amounts of mass, heat, carbon, and oxygen. Understanding, monitoring, and predicting the exchanges of these quantities across the ocean’s surface, their interactions with the atmosphere, and their horizontal and vertical pathways through the global oceans, are key for advancing fundamental knowledge and improving forecasts and longer-term projections of climate, weather, and ocean ecosystems. The existing global observing system provides immense value for science and society in this regard by supplying the data essential for these advancements. The tropical ocean observing system in particular has been developed over decades, motivated in large part by the far-reaching and complex global impacts of tropical climate variability and change. However, changes in observing needs and priorities, new challenges associated with climate change, and advances in observing technologies demand periodic evaluations to ensure that stakeholders’ needs are met. Previous reviews and assessments of the tropical observing system have focused separately on individual basins and their associated observing needs. Here we provide a broader perspective covering the tropical observing system as a whole. Common gaps, needs, and recommendations are identified, and interbasin differences driven by socioeconomic disparities are discussed, building on the concept of an integrated pantropical observing system. Finally, recommendations for improved observations of tropical basin interactions, through oceanic and atmospheric pathways, are presented, emphasizing the benefits that can be achieved through closer interbasin coordination and international partnerships.
1 Introduction
The tropical oceans strongly influence weather and climate regionally through air-sea interactions and globally through remote impacts on atmospheric and oceanic circulation patterns and heat and carbon storage. There are distinct modes of variability and related ocean-atmosphere phenomena in each basin that have historically motivated separately designed observing systems specifically tailored to the Atlantic, Pacific, and Indian Oceans. The history of the observing systems and networks and the degree of maturity of each system also explain the differences in design. For example, the sustained Tropical Pacific Observing System (TPOS) originated as a grid-like array of equatorial moorings, motivated by the need to understand, monitor, and predict El Niño Southern Oscillation (ENSO) prior to sustained ocean satellite observations and Argo (McPhaden et al., 1998; Davis et al., 2019). The Tropical Atlantic Observing System (TAOS) and Indian Ocean Observing System (IndOOS) were initially motivated by distinct climate modes of variability in those basins as well as other societally important phenomena such as monsoons. As the science and modeling capabilities and demands have evolved, so have the observing systems, which now need to serve multiple purposes: (1) advance our fundamental understanding of the climate system, (2) maintain and extend the climate record to monitor climate variability and change, and (3) improve weather, climate, and ocean forecasting systems, including more accurate predictions of extreme events.
Climatological features unique to the Pacific, Indian, and Atlantic Oceans have in the past provided a rationale for observing system development in each separate tropical ocean basin (Figure 1). Along the equator, the Pacific is more than twice as wide as the Atlantic and Indian Oceans, supporting a large deep pool of warm water in the west collocated with strong atmospheric convection, which drives the pronounced zonal-vertical Walker circulation that links all tropical basins (Figure 1A). The Indian Ocean is closed off to the north by land, resulting in dramatic seasonal reversals of winds and currents associated with monsoons. The Indian and Pacific Oceans are uniquely connected at low latitudes by the Indonesian Throughflow (ITF), while the tropical Atlantic experiences net northward flow from the Atlantic Meridional Overturning Circulation (AMOC) and is connected to the Indian Ocean via the Agulhas Current system. On interannual timescales, the tropical Pacific is dominated by ENSO (McPhaden et al., 2006), with smaller contributions from the Pacific Meridional Mode (Chiang and Vimont, 2004), but multiple modes of variability are important in the tropical Atlantic and Indian Oceans, including meridional and zonal modes in the Atlantic (Nobre and Shukla, 1996; Lübbecke et al., 2018) and the Indian Ocean dipole and basin modes in the Indian Ocean (Figure 2; Saji et al., 1999; Webster et al., 1999; Klein et al., 1999). There is distinct Pacific decadal variability (Power et al., 2021; Capotondi et al., 2023b) and Atlantic multidecadal variability (Knight et al., 2005), but decadal-multidecadal variations are less robust in the Indian Ocean (Dong and McPhaden, 2017). These climate scale variations interact across time scales and across the three tropical oceans (Cai et al., 2019).
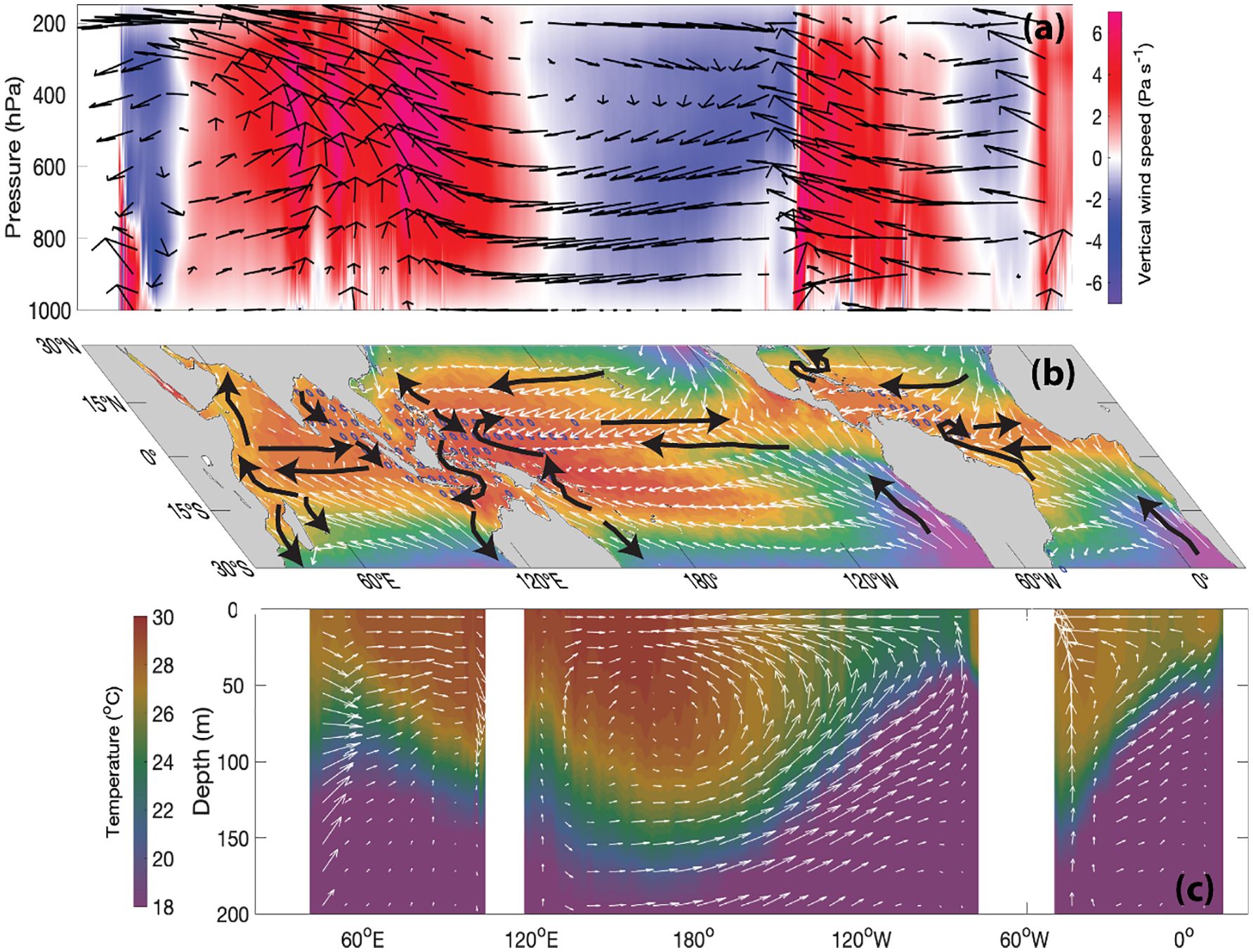
Figure 1. Annual mean (A) ECMWF Reanalysis version 5 (ERA5; Hersbach et al., 2020) zonal and vertical winds (black arrows) and vertical wind speed (shading) averaged between 5°S and 5°N, (B) World Ocean Atlas 2018 (WOA18; Boyer et al., 2018; Locarnini et al., 2019; Zweng et al., 2019) ocean surface temperature [shaded, colorbar shown in (C)], ERA5 near-surface winds (white arrows), regions with low surface salinity in the Atlantic (<36 psu), Indian (<34 psu), and Pacific (<34 psu) based on WOA18 (blue circles), and major near-surface currents (black arrows), (C) WOA18 ocean temperature (shaded) and Estimating the Circulation and Climate of the Ocean (ECCO; Forget et al., 2015) version 4 zonal-vertical currents (white arrows) averaged between 5°S and 5°N.
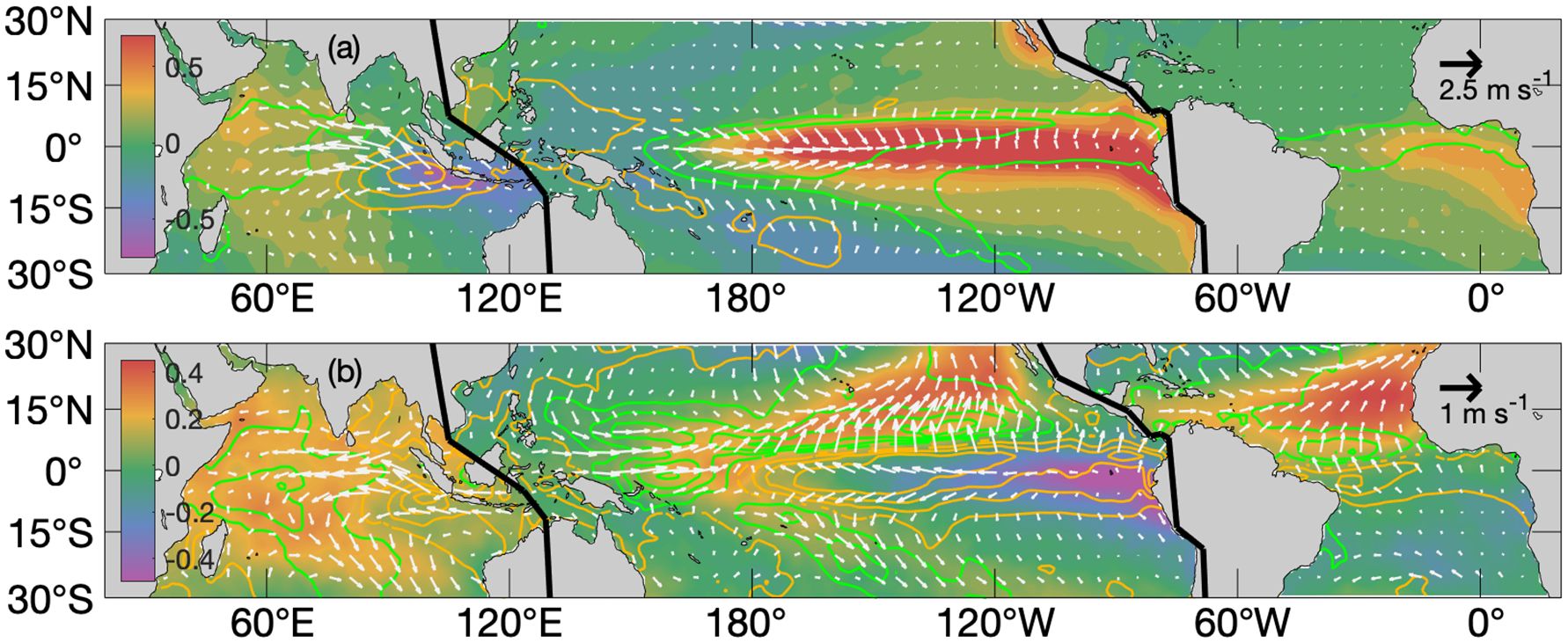
Figure 2. (A) Anomalies of SST from Hadley Center Sea Ice and SST Dataset (HadISST, Rayner et al., 2003; shaded, °C), near-surface winds from ERA5 (white arrows, scale in upper-right), and rainfall from Global Precipitation Climatology Project (GPCP, Adler et al., 2016; green >0 and brown <0, contours shown every ±0.75 mm day-1) regressed onto the index of the dominant near-equatorial mode in each basin during 1980-2023: Indian Ocean Dipole, ENSO (Nino3 index), and Atlantic Nino (ATL3 index), each normalized by its standard deviation. (B) Same as in (A) except for the Indian Ocean Basin mode, Pacific Meridional Mode, and Atlantic Meridional Mode. Solid black lines in (A, B) separate regions in which regressions are calculated.
The tropical oceans are a large heat sink for the increased surface radiative forcing from anthropogenic greenhouse gas emissions, though the ocean heat absorption and resultant SST change vary greatly between and within basins (Cheng et al., 2022). Global climate and weather interactions, and extreme events such as marine heatwaves and tropical cyclones, often have severe and distinct impacts, especially in near-coastal regions. More fundamentally, the tropical oceans are a source of atmospheric deep convection that forces tropical and extratropical teleconnections. The tropical oceans also play a key role in the global carbon cycle, accounting for the largest source of natural carbon outgassing from the ocean to the atmosphere (Takahashi et al., 1997), and are home to biologically productive regions and diverse ecosystems of economic importance, especially in near-coastal regions. Their weak ventilation at depth sustains oxygen deficient zones that play crucial roles in shaping marine ecosystem habitats and the cycling of nutrients and powerful greenhouse gasses such as nitrous oxide. These biogeochemical cycles are tightly coupled to ocean and atmospheric physical processes and modes of variability (Chavez et al., 1999; Feely et al., 2002), and may also influence climate via biological and biogeochemical feedbacks (Park et al., 2011). As a result, it has long been recognized that the observing system must extend beyond physical parameters to include more biogeochemical and biological observations, which have been increasingly included in recent observing system designs.
Complicating these efforts to design and maintain a comprehensive observing system are sharp intra- and inter-basin contrasts in the societal capacity to support basin-wide observing systems. In many cases, the regions most adversely affected by climate variability and change and extreme weather events (e.g., Indian Ocean rim countries, Pacific and Caribbean islands, West Africa) have fewer resources and limited capacity for building and sustaining robust observing systems. Historically, highly industrialized nations have contributed more to building up the Pacific and Atlantic Ocean observing systems compared to the Indian Ocean, though there are areas in all tropical basins, such as coastal upwelling zones in the eastern tropical Atlantic and Pacific Oceans, that are not well observed (Figure 3). These regions are critically important because they experience strong seasonal to interannual variability that impacts regional climate, ecosystems, and fisheries.
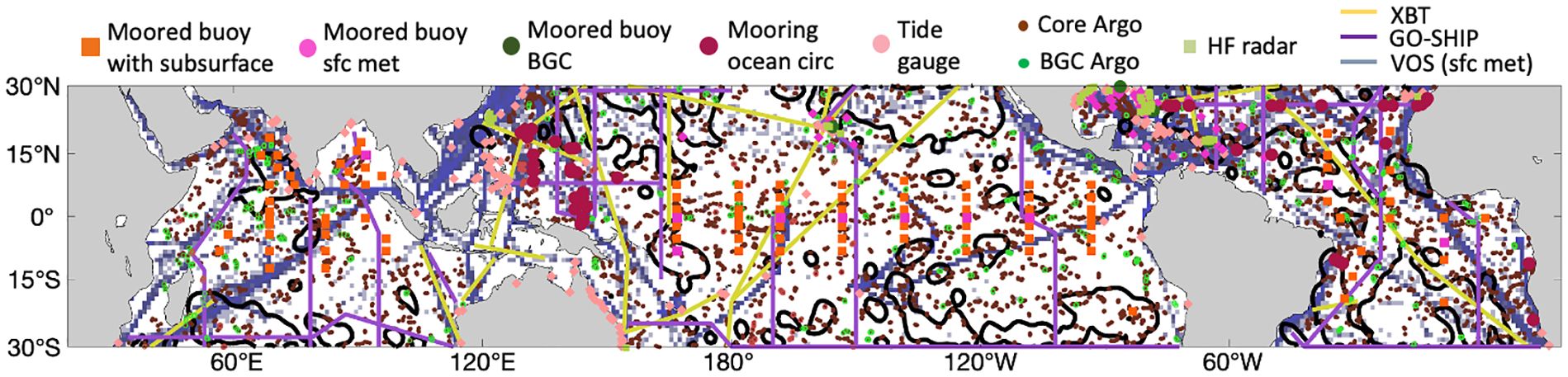
Figure 3. Global tropical in-situ observing system as of January 2024. Black contours enclose 1°x1° bins with at least five 6-hourly surface drifter observations during January 2024. Blue lines/shading indicate the number of volunteer observing ship reports during January 2024, in each 1°x1° bin, that contain at least wind speed and SST, from a minimum of zero (no shading) to 544 (darkest blue shading). XBT and GO-SHIP (Global Ocean Ship-based Hydrographic Investigations Program) lines shown (yellow and purple, respectively) are those that were active as of January 2024, even if the individual lines were not being conducted during that time period. Brown and green dots show locations of Core and BGC Argo profiles, respectively, made during January 2024. Surface and subsurface moored buoys are shown as if they were being actively maintained as of January 2024, even if there are recent data gaps. Pink squares indicate global tropical moored buoys with CO2 sensors. Light green squares are high-frequency (HF) radar stations that were active during 2023-2024 and made their data publicly available.
The aforementioned phenomena and challenges point to a growing need to treat the tropical basins as parts of one interconnected global system that encompasses both the near-coastal and open ocean seamlessly. Ultimately, the entire global ocean observing system must be considered as a whole because of close links between all components. However, here we focus on the tropical observing system, in part because it is in many respects the most mature of the global observing systems (Figure 3). Commonalities across all tropical basins include the tightly coupled ocean-atmosphere interactions that govern their mean states and variability, and their increasingly pronounced responses to climate change. In the 60 years since the discovery of the remote influence of the tropical Pacific Ocean on the global atmospheric circulation (Bjerknes, 1969), it has also become clear that there are interactions between all tropical ocean basins (Cai et al., 2019). These discoveries translate to a greater need for a coordinated network of global tropical ocean observations for research and for initializing and validating coupled weather and climate forecast models. An integrated pantropical observing system has great potential to advance research and maximize forecast skill through accurate representation of tropical basin interactions, filling gaps left by focusing on separate observing systems for individual basins. Furthermore, fundamental ocean and coupled processes, such as air-sea heat, momentum, and greenhouse gas fluxes, and ocean heat and carbon storage, occur globally and require global observations for monitoring and research.
In the past five years, major observing system reviews and reports have been completed separately and rather independently for each tropical basin with the goal of assessing the current state of the observing system and its future needs while taking into account feedback from the research, monitoring, modeling, and forecast communities and their stakeholders (Hermes et al., 2019; Foltz et al., 2019; Smith et al., 2019; Beal et al., 2020; Hu et al., 2020; Johns et al., 2021; Kessler et al., 2021). The reviews grew out of different circumstances. The TPOS review was motivated by a sudden and drastic decrease in TAO/TRITON (Tropical Atmosphere-Ocean/Triangle Trans-Ocean Buoy Network) data return during 2012-2013 due to a sharp reduction in NOAA ship time for servicing the moorings followed by Japan’s phased withdrawal of TRITON moorings. This threat to the observing system generated strong interest in making TPOS more robust, sustainable, and integrated. It also created an opportunity to expand biogeochemical observations (Cravatte et al., 2016). The TAOS and IndOOS reviews were motivated by changing observing and societal needs following the inception of those observing systems in the late 1990s and early 2000s (McPhaden et al., 2009; Bourlès et al., 2019). The Northwestern Pacific Ocean Circulation and Climate Experiment (NPOCE) review (Wang et al., 2018) was motivated by the widely accepted but poorly quantified importance of Pacific western boundary currents for equatorial Pacific circulation and variations of climate modes such as ENSO and the Pacific Decadal Oscillation. All reviews resulted in the identification of important gaps in the observing systems, including biogeochemical and ecosystem measurements.
The individual basin reviews, combined with the growing awareness of the importance of tropical basin and tropical-extratropical interactions, motivate syntheses of the state of global tropical observations, recommendations for the future, and a path toward an integrated pantropical ocean observing system. Therefore, the goal of this review is to emphasize common gaps, needs, and recommendations for the current tropical observing systems, including those required to better understand and predict tropical basin interactions. This includes assessing how observations and lessons learned in one basin can aid development in other basins. This review focuses primarily on the upper ocean, which has the densest observational coverage and is home to energetic signals on a wide range of temporal and spatial scales, including vigorous air-sea interactions. The upper ocean was also the focus of the three tropical basin reviews that motivate this review. Deep ocean observing needs are reviewed in Levin et al. (2019). In Section 2 we summarize the similarities across the recent individual basin reviews in the context of the tropical observing system, highlighting common observing needs and proposed recommendations. After discussing each specific scientific issue, relevant common recommendations will immediately follow. Section 3 discusses the observing system in the context of tropical basin interactions, and Section 4 concludes with a discussion of current issues and perspectives on the future pantropical observing system.
2 Observing system commonalities
One of the purposes of the recent individual tropical basin reviews was to develop a path toward a more integrated observing system for each tropical ocean, combining multiple satellite and in-situ platforms while taking into account societal needs, research priorities, operational needs, and resource constraints. Here we discuss commonalities between the individual observing systems and their motivations as well as the common observing needs, gaps, and recommendations, with the goal of emphasizing priorities for growing and evolving the pantropical observing system (Figure 3). While our focus is on the ocean and air-sea interface, there are also pressing needs for observations of the atmospheric boundary layer (NASEM, 2018; Teixeira et al., 2021) and for tropical-extratropical interactions, as part of a comprehensive global observing system for weather and climate.
2.1 Climate monitoring and maintenance of the observing backbone
All tropical oceans exhibit variations on timescales longer than interannual, which may occur naturally or due to climate change (Figure 4). These changes, together with a diversity of individual seasonal-interannual climate mode events, motivate the need for robust climate monitoring and maintenance of a dedicated observing “backbone” on which additional observations can be built. In the tropical Pacific, decadal variability modulates ENSO characteristics and its remote impacts (Power et al., 2021; Capotondi and Qiu, 2023a), but the processes are not well understood (Stuecker, 2018; Capotondi and Qiu, 2023a). The eastern equatorial Pacific is undergoing a cooling trend (Li et al., 2023) that is in disagreement with the warming trend simulated by most climate models over the historical period (e.g., Cai et al., 2021), calling for a clearer separation of internal and anthropogenically-forced variability (Jiang et al., 2024). Variations in these physical processes also drive changes in upper-ocean biogeochemistry (Feely et al., 2006; Poupon et al., 2023).
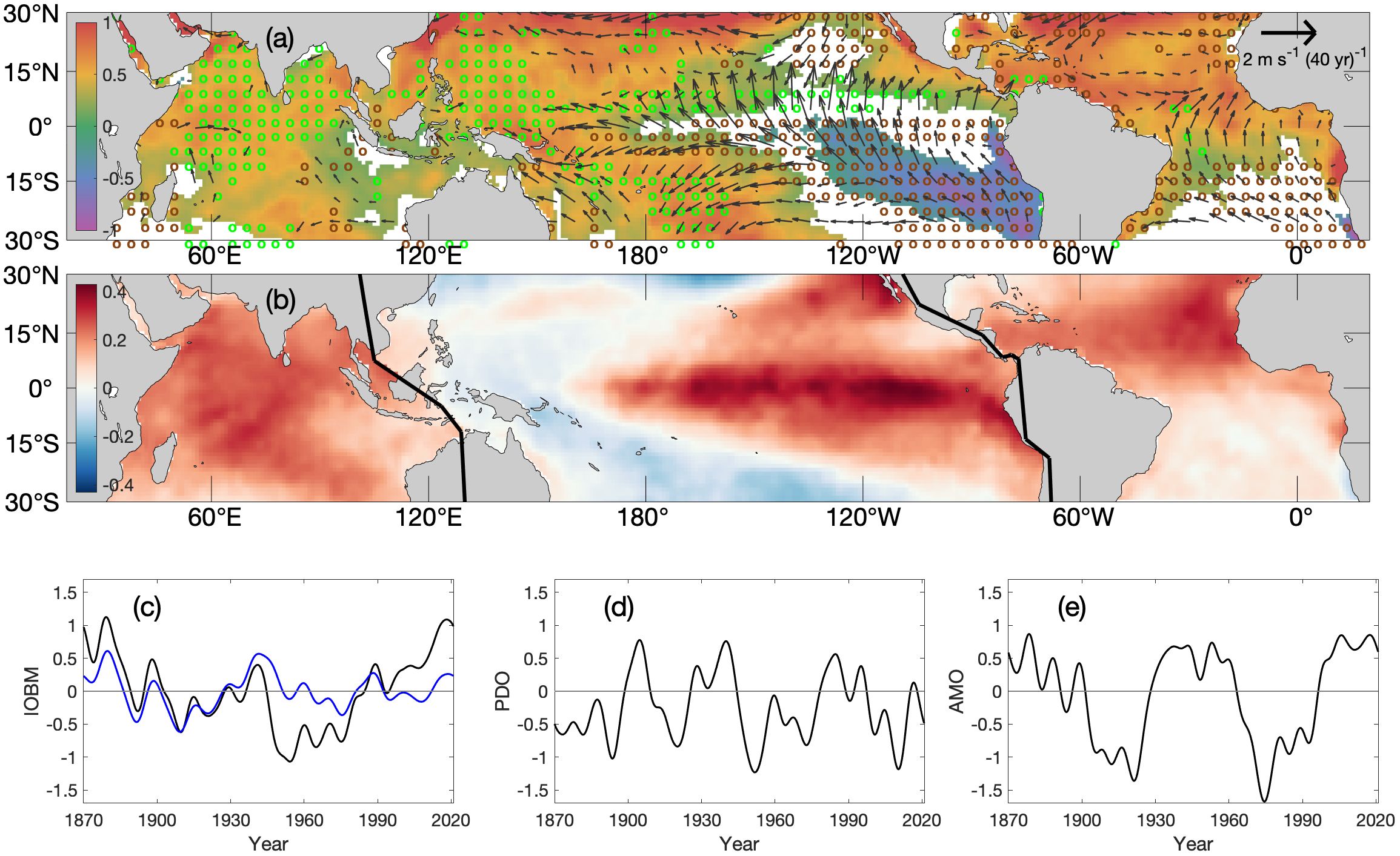
Figure 4. (A) 1980-2020 trends in SST from HadISST (shaded, °C per 40 yr), near-surface winds from ERA5 (arrows), and rainfall from GPCP (green circles for trends >0, brown circles for trends <0). Values are only plotted when significant at the 5% level. (B) SST regressed onto the Indian Ocean Basin Mode (IOBM), Pacific Decadal Oscillation (PDO), and Atlantic Multidecadal Oscillation (AMO) indices separately in each corresponding basin and then combined into a global map. Indices were obtained from https://psl.noaa.gov/data/climateindices/list/. Solid black lines separate regions in which regressions are calculated. The indices were normalized by their standard deviations before computing regression coefficients. (C) Black: 10-year low-pass filtered IOBM index. Blue: 10-year low-pass filtered IOBM index after removing the 1870-1945 trend and the 1945-2020 trend from each corresponding time period to reduce centennial variability and emphasize decadal-multidecadal variability. (D, E): Same as (C) except for PDO and AMO indices, respectively.
In the same way that Pacific decadal variability affects ENSO and its teleconnections, Atlantic multidecadal variability modulates equatorial Atlantic interannual variability (Martín-Rey et al., 2014) and its remote forcing of the tropical Pacific (Losada and Rodríguez-Fonseca, 2016, Wang et al., 2024). There is debate regarding the drivers of Atlantic multidecadal variability, with some studies showing forcing from the AMOC (Knight et al., 2005) and others arguing for the important role of surface heat fluxes driven by internal atmospheric variability such as the North Atlantic Oscillation (Clement et al., 2015) and volcanic eruptions (Mann et al., 2021). After removing strong centennial variability in the Indian Ocean, including a strong warming trend since the 1940s, decadal-multidecadal variability in the Indian Ocean is less energetic compared to the Pacific and Atlantic (blue curve in Figure 4C; Zhang et al., 2018), though there are more pronounced multidecadal variations in the southern Indian Ocean (Shi et al., 2007; Trenary and Han, 2013). Understanding of these low-frequency tropical variations has received renewed attention in the context of multiyear to decadal prediction efforts (Collins et al., 2006; Meehl et al., 2014; Liu et al., 2019). In addition, enhanced understanding of the oceanic and atmospheric processes responsible for internal decadal variations can aid identification of the climate change signal, the pattern and time evolution of which are the subjects of intense debate, especially in the tropical Pacific (Meehl et al., 2013; Dai et al., 2015; Seager et al., 2022). It is also clear that the deep ocean must be observed in a sustained manner in order to monitor climate change and especially the role of the ocean in the global heat and carbon budgets (Heuzé et al., 2022; Müller et al., 2023).
Decadal variations in each basin may not simply arise from processes internal to that basin, but can be influenced by processes occurring in other basins, motivating the need for an integrated pantropical observing system. There is debate about the influence of tropical Atlantic warming trends on the tropical Pacific (McGregor et al., 2014; Zhao and Capotondi, 2024). In the Indian Ocean, the leading mode of decadal variability is associated with a basin-wide warming that is in phase with tropical Pacific decadal variability (Dong et al., 2016; Ummenhofer et al., 2020). The Indian Ocean also feeds back to the Pacific on decadal timescales, potentially enhancing Pacific trade winds (Dong and McPhaden, 2017). Multidecadal variations in the South Indian Ocean have been linked to changes in the Pacific Decadal Oscillation and the Pacific trade winds, which impact the ITF (Ummenhofer et al., 2017; Trenary and Han, 2008; Schwarzkopf and Böning, 2011), while the global warming trend in the Indian Ocean modulates global atmospheric circulation (Dhame et al., 2020). The uncertain nature of these inter-basin feedbacks, the relative roles of natural variability and global warming in observed and projected trends, and models’ difficulties in simulating decadal-multidecadal variability (Ba et al., 2014; Zhao et al., 2023a), call for sustained observations that can constrain climate models and allow for assessments of models’ performance.
2.1.1 Common recommendations
Previous individual tropical basin observing system reviews all put a high priority on maintaining observations that have proven value for ocean and climate monitoring and for aiding forecasts, assessments, and longer-term projections. These include elements of the present in-situ sustained observing system (Figure 3) plus satellite observations, with some records going back more than 40 years in the Pacific, nearly 30 years in the Atlantic, and 20 years in the Indian Ocean. The in-situ records are long enough that decadal variations and trends related to climate change can be detected. These records become more valuable as they grow in length, increasing the range of timescales that can be examined, sampling a greater range of natural variations, and providing a baseline for assessing the impacts of future climate change on the tropical ocean and overlying atmosphere. In particular, measurements of wind, heat, momentum, and greenhouse gas fluxes, as well as full-depth ocean heat content and sea level, are key for the understanding of decadal-multidecadal variability and climate change. The continuation of all existing long-term records of the state variables needed to calculate these quantities, including air temperature, humidity, SST, winds, surface ocean partial pressure of CO2 (pCO2), surface currents, and subsurface ocean temperature and salinity, is recommended across all observing system reviews. The reviews consistently call for an increase in Argo float coverage and continued expansion of BGC Argo and Deep Argo, which provides full-depth temperature and salinity profiles (Roemmich et al., 2019). It is important to ensure temporal overlap and some redundancy for these measurements to guard against gaps in individual observing systems and changes in observing system platforms. Redundant measurements are also extremely valuable for satellite calibration and in-situ intercalibration.
There is also a need in all tropical basins to maintain some high-accuracy continuous reference sites that measure the variables needed to calculate all surface heat, freshwater, and momentum fluxes, together with barometric pressure and profiles of ocean temperature, salinity, and currents (McPhaden et al., 2023; see also Section 2.2). These enhanced sites would enable validation of satellite data and models and monitoring of long-term changes. Such changes are challenging to diagnose with the present observing system, in part because they have much smaller amplitudes and require higher-accuracy measurements compared to typical intraseasonal to interannual variability. Complementing the tropical ocean observing system, continued and enhanced measurements of the atmospheric boundary layer are critical for improved understanding and predictions of weather and climate. These include satellite observations and active and passive surface-based profilers, which are extremely sparse over the ocean.
A common need throughout the tropics is to enhance biogeochemical measurements and sustain the limited ongoing decadal observations (see Section 2.6). It is also important to extend the decadal records back in time through continued development of paleo proxies for past ocean, climate, biogeochemistry, and ecosystem variability, for example from large well-established coral reefs and ocean sediment. These proxies are valuable for analyses of decadal to century-scale variability that are not possible with the limited observational record (Dee et al., 2020; Henderiks et al., 2020). Finally, a common need across the tropics and beyond is continued monitoring of atmospheric greenhouse gases and other climate-relevant tracers such as aerosols and oxygen. While not directly within the purview of the tropical ocean observing systems, these measurements are critical for understanding externally forced drivers of climate change and variability, and provide an integrated perspective on changes in ocean carbon sinks and sources in the context of global change.
2.2 Key ocean-atmosphere parameters and processes
2.2.1 Needs for improved understanding of air-sea exchanges
The ocean and atmosphere communicate primarily through air-sea heat and momentum exchanges as well as biogeochemical fluxes (see Section 2.6). These are normally estimated using bulk formulas that require knowledge of near-surface temperature, humidity, wind, surface currents, and gas concentrations. All tropical oceans are severely undersampled in terms of near-surface air temperature and humidity, which cannot currently be measured reliably from satellites. This is mainly because existing satellite instrumentation cannot resolve the atmospheric boundary layer with the required vertical resolution. There are also very limited in-situ observations for calibrating and validating satellite retrievals, and the relationships between satellite and in-situ measurements can be complex and highly regime-dependent (Yu and Jin, 2018). As a result, the net surface turbulent heat flux is poorly constrained in the tropics (Figure 5C, Clayson et al., 2023a; Yu, 2019; Cronin et al., 2019). This puts limitations on weather and climate predictions (Penny et al., 2019). Measurements of surface solar and longwave radiation over the tropical oceans are even sparser, increasing the uncertainty of the net surface heat flux throughout the tropics and limiting advancements in the understanding and modeling of clouds, aerosols, and their radiative forcing (Figures 5B, C, Yu, 2019). Some of the most difficult areas from a climate modeling perspective are the eastern Pacific and eastern Atlantic, especially the areas encompassing the cold tongue, intertropical convergence zone (ITCZ), eastern boundary currents, upwelling, and stratus clouds. These areas have some of the largest and most persistent observational uncertainties and coupled model biases in the tropics (Zhang et al., 2023c). There are also very few long-term measurements of direct covariance turbulent momentum, heat, and CO2 fluxes in the tropics, especially in high-wind conditions such as tropical cyclones. These data, along with concurrent and collocated measurements of ocean surface wave properties and near-surface currents, are needed to improve parameterizations of fluxes in terms of roughness length, drag and enthalpy exchange coefficients, and gas transfer velocity.
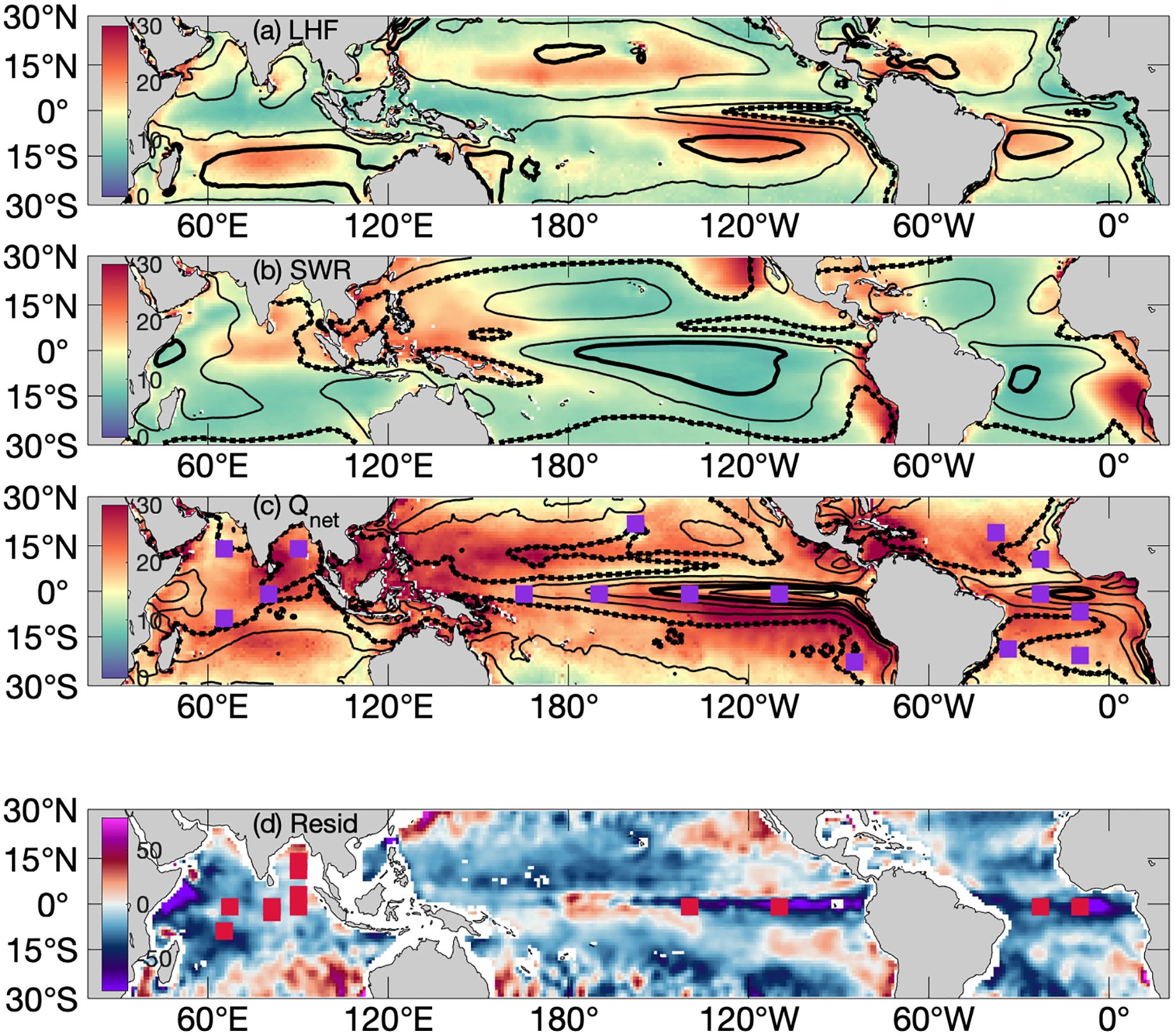
Figure 5. (A) Shading: Standard deviation (W m-2) of monthly mean surface latent heat flux across four products: ERA5, MERRA2 (Gelaro et al., 2017), OAFlux (Yu and Weller, 2007), and TropFlux (Kumar et al., 2012). Values are calculated at each grid point and then averaged over all months during 1980-2018. Contours: Annual mean latent heat flux, contoured every 25 W m-2, with dashed and bold contours for 75 and 150 W m-2, respectively. Positive values indicate cooling of the ocean. (B, C): same as (A) except surface solar radiation and net surface heat flux, respectively during 1983-2009. Dashed and bold contours show annual mean values of 200 and 250 W m-2 for solar, respectively, 25 and 100 W m-2 for Qnet. Positive values indicate warming of the ocean. (D) Annual mean ocean mixed layer heat budget residual (mixed layer heat storage rate minus the sum of Qnet and horizontal heat advection, in W m-2) as an estimate of the contribution from vertical mixing, calculated as in Foltz et al. (2019). Horizontal heat advection was calculated as the product of seawater density, heat capacity, and monthly-varying ORAS5 (Zuo et al., 2019) mixed layer depth (MLD), satellite SST gradients, and drifter-wind-altimetry synthesis horizontal near-surface currents (Lumpkin and Garzoli, 2011), which were scaled to be representative of the local MLD. Purple squares in (C) are locations of full-flux reference moorings. Red squares in (D) are locations of moorings with past or ongoing ocean turbulence sensors. Most record lengths in the Indian Ocean are 1-2 years, whereas those in the Atlantic and Pacific are several years.
2.2.2 Needs for improved understanding of upper ocean physics
Upper-ocean mixing and upwelling help shape the spatial distributions of ocean temperature, density, and horizontal currents in the tropics, which couple with surface heat and momentum fluxes to drive tropical climate variability. There are few sustained direct measurements of turbulent mixing in the tropics (Moum et al., 2022), and upwelling is usually estimated from horizontal surface current divergence and/or changes in isotherm depths. The time and space scales of mixing are poorly known, leading to uncertainties in modeling and parameterizing these processes and their impacts on SST (Zhu and Zhang, 2019; Deppenmeier et al., 2020; Whitt et al., 2022; Mrozowska et al., 2024; Bastin et al., 2024). It is very rare to have collocated measurements of vertical current shear, stratification, and mixing, which is the minimum dataset required to quantify and understand diapycnal turbulent fluxes of heat, salt, and buoyancy. The need for enhanced efforts to measure these variables (Le Boyer et al., 2023) is underlined by the fact that turbulent diapycnal fluxes were recently added as a pilot essential ocean variable (EOV; Lindstrom et al., 2012) by the Global Ocean Observing System (GOOS) expert panels. It is known that the diurnal cycle is an important modulator of equatorial current shear and mixing (Smyth et al., 2013; Moum et al., 2022; Masich et al., 2021; Hans et al., 2024). However, the contributions from the diurnal cycle, wind stress, wind-forced near-inertial waves, and other internal waves generated by wind, tides, and ocean topography, are not well known (Koch-Larrouy et al., 2015; Whalen et al., 2020; Hummels et al., 2020; Moum et al., 2023). Sustained measurements of mixing are also needed to assess interactions of micro-scale mixing with other phenomena such as tropical instability waves, Kelvin waves, the seasonal cycle, and interannual-decadal climate modes (Holmes and Thomas, 2015; Deppenmeier et al., 2022), and their impacts on heat and biogeochemical fluxes and budgets in the tropics (Whitt et al., 2022; Eddebbar et al., 2024; Brandt et al., 2025).
All tropical basins have regions of low surface salinity and barrier layers created by the combination of river outflow and heavy rainfall (blue circles in Figure 1B). The additional density stratification and vertical distance between the pycnocline and thermocline can act to reduce turbulent mixing and cooling of SST (Vialard and Delecluse, 1998; Li et al., 2017; Gevaudan et al., 2021; McPhaden et al., 2024) and influence tropical cyclone intensification (Hlywiak and Nolan, 2019; Balaguru et al., 2020), but quantification is challenging and observations are complicated by poorly known spatial and temporal scales of variability (Liu et al., 2022). Low-salinity, nutrient-rich river plumes also affect ocean biogeochemistry and air-sea CO2 fluxes in ways that are not well understood (Subramaniam et al., 2008; Joshi et al., 2021). In all tropical basins, there are regions of vigorous mesoscale, submesoscale, and frontal variability that contribute to upwelling, mixing, and surface heat, momentum, and gas fluxes, processes that are poorly known because of the small spatial and temporal scales that must be resolved (Skyllingstad et al., 2019; Seo et al., 2023). This small-scale variability affects the atmosphere and influences longer-timescale ocean and climate variability (Chelton et al., 2001; Maillard et al., 2022; Holmes et al., 2024) as well as biogeochemical processes (Feely et al., 1994; Evans et al., 2009), but remains rarely observed across all basins (Kennan and Flament, 2000; Menkes et al., 2002).
2.2.3 Common recommendations
High-priority recommendations across the tropics are to (1) continue development of the capability to measure near-surface air temperature, humidity, wind, and SST from the same satellite (e.g., the proposed Butterfly mission; Clayson et al., 2023b), as well as satellites for simultaneous measurements of surface currents and winds (e.g., ODYSEA: Ocean Dynamics and Surface exchange with the Atmosphere; Torres et al., 2023), (2) increase the number of moored buoys with full surface heat flux measurements (latent, sensible, shortwave, longwave) and upper-ocean currents, and (3) enhance the vertical resolutions of temperature and salinity in the ocean mixed layer. These augmentations will enable better characterization of ocean mixed layer processes, heat budgets, and mixed layer-thermocline interactions, which are often not well simulated in climate models (Zhu and Zhang, 2019; Farneti et al., 2022). To improve understanding and monitoring of surface heat and momentum fluxes, all tropical basin reviews recommend enhancing a subset of moorings with high-frequency sampling (at least 10-20 Hz) of air temperature, humidity, and winds for direct covariance measurements of surface heat and momentum fluxes. These should be targeted for regimes where bulk flux algorithms show the greatest uncertainties, such as low winds and areas of high tropical cyclone track density (periodic high winds and large waves). Collocated surface fluxes and directional surface wave spectra, and enhanced in-situ measurements of waves from moorings and drifting buoys, are recommended for improving surface heat and momentum flux parameterizations. More generally, all tropical basin reviews recommend expanding moored buoy coverage to include other regions that are important scientifically and would aid satellite calibration and validation. For example, TPOS recommended additional near-equatorial moorings and expansion into high-rainfall regions such as the ITCZ and South Pacific convergence zone (SPCZ). It was also recommended to prioritize completion of RAMA in the western Indian Ocean and to expand the number of moored buoys in the northwestern and southeastern tropical Atlantic.
There is a common need for increased coverage of direct measurements of turbulent mixing in key areas of all tropical oceans. High priorities include (1) marginally unstable regions such as the eastern equatorial Atlantic and Pacific cold tongues above the core of the Equatorial Undercurrent, and the Indonesian Seas, where tidal mixing is strong, and (2) off the equator where near-inertial and other internal waves are generated and measurements are sparse (Figure 5D). At a subset of sites, measurements of turbulent mixing should be combined with very high resolution measurements of vertical current shear, temperature stratification, and salinity stratification to diagnose the drivers of mixing on multiple timescales and improve mixing parameterizations. High resolution measurements of key biogeochemical tracers, such as pCO2, chlorophyll, and oxygen, should also be considered for these sites, to assess the impact of mixing on productivity, carbon flux, and oxygen ventilation. It is recommended to augment some of these sites with complete surface flux measurements, waves, profiles of winds and atmospheric thermodynamics, measurements of clouds, and the means to measure spatial gradients, to form “super sites” that will accelerate process understanding across and between a wide range of temporal and spatial scales (including mesoscale and submesoscale/frontal), improve monitoring, and aid satellite retrievals, model initialization and validation (Hagos et al., 2020; Clayson et al., 2023a). Related to super sites but not part of the sustained observing strategy, process studies are needed to improve understanding and parameterizations of mesoscale, submesoscale, and frontal variability. They should include ship-based, satellite, and uncrewed surface and subsurface vehicles, ideally in conjunction with moored observations.
2.3 Boundary currents and the Indonesian Throughflow
Western boundary currents are important components of every tropical ocean basin (Figure 1B). In addition, the ITF in the western Pacific is a unique low-latitude oceanic connector of two tropical basins. Eastern boundary currents include the Leeuwin Current flowing southward west of Australia, and the Benguela and Humboldt Currents flowing northward in the eastern Atlantic and Pacific, respectively (Figure 1B). The upper-ocean boundary currents transport large amounts of heat, freshwater, carbon, oxygen, and nutrients equatorward and poleward and, in the case of the ITF and the Agulhas Current, between basins, and therefore must be observed in order to monitor global ocean circulation and climate (Todd et al., 2019). Low-latitude western boundary currents in the Pacific regulate ENSO upper-ocean heat recharging and discharging and communicate ENSO and decadal variations of western Pacific mass, heat, and freshwater to the Indian Ocean via the ITF (Hu et al., 2015; Dong and McPhaden, 2016). The ITF plays a fundamental role in the coupled climate system (Sprintall et al., 2014), and is expected to significantly modulate the oxygen content of the Indian ocean (Ditkovsky et al., 2023). In the past 15 years, substantial progress has been made in understanding the three-dimensional multi-scale variability and dynamical mechanisms of the subsurface current system in the western tropical Pacific, mainly through direct measurements by subsurface moorings (Hu et al., 2013; Wang et al., 2016; Hu et al., 2020; Wang et al., 2021; Zhang et al., 2023b).
In the Atlantic, the AMOC regulates global climate (Zhang et al., 2019) and sea level (Little et al., 2019), and the Loop Current sheds eddies with anomalously high heat content that make tropical cyclone intensification more likely (Shay et al., 2000). The heat transport by the Leeuwin Current influences seasonal Indian Ocean climate and marine heatwaves (Pearce and Feng, 2013; Feng et al., 2015). The seasonally reversing Somali Current is part of one of the most biologically productive areas of the world ocean. Boundary currents are also an important link between the coastal and open ocean through upwelling from mesoscale and frontal systems and the lateral exchanges of heat, freshwater, carbon, oxygen, and nutrients (Todd et al., 2019). For example, the Greater Agulhas Current system, one of the most energetic current systems in the world, serves as a key conduit for the exchange of heat, nutrients, carbon, and oxygen between ocean basins (Biastoch et al., 2024; Sinyanya et al., 2024), while its strong mesoscale and submesoscale activity drives upwelling and lateral mixing, impacting marine productivity and contributing to the formation of Agulhas Leakage, which affects the AMOC (Jackson et al., 2012; Schubert et al., 2021).
Long-term monitoring of boundary currents is challenging in all tropical basins because they vary on a wide range of spatial and temporal scales. They are often narrow, fast-flowing, and can be constrained by shallow topography with intense eddying recirculations, further complicating observing efforts. Typically, boundary current monitoring throughout the tropics cannot be achieved by a single platform but instead requires a combination of complementary long-term platforms (Morris et al., 2017; Todd et al., 2019; Smith et al., 2019; Sprintall et al., 2019; Ayoub et al., 2024). The most developed western boundary current observing system is in the Atlantic, where there are coordinated efforts to observe the AMOC (Frajka-Williams et al., 2019) through the Florida Current (Meinen et al., 2010), across the Atlantic at 26.5°N (Cunningham et al., 2007), in the deep limb of the AMOC at 16°N (Send et al., 2011), and in the western Atlantic at 11°S (Hummels et al., 2015). In the past ten years, an observing network with more than 20 subsurface moorings has been established to measure full-depth velocity, temperature, and salinity in the western tropical Pacific (Levin et al., 2019; Wang et al., 2020). The long-term data aid understanding of upper-ocean zonal and western boundary currents and the Pacific meridional overturning circulation (Wang et al., 2021; Zhang et al., 2023b).
International and regional cooperation is vital for sustained boundary current observations. Dedicated personnel efforts will be necessary to sustain an observational network, especially in remote areas, and to coordinate data sharing to freely deliver the data for operational use and scientific analysis. There has been a recent concerted community effort to collectively determine appropriate strategies to improve boundary current observing. As part of the U.N. Ocean Decade for Sustainable Development, development of an integrated fit-for-use observing system for boundary currents is one of six user-focused exemplar projects under the GOOS Co-Design Programme, with the goal to provide a sustainably funded infrastructure for ocean observing that will benefit society and is cost-effective for investors (https://goosocean.org/what-we-do/goos-at-the-heart-of-the-ocean-decade/ocean-observing-co-design/). Another effort to design an integrated boundary current observing system is being undertaken by the Ocean Observations Physics and Climate Panel (OOPC) Boundary System Task Team (BSTT). A recent assessment of six well-observed boundary current systems helped identify knowledge gaps and develop a conceptual design for sustained observing applicable to a wide variety of boundary current systems (Ayoub et al., 2024).
2.3.1 Common recommendations
In the Pacific and Indian Oceans, it is likely that pilot studies will continue to be required to determine appropriate measurement strategies in terms of spatial and temporal sampling and prioritizing the variables to measure in boundary currents. Short-term process studies and other efforts have been organized (Alberty et al., 2019; Kessler et al., 2019; Anutaliya et al., 2019), but there is a need to evolve these shorter-term field campaigns into more coordinated pilot studies that will encourage greater international participation, as has been effective for the North and South Atlantic AMOC arrays. Initial analysis suggests the need to monitor the low-latitude western boundary heat transport to at least 500 m depth at monthly and seasonal timescales, including simultaneous heat transport measurements in the northern and southern branches in the Pacific and across the ITF (Cravatte et al., 2016; Todd et al., 2019; Smith et al., 2019; Sprintall et al., 2019). The pilot study proposed by Cravatte et al. (2016) recommended a multi-platform approach consisting of a combination of moorings, repeat line transects from gliders and XBTs, and broad-scale sampling from Argo floats, surface drifting buoys, and satellites. For the ITF, a two-phased multi-platform approach was detailed by Sprintall et al. (2019). Concurrent measurements of key biogeochemical tracers across these platforms should be undertaken, as feasible, to monitor the transports of oxygen, carbon, and nutrients via low latitude western boundary currents such as the ITF and Agulhas Leakage.
Similar general recommendations have been made for the Indian Ocean western and eastern boundary currents (Beal et al., 2020). For example, there is a need to maintain existing XBT lines, expand Argo coverage, and a call for implementing an off-shore deeper end-point mooring array in order to better capture the volume, freshwater, and heat fluxes of the Leeuwin and Agulhas Currents. These similar needs and common difficulties, such as the need for permission to sample in Exclusive Economic Zones (EEZs) from multiple countries and challenges securing resources and funding, emphasize the importance of developing high-level governance and maintaining international working groups that can also facilitate transfer of knowledge and lessons learned among the three tropical basins.
2.4 Coastal regions
The coastal regions of all tropical basins are often undersampled, especially considering their smaller spatial scales and shorter timescales. Sea level rise threatens many coastal communities in the tropics. However, monitoring and understanding of near-coastal sea level variability and change are limited by sparse in-situ observations from tide gauges, especially in the Indian Ocean, Maritime Continent, and eastern Pacific and Atlantic (Figure 6), while high-resolution satellite measurements have become available only recently from the Surface Water and Ocean Topography (SWOT) mission. There are also unique processes in the near-shore regions that require tailored observing systems, such as local wind-driven and remotely-forced coastal upwelling of colder nutrient-rich waters, tidal mixing, interactions with ocean eddies, fronts and filaments, and impacts on primary productivity and ecosystems (Montecino and Lange, 2009; Hutchings et al., 2009; McWhorter et al., 2024; Körner et al., 2024). Coastal regions throughout the tropics are also influenced by low-salinity plumes from river outflow and continental runoff, which impact SST, stratification, ocean circulation, and biogeochemistry (Coles et al., 2013; Li et al., 2017; Silva et al., 2022). Improving the monitoring of nutrients in river mouths and river discharge is critical to better understand the impact of rivers on near-coastal marine ecosystems. Coastal regions account for about 10% of the global ocean surface area but are more efficient at absorbing CO2 than the open ocean and therefore are an important component of the global carbon budget (Roobaert et al., 2019). Their marine ecosystems account for more than 90% of fisheries catches (Garcia and de Leiva Moreno, 2003). Coastal mangrove ecosystems, particularly in tropical regions, play a crucial role in carbon sequestration, shoreline stabilization, and supporting biodiversity, acting as natural buffers against erosion, storm surges, and the impacts of sea-level rise (Alongi, 2014; Donato et al., 2011).
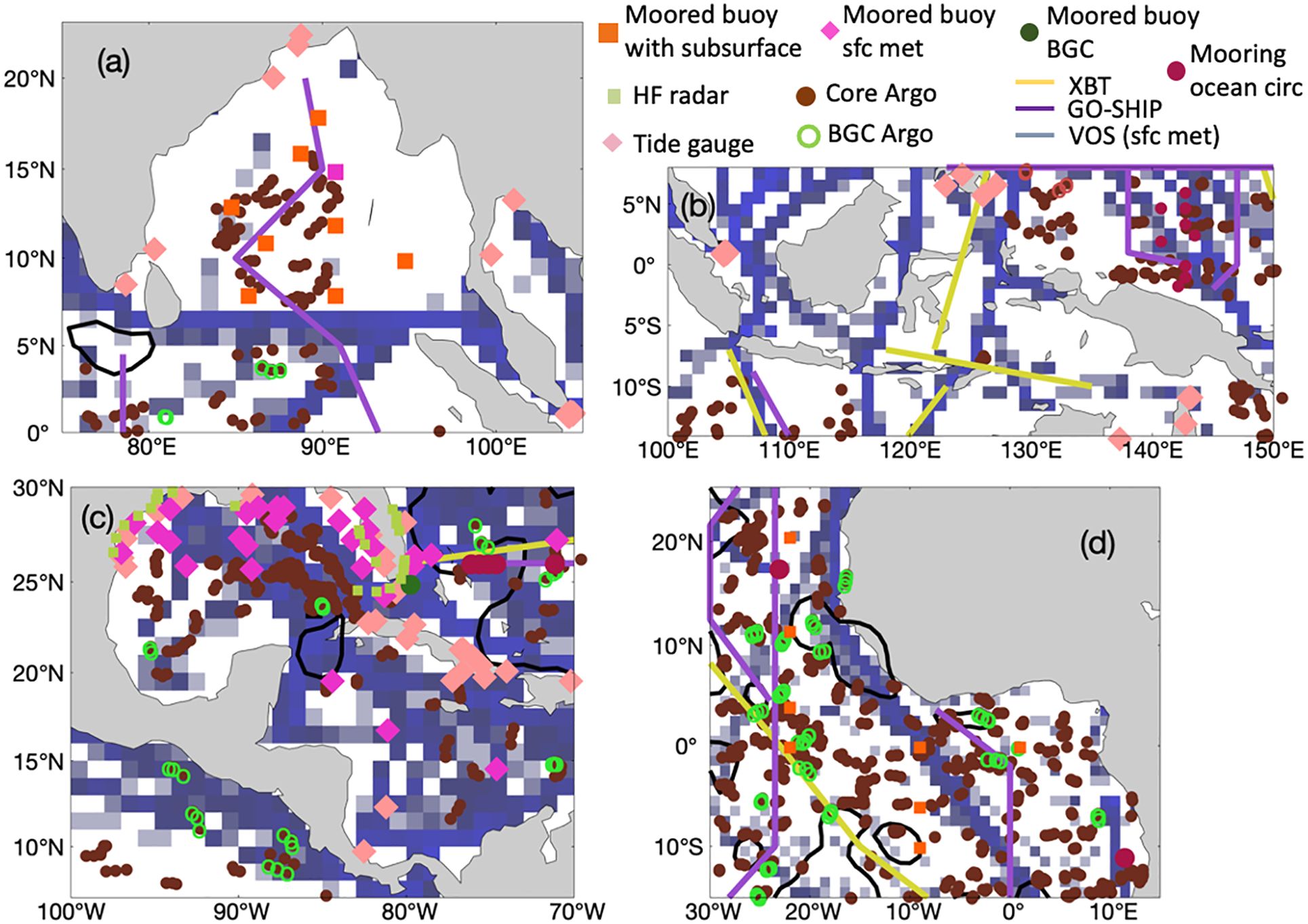
Figure 6. Examples of the sustained coastal observing systems: (A) Bay of Bengal, (B) Maritime Continent, (C) Gulf of Mexico and Caribbean, (D) eastern Atlantic. See Figure 3 for details of the observations and the time periods shown.
Coral reefs and other calcifying organisms are impacted by ocean acidification, pollution, and marine heatwaves (Cooley et al., 2015; Pinheiro et al., 2023), while oxygen minimum zones and harmful algal blooms are exacerbated by ocean warming, changes in salinity, changing seawater carbonate chemistry, and excess nutrient runoff from human activities (Fennel and Testa, 2019; Zohdi and Abbaspour, 2019). It is also important to observe the exchanges of physical and biogeochemical properties between the near-coastal and offshore regions and to gain information to diagnose and predict ecosystem changes. Examples include the impacts of eddies, river runoff, and coastal circulation in the Gulf of Mexico on near-coastal ocean stratification, larval fish transport and dispersion, and hurricane intensification (Compaire et al., 2021; John et al., 2023), and the processes that determine near-coastal primary productivity and carbon storage in the Arabian Sea (Ghosh et al., 2022). Additionally, coastal processes such as river input of nutrients may have far-reaching impacts on ocean biogeochemistry and marine ecosystems throughout the open ocean (Tivig et al., 2024).
There are many unique challenges associated with observing the near-coastal regions of the tropical oceans in a sustained manner. As a result, there are very few quantitative estimates of coastal-open ocean exchanges of physical, biogeochemical, and ecosystem parameters. Shallow bathymetry makes observations difficult from traditional platforms such as Argo floats and surface drifters, which quickly run aground, as indicated by the lack of Argo floats and surface drifters on the continental shelves near most coastlines (brown dots and solid black contours, respectively, in Figures 6A–C). Satellite observations are often not available near the coast with the required resolution and accuracy (Bourassa et al., 2019). Piracy and limited access to the EEZs are major hurdles to sustained coastal observations in the Indian Ocean (Smith et al., 2011; Beal et al., 2020). Resolving these issues requires even closer collaboration and regional involvement than is needed for observations in the open ocean (Iwamoto et al., 2019). Existing near-coastal measurements are usually too sparse to provide the information needed to develop sustained observing systems. Therefore, additional coordinated observations, including those from emerging technologies and new approaches, will likely be required to assess longer term observing needs. One particular nontraditional approach is the global Animal-borne Ocean Sensor network (AniBOS; McMahon et al., 2021; McMahon and Roquet, 2022), which provides valuable temperature and salinity data in poorly sampled regions such as shallow continental shelves, including the ITF and the Timor and Arafura Seas. The additional data from AniBOS, particularly in the upper 100 m, have the potential to aid tropical cyclone research and forecasting in the important near-shore region and farther offshore (Bousquet et al., 2020).
2.4.1 Common recommendations
A strong recommendation from all three tropical observing reviews is to develop process and pilot studies aimed at laying the groundwork for a sustained tropical near-coastal observing system that involves regional participation (e.g., coordination and data sharing) and monitors physical and biogeochemical coastal-open ocean exchanges. The emphasis in those reviews was mostly on coastal upwelling regions, and such studies will likely overlap with and leverage those for boundary currents but must cover wider geographical areas. All tropical observing system reviews recommend increased use of uncrewed underwater vehicles, such as gliders, for monitoring coastal regions that are too shallow for standard Argo floats. The use of profiling floats in shallow coastal regions should continue to be explored (Siiriä et al., 2018). Increased use of uncrewed surface vehicles would also be beneficial for filling gaps left by more traditional platforms such as drifting and moored buoys. Continued expansion of near-coastal high-frequency radar networks is needed for improved monitoring and model assimilation of ocean surface currents (Roarty et al., 2019). Satellite monitoring of sea level, ocean mass, and other important near-coastal signals, must continue. One example is SWOT, which provides high-resolution measurements of sea surface height to within 10 km of coastlines, enabling unprecedented monitoring of sea level to aid model validation and impact assessments and projections. Tide gauges are crucial for calibrating and validating satellite observations, and additional gauges with global navigation satellite system (GNSS) are required to account for vertical motion of the earth’s crust. Expanding the tide gauge network will require increased cooperation with coastal and island states, especially in the Indian Ocean. Improved in-situ monitoring of primary productivity is needed near river mouths, where satellite-based chlorphyll products are not reliable because of turbidity.
For the near-coastal observing system, increased emphasis is placed on collocated near-surface ocean and atmospheric measurements, such as winds, ocean temperature and salinity, surface currents, waves, and surface heat fluxes, to monitor and diagnose changes in coastal upwelling, SST, and cloudiness as well as cross-shore transport of nutrients and phytoplankton and impacts on ecosystems. Currently these collocated observations are mostly limited to moorings, which rarely measure all of the required variables. Coordinated multi-satellite missions to resolve the diurnal cycle of near-coastal winds would greatly aid monitoring and forecasting of coastal circulation and horizontal and vertical exchange processes (Samelson, 2019; Villas Bôas et al., 2019). The most effective observing systems will likely include a combination of established platforms such as moored buoys, Argo floats, ship surveys, and satellites, together with uncrewed underwater and surface vehicles and other nontraditional techniques and low-cost observing systems (e.g., Van Vranken et al., 2020). Continued support for cost-efficient and easily implemented observing techniques and instruments will be required to increase observations globally in the coastal regions. It is crucial to maintain and expand regional and national integrated coastal observing systems such as the U.S. IOOS (Integrated Ocean Observing System) and IMOS (Australia’s Integrated Marine Observing System), which provide valuable near-real-time data for a wide range of services such as operational forecasting, ecosystem management, and climate adaptation. Both IOOS and IMOS are part of a larger network of GOOS Regional Alliances.
Development of international process and pilot studies that include regional partners from the onset is one promising path toward establishing a blueprint and the capacity for sustained observations. An example of such a pilot study is the combined Atlantic glider-saildrone hurricane observing campaigns, which began in 2021. The observations collected by these systems represent many of the uncrewed underwater and surface vehicle tracks in the Atlantic, Caribbean, and Gulf of Mexico in Figure 7. Similar pilot studies would be beneficial in other near-coastal areas of the tropics. The development of regional high-resolution ocean reanalyses is also recommended for key near-coastal regions to help maximize the value of observations, demonstrate the importance and added value of data sharing for regional partners, and aid in the planning of future process and pilot studies. Currently, these coastal reanalyses are lacking in the tropical regions, but have great promise for integrating in-situ and satellite observations with numerical modeling.
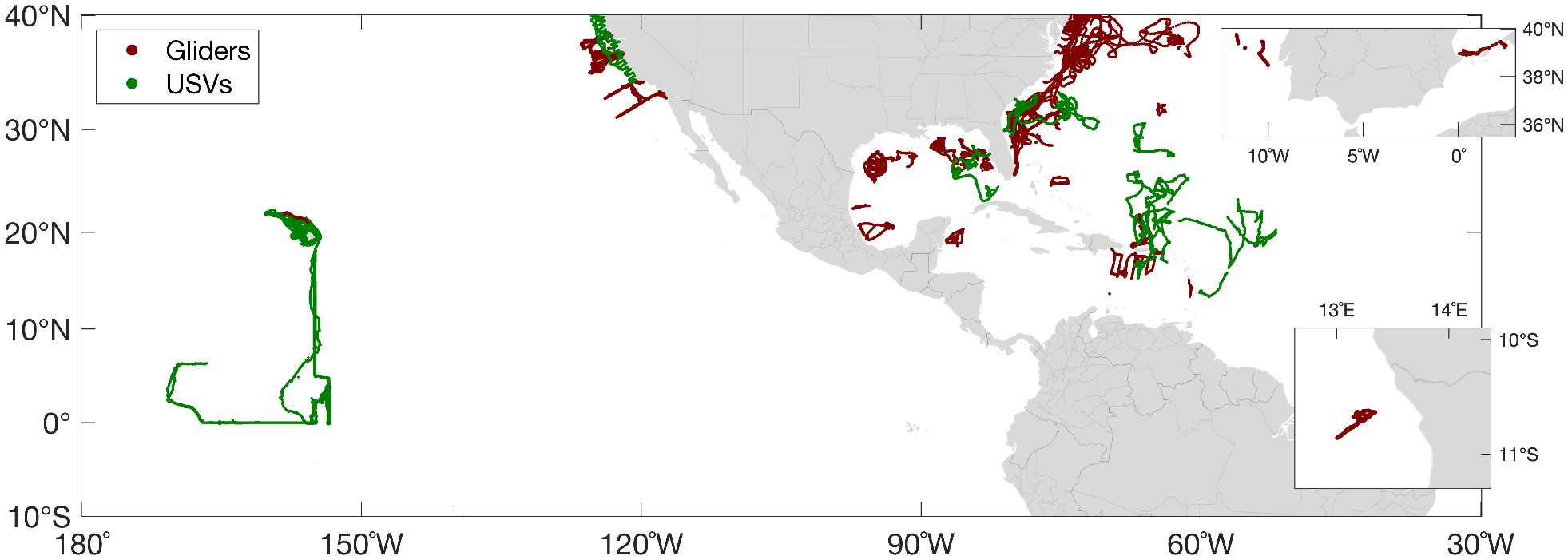
Figure 7. Underwater glider (red) and uncrewed surface vehicle (USV; green) paths during 2023. Data here was collected from the Global Telecommunications System (GTS) for gliders and USVs, and additionally the U.S. IOOS (Integrated Ocean Observing System) Glider Data Assembly Center for gliders and NOAA’s Pacific Marine Environmental Laboratory Saildrone datasets for USVs. The inset map in the top right depicts gliders around Spain and Portugal. The inset map in the bottom right depicts a glider offshore of Angola.
2.5 Extreme events
Extreme events, such as tropical cyclones and marine heatwaves, occur in all tropical basins (Figure 8) and in some cases are likely to become more frequent and intense under climate change (Frölicher et al., 2018; Bhatia et al., 2019). By definition of being extreme, they occur only a small fraction of the time, but they can have disproportionately large societal impacts. The occurrence of extreme events in one basin may be promoted by influences from other basins through atmospheric teleconnections, further emphasizing the need for an integrated observing system. Examples are the known connections between marine heatwaves and climate modes of variability such as ENSO, Indian Ocean Dipole, Pacific decadal variability, the North Atlantic Oscillation, and the Madden-Julian Oscillation (MJO) (Scannell et al., 2016; Holbrook et al., 2019; Capotondi et al., 2022; Gregory et al., 2024; Dutheil et al., 2024). However, the processes behind these teleconnections are not well understood. Marine heatwaves are often driven by persistent high-pressure centers that are remotely forced and can also lead to co-occurring drought conditions over land (Rodrigues et al., 2019; Shi et al., 2021). Marine heatwaves also make tropical cyclone intensification more likely (Dzwonkowski et al., 2020; Choi et al., 2024) and often occur in conjunction with biogeochemical extremes such as ocean acidity and low nutrient and oxygen concentrations, resulting in multiple stressor events for marine species (Gruber et al., 2021; Li et al., 2024). On the other hand, tropical cyclones may abruptly end a marine heatwave (Mawren et al., 2022; Dutheil et al., 2024). Diversity of marine heatwave events, both at the surface and in the ocean subsurface, is only beginning to be explored (Wyatt et al., 2023; Zhang et al., 2023d; Capotondi et al., 2024), and their impacts and synergy with other marine ecosystem stressors are far less understood (Smith et al., 2023).
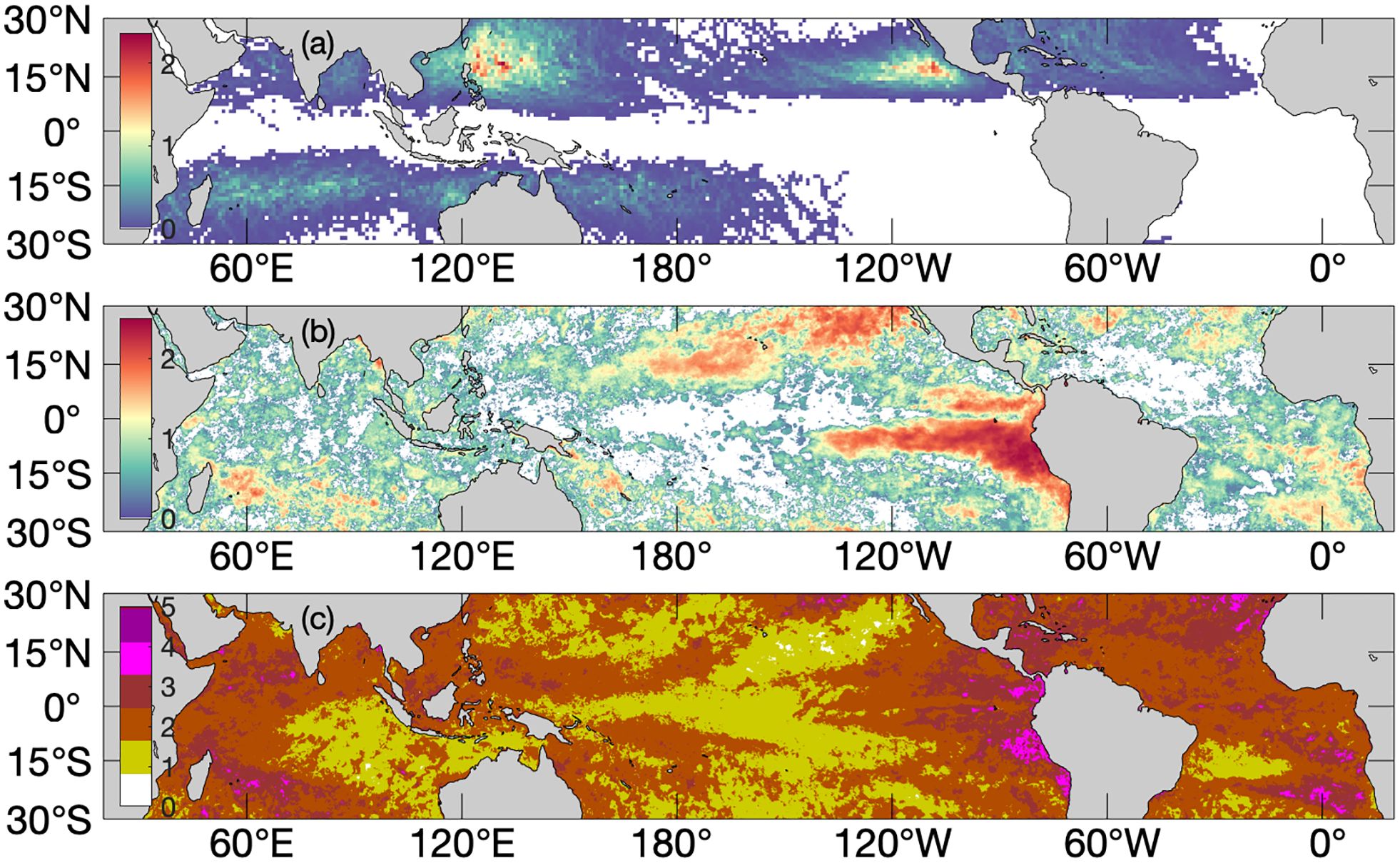
Figure 8. (A) Annual mean tropical cyclone accumulated cyclone energy (ACE) during 1980-2023 (104 kt2). (B) Number of days (log10) with severe marine heatwave conditions (cat. 3-5) during 1980-2023 (data from https://coralreefwatch.noaa.gov/product/marine_heatwave/). (C) Maximum heatwave category experienced during 2023. Heatwave categories are defined in Hobday et al. (2018) and are based on the extent to which SST at a given location exceeds the 90th percentile climatology. For category 1, SST exceeds the 90th percentile but is less than the climatology plus twice the difference between the 90th percentile and climatology; for category 2, SST exceeds the climatology plus twice the difference, but is less than three times the difference, and so on.
Tropical cyclones can present severe threats to coastal communities through storm surges, which are becoming more severe due to sea level rise (e.g., Yin, 2023). Tropical cyclones also influence western boundary currents (Todd et al., 2018; Zhang et al., 2020) and meridional heat transport (Scoccimarro et al., 2011) and leave wakes of turbulent mixing in the thermocline for weeks after they pass, with potential implications for climate (Brizuela et al., 2023). Storm-generated turbulence often transfers heat downward, though in regions like the Bay of Bengal, which is characterized by thick barrier layers and widespread temperature inversions, tropical cyclones can also transfer heat upward (Jarugula and McPhaden, 2022). Operationally, ocean observations provide critical information ahead of storms that aids forecast model initialization and reduces errors in tropical cyclone intensity forecasts (Domingues et al., 2019; Le Hénaff et al., 2021). Future tropical cyclone activity is dependent on the spatial patterns of tropical SST warming, which are not well constrained (Zhao and Knutson, 2024), and tropical cyclone-induced SST cooling is a negative feedback on decadal and longer-term increasing trends of tropical cyclone intensity (Huang et al., 2015; Da et al., 2021). Tropical cyclones also influence biogeochemistry both locally during and after storm passage, and regionally and globally through air-sea CO2 exchange (Bates et al., 1998), and they directly impact coastal and ocean ecosystems (Mumby et al., 2011; Fiedler et al., 2013; Pruitt et al., 2019; Thompson et al., 2023). None of the aforementioned processes are well understood or monitored because of the scarcity of ocean data in and around marine heatwaves and tropical cyclones, and collocated observations of the full air-sea transition zone are especially limited.
An effective observing system must monitor extreme events. The data must be of high quality and available in real-time because of the often rapidly evolving ocean-atmosphere conditions and the need for assimilation into forecast models. This will likely be achieved most effectively through targeted and adaptive observations. In all tropical basins, there is a need for collocated ocean-atmosphere observations in and around tropical cyclones and marine heatwaves to aid data assimilation and forecasts of their spatiotemporal distributions and intensities and to understand and monitor their longer-term effects on the ocean’s physical and biogeochemical states and subsequent effects on ecosystems. An example of an integrated observing system for marine heatwave monitoring is provided by the Australian IMOS Event Based Sampling sub-facility (https://imos.org.au/facility/ocean-gliders/event-based-sampling). Such a system leverages sustained observations from moorings and Argo floats with platforms such as gliders, which can be deployed rapidly at targeted locations to provide surface and sub-surface measurements in near-real-time (Capotondi et al., 2024). The annual Atlantic Hurricane Field Program is another example of a successful strategy for targeted observations in extreme events (Zawislak et al., 2022). However, the primary focus has been on atmospheric measurements mostly in and above the atmospheric boundary layer, with limited deployments of ocean instruments such as expendable probes, floats, and surface drifting buoys. Uncrewed underwater and surface vehicles have been used in the tropical Atlantic since 2015 and 2021, respectively, to help fill some of these gaps (Figure 7; Domingues et al., 2019; Zhang et al., 2023a). Uncrewed systems are also being used to acquire observations within typhoons in the western Pacific (Zhang et al., 2024). Autonomous instruments have also been deployed in the Southern Ocean to gain new insights on the impacts of storms on air-sea carbon exchange (Nicholson et al., 2022; Carranza et al., 2024), and similar observations could be applied to tropical regions. Recently, progress has been made toward a concerted effort to coordinate measurements from uncrewed and crewed atmospheric and oceanic observations as part of NOAA’s Extreme Events Program (https://globalocean.noaa.gov/research/extreme-events-program). Other basins lack similar coordinated programs for observing tropical cyclones, though OMNI (Ocean Moored buoy Network for northern Indian Ocean; Acharya and Chattopadhyay, 2019) is an example of a moored buoy array for monitoring subsurface ocean and near-surface atmospheric conditions in a tropical cyclone-prone region. One of the six user-focused exemplar projects under the GOOS Co-Design Programme is the Tropical Cyclone Exemplar, which focuses on enhancing ocean observations of tropical cyclones and co-designing ocean observing systems to improve tropical cyclone forecasts regionally and globally. Outside of the Indo-Pacific region near Australia, there are no programs that support targeted observing campaigns in other extremes such as marine heatwaves.
2.5.1 Common recommendations
All tropical observing system reviews recognize the value of observations that aid understanding and prediction of tropical cyclones. Historically, the tropical ocean observing systems have not been specifically designed for monitoring, understanding, and predicting extreme events like tropical cyclones, so additional measurements are required. A common recommendation is the establishment of new moored buoys, with surface meteorology, barometric pressure, and subsurface temperature and salinity sensors, in tropical cyclone-prone regions and especially where landfalls are common: the western Pacific, western Atlantic including the Caribbean and Gulf of Mexico, eastern Pacific, the region northwest of Australia, and east of Madagascar (Figure 8A). These additional observations would provide valuable background ocean-atmosphere conditions to aid model initialization and validation and to improve monitoring and process understanding.
Moored buoys provide high-quality collocated ocean-atmosphere measurements, but they cannot be moved from their deployment locations. For this reason, it is recommended to continue observations from uncrewed systems such as underwater gliders and surface vehicles in the Atlantic and in the Indo-Pacific region near Australia, and to develop similar capabilities in tropical areas frequented by marine heatwaves and tropical cyclones but where observations are lacking, such as the Indian Ocean. Ideally these uncrewed observations could be augmented by profiling floats with adaptive profiling in shallow near-coastal regions.
Another common recommendation is to continue to advance capabilities for collocated observations of the ocean and atmospheric boundary layers in extreme weather environments (Clayson et al., 2023a; Zhang et al., 2023a), similar to the ‘super sites’ concept except with mobile and adaptive sampling. These observations should include ocean temperature, salinity, currents, directional wave spectra, air-sea CO2 flux, and chlorophyll, as well as the parameters required for bulk surface heat flux calculation and some high-frequency measurements (10-20 Hz) for direct covariance heat and momentum fluxes. Surface-based active and passive atmospheric profilers are also needed to measure boundary layer height, winds, temperature, and humidity and their links to the ocean. It is critical to maintain satellite observations, especially SST, surface salinity, sea level, rainfall, and scatterometer and synthetic aperture radar winds. Enhancements are also needed, such as continued improvements in near-shore wind measurements.
Though there are no common recommendations for marine heatwaves in the tropical observing system reviews, likely because of their more recent emergence as an important phenomenon and distinct underlying dynamics in each basin, discussions are underway to develop effective strategies to observe these events. In particular, there is a specific need to monitor marine heatwaves in the subsurface. Most of the studies to date monitor marine heatwaves from global satellite data, thus only capturing their surface signatures. Recommendations are to enhance the temporal and spatial resolutions of subsurface measurements, mainly in the coastal regions. Thus, many of the recommendations for the coastal observing system (Section 2.4) and tropical cyclones also apply to marine heatwaves because of their strong impacts near the coast and the need for adaptive sampling. When designing the observing system for tropical cyclones, marine heatwaves, and other extreme events, it is crucial to involve modeling and forecasting communities, and regional stakeholders, who can help guide the choice of observations that are most needed. These strategies are currently being carried out by the U.N. Ocean Decade endorsed GOOS Co-Design Programme Tropical Cyclone and Marine Heatwave Exemplars.
2.6 Strategy for sustained biogeochemical and ecosystem observations
The tropical basins play critical roles in global biogeochemical cycles, representing the main return pathway of ocean carbon to the atmosphere (Takahashi et al., 1997; Nakano et al., 2015) and a highly efficient biological pump that draws carbon to depth (DeVries et al., 2012). Upper-ocean physics (Section 2.2) and air-sea interactions strongly modulate this carbon flux and export primarily along the equatorial cold tongues and coastal regions (Takahashi et al., 2009), where cold waters rich in dissolved inorganic carbon (DIC) and nutrients are upwelled and warmed at the surface, driving intense carbon outgassing (Figure 9A). Phytoplankton utilize DIC and nutrients (nitrate, phosphate, iron) and produce organic carbon that sinks to depth and is remineralized by microbial consumption of dissolved oxygen (O2). This large removal of O2 is only weakly replenished by sluggish ventilation (Wyrtki, 1962), sustaining large oxygen minimum zones (OMZs) in the tropics (Brandt et al., 2015) and intense production of nitrous oxide, a powerful greenhouse gas. The supply of carbon, oxygen, and nutrients to the tropics is driven mainly by equator-bound mode and intermediate waters (Ryan et al., 2006; Ishii et al., 2020; Gray, 2024). These biogeochemical properties are further modulated locally by ocean dynamics and air-sea interactions, influencing the observed intensity and variability of tropical carbon outgassing (Figure 9A), OMZs (Figures 9C, D), and biological productivity (Figure 9B) and their impacts on the global carbon cycle and upper trophic levels (Chavez et al., 1999; Strutton et al., 2001; Resplandy et al., 2012).
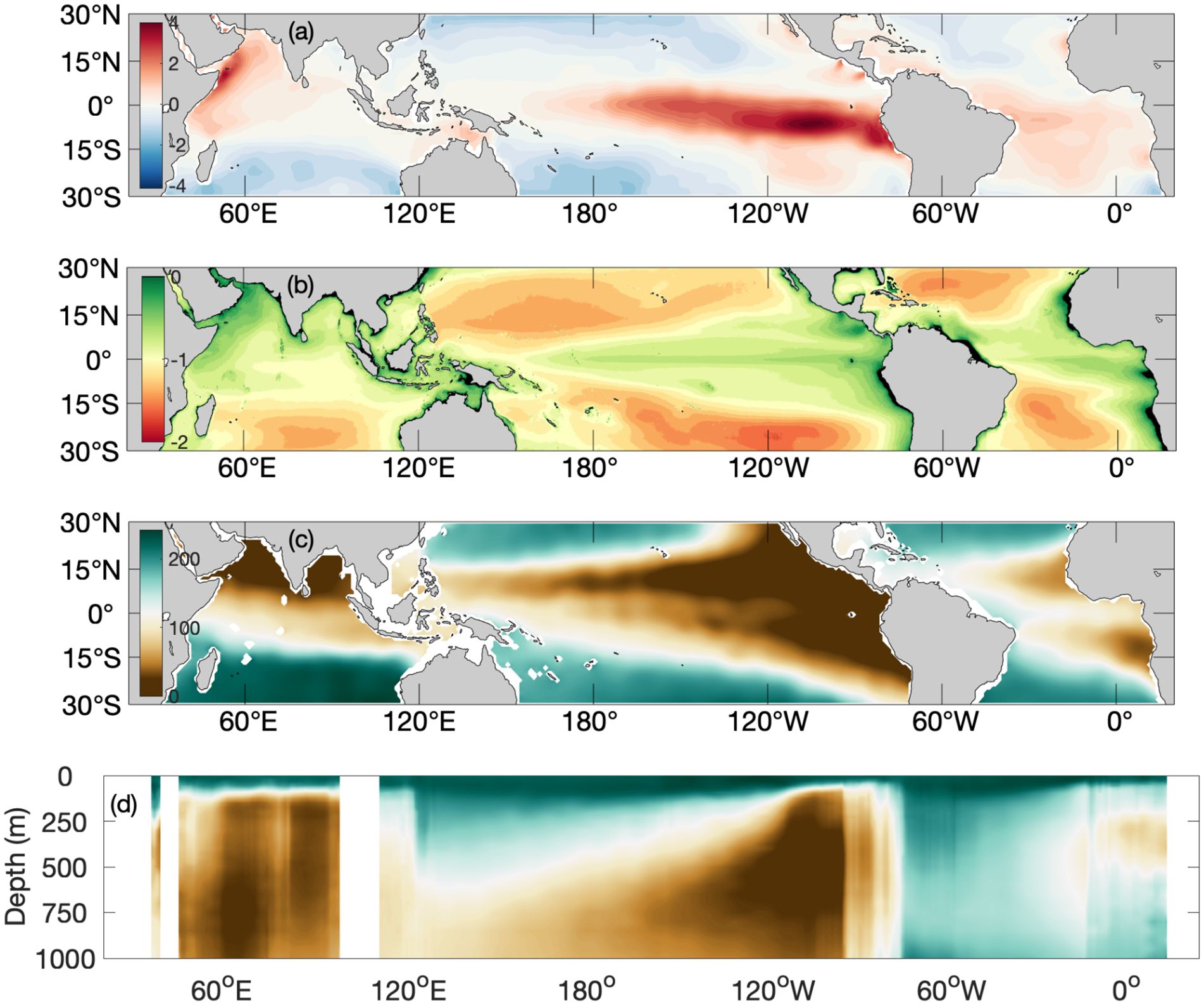
Figure 9. (A) Annual mean surface CO2 flux (mol m-2 yr-1) from the Max Planck Institute (MPI) merged product (https://www.ncei.noaa.gov/data/oceans/ncei/ocads/data/0160558/MPI_SOM-FFN_v2022/). Positive values denote carbon outgassing. (B) Annual mean surface chlorophyll concentration (log10[mg m-3]) from a combined satellite analysis (http://www.esa-oceancolour-cci.org/). (C) Annual mean ocean oxygen concentration (µmol kg-1) at a depth of 450 m from WOA18 (Garcia et al., 2019). (D) Annual mean oxygen concentration with depth averaged between the equator and 30°N as a function of longitude. The color scale is the same as in (C).
Development of a strategy for sustained pantropical observing networks should take into account shared gaps in ocean biogeochemical processes across the tropics. Many fundamental questions remain, particularly on the roles of climate and ocean physical and biological processes in driving the intensity and variability of (1) carbon outgassing and its export to depth (Lefèvre et al., 2024; Pittman et al., 2022), (2) ventilation of the OMZs (Brandt et al., 2015), and (3) the nutrient supply to the euphotic zone and its influence on primary productivity. The large-scale mean structures of some of these variables are known (Figure 9), but data are often too sparse (Figure 10) to support studies on intraseasonal, interannual, and longer timescales and on small spatial scales (e.g., mesoscale and below). A key uncertainty concerns the relative roles of remotely-driven changes in anthropogenic carbon transport via mode and intermediate water masses, compared to local changes in upwelling of DIC and carbon export, in driving variability in the tropical carbon flux (Nakano et al., 2015; Pittman et al., 2022). The tropical OMZs have been expanding in recent decades as the oceans warm and stratify (Stramma et al., 2008) but a direct mechanistic link for the OMZ expansion remains unclear (Oschlies et al., 2018), in part due to poor observational constraints on the oxygen budget (Brandt et al., 2015). The attribution of anthropogenic trends in the tropics is made more challenging by weak signal-to-noise ratios and large biases for biogeochemical parameters in models well into the 21st century (Schlunegger et al., 2020; Oschlies et al., 2018; Séférian et al., 2020).
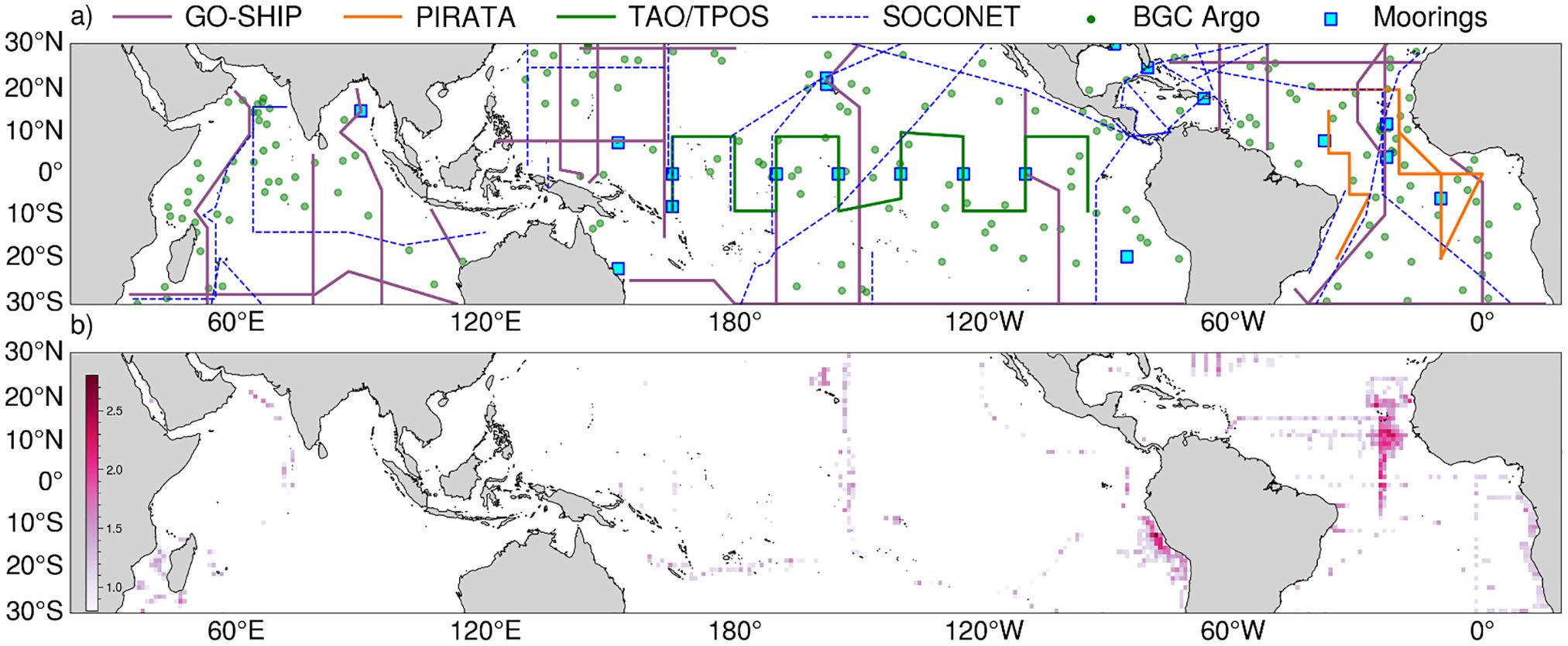
Figure 10. (A) Global tropical BGC observing system as of April 2024. The majority of in-situ sampling from moorings (cyan boxes) and volunteer observing ships (dashed blue) mainly target surface parameters (pCO2) as part of SOCONET (Wanninkhof et al., 2019). Mooring servicing cruises (green and orange lines for TAO/TPOS and PIRATA respectively) additionally often measure subsurface O2, chlorophyll, and/or nutrients. GO-SHIP (purple lines) and BGC Argo (green circles) sample a range of subsurface BGC parameters, including dissolved oxygen, pH, nutrients, and chlorophyll amongst other variables. (B) Total number (log10) of observations of plankton size distributions since 1996, from underwater vision profilers and onboard images and scanners, summed overall size ranges (Dugenne et al., 2024).
Earth system models also diverge widely in their future projections of tropical ocean primary production (Séférian et al., 2020; Modi and Roxy, 2023), reflecting poor representation, or lack thereof, of key physical and biogeochemical processes that are not well observed in the tropics. Little is known about phytoplankton and zooplankton community structure and how they vary spatially and temporally, which in turn affect nutrient utilization and the biological carbon pump, including particulate organic carbon (POC) and dissolved organic carbon (DOC). Phytoplankton growth is often limited by one or more nutrients (Browning and Moore, 2023). Knowledge and improved modeling of the physical processes that enhance nutrient availability, and how effectively the nutrients are utilized by phytoplankton, can aid ecosystem forecasts and projections. There are also physical teleconnections between tropical basins that generate remotely-forced ecosystem and fisheries variability (Lopez-Parages et al., 2020; Gomara et al., 2021). Improved understanding and modeling of these processes and phenomena must be combined with advances in biogeochemical data assimilation, which lags far behind physical data assimilation, due in part to extremely limited biogeochemical observations (Fennel et al., 2019).
Integrated global CO2 (Wanninkhof et al., 2019) and plankton (Batten et al., 2019) networks and datasets have been developed much more recently than corresponding networks for physical variables (Boyer et al., 2018; Freeman et al., 2017). A critical need across the tropical observing networks is enhanced subsurface observations of ocean biogeochemistry, particularly for key parameters such as O2, chlorophyll, nutrients, and carbon including its inorganic (e.g., pH and DIC) and organic (POC and DOC) components. Concurrent measurements of these parameters provide constraints on carbon export and organic remineralization cycles and their coupling. They can also inform physical processes, such as upwelling, mixing, and water mass sources, due to pronounced gradients of biogeochemical properties in the upper tropical oceans (e.g., Cromwell, 1953; Wyrtki and Kilonsky, 1984). In-situ surface observations of chlorophyll are critical for calibrating and validating satellite retrievals (Sá et al., 2015; Buman et al., 2022). Measurements of chlorophyll through the water column can only be acquired through in-situ observations and are essential for monitoring the base of the marine food web and biological pump. These subsurface observations are needed for monitoring the deep chlorophyll maximum, a dominant feature of the tropical basins (Cornec et al., 2021), and are relevant for characterizing the penetration depth of solar radiation and its impact on tropical ocean circulation (Murtugudde et al., 2002). There are even larger observing gaps for zooplankton, the trophic level between phytoplankton and fish.
2.6.1 Common recommendations
Given the severe undersampling of biogeochemical and ecosystem variables throughout the tropics, and especially the Indian Ocean, a top priority for the sustained observing system is to maintain and expand the present observational efforts aimed at providing broad global coverage of essential ocean biogeochemical and ecosystem variables (Miloslavich et al., 2018). Emphasis in all basin observing system reviews was put on maintaining and expanding (1) surface ocean pCO2, (2) BGC Argo, (3) underway sampling and profiling from ships, and (4) satellite ocean color. These are summarized in the following paragraphs.
There is a 40-year observational record of pCO2 in the tropical Pacific from ships (Feely et al., 2006) and for the past 20 years from moorings (Sutton et al., 2014). Similar records began more recently in the tropical Atlantic (Lefèvre et al., 2024), and there are much fewer observations in the Indian Ocean. It is important to maintain and expand these measurements from ships and moorings, and advance monitoring capabilities using uncrewed surface vehicles. It is also essential to expand BGC Argo coverage and the number of parameters measured on each float. BGC Argo floats can now sample nitrate, dissolved oxygen, pH, chlorophyll fluorescence, particulate backscatter, and downwelling irradiance every 10 days. They are currently the best and most cost-efficient means to achieve regular subsurface open ocean monitoring throughout the global tropics, with potential for addressing long-standing inequities in sampling BGC across the tropical basins. The global BGC Argo float array currently includes 683 floats, with the aim of reaching 1,000 floats over the next decade (Johnson and Claustre, 2016; Claustre et al., 2020). About 500 of these floats are expected to be deployed and maintained throughout the tropical basins, guided by optimized strategies as feasible (Chamberlain et al., 2023) and according to specific tropical observing system needs and basin sizes (Smith et al., 2019; Foltz et al., 2019; Beal et al., 2020).
Another common recommendation from all tropical observing system reviews is to add underway and profile measurements (e.g., CTD, bottle samples) of essential variables such as pCO2, O2, N2O, pH, DIC, DOC, nutrients, and plankton using Continuous Plankton Recorders (CPR), alongside physical variables. These essential data should be collected during all tropical moored buoy servicing cruises and expanded to include underway sampling from voluntary observing ships. Complementing these in-situ observations, global satellite measurements of ocean color extend back more than two decades, providing critical information on this base of the ocean ecosystem and its interactions with biogeochemistry and the physical climate system. Satellite remote sensing of ocean color provides stable observations with high spatial and temporal resolutions over long periods, supporting concurrent estimation of pCO2 and air-sea CO2 fluxes. These records must be maintained while ensuring precision and accuracy through overlap and sensor redundancy across platforms and missions. More recent sampling of plankton community structure and diversity through hyperspectral remote sensing missions (e.g., Plankton, Aerosol, Cloud, ocean Ecosystem (PACE)) will require rigorous validation through in-situ sampling.
In addition to maintaining and expanding the existing observations in all tropical oceans, pilot studies are needed to improve knowledge of the temporal and spatial scales of biogeochemical and ecosystem variability and inform the design of a more comprehensive and sustained observing system. These studies should start in areas with large signals, such as the equatorial and coastal upwelling zones with high productivity and CO2 outgassing (Figures 9A, B), and oxygen minimum zones (Figures 9C, D), building on existing programs such as BGC Argo and GO-SHIP. Emerging technologies such as uncrewed surface and underwater vehicles (Sabine et al., 2020) will be key for filling large gaps in biogeochemical and ecosystem observations, particularly in near-coastal areas (Figures 6, 7), and for exploring new phenomena at finer scales (Nicholson et al., 2022; Carranza et al., 2024).
The development of standardized methods for ecosystem data collection is highly recommended, along with cross-comparisons and inter-calibration between different measurement techniques and instruments. This will provide a pathway toward integration of essential measurements (e.g., plankton) into existing observing efforts such as GO-SHIP, moored buoys, and BGC Argo (Lombard et al., 2019). Improved international coordination, especially with regional partners and stakeholders, is needed to link fisheries, ecosystem, and environmental information from all sources to allow monitoring in the context of climate variability and change and reassessments of user needs (Schmidt et al., 2019).
2.7 Framework for evaluating observing system needs
Data from the observing systems improve weather and climate modeling and forecasts (Fujii et al., 2011; Zuo et al., 2019), but it is unclear to what extent the observations are optimized for that purpose. Observing System Experiments (OSEs) run models with and without certain existing observations to assess the impact of those observations. They are an effective way to diagnose the value of certain observations for data assimilation and forecasts (Fujii et al., 2015; Xue et al., 2017), but they do not inform observing system design, and their usefulness can be limited due to model errors, assimilation errors, and error compensation (Subramanian et al., 2019; Penny et al., 2019). Observing System Simulation Experiments (OSSEs) test the ability of different hypothetical observational configurations to improve model-simulated fields. They have been used successfully for guiding individual observing systems for specific purposes, such as physical ocean variables in individual basins and regions (Vecchi and Harrison, 2007; Oke and Schiller, 2007; Gasparin et al., 2019), Indian Ocean pCO2 (Valsala et al., 2021), and to determine the BGC Argo array size needed for monitoring biogeochemical processes (Majkut et al., 2014; Kamenkovich et al., 2017; Chamberlain et al., 2023). Conducting OSSEs for a basin-wide observing system across multiple time-space scales and many ocean-atmosphere variables would be very complex, especially given significant errors in most coupled climate models. However, further development, use, and intercomparison of OSSEs for individual components of the tropical observing systems is a promising path toward quantifying requirements for specific needs.
2.7.1 Common recommendations
Large-scale efforts are needed to investigate deficiencies in data assimilation systems and determine how existing data can be used most effectively to constrain reanalyses and forecasts. It is often unclear how much of the model uncertainty and error results from misrepresentation of underlying processes in the model versus observational uncertainty and measurement error. It is also difficult to create a suitable metric that quantifies the influence of observations, which is the first step. To address this, multi-system evaluation across different data assimilation schemes and forecast models is recommended. Information exchanges among centers on observation utilization (which observations are ingested) and influence (analysis increments or more sophisticated metrics) are recommended, and should be routinely assessed. This will require greater international coordination. There is also a need to strengthen collaborations between the data assimilation, modeling, and observing communities so that each understands the needs and capabilities of the others. Continued development of strongly coupled data assimilation is highly recommended, as it has potential to reduce systematic errors because ocean observations can directly affect the atmospheric state, and conversely atmospheric observations affect the ocean. Due to pronounced gradients of biogeochemical variables in the tropics, enhanced biogeochemistry observations and their assimilation in ocean models (e.g., Verdy and Mazloff, 2017) can provide additional constraints on ocean circulation and climate that are not possible from physical variables alone.
2.8 Data management and integration
The main purpose of all tropical observing systems is to provide data to a range of users, from individual researchers to operational weather forecast centers. Common requirements are high-quality data that are publicly accessible in standard formats, and appropriate descriptions of the data (i.e., metadata). It is important to provide near-real-time data for assimilation into forecast models and to aid forecaster situational awareness. Usually these data streams have minimal and/or automated quality-control (QC), if any, and more stringent QC is performed by the operational centers using the data. Delayed-mode, QC’d data is also a requirement for scientific research, monitoring, and model assessments. It is difficult to provide one delayed-mode QC’d product that satisfies all diverse users, so it is also important to retain the original unaltered data.
There are several data management challenges that are common across all tropical observing systems. There is a large volume of diverse data from a diverse and ever-changing collection of conventional and emerging platforms and sensors, each with its own unique measurements, sampling rates, data formats, and QC procedures. It is not always clear how to incorporate these data streams into the observing system. Promoting the exchange of community practices and standardized approaches among the pantropical observing community can enhance interoperability and improve overall system performance. One of the biggest challenges is finding the resources to support regular and consistent data management, including transmission of real-time data, QC and archiving of delayed-mode data, and adequate metadata documentation. In general, data management is poorly supported compared to the acquisition of the data itself.
2.8.1 Common recommendations
The main recommendation for tropical basin observing system data management and services is to continue to work toward FAIR principles (Findability, Accessibility, Interoperability, Reusability; Tanhua et al., 2019). Overall, the individual observing system reviews did not find any major gaps in terms of data management and services, though in some cases more integration of related datasets is recommended. One such example is to collect all data from moored buoy servicing cruises (e.g., underway surface measurements and vertical profiles of temperature, salinity, ocean currents, oxygen, nutrients, and chlorophyll from CTD casts) and provide it through a single web service in a common format. There are often separate data repositories for physical, biogeochemical, and biological data from moored buoys. Data integration is needed to merge related datasets, such as surface heat and carbon fluxes and the parameters required for their calculation. The GOOS Observations Coordination Group (OCG) Open Access to the Global Telecommunications System (Open-GTS) pilot project has great potential to expand accessibility to real-time data through improved web-accessible tools and visualizations. Currently, it can be very difficult to gather and assemble real-time in-situ data from different platforms through the GTS or from individual projects’ data portals. In general, all tropical observing system reviews recommend reserving at least 10% of the cost of the observing system for data management to ensure that data are processed routinely using FAIR principles and are available to the community.
3 An integrated observing system for pantropical basin interactions
Beginning with the discovery of ENSO atmospheric teleconnections (Bjerknes, 1969), there has been increasing appreciation that variability in the tropical oceans more generally can influence global weather patterns and climate. It is also well known that variability outside the tropics can strongly impact tropical weather and climate (Behera and Yamagata, 2001; Liu et al., 2020; Deng and Dai, 2024). These close two-way connections motivate the advancement of the broader Global Ocean Observing System in which the tropical ocean observing system is embedded. It has been established more recently that all tropical basins interact (Figure 11). The Indian Ocean Basin Mode affects the western Pacific and ENSO (Watanabe and Jin, 2002; Annamalai et al., 2005), while the Indian Ocean Dipole modulates ENSO amplitude and phasing (Izumo et al., 2010) and equatorial Atlantic variability (Zhang and Han, 2021). The equatorial Atlantic affects ENSO (Rodriguez-Fonseca et al., 2009) and the Indian summer monsoon (Kucharski et al., 2008), while SST variability in the tropical North Atlantic can alter tropical Pacific wind patterns and ENSO (Rong et al., 2010; Ham et al., 2013). The tropical Pacific and Indian Oceans also interact via the ITF (Section 2.3), with major implications for ocean biogeochemistry and ecosystems (e.g., oxygen, Ditkovsky et al., 2023). Anomalous carbon, oxygen, and nitrous oxide fluxes propagate quickly beyond their original tropical sources, with major influences on their global atmospheric budgets. Tropical basin interactions have been the subject of comprehensive reviews (Wang, 2019; Cai et al., 2019), and the needs for deeper understanding of tropical basin interactions and their impacts on seasonal to decadal forecast skill motivated CLIVAR’s Tropical Basin Interaction Research Focus (https://www.clivar.org/research-foci/basin-interaction), which started in 2020 and is active through 2025.
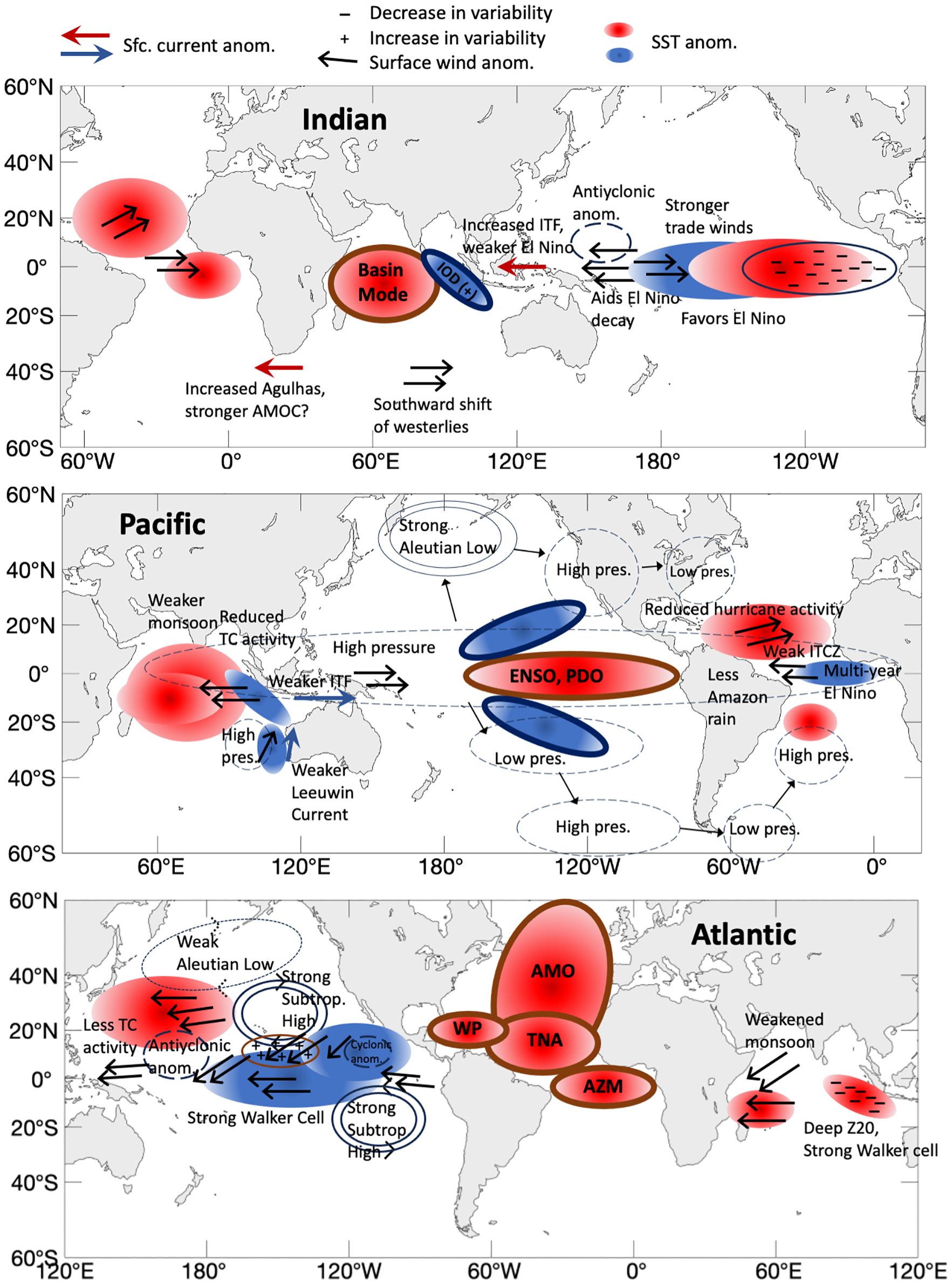
Figure 11. Schematics of tropical basin interactions. Top: Impacts of the tropical Indian Ocean on the Pacific and Atlantic. Middle: Impacts of the tropical Pacific on the Indian and Atlantic. Bottom: Impacts of the tropical Atlantic on the Indian and Pacific. Red arrows indicate ocean currents that increase heat transport. Blue arrows indicate currents that decrease heat transport. Dashes inside ovals indicate that SST variability is reduced and pluses show where variability is enhanced. Red and blue ovals with thick borders show warm and cold SST anomalies, respectively, that drive the variability in other basins.
Important societal benefits can be gained from an integrated observing system for pantropical interactions because of the potential for greater forecast skill. ENSO is the main source of global climate predictability on seasonal timescales, including strong links to the tropical Atlantic (Saravanan and Chang, 2000) and Indian Ocean (Dommenget and Latif, 2002). ENSO also responds to conditions outside the tropical Pacific. Proper representations of tropical Atlantic and Indian Ocean variability increase ENSO forecast skill (Frauen and Dommenget, 2012; Keenlyside et al., 2013; Terray et al., 2016; Zhao et al., 2023b, 2024; Exarchou et al., 2021) because initial conditions in the Atlantic and Indian Oceans, and their multi-month persistence, lead to better representations and predictions of tropical basin interactions (Zhao et al., 2024). Accurate initial conditions, and forecasts of their changes with time, rely on an integrated pantropical observing system that provides information in areas and on timescales that are most beneficial for forecast model initialization. In addition to aiding forecasts directly, the global tropical observing system must provide the data required to advance understanding of tropical basin interactions that occur through ocean and atmospheric pathways.
Needs and recommendations for tropical basin interactions include all of those discussed in Sections 2.1-2.8 because understanding and predicting interactions between basins require comprehensive observations of processes and phenomena within each individual basin. In addition, a broader global perspective is required. Here we emphasize some of the most important requirements for advancing tropical basin interaction research and improving forecast model initialization. Though our focus is on the ocean observing system, we recognize the crucial need for atmospheric measurements above the air-sea interface because most tropical basin interactions are communicated through the atmosphere.
3.1 Global satellite measurements for continuous and overlapping time periods
Most tropical basin interactions are facilitated by atmospheric teleconnections that are driven by changes in SST. However, the pathways are often unclear, especially the links between atmospheric convection in one basin and the resultant changes in atmospheric and oceanic conditions in another. The continuation and advancement of global tropical satellite observations is crucial for continued progress in tropical basin interaction research and forecasting. Important variables include SST, near-surface wind, outgoing longwave radiation, precipitation, chlorophyll, and atmospheric temperature and humidity profiles. It is essential to ensure that there is overlap between satellite missions to aid intercalibration. In-situ data are also invaluable for this purpose.
3.2 Multidecadal data records
Many of the existing observational records are too short for meaningful analyses of decadal-multidecadal tropical basin interactions and long-term modulations of seasonal-interannual interactions. Distinguishing the roles of natural variability and anthropogenic climate change can also be extremely challenging because of the short records, and there are ongoing questions about the impacts of changing observing systems on multidecadal datasets and associated trend detection. It is important to maintain the measurement of the EOVs of the existing sustained observing system to build consistent records into the future (Section 2.2). This is particularly important for ocean observations because of the ocean’s longer memory in the climate system. It is also critical to continue atmospheric measurements of greenhouse gases, clouds, and aerosols, which can have strong imprints on decadal-multidecadal climate variability and the formation of warming patterns. Continued efforts to extend important datasets back in time through paleoclimate proxies must be continued, as they provide the potential for significantly more rapid lengthening of time series, though at reduced temporal and spatial resolutions, compared to ongoing satellite and in-situ observations.
3.3 Tropical ocean boundaries
The tropical oceans communicate directly through the ITF and indirectly through the Agulhas leakage (Section 2.3). However, the impacts of decadal-multidecadal interbasin ocean exchanges on global climate are poorly known because of limited sustained Agulhas Current and ITF observations. These pathways must be monitored in order to understand and predict global tropical variability and interactions between basins on interannual to multidecadal timescales. For pantropical interactions, the most important variables to measure are monthly horizontal mass, heat, and freshwater fluxes from the surface to at least 500 m, and to at least 1000 m in the Agulhas Current and downstream leakage region. Concurrent measurements of key biogeochemical quantities should also be undertaken as feasible to quantify and monitor changes in interbasin exchanges of carbon, oxygen and nutrients, particularly along the ITF. Shorter-duration process studies are also valuable for assessing vertical and diapycnal fluxes driven by upwelling and mixing.
3.4 International coordination
Establishing an integrated pantropical ocean observing system for understanding and predicting the extensive ocean-atmosphere connections between tropical basins will likely require extensive international coordination along the lines of the Intergovernmental Oceanographic Commission’s GOOS. Currently, observing systems are often implemented with regional contributions from organizations that have their own specific priorities. This is beneficial because it ensures that stakeholders provide resources to help build and sustain the observing system in ways that meet their needs. However, it does not necessarily translate to a broader coordinated effort, particularly given the increasingly critical role of global autonomous sampling programs (e.g., Argo) in monitoring the tropical oceans. Future challenges involve determining pathways for more coordinated observing efforts and establishing criteria for assessing the added value of the coordination and the balance between regional and broader, but more complex, coordination.
4 Discussion
The need for ocean observations has never been greater. The motivations that drove advancements of the tropical observing system beginning in the 1980s, such as ENSO and other tropical climate modes, still exist and in many cases are becoming more challenging to understand and predict because of decadal and longer timescale modulations of their behaviors. The more recent efforts to bring tropical in-situ observations, including surface drifting buoys, Argo floats, and moored buoys, to their target numbers took place against the backdrop of accelerating global warming and associated fundamental changes to weather, climate, and ocean ecosystems. With the increasing frequency of disruptive climate and weather events, the demand for global tropical ocean observations will only increase. The global tropical observing system must proactively evolve and anticipate these needs.
The tropics are expected to experience high rates of change in temperature and precipitation extremes over the next 20 years (Iles et al., 2024; Roxy et al., 2024), exposing high-density human populations to increasing risks from extreme events (Jing et al., 2024). An important finding from this review, consistent with a recent air-sea transition zone report (Clayson et al., 2023a), is that the present tropical observing system is inadequate to address the growing needs to observe, understand, and predict extreme weather and climate events, such as marine heatwaves and tropical cyclones. The deficiencies are especially acute in near-coastal regions, where the societal impacts are often greatest, and in the subsurface ocean, which satellite observations cannot monitor. Marine heatwaves in particular can have severe impacts on ocean ecosystems. Currently, many of the tropical nations and island communities that may benefit the most from the observational networks are not well connected to the observing communities. Adapting the present observing system to provide the high-frequency real-time observations needed to advance understanding, prediction, and adaptation will require technological advancements, international coordination, and involvement of local stakeholders. Continued innovations are needed to aid in-situ monitoring, including uncrewed underwater and surface vehicles, and to expand satellite observations, such as potential missions to measure total surface currents (Ardhuin et al., 2018; Gille et al., 2022; Torres et al., 2023) and the state variables required to estimate surface heat fluxes (Clayson et al., 2023b). Full-depth ocean heat and carbon storage must be monitored globally, since long-term changes in these parameters strongly affect global warming and resultant changes in extreme events.
A second major conclusion is that the global tropics are severely undersampled in terms of biogeochemistry and ecosystems, given the importance of the tropical ocean for the global carbon cycle and the societally relevant changes in ocean ecosystems driven by pronounced tropical climate variability and trends. It is crucial to maintain existing ship, mooring, and satellite-based observations while advancing BGC Argo to its optimized target density and full range of BGC sensors. This will serve as a starting point for designing and implementing more extensive in-situ biogeochemistry and ecosystem observational programs, building on the recently launched PACE (Plankton, Aerosol, Cloud, and ocean Ecosystem) satellite and the future GeoXO (Geostationary Extended Observations), which will provide high-resolution (390 m) ocean color and water quality data every two hours with the capability to distinguish between different phytoplankton types. Recent advances in uncrewed and autonomous sampling should be leveraged to fill the sampling gaps left by the existing networks and to explore high-frequency variability at finer spatial scales.
The COVID-19 pandemic highlighted vulnerabilities in global observing systems, including disruptions to data collection and maintenance due to restrictions on travel, ship operations, and fieldwork, as well as supply chain issues for procurement and refurbishment of equipment (Boyer et al., 2023; Sprintall et al., 2024). The Indian Ocean RAMA array, which before COVID-19 had data returns of 87%, was reduced to 2% data return in 2022 (Connell et al., 2023). Redundancy in observations—through overlapping systems, diverse platforms, and multiple data streams—helps ensure continuous data flow even when individual components are compromised. By proactively integrating these elements into the design of pantropical observing systems, the scientific community can better safeguard critical ocean data and ensure continuous monitoring of climate variability, extreme events, and ecosystem changes.
An integrating theme for the tropical observing system is pantropical basin interactions, which demand international and transbasin coordination and strengthening of regional organizations. The challenges of understanding and predicting variability in individual basins are greatly amplified for tropical basin interactions, which require pantropical observations of the ocean and atmosphere on timescales ranging from intraseasonal to multidecadal. It is crucial to develop an observing system that considers the ocean and atmosphere in an integrated manner, including collocated measurements throughout the air-sea transition zone that encompasses the oceanic and atmospheric boundary layers. The observing system must continue to advance in order to better understand and predict these tropical basin interactions, while maintaining the valuable observing system of the present.
Author contributions
GF: Conceptualization, Project administration, Software, Visualization, Writing – original draft, Writing – review & editing. YE: Conceptualization, Writing – original draft, Writing – review & editing, Visualization. JS: Conceptualization, Writing – original draft, Writing – review & editing. AC: Conceptualization, Writing – original draft, Writing – review & editing. SC: Conceptualization, Writing – original draft, Writing – review & editing. PB: Conceptualization, Writing – original draft, Writing – review & editing. AJS: Conceptualization, Writing – original draft, Writing – review & editing. TM: Conceptualization, Writing – original draft, Writing – review & editing. JH: Conceptualization, Writing – original draft, Writing – review & editing. CM: Writing – original draft, Writing – review & editing. MM: Conceptualization, Writing – original draft, Writing – review & editing. LL: Visualization, Writing – review & editing. FT: Visualization, Writing – review & editing. MR: Writing – original draft, Writing – review & editing. FW: Writing – review & editing. FC: Writing – review & editing. RR: Writing – original draft. BR-F: Writing – review & editing. AS: Writing – review & editing. MD: Writing – original draft. CS: Writing – review & editing. KB: Writing – review & editing. WY: Writing – review & editing.
Funding
The author(s) declare that financial support was received for the research, authorship, and/or publication of this article. GF was supported by base funds to NOAA AOML’s Physical Oceanography Division. JS acknowledges support from NOAA GOMO NA20OAR4320278 and NSF OCE OCE-1851316. YE acknowledges support from NSF OCE-2421546 and NSF OCE-1948599. AC was supported by the NOAA CPO CVP award #NA24OARX431C0024-T1-01.
Acknowledgments
This is PMEL contribution 5691.
Conflict of interest
The authors declare that the research was conducted in the absence of any commercial or financial relationships that could be construed as a potential conflict of interest.
Generative AI statement
The author(s) declare that no Generative AI was used in the creation of this manuscript.
Publisher’s note
All claims expressed in this article are solely those of the authors and do not necessarily represent those of their affiliated organizations, or those of the publisher, the editors and the reviewers. Any product that may be evaluated in this article, or claim that may be made by its manufacturer, is not guaranteed or endorsed by the publisher.
References
Acharya R., Chattopadhyay S. (2019). OMNI (Ocean Moored buoy network for northern Indian Ocean) Buoy System - a critical component of ocean observational programme of ESSO (earth System Science organization), Ministry of Earth Sciences, Government of India. J. Indian Geophysical Union 23, 101–105.
Adler R., Wang J.-J., Sapiano M., Huffman G., Chiu L., Xie P. P., et al. (2016). Global precipitation climatology project (GPCP) climate data record (CDR), version 2.3 (Monthly) (Washington, D.C. USA: National Centers for Environmental Information). doi: 10.7289/V56971M6
Alberty M., Sprintall J., MacKinnon J., Germineaud C., Cravatte S., Ganachaud A. (2019). Moored observations of transport in the Solomon Sea. J. Geophys. Res. 124, 8166–8192. doi: 10.1029/2019JC015143
Alongi D. M. (2014). Carbon cycling and storage in mangrove forests. Ann. Rev. Mar. Sci. 6, 195–219. doi: 10.1146/annurev-marine-010213-135020
Annamalai H., Xie S. P., McCreary J. P., Murtugudde R. (2005). Impact of Indian Ocean sea surface temperature on developing El Niño. J. Clim 18, 302–319. doi: 10.1175/JCLI-3268.1
Anutaliya A., Send U., Sprintall J., McClean J. L., Lankhorst M., Koelling J. (2019). Mooring and seafloor pressure end point measurements at the southern entrance of the Solomon Sea: Subseasonal to interannual flow variability. J. Geophys. Res. 124, 5085–5104. doi: 10.1029/2019JC015157
Ardhuin F., Aksenov Y., Benetazzo A., Bertino L., Brandt P., Caubet E., et al. (2018). Measuring currents, ice drift, and waves from space: the sea surface kinematics multiscale monitoring (SKIM) concept. Ocean Sci. 14, 337–354. doi: 10.5194/os-14-337-2018
Ayoub N. K., Chidichimo M. P., Dever E., Guo X., Kim S. Y., Krug M., et al. (2024). Observing ocean boundary currents: Lessons learned from six regions with mature observational and modeling systems. Oceanography. 37, 82–91. doi: 10.5670/oceanog.2024.504
Ba J., Keenlyside N. S., Latif M., Park W., Ding H., Lohmann K., et al. (2014). A multi-model comparison of Atlantic multidecadal variability. Clim. Dyn. 43, 2333–2348. doi: 10.1007/s00382-014-2056-1
Balaguru K., Foltz G. R., Leung L. R., Kaplan J., Xu W., Reul N., et al. (2020). Pronounced impact of salinity on rapidly intensifying tropical cyclones. Bull. Am. Meteorol. Soc. 101, E1497–E1511. doi: 10.1175/BAMS-D-19-0303.1
Bastin S., Koldunov A., Schütte F., Gutjahr O., Mrozowska M. A., Fischer T., et al. (2024). Sensitivity of the tropical Atlantic to vertical mixing in two ocean models (ICON-O v2.6.6 and FESOM v2.5). EGUsphere [preprint]. doi: 10.5194/egusphere-2024-2281
Bates N. R., Knap A. H., Michaels A. F. (1998). Contribution of hurricanes to local and global estimates of air–sea exchange of CO2. Nature 395, 58–61. doi: 10.1038/25703
Batten S. D., Abu-Alhaija R., Chiba S., Edwards M., Graham G., Jyothibabu R., et al. (2019). A global plankton diversity monitoring program. Front. Mar. Sci. 6. doi: 10.3389/fmars.2019.00321
Beal L. M., Vialard J., Roxy M. K., Li J., Andres M., Annamalai H., et al. (2020). A roadmap to IndOOs-2: Better observations of the rapidly warming Indian Ocean. Bull. Am. Meteorol. Soc 101, E1891–E1913. doi: 10.1175/BAMS-D-19-0209.1
Behera S. K., Yamagata T. (2001). Subtropical SST dipole events in the southern Indian Ocean. Geophys. Res. Lett. 28, 327–330. doi: 10.1029/2000GL011451
Bhatia K. T., Vecchi G. A., Knutson T. R., Murakami H., Kossin J., Dixon K. W., et al. (2019). Recent increases in tropical cyclone intensification rates. Nat. Commun. 10, 635. doi: 10.1038/s41467-019-08471-z
Biastoch A., Ruhs S., Ivanciu I., Schwarzkopf F. U., Veitch J., Reason C., et al. (2024). “The Agulhas Current System as an important driver for oceanic and terrestrial climate,” in Sustainability of southern african ecosystems under global change. Ecological studies, vol. 248 . Ed. von Maltitz G. P., et al (Springer, Cham). doi: 10.1007/978-3-031-10948-5_8
Bjerknes J. (1969). Atmospheric teleconnections from the equatorial Pacific. Mon. Weath. Rev. 97, 163–172. doi: 10.1175/1520-0493(1969)097<0163:ATFTEP>2.3.CO;2
Bourassa M. A., Meissner T., Cerovecki I., Chang P. S., Dong X., De Chiara G., et al. (2019). Remotely sensed winds and wind stresses for marine forecasting and ocean modeling. Front. Mar. Sci. 6. doi: 10.3389/fmars.2019.00443
Bourlès B., Araujo M., McPhaden M. J., Brandt P., Foltz G. R., Lumpkin R., et al. (2019). PIRATA: A sustained observing system for tropical Atlantic climate research and forecasting. Earth Space Sci. 6, 577–616. doi: 10.1029/2018EA000428
Bousquet O., Dalleau M., Bocquet M., Gaspar P., Bielli S., Ciccione S., et al. (2020). Sea Turtles for Ocean Research and Monitoring: Overview and initial results of the STORM project in the Southwest Indian Ocean. Front. Mar. Sci. 7. doi: 10.3389/fmars.2020.594080
Boyer T., Zhang H.-M., O’Brien K., Reagan J., Diggs S., Freeman E., et al. (2023). Effects of the pandemic on observing the global ocean. Bull. Am. Meteorol. Soc. 104, E389–E410. doi: 10.1175/bams-d-21-0210.1
Boyer T. P., Baranova O. K., Coleman C., Garcia H. E., Grodsky A., Locarnini R. A., et al. (2018). World ocean database 2018. Ed. Mishonov A. V. (Washington, D.C. USA: NOAA Atlas NESDIS), 87.
Brandt P., Bange H. W., Banyte D., Dengler M., Didwischus S. H., Fischer T., et al. (2015). On the role of circulation and mixing in the ventilation of oxygen minimum zones with a focus on the eastern tropical North Atlantic. Biogeosciences 12, 489–512. doi: 10.5194/bg-12-489-2015
Brandt P., Körner M., Moum J. N., Roch M., Subramaniam A., Czeschel R., et al. (2025). Seasonal productivity of the equatorial Atlantic shaped by distinct wind-driven processes. Nat. Geosci. 18, 84–90. doi: 10.1038/s41561-024-01609-9
Brizuela N. G., Alford M. H., Xie S.-P., Sprintall J., Voet G., Warner S. J., et al. (2023). Prolonged thermocline warming by near-inertial internal waves in the wakes of tropical cyclones. Proc. Natl. Acad. Sci. 120, e2301664120. doi: 10.1073/pnas.2301664120
Browning T. J., Moore C. M. (2023). Global analysis of ocean phytoplankton nutrient limitation reveals high prevalence of co-limitation. Nat. Commun. 14, 5014. doi: 10.1038/s41467-023-40774-0
Buman B., Hueni A., Colombo R., Cogliati S., Celesti M., Julitta T., et al. (2022). Towards consistent assessments of in situ radiometric measurements for the validation of fluorescence satellite missions. Remote Sens. Environ. 274, 112984. doi: 10.1016/j.rse.2022.112984
Cai W., Santoso A., Collins M., Dewitte B., Karamperidou C., Kug J.-S., et al. (2021). Changing El Nino Southern Oscillation in a changing climate. Nat. Rev. Earth Environ. 2, 628–644. doi: 10.1038/s43017-021-00199-z
Cai W., Wu L., Lengaigne M., Li T., McGregor S., Kug J.-S., et al. (2019). Pantropical climate interactions. Science 363, eaav4236. doi: 10.1126/science.aav4236
Capotondi A., McGregor S., McPhaden M. J., Cravatte S., Holbrook N. J., Imada Y., et al. (2023b). Mechanisms of tropical Pacific decadal variability. Nat. Rev. Earth Environ. 4, 754–769. doi: 10.1038/s43017-023-00486-x
Capotondi A., Newman M., Xu T., Di Lorenzo E. (2022). An optimal precursor of Northeast Pacific marine heatwaves and Central Pacific El Nino events. Geophys. Res. Lett. 49, e2021GL097350. doi: 10.1029/2021GL097350
Capotondi A., Qiu B. (2023a). Decadal variability of the Pacific shallow overturning circulation and the role of local wind forcing. J. Climate 36, 1001–1015. doi: 10.1175/JCLI-D-22-0408.1
Capotondi A., Rodrigues R. R., Sen Gupta A., Benthuysen J. A., Deser C., Frölicher T. L., et al. (2024). A global overview of marine heatwaves in a changing climate. Commun. Earth Env. 5, 701. doi: 10.1038/s43247-024-01806-9
Carranza M. M., Long M. C., Di Luca A., Fassbender A. J., Johnson K. S., Takeshita Y., et al. (2024). Extratropical storms induce carbon outgassing over the Southern Ocean. Climate Atmospheric Sci. 7, 106. doi: 10.1038/s41612-024-00657-7
Chamberlain P., Talley L. D., Cornuelle B., Mazloff M., Gille S. T. (2023). Optimizing the biogeochemical Argo float distribution. J. Atmos. Oceanic Tech. 40, 1355–1379. doi: 10.1175/JTECH-D-22-0093.1
Chavez F. P., Strutton P. G., Friederich C. E., Feely R. A., Feldman G. C., Foley D. C., et al. (1999). Biological and chemical response of the equatorial Pacific Ocean to the 1997-98 El Niño. Science 286, 2126–2131. doi: 10.1126/science.286.5447.2126
Chelton D. B., Esbensen S. K., Schlax M. G., Thum N., Freilich M. H., Wentz F. J., et al. (2001). Observations of coupling between surface wind stress and sea surface temperature in the eastern tropical Pacific. J. Climate 14, 1479–1498. doi: 10.1175/1520-0442(2001)014<1479:OOCBSW>2.0.CO;2
Cheng L., von Schuckmann K., Abraham J. P., Trenberth K. E., Mann M. E., Zanna L., et al. (2022). Past and future ocean warming. Nat. Rev. Earth Environ. 3, 776–794. doi: 10.1038/s43017-022-00345-1
Chiang J. C. H., Vimont D. J. (2004). Analogous Pacific and Atlantic meridional modes of tropical atmosphere-ocean variability. J. Climate 17, 4143–4158. doi: 10.1175/JCLI4953.1
Choi H. Y., Park M. S., Kim H. S., Lee S. (2024). Marine heatwave events strengthen the intensity of tropical cyclones. Commun. Earth Environ. 5, 69. doi: 10.1038/s43247-024-01239-4
Claustre H., Johnson K. S., Takeshita Y. (2020). Observing the global ocean with biogeochemical-Argo. Annu. Rev. Mar. Sci. 12, 23–48. doi: 10.1146/annurev-marine-010419-010956
Clayson C. A., DeMott C. A., de Szoeke S. P., Chang P., Foltz G. R., Krishnamurthy R., et al. (2023a). A new paradigm for observing and modeling of air-sea interactions to advance earth system prediction. A US CLIVAR Report (Washington, D.C. USA: US CLIVAR Project Office), 86. doi: 10.5065/24j7-w583
Clayson C. A., Subramanian A. C., Brown S. T., Lee T., Boland J., Bourassa M. A., et al. (2023b). Butterfly: a satellite mission to transform our understanding of the contribution of air-sea fluxes to weather and climate, Abstract J10.2, presented at 2023 AMS Annual Meeting, 8-12 January. Boston, MA USA: American Meteorological Society (AMS).
Clement A., Bellomo K., Murphy L. N., Cane M. A., Mauritsen T., Rädel G., et al. (2015). The Atlantic multidecadal oscillation without a role for ocean circulation. Science 350, 320–324. doi: 10.1126/science.aab3980
Coles V. J., Brooks M. T., Hopkins J., Stukel M. R., Yager P. L., Hood R. R. (2013). The pathways and properties ofthe Amazon River Plume in the tropical North Atlantic Ocean. J. Geophys. Res. Oceans 118, 6894–6913. doi: 10.1002/2013JC008981
Collins M., Botzet M., Carril A. F., Drange H., Jouzeau A., Latif M., et al. (2006). Interannual to decadal climate predictability in the North Atlantic: a multimodel-ensemble study. J. Clim 19, 1195–1203. doi: 10.1175/JCLI3654;1
Compaire J. C., Perez-Brunius P., Jimenez-Rosenberg S. P. A., Outerelo J. R., Garcia L. P. E., Herzka S. Z. (2021). Connectivity of coastal and neritic fish larvae to the deep waters. Limnology Oceanography 66, 2423–2441. doi: 10.1002/lno.11762
Connell K. J., McPhaden M. J., Foltz G. R., Perez R. C., Grissom K. (2023). Surviving piracy and the Coronavirus pandemic. Oceanography 36, 44–45. doi: 10.5670/oceanog.2023.212
Cooley S. R., Kite-Powell H. L., Doney S. C. (2015). Ocean acidification’s potential to alter global marine ecosystem services. Oceanography 22, 172–181. doi: 10.5670/oceanog.2009.106
Cornec M., Claustre H., Mignot A., Guidi L., Lacour L., Poteau A., et al. (2021). Deep chlorophyll maxima in the global ocean: Occurrences, drivers and characteristics. Global Biogeochemical Cycles 35, e2020GB006759. doi: 10.1029/2020GB006759
Cravatte S., Kessler W. S., Smith N., Wijffels S. E. (2016). First report of TPOS 2020. Available online at: http://tpos2020.org/first-report/ (Accessed June 14, 2024).
Cromwell T. (1953). Circulation in a meridional plane in the central equatorial Pacific. J. Mar. Res. 12, 196–213.
Cronin M. F., Gentemann C. L., Edson J., Ueki I., Bourassa M., Brown S., et al. (2019). Air-sea fluxes with a focus on heat and momentum. Front. Marine. Sci. 6. doi: 10.3389/fmars.2019.00430
Cunningham S. A., Kanzow T., Rayner D., Baringer M. O., Johns W. E., Marotzke J., et al. (2007). Temporal variability of the Atlantic Meridional Overturning Circulation at 26.5°N. Science 317, 935–938. doi: 10.1126/science.1141304
Da N. D., Foltz G. R., Balaguru K. (2021). Observed global increases in tropical cyclone-induced ocean cooling and primary production. Geophys. Res. Lett. 48, e2021GL092574. doi: 10.1029/2021GL092574
Dai A., Fyfe J., Xie S. P., Dai X. (2015). Decadal modulation of global surface temperature by internal climate variability. Nat. Clim. Change 5, 555–559. doi: 10.1038/nclimate2605
Davis R. E., Talley L. D., Roemmich D., Owens W. B., Rudnick D. L., Toole J., et al. (2019). 100 years of progress in ocean observing systems. Meteorological Monogr. 59, 3.1–3.46. doi: 10.1175/AMSMONOGRAPHS-D-18-0014.1
Dee S. G., Cobb K. M., Emile-Geay J., Ault T. R., Edwards R. L., Cheng H., et al. (2020). No consistent ENSO response to volcanic forcing over the last millennium. Science 367, 1477–1481. doi: 10.1126/science.aax2000
Deng J., Dai A. (2024). Arctic sea ice-air interactions weaken El Nino - Southern Oscillation. Sci. Adv. 10. doi: 10.1126/sciadv.adk3990
Deppenmeier A. L., Bryan F. O., Kessler W. S., Thompson L. (2022). Diabatic upwelling in the tropical Pacific: Seasonal and subseasonal variability. J. Phys. Oceanogr. 52, 2657–2668. doi: 10.1175/JPO-D-21-0316.1
Deppenmeier A. L., Haarsma R. J., van Heerwaarden C., Hazeleger W. (2020). The southeastern tropical Atlantic SST bias investigated with a coupled atmosphere-ocean single-column model at a PIRATA mooring site. J. Climate 33, 6255–6271. doi: 10.1175/JCLI-D-19-0608.1
DeVries T., Primeau F., Deutsch C. (2012). The sequestration efficiency of the biological pump. Geophys. Res. Lett. 39, L13601. doi: 10.1029/2012GL051963
Dhame S., Tashetto A. S., Santoso A., Meissner K. J. (2020). Indian Ocean warming modulates global atmospheric circulation trends. Clim. Dyn. 55, 2053–2073. doi: 10.1007/s00382-020-05369-1
Ditkovsky S., Resplandy L., Busecke J. (2023). Unique ocean circulation pathways reshape the Indian Ocean oxygen minimum zone with warming. Biogeosciences 20, 4711–4736. doi: 10.5194/bg-20-4711-2023
Domingues R., Kuwano-Yoshida A., Chardon-Maldonado P., Todd R. E., Halliwell G., Kim H.-S., et al. (2019). Ocean observations in support of studies and forecasts of tropical and extratropical cyclones. Front. Mar. Sci. 6. doi: 10.3389/fmars.2019.00446
Dommenget D., Latif M. (2002). A cautionary note on the interpretation of EOFs. J. Climate 15, 216–225. doi: 10.1175/1520-0442(2002)015<0216:ACNOTI>2.0.CO;2
Donato D., Kauffman J., Murdiyarso D., Jurnianto S., Stidham M., Kanninen M. (2011). Mangroves among the most carbon-rich forests in the tropics. Nat. Geosci 4, 293–297. doi: 10.1038/ngeo1123
Dong L., McPhaden M. J. (2016). Interhemispheric SST gradient trends in the Indian Ocean prior to and during the recent global warming hiatus. J. Climate 29, 9077–9095. doi: 10.1175/JCLI-D-16-0130.1
Dong L., McPhaden M. J. (2017). Why has the relationship between Indian and Pacific Ocean decadal variability changed in recent decades? J. Climate 30, 1971–1983. doi: 10.1175/JCLI-D-16-0313.1
Dong L., Zhou T., Dai A., Song F., Wu B., Chen X. (2016). The footprint of the inter-decadal Pacific oscillation in Indian Ocean sea surface temperatures. Sci. Rep. 6, 21251. doi: 10.1038/srep21251
Dugenne M., Corrales-Ugalde M., Luo J. Y., Kiko R., O’Brien T. D., Irisson, et al. (2024). First release of the Pelagic Size Structure database: global datasets of marine size spectra obtained from plankton imaging devices. Earth Syst. Sci. Data 16, 2971–2999. doi: 10.5194/essd-16-2971-2024
Dutheil C., Lal S., Lengaigne M., Cravatte S., Menkes C., Receveur A., et al. (2024). The massive 2016 marine heatwave in the Southwest Pacific: An “El Niño–Madden-Julian Oscillation” compound event. Sci. Adv. 10, eadp2948. doi: 10.1126/sciadv.adp2948
Dzwonkowski B., Coogan J., Fournier S., Lockridge G., Park K., Lee T. (2020). Compounding impact of severe weather events fuels marine heatwave in the coastal ocean. Nat. Commun. 11, 4623. doi: 10.1038/s41467-020-18339-2
Eddebbar Y. A., Whitt D. B., Verdy A., Mazloff M. R., Subramanian A. C., Long M. C. (2024). Eddy-mediated turbulent mixing of oxygen in the equatorial Pacific. J. Geophys. Res. 129, e2023JC020588. doi: 10.1029/2023JC020588
Evans W., Strutton P. G., Chavez F. P. (2009). Impact of tropical instability waves on nutrient and chlorophyll distributions in the equatorial Pacific. Deep Sea Res. Part I: Oceanographic Res. Papers 56, 178–188. doi: 10.1016/j.dsr.2008.08.008
Exarchou E., Ortega P., Rodríguez-Fonseca B., Losada T., Polo I., Prodhomme C. (2021). Impact of equatorial Atlantic variability on ENSO predictive skill. Nat. Commun. 12, 1612. doi: 10.1038/s41467-021-21857-2
Farneti R., Stiz A., Ssebandeke J. B. (2022). Improvements and persistent biases in the southeast tropical Atlantic in CMIP models. NPJ Clim Atmos Sci. 5, 42. doi: 10.1038/s41612-022-00264-4
Feely R. A., Boutin J., Cosca C. E., Dandonneau Y., Etcheto J., Inoue H. Y., et al. (2002). Seasonal and interannual variability of CO2 in the equatorial Pacific. Deep Sea Res. Part II: Topical Stud. Oceanography 49, 2443–2469. doi: 10.1016/S0967-0645(02)00044-9
Feely R. A., Takahashi T., Wanninkhof R., McPhaden M. J., Cosca C. E., Sutherland S. C., et al. (2006). Decadal variability of the air-sea CO2 fluxes in the equatorial Pacific Ocean. J. Geophys Res. 111, C08S90. doi: 10.1029/2005JC003129
Feely R. A., Wanninkhof R., Cosca C. E., McPhaden M. J., Byrne R. H., Millero F. J., et al. (1994). The effect of tropical instability waves on CO2 species distributions along the equator in the eastern equatorial Pacific during the 1992 ENSO event. Geophys. Res. Lett. 21, 277–280. doi: 10.1029/93GL03212
Feng M., Hendon H. H., Xie S.-P., Marshall A. G., Schiller A., Kosaka Y., et al. (2015). Decadal increase in Ningaloo Niño since the late 1990s. Geophys. Res. Lett. 42, 104112. doi: 10.1002/2014GL062509
Fennel K., Gehlen M., Brasseur P., Brown C. W., Ciavatta S., Cossarini G., et al. (2019). Advancing marine biogeochemical and ecosystem reanalyses and forecasts as tools for monitoring and managing ecosystem health. Front. Mar. Sci. 6. doi: 10.3389/fmars.2019.00089
Fennel K., Testa J. M. (2019). Biogeochemical controls on coastal hypoxia. Ann. Rev. Mar. Sci. 11, 105–130. doi: 10.1146/annurev-marine-010318-095138
Fiedler P. C., Redfern J. V., Van Noord J., Hall C., Pitman R. L., Ballance L. T. (2013). Effects of a tropical cyclone on a pelagic ecosystem from the physical environment to top predators. Mar. Ecol. Prog. Ser. 484, 1–16. doi: 10.3354/meps10378
Foltz G. R., Brandt P., Richter I., Rodriguez-Fonseca B., Hernandez F., Dengler M., et al. (2019). The tropical atlantic observing system. Front. Mar. Sci. 6. doi: 10.3389/fmars.2019.00206
Forget G., Campin J.-M., Heimbach P., Hill C. N., Ponte R. M., Wunsch C. (2015). ECCO version 4: An integrated framework for non-linear inverse modeling and global ocean state estimation. Geoscientific Model. Dev. 8, 3071–3104. doi: 10.5194/gmd-8-3071-2015
Frajka-Williams E., Ansorge I. J., Baehr J., Bryden H. L., Chidichimo M. P., Cunningham S. A., et al. (2019). Atlantic Meridional Overturning Circulation: Observed transport and variability. Front. Mar. Sci. 6, 260. doi: 10.2289/fmars.2019.00260
Frauen C., Dommenget D. (2012). Influences of the tropical Indian and Atlantic Oceans on the predictability of ENSO. Geophys. Res. Lett. 39, L02706. doi: 10.1029/2011GL050520
Freeman E., Woodruff S. D., Worley S. J., Lubker S. J., Kent E. C., Angel W. E., et al. (2017). ICOADS Release 3.0: A major update to the historical marine climate record. Int. J. Climatol. 37, 2211–2237. doi: 10.1002/joc.4775
Frölicher T. L., Fischer E. M., Gruber N. (2018). Marine heatwaves under global warming. Nature 560, 360–364. doi: 10.1038/s41586-018-0383-9
Fujii Y., Cummings J., Xue Y., Schiller A., Lee T., Alonso M., et al. (2015). Evaluation of the Tropical Pacific Observing System from the ocean data assimilation perspective. Q. J. R. Meteorol. Soc 141, 2481–2496. doi: 10.1002/qj2579
Fujii Y., Kamachi M., Nakaegawa T., Yasuda T., Yamanaka G., Toyoda T., et al. (2011). “Assimilating ocean observation data for ENSO monitoring and forecasting,” in Climate variability - some aspects, challenges and prospects. Ed. Hannachi A. (InTech Open, Rijeka), 75–98. doi: 10.5772/30330
Garcia S. M., de Leiva Moreno I. (2003). Global overview of marine fisheries. In Responsible fisheries in the marine ecosystem. Eds. Sinclair M., Valdimarsson G. (Wallingford, UK: CABI Publishing).
Garcia H. E., Weathers K. W., Paver C. R., Smolyar I. V., Boyer T. P., Locarnini R. A., et al. (2019). World ocean atlas 2018, volume 3: dissolved oxygen, apparent oxygen utilization, and oxygen saturation. Ed. Mishonov A. (Washington, D.C. USA: NOAA Atlas NESDIS), 83.
Gasparin F., Guinehut S., Mao C., Mirouze I., Rémy E., King R. R., et al. (2019). Requirements for an integrated in situ Atlantic Ocean observing system from coordinated observing system simulation experiments. Front. Mar. Sci. 6. doi: 10.3389/fmars.2019.00083
Gelaro R., McCarty W., Suarez M. J., Todling R., Molod A., Takacs L., et al. (2017). The modern-era retrospective analysis for research and applications, version 2 (MERRA-2). J. Climate 30, 5419–5454. doi: 10.1175/JCLI-D-16-0758.1
Gevaudan M., Jouanno J., Durand F., Morvan G., Renault L., Samson G. (2021). Influence of ocean salinity stratification on the tropical Atlantic Ocean surface. Climate Dyn. 57, 321–340. doi: 10.1007/s00382-021-05713-z
Ghosh J., Chakraborty K., Bhattacharya T., Valsala V., Baduru B. (2022). Impact of coastal upwelling dynamics on the pCO2 variability in the southeastern Arabian Sea. Prog. Oceanogr. 203, 102785. doi: 10.1016/j.pocean.2022.102785
Gille S. T., Lee T., Boland J., Chang P., Farrar J. T., Jacobs G. A., et al. (2022). Simultaneous winds and surface currents from space: ODYSEA (Ocean Dynamics and Surface Exchange with the Atmosphere), OS12D-0775, presented at 2022 AGU Fall Meeting, 12-16 December. Washington, D.C. USA: American Geophysical Union (AGU).
Gomara I., Rodriguez-Fonseca B., Mohino E., Losada T., Polo I., Coll M. (2021). Skillful prediction of tropical Pacific fisheries provided by Atlantic Ninos. Environ. Res. Lett. 16, 054066. doi: 10.1088/1748-9326/abfa4d
Gray A. R. (2024). The four-dimensional carbon cycle of the Southern Ocean. Annu. Rev. Mar. Sci. 16, 163–190. doi: 10.1146/annurev-marine-041923-104057
Gregory C. H., Holbrook N. J., Marshall A. G., Spillman C. M. (2024). Sub-seasonal to seasonal drivers of regional marine heatwaves around Australia. Clim. Dyn. 62, 6599–6623. doi: 10.1007/s00382-024-07226-x
Gruber N., Boyd P. W., Frölicher T. L., Vogte M. (2021). Biogeochemical extremes and compound events in the ocean. Nature 600, 395–407. doi: 10.1038/s41586-021-03981-7
Hagos S., Foltz G. R., Zhang C., Thompson E., Seo H., Chen S., et al. (2020). Atmospheric convection and air-sea interactions over the tropical oceans: Scientific progress, challenges and opportunities. Bull. Am. Meteorol. Soc 101, E253–E258. doi: 10.1175/BAMS-D-19-0261.1
Ham Y. G., Kug J. S., Park J. Y., Jin F. F. (2013). Sea surface temperature in the north tropical Atlantic as a trigger for El Niño/Southern Oscillation events. Nat. Geosci 6, 112–116. doi: 10.1038/ngeo1686
Hans A. C., Brandt P., Gasparin F., Claus M., Cravatte S., Horstmann J., et al. (2024). Observed diurnal cycles of near-surface shear and stratification in the equatorial Atlantic and their wind dependence. J. Geophys. Res. 129, e2023JC020870. doi: 10.1029/2023JC020870
Henderiks J., Bartol M., Pige N., Karatsolis B.-T., Lougheed B. C. (2020). Shifts in phytoplankton composition and stepwise climate change during the middle Miocene. Paleoceanography Paleoclimatology 35, e2020PA003915. doi: 10.1029/2020PA003915
Hermes J. C., Masumoto Y., Beal L. M., Roxy M. K., Vialard J., Andres M., et al. (2019). A sustained ocean observing system in the Indian Ocean for climate related scientific knowledge and societal needs. Front. Mar. Sci. 6. doi: 10.3389/fmars.2019.00355
Hersbach H., Bell B., Berrisford P., Hirahara S., Horanyl A., Munoz-Sabater J., et al. (2020). The ERA5 global reanalysis. Q. J. R. Meteorol. Soc 146, 1999–2049. doi: 10.1002/qj.3803
Heuzé C., Purkey S. G., Johnson G. C. (2022). It is high time we monitor the deep ocean. Environ. Res. Lett. 17, 121002. doi: 10.1088/1748-9326/aca622
Hlywiak J., Nolan D. S. (2019). The influence of oceanic barrier layers on tropical cyclone intensity as determined through idealized, coupled numerical simulations. J. Phys. Oceanogr. 49, 1723–1745. doi: 10.1175/JPO-D-18-0267.1
Hobday A. J., Oliver E. C. J., Sen Gupta A., Benthuysen J. A., Burrows M. T., Donat M. G., et al. (2018). Categorizing and naming marine heatwaves. Oceanography 31, 162–173. doi: 10.5670/oceanog.2018.205
Holbrook N. J., Scannell H. A., Sen Gupta A., Benthuysen J. A., Feng M., Oliver E. C. J., et al. (2019). A global assessment of marine heatwaves and their drivers. Nat. Commun. 10, 2624. doi: 10.1038/s41467-019-10206-z
Holmes R. M., Renault L., Maillard L., Boucharel J. (2024). Air-sea coupling feedbacks over tropical instability waves. J. Phys. Oceanogr. 54, 2165–2184. doi: 10.1175/JPO-D-24-0010.1
Holmes R. M., Thomas L. N. (2015). The modulation of equatorial turbulence by tropical instability waves in a regional ocean model. J. Phys. Oceanogr. 45, 1155–1173. doi: 10.1175/JPO-D-14-0209.1
Hu D., Hu S., Wu L., Li L., Zhang L., Diao X., et al. (2013). Direct measurements of the luzon undercurrent. J. Phys. Oceanogr. 43, 1417–1425. doi: 10.1175/JPO-D-12-0165.1
Hu D., Wang F., Sprintall J., Wu L., Riser S., Cravatte S., et al. (2020). Review on observational studies of western tropical Pacific Ocean circulation and climate. J. Ocean. Limnol. 38, 906–929. doi: 10.1007/s00343-020-0240-1
Hu D., Wu L., Cai W., Sen Gupta A., Ganachaud A., Qiu B., et al. (2015). Pacific western boundary currents and their roles in climate. Nature 522, 299–308. doi: 10.1038/nature14504
Huang P., Lin I. I., Chou C., Huang R. H. (2015). Change in ocean subsurface environment to suppress tropical cyclone intensification under global warming. Nat. Commun. 6, 7188. doi: 10.1038/ncomms8188
Hummels R., Brandt P., Dengler M., Fischer J., Araujo M., Veleda D., et al. (2015). Interannual to decadal changes in the western boundary circulation in the Atlantic at 11 degrees S. Geophys. Res. Lett. 42, 7615–7622. doi: 10.1002/2015gl065254
Hummels R., Dengler M., Rath W., Foltz G. R., Schutte F., Fischer T., et al. (2020). Surface cooling caused by rare but intense near-inertial wave induced mixing in the tropical Atlantic. Nat. Commun. 11, 3829. doi: 10.1038/s41467-020-17601-x
Hutchings L., van der Lingen C. D., Shannon L. J., Crawford R. J. M., Verheye H. M. S., Bartholomae C. H., et al. (2009). The Benguela Current: An ecosystem of four components. Prog. Oceanogr. 83, 15–32. doi: 10.1016/j.pocean.2009.07.046
Iles C. E., Samset B. H., Sandstad M., Schuhen N., Wilcox L. J., Lund M. T. (2024). Strong regional trends in extreme weather over the next two decades under high- and low-emissions pathways. Nat. Geosci. 17, 845–850. doi: 10.1038/s41561-024-01511-4
Ishii M., Rodgers K. B., Inoue H. Y., Toyama K., Sasano D., Kosugi N., et al. (2020). Ocean acidification from below in the tropical pacific. Global Biogeochemical Cycles 34, e2019GB006368. doi: 10.1029/2019GB006368
Iwamoto M. M., Dorton J., Newton J., Yerta M., Gibeaut J., Shyka T., et al. (2019). Meeting Regional, coastal and ocean user needs with tailored data products: A stakeholder-driven process. Front. Mar. Sci. 6. doi: 10.3389/fmars.2019.00290
Izumo T., Vialard J., Lengaigne M., de Boyer Montegut C., Behera S. K., Luo J.-J., et al. (2010). Influence of the state of the Indian Ocean Dipole on the following years El Niño. Nat. Geosci 3, 168–172. doi: 10.1038/ngeo760
Jackson J. M., Rainville L., Roberts M. J., McQuaid C. D., Lutjeharms J. R. E. (2012). Mesoscale bio-physical interactions between the Agulhas Current and the Agulhas Bank, South Africa. Continental Shelf Res. 49, 10–24. doi: 10.1016/j.csr.2012.09.005
Jarugula S. L., McPhaden M. J. (2022). Ocean mixed layer response to two post-monsoon cyclones in the Bay of Bengal in 2018. J. Geophys. Res. 127, e2022JC018874. doi: 10.1029/2022JC018874
Jiang F., Seager R., Cane M. A. (2024). A climate change signal in the tropical Pacific emerges from decadal variability. Nat. Comm 15, 8291. doi: 10.1038/s41467-024-52731-6
Jing R., Heft-Neal S., Chavas D. R., Griswold M., Wang Z., Clark-Ginsberg A., et al. (2024). Global population profile of tropical cyclone exposure from 2002 to 2019. Nature 626, 549–554. doi: 10.1038/s41586-023-06963-z
John E. D., Balaguru K., Leung L. R., Foltz G. R., Hetland R., Hagos S. M. (2023). Intensification of hurricane sally, (2020) over the Mississippi River plume. Weather Forecasting 38, 1391–1404. doi: 10.1175/WAF-D-22-0191.1
Johns W., Speich S., Araujo M. (2021). Tropical atlantic observing system (TAOS) review report. (Qingdao, China: International CLIVAR Project Office). 218 pp.
Johnson K., Claustre H. (2016). Bringing biogeochemistry into the Argo age. Eos 97. doi: 10.1029/2016EO062427
Joshi A. P., Chowdhury R. R., Warrior H. V., Kumar V. (2021). Influence of the freshwater plume dynamics and the barrier layer thickness on the CO2 source and sink characteristics of the Bay of Bengal. Mar. Chem. 236, 104030. doi: 10.1016/j.marchem.2021.104030
Kamenkovich I., Haza A., Gray A. R., Dufour C. O., Garraffo Z. (2017). Observing System Simulation Experiments for an array of autonomous biogeochemical profiling floats in the Southern Ocean. J. Geophys. Res. Oceans 122, 7595–7611. doi: 10.1002/2017JC012819
Keenlyside N. S., Ding H., Latif M. (2013). Potential of equatorial Atlantic variability to enhance El Niño prediction. Geophys. Res. Lett. 40, 2278–2283. doi: 10.1002/grl.50362
Kennan S. C., Flament P. J. (2000). Observations of a tropical instability vortex. J. Phys. Oceanogr. 30, 2277–2301. doi: 10.1175/1520-0485(2000)030
Kessler W. S., Cravatte S., Strutton P. G., Sutton A. J., Kumar A., Takaya Y., et al. (2021). Final report of TPOS 2020. GOOS-268, 83 pp. Available online at: https://tropicalpacific.org/tpos2020-project-archive/reports/ (Accessed June 14, 2024).
Kessler W. S., Hristova H. G., Davis R. E. (2019). Equatorward western boundary transport from the South Pacific: Glider observations, dynamics and consequences. Prog. Oceanogr. 175, 208–225. doi: 10.1016/j.pocean.2019.04.005
Klein S. A., Soden B. J., Lau N. G. (1999). Remote sea surface temperature variation during ENSO: Evidence for a tropical atmosphere bridge. J. Climate 12, 917–932. doi: 10.1175/1520-0442(1999)012<0917:RSSTVD>2.0.CO;2
Knight J. R., Allan R. J., Folland C. K., Vellinga M., Mann M. E. (2005). A signature of persistent natural thermohaline circulation cycles in observed climate. Geophys. Res. Lett. 32, L20708. doi: 10.1029/2005GL024233
Koch-Larrouy A., Atmadipoera A., van Beek P., Madec G., Aucan J., Lyard F., et al. (2015). Estimates of tidal mixing in the Indonesian archipelago from multidisciplinary INDOMIX in-situ data. Deep Sea Res. I 106, 136–153. doi: 10.11016/j.dsr.2015.09.007
Körner M., Brandt P., Illig S., Dengler M., Subramaniam A., Bachèlery M.-L., et al. (2024). Coastal trapped waves and tidal mixing control primary production in the tropical Angolan upwelling system. Sci. Adv. 10, eadj6686. doi: 10.1126/sciadv.adj6686
Kucharski F., Bracco A., Yoo J. H., Molteni F. (2008). Atlantic forced component of the Indian monsoon interannual variability. Geophys Res. Lett. 35, L04706. doi: 10.1029/2007GL033037
Kumar B. P., Vialard J., Lengaigne M., Merty V. S. N., McPhaden M. J. (2012). TropFlux: Air-sea fluxes for the global tropical oceans – description and evaluation. Clim. Dyn. 38, 1521–1543. doi: 10.1007/s00382-011-1115-0
Le Boyer A., Couto N., Alford M. H., Drake H. F., Bluteau C. E., Hughes K., et al. (2023). Turbulent diapycnal fluxes as a pilot Essential Ocean Variable. Front. Mar. Sci. 10. doi: 10.3389/fmars.2023.1241023
Lefèvre N., Veleda D., Beaumont L. (2024). Trends and drivers of CO2 parameters, from 2006 to 2021, at a time-series station in the Eastern Tropical Atlantic (6° S, 10° W). Front. Mar. Sci. 11. doi: 10.3389/fmars.2024.1299071
Le Hénaff M., Domingues R., Halliwell G., Zhang J. A., Kim H.-S., Aristizabal M., et al. (2021). The role of the Gulf of Mexico ocean conditions in the intensification of Hurricane Michael, (2018). J. Geophys. Res. 126, e2020JC016969. doi: 10.1029/2020JC016969
Levin L. A., Bett B. J., Gates A. R., Heimbach P., Howe B. M., Janssen F., et al. (2019). Global observing needs in the deep ocean. Front. Mar. Sci. 6. doi: 10.3389/fmars.2019.00241
Li Y., Han W., Wang W., Ravichandran M., Lee T., Shinoda T. (2017). Bay of Bengal salinity stratification and Indian summer monsoon intraseasonal oscillation: 2. Impact on SST and convection. J. Geophys. Res. Oceans 122, 4312–4328. doi: 10.1002/2017JC012692
Li X., Hu Z.-Z., McPhaden M. J., Zhu C., Liu Y. (2023). Triple-dip La Niñas in 1998-2001 and 2020-2023: Impact of mean state changes. J. Geophys. Res. 128, e2023JD038843. doi: 10.1029/2023JD038843
Li C., Huang J., Liu X., Ding L., He Y., Xie Y. (2024). The ocean losing its breath under the heatwaves. Nat. Commun. 15, 6840. doi: 10.1038/s41467-024-51323-8
Lindstrom E., Gunn J., Fischer A., McCurdy A., Glover L. K. (2012). A framework for ocean observing. By the task team for an Integrated Framework for Sustained Ocean Observing (Paris, France: UNESCO).
Little C. M., Hu A., Hughes C. W., McCarthy G. D., Piecuch C. G., Ponte R. M., et al. (2019). The relationship between U.S. East Coast sea level and the Atlantic Meridional Overturning Circulation: A review. J. Geophys. Res. 124, 6435–6458. doi: 10.1029/2019JC015152
Liu Y., Donat M. G., Taschetto A. S., Doblas-Reyes F. J., Alexander L. V., England M. H. (2019). A framework to determine the limits of achievable skill for interannual to decadal climate predictions. J.Geophys. Res. 124, 2882–2896. doi: 10.1029/2018JD029541
Liu W., Fedorov A. V., Xie S.-P., Hu S. (2020). Climate impacts of a weakened Atlantic Meridional Overturning Circulation in a warming climate. Sci. Adv. 6. doi: 10.1126/sciadv.aaz4876
Liu C., Huo D., Liu Z., Wang X., Guan C., Qi J., et al. (2022). Turbulent mixing in the barrier layer of the equatorial Pacific Ocean. Geophys. Res. Lett. 49, e2021GL097690. doi: 10.1029/2021GL097690
Locarnini R. A., Mishonov A. V., Baranova O. K., Boyer T. P., Zweng M. M., Garcia H. E., et al. (2019). World ocean atlas 2018, volume 1: temperature. Ed. Mishonov A. (Washington, D.C. USA: NOAA Atlas NESDIS), 52 pp.
Lombard F., Boss E., Waite A. M., Vogt M., Uitz J., Stemmann L., et al. (2019). Globally consistent quantitative observations of planktonic ecosystems. Front. Mar. Sci. 6. doi: 10.3389/fmars.2019.00196
Lopez-Parages J., Auger P.-A., Rodriguez-Fonseca B., Keenlyside N., Gaetan C., Rubino A., et al. (2020). El Nino as a predictor of round sardinella distribution along the northwest African coast. Prog. Oceanogr. 186, 102341. doi: 10.1016/j.pocean.2020.102341
Losada T., Rodríguez-Fonseca B. (2016). Tropical atmospheric response to decadal changes in the Atlantic Equatorial Mode. Climate Dynamics 47, 1211–1224. doi: 10.1007/s00382-015-2897-2
Lübbecke J. F., Rodriguez-Fonseca B., Richter I., Martin-Rey M., Losada T., Polo I., et al. (2018). Equatorial Atlantic variability - modes, mechanisms, and global teleconnections. Wiley Interdiscip. Rev. - Climate Change 9, e527. doi: 10.1002/wcc.527
Lumpkin R., Garzoli S. (2011). Interannual to decadal changes in the western South Atlantic’s surface circulation. J. Geophys. Res. 116, C01014. doi: 10.1029/2010JC006285
Maillard L., Boucharel J., Renault L. (2022). Direct and rectified effects of tropical instability waves on the eastern tropical Pacific mean state in a regional ocean model. J. Phys. Oceanogr. 52, 1817–1834. doi: 10.1175/JPO-D-21-0300.1
Majkut J. D., Carter B. R., Froelicher T. L., Dufour C. O., Rodgers K. B., Sarmiento J. L. (2014). An observing system simulation for Southern Ocean carbon dioxide uptake. Philos. Trans. R. Soc. a-Mathematical Phys. Eng. Sci. 372, 20130046. doi: 10.1098/rsta.2013.0046
Mann M. E., Steinman B. A., Brouillette D. J., Miller S. K. (2021). Multidecadal climate oscillations during the past millennium driven by volcanic forcing. Science 371, 1014–1019. doi: 10.1126/science.abc581
Martín-Rey M., Rodríguez-Fonseca M. B., Polo I., Kucharski F. (2014). On the Atlantic–Pacific Niños connection: A multidecadal modulated mode. Climate Dynamics 43, 3163–3178. doi: 10.1007/s00382-014-2305-3
Masich J., Kessler W. S., Cronin M. F., Grissom K. R. (2021). Diurnal cycles of near-surface currents across the tropical Pacific. J. Geophys. Res. 126, e2020JC016982. doi: 10.1029/2020JC016982
Mawren D., Hermes J., Reason C. J. C. (2022). Marine heatwaves and tropical cyclones - two devastating types of coastal hazard in southeastern Africa. Estuarine Coast. Shelf Sci. 277, 108056. doi: 10.1016/j.ecss.2022.108056
McGregor S., Timmermann A., Stuecker M., England M. H., Merrifield M., Jin F.-F., et al. (2014). Recent Walker circulation strengthening and Pacific cooling amplified by Atlantic warming. Nat. Clim Change 4, 888–892. doi: 10.1038/nclimate2330
McMahon C. R., Roquet F. (2022). Animal-borne ocean sensors: A decadal vision through new eyes. Mar. Tech. Soc J. 3, 36–38. doi: 10.4031/MTSJ.56.3.2
McMahon C. R., Roquet F., Baudel S., Belbeoch M., Bestley S., Blight C., et al. (2021). Animal borne ocean sensors – AniBOS – an essential component of the Global Ocean Observing System. Front. Mar. Sci. 8. doi: 10.3389/fmars.2021.751840
McPhaden M. J., Busalacchi A. J., Cheney R., Donguy J.-R., Gage K. S., Halpern D., et al. (1998). The tropical ocean-global atmosphere observing system: a decade of progress. J. Geophys. Res. 103, 14169–14240. doi: 10.1029/97JC02906
McPhaden M. J., Connell K. J., Foltz G. R., Perez R. C., Grissom K. (2023). Tropical ocean observations for weather and climate: A decadal overview of the Global Tropical Moored Buoy Array. Oceanography 36, 33–43. doi: 10.5670/oceanog.2023.211
McPhaden M. J., Jarugula S., Aroucha L. C., Lübbecke J. F. (2024). Indian Ocean Dipole intensifies Benguela Niño through Congo River discharge. Commun. Earth Environ. 5, 779. doi: 10.1038/s43247-024-01955-x
McPhaden M. J., Meyers G., Ando K., Masumoto Y., Murty V. S. N., Ravichandran M., et al. (2009). RAMA: the research moored array for african-asian-Australian monsoon analysis and prediction. Bull. Am. Meteorological Soc. 90, 459–480. doi: 10.1175/2008BAMS2608.1
McPhaden M. J., Zebiak S. E., Glantz M. H. (2006). ENSO as an integrating concept in earth science. Science 314, 1740–1745. doi: 10.1126/science.1132588
McWhorter J. K., Roman-Stork H. L., LeHénaff M., Frenzel H., Johnston M. A., Cornec M., et al. (2024). Mesoscale eddies influence coral reef environments in the northwest Gulf of Mexico. J. Geophys. Res. 129, e2023JC020821. doi: 10.1029/2023JC020821
Meehl G. A., Goddard L., Boer G., Burgman R., Branstator G., Cassou C., et al. (2014). Decadal climate prediction: An update from the trenches. Bull. Am. Meteorol. Soc 95, 243–267. doi: 10.1175/BAMS-D-12-00241.1
Meehl G. A., Hu A., Arblaster J., Fasullo J. T., Trenberth K. E. (2013). Externally forced and internally generated decadal climate variability in the Pacific. J. Clim 26, 7298–7310. doi: 10.1175/JCLI-D-12-00548.1
Meinen C. S., Baringer M. O., Garcia R. F. (2010). Florida Current transport variability: An analysis of annual and longer-period signals. Deep Sea Res. I 57, 835–846. doi: 10.1016/j.dsr.2010.04.001
Menkes C. E., Kennan S. C., Flament P., Dandonneau Y., Masson S., Biessy B., et al. (2002). A whirling ecosystem in the equatorial Atlantic. Geophys. Res. Lett. 29, 48–1-48-4. doi: 10.1029/2001GL014576
Miloslavich P., Bax N. J., Simmons S. E., Klein E., Appeltans W., Aburto-Oropeza O., et al. (2018). Essential ocean variables for global sustained observations of biodiversity and ecosystem changes. Glob. Change Biol. 24, 2416–2433. doi: 10.1111/gcb.14108
Modi A., Roxy M. K. (2023). “Past trends and future projections of marine primary productivity in the tropical Indian ocean,” in Dynamics of planktonic primary productivity in the Indian ocean (Springer International Publishing, Cham), 191–206. doi: 10.1007/978-3-031-34467-1_9
Montecino V., Lange C. B. (2009). The Humbuldt Current System: Ecosystem components and processes, fisheries, and sediment studies. Prog. Oceanogr. 83, 65–79. doi: 10.1016/j.pocean.2009.07.041
Morris T., Hermes J., Beal L., du Plessis M., Duncombe Rae C., Gulekana M., et al. (2017). The importance of monitoring the Greater Agulhas Current and its inter-ocean exchanges using large mooring arrays. South Afr. J. Sci. 113, 2016–0330. doi: 10.17159/sajs.2017/20160330
Moum J. N., Hughes K. G., Shroyer E. L., Smyth W. D., Cherian D., Warner S. J., et al. (2022). Deep cycle turbulence in Atlantic and Pacific cold tongues. Geophys. Res. Lett. 49, e2021GL097345. doi: 10.1029/2021GL097345
Moum J. N., Smyth W. D., Hughes K. G., Cherian D., Warner S. J., Bourlès B., et al. (2023). Wind dependencies of deep cycle turbulence in the equatorial cold tongues. J. Phys. Oceanogr. 53, 1979–1995. doi: 10.1175/JPO-D-22-0203.1
Mrozowska M. A., Jochum M., Bastin S., Hummels R., Koldunov A., Dengler M., et al. (2024). Using NIW observations to assess mixed layer parameterizations: A case study in the tropical Atlantic. J. Geophys. Res. 129, e2024JC020985. doi: 10.1029/2024JC020985
Müller J. D., Gruber N., Carter B., Feely R., Ishii M., Lange N., et al. (2023). Decadal trends in the oceanic storage of anthropogenic carbon from 1994 to 2014. AGU Adv. 4, e2023AV000875. doi: 10.1029/2023AV000875
Mumby P. J., Vitolo R., Stephenson D. B. (2011). Temporal clustering of tropical cyclones and its ecosystem impacts. Proc. Nat. Acad. Sci. 108, 17626–17630. doi: 10.1073/pnas.110043610
Murtugudde R., Beauchamp J., McClain C. R., Lewis M., Busalacchi A. J. (2002). Effects of penetrative radiation on the upper tropical ocean circulation. J. Climate 15, 470–486. doi: 10.1175/1520-0442(2002)015<0470:EOPROT>2.0.CO;2
Nakano H., Ishii M., Rodgers K. B., Tsujino H., Yamanaka G. (2015). Anthropogenic CO2 uptake, transport, storage, and dynamical controls in the ocean imposed by the meridional overturning circulation: A modeling study. Global Biogeochemical Cycles 29, 1706–1724. doi: 10.1002/2015GB005128
NASEM (2018). Thriving on our changing planet: A decadal strategy for earth observation from space (Washington, DC: The National Academies Press).
Nicholson S. A., Whitt D. B., Fer I., du Plessis M. D., Lebéhot A. D., Swart S., et al. (2022). Storms drive outgassing of CO2 in the subpolar Southern Ocean. Nat. Commun. 13, 158. doi: 10.1038/s41467-021-27780-w
Nobre P., Shukla J. (1996). Variations of sea surface temperature, wind stress, and rainfall over the tropical Atlantic and South America. J. Climate 9, 2464–2479. doi: 10.1175/1520-0442(1996)009<2464;VOSSTW>2.0.CO;2
Oke P. R., Schiller A. (2007). Impact of Argo, SST, and altimeter data on an eddy-resolving ocean reanalysis. Geophys. Res. Lett. 34, L19601. doi: 10.1029/2007GL031549
Oschlies A., Brandt P., Stramma L., Schmidtko S. (2018). Drivers and mechanisms of ocean deoxygenation. Nat. Geosci. 11, 467–473. doi: 10.1038/s41561-018-0152-2
Park J. Y., Kug J. S., Park J., Yeh S. W., Jang C. J. (2011). Variability of chlorophyll associated with El Niño-Southern Oscillation and its possible biological feedback in the equatorial Pacific. J. Geophys. Res. 116, C10001. doi: 10.1029/2011JC007056
Pearce A. F., Feng M. (2013). The rise and fall of the “marine heat wave” off Western Australia during the summer of 2010/11. J. Mar. Syst. 111-112, 139–156. doi: 10.1016/j.jmarsys.2012.10.009
Penny S. G., Akella S., Balmaseda M. A., Browne P., Carton J. A., Chevallier M., et al. (2019). Observational needs for improving ocean and coupled reanalysis, S2S prediction, and decadal prediction. Front. Mar. Sci. 6. doi: 10.3389/fmars.2019.00391
Pinheiro H. T., MacDonald C., Santos R. G., Ali R., Bobat A., Cresswell B. J., et al. (2023). Plastic pollution on the world’s coral reefs. Nature 619, 311–316. doi: 10.1038/s41586-023-06113-5
Pittman N. A., Strutton P. G., Johnson R., Matear R. J., Sutton A. J. (2022). Relationships between air-sea CO2 flux and new production in the Equatorial Pacific. Global Biogeochemical Cycles 36, e2021GB007121. doi: 10.1029/2021GB007121
Poupon M. A., Resplandy L., Lévy M., Bopp L. (2023). Pacific decadal oscillation influences tropical oxygen minimum zone extent and obscures anthropogenic changes. Geophys. Res. Lett. 50, e2022GL102123. doi: 10.1029/2022GL102123
Power S., Lengaigne M., Capotondi A., Khodri M., Vialard J., Jebri B., et al. (2021). Decadal climate variability in the tropical Pacific: Characteristics, causes, predictability, and prospects. Science 374. doi: 10.1126/science.aay9165
Pruitt J. N., Little A. G., Majumdar S. J., Schoener T. W., Fisher D. N. (2019). Call-to-action: A global consortium for tropical cyclone ecology. Trends Ecol. Evol. 34, 588–590. doi: 10.1016/j.tree.2019.04.009
Rayner N. A., Parker D. E., Horton E. B., Folland C. K., Alexander L. V., Rowell D. P., et al. (2003). Global analyses of sea surface temperature, sea ice, and night marine air temperature since the late nineteenth century. J. Geophys. Res. 108, 4407. doi: 10.1029/2002JD002670
Resplandy L., Lévy M., Bopp L., Echevin V., Pous S. V. V. S. S., Sarma V. V. S. S., et al. (2012). Controlling factors of the oxygen balance in the Arabian Sea’s OMZ. Biogeosciences 9, 5095–5109. doi: 10.5194/bg-9-5095-2012
Roarty H., Cook T., Hazard L., George D., Harlan J., Cosoli S., et al. (2019). The global high frequency radar network. Front. Mar. Sci. 6. doi: 10.3389/fmars.2019.00164
Rodrigues R. R., Tashetto A. S., Sen Gupta A., Foltz G. R. (2019). Common cause for severe droughts in South America and marine heatwaves in the South Atlantic. Nat. Geosci. 12, 620–626. doi: 10.1038/s41561-019-0393-8
Rodriguez-Fonseca B., Polo I., Garcia-Serrano J., Losada T., Mohino E., Mechoso C. R., et al. (2009). Are Atlantic Ninos enhancing Pacific ENSO events in recent decades? Geophys. Res. Lett. 36, L20705. doi: 10.1029/2009GL040048
Roemmich D., Alford M. H., Claustre H., Johnson K., King B., Moum J., et al. (2019). On the future of Argo: A global, full-depth, multi-disciplinary array. Front. Mar. Sci. 6. doi: 10.3389/fmars.2019.00439
Rong X. Y., Zhang R. H., Li T. (2010). Impacts of Atlantic sea surface temperature anomalies on Indo-East Asian summer monsoon-ENSO relationship. Chin. Sci. Bull. 55, 2458–2468. doi: 10.1007/s11434-010-3098-3
Roobaert A., Laruelle G. G., Landschützer P., Gruber N., Chou L., Regnier P. (2019). The spatiotemporal dynamics of the sources and sinks of CO2 in the global coastal ocean. Global Biogeochem. Cycles 33, 1693–1714. doi: 10.1029/2019GB006239
Roxy M. K., Saranya J. S., Modi A., Anusree A., Cai W., Resplandy L., et al. (2024). “Future projections for the tropical Indian Ocean,” in The Indian ocean and its role in the global climate system (Amsterdam, Netherlands: Elsevier), 469–482. doi: 10.1016/B978-0-12-822698-8.00004-4
Ryan J. P., Ueki I., Chao Y., Zhang H. C., Polito P. S., Chavez F. P. (2006). Western Pacific modulation of large phytoplankton blooms in the central and eastern equatorial Pacific. J. Geophys. Res.-Biogeosci 111, G02013. doi: 10.1029/2005jg000084
Sá C., D’Alimonte D., Brito A. C., Kajiyama T., Mendes C. R., Vitorino J., et al. (2015). Validation of standard and alternative satellite ocean-color chlorophyll products off Western Iberia. Remote Sens. Environ. 168, 403–419. doi: 10.1016/j.rse.2015.07.018
Sabine C., Sutton A., McCabe K., Lawrence-Slavas N., Alin S., Feely R., et al. (2020). Evaluation of a new carbon dioxide system for autonomous surface vehicles. J. Atmos. Oceanic Tech. 37, 1305–1317. doi: 10.1175/JTECH-D-20-0010.1
Saji N. H., Goswami B. N., Vinayachandran P. N., Yamagata T. (1999). A dipole mode in the tropical Indian Ocean. Nature 401, 360–363. doi: 10.1038/43855
Samelson R. M. (2019). Challenges and opportunities in coastal prediction. Eos 100. doi: 10.1029/2019EO113841
Saravanan R., Chang P. (2000). Interaction between tropical Atlantic variability and El Nino - Southern Oscillation. J. Clim. 13, 2177–2194. doi: 10.1175/1520-0442(2000)013<2177:IBTAVA>2.0.CO;2
Scannell H. A., Pershing A. J., Alexander M. A., Thomas A. C., Mills K. E. (2016). Frequency of marine heatwaves in the North Atlantic and North Pacific since 1950. Geophys. Res. Lett. 43, 2069–2076. doi: 10.1002/2015GL067308
Schlunegger S., Rodgers K. B., Sarmiento J. L., Ilyina T., Dunne J. P., Takano Y., et al. (2020). Time of emergence and large ensemble intercomparison for ocean biogeochemical trends. Global Biogeochemical Cycles 34, e2019GB006453. doi: 10.1029/2019GB006453
Schmidt J. O., Bograd S. J., Arrizabalaga H., Azevedo J. L., Barbeaux S. J., Barth J. A., et al. (2019). Future ocean observations to connect climate, fisheries and marine ecosystems. Front. Mar. Sci. 6. doi: 10.3389/fmars.2019.00550
Schubert R., Gula J., Biastoch A. (2021). Submesoscale flows impact Agulhas leakage in ocean simulations. Commun. Earth Environ. 2, 197. doi: 10.1038/s43247-021-00271-y
Schwarzkopf F. U., Böning C. W. (2011). Contribution of Pacific wind stress to multi-decadal variations in upper-ocean heat content and sea level in the tropical south Indian Ocean. Geophys. Res. Lett. 38, 1–6. doi: 10.1029/2011GL04765
Scoccimarro E., Gualdi S., Bellucci A., Sanna A., Fogli P. G., Manzini E., et al. (2011). Effects of tropical cyclones on ocean heat transport in a high-resolution coupled general circulation model. J. Climate 24, 4368–4384. doi: 10.1175/2011JCLI4104.1
Seager R., Henderson N., Cane M. (2022). Persistent discrepancies between observed and modeled trends in the tropical Pacific Ocean. J. Climate 35, 4571–4584. doi: 10.1175/JCLI-D-21-0648.1
Séférian R., Berthet S., Yool A., Palmiéri J., Bopp L., Tagliabue A., et al. (2020). Tracking improvement in simulated marine biogeochemistry between CMIP5 and CMIP6. Curr. Climate Change Rep. 6, 95–119. doi: 10.1007/s40641-020-00160-0
Send U., Lankhorst M., Kanzow T. (2011). Observation of decadal change in the Atlantic Meridional Overturning Circulation using 10 years of continuous transport data. Geophys. Res. Lett. 38, L24606. doi: 10.1029/2011GL049801
Seo H., O’Neill L. W., Bourassa M. A., Czaja A., Drushka K., Edson J. B., et al. (2023). Ocean mesoscale and frontal-scale ocean-atmosphere interactions and influence on large-scale climate: A review. J. Climate 36, 1981–2013. doi: 10.1175/JCLI-D-21-0982.1
Shay L. K., Goni G. J., Black P. G. (2000). Effects of a warm oceanic feature on Hurricane Opal. Mon. Weath. Rev. 128, 1366–1383. doi: 10.1175/1520-0493(2000)128<1366:EOAWOF>2.0.CO;2
Shi H., García-Reyes M., Jacox M. G., Rykaczewski R. R., Black B. A., Bograd S. J., et al. (2021). Co-occurrence of California drought and northeast Pacific marine heatwaves under climate change. Geophys. Res. Lett. 48, e2021GL092765. doi: 10.1029/2021GL092765
Shi G., Ribbe J., Cai W., Cowan T. (2007). Multidecadal variability inthe transmission of ENSO signals to the Indian Ocean. Geophys. Res.Lett. 34, L09706. doi: 10.1029/2007GL029528
Siiriä S., Roiha P., Tuomi L., Purokoski T., Haavisto N., Alenius P. (2018). Applying area-locked, shallow water Argo floats in Baltic Sea monitoring. J. Operational Oceanography 12, 58–72. doi: 10.1080/1755876X.2018.1544783
Silva B. J., Ibanhez J. S. P., Pinheiro B. R., Ladle R. J., Malhado A. C., Pinto T. K., et al. (2022). Seasonal influence of surface and underground continental runoff over a reef system in a tropical marine protected area. J. Mar. Syst. 226, 103660. doi: 10.1016/j.jmarsys.2021.103660
Sinyanya K. Y., Marshall T. A., Flynn R. F., Harris E., Mdutyana M., Toman R., et al. (2024). Wintertime productivity and carbon export potential across the Agulhas Current system. Deep Sea Res. Part I 213, 104405. doi: 10.1016/j.dsr.2024.104405
Skyllingstad E. D., de Szoeke S. P., O’Neill L. W. (2019). Modeling the transient response of tropical convection to mesoscale SST variations. J. Atmos. Sci. 76, 1227–1244. doi: 10.1175/JAS-D-18-0079.1
Smith S. R., Bourassa M. A., Long M. (2011). Pirate attacks affect Indian ocean climate research. Eos Trans. Am. Geophys. Union 92, 27. doi: 10.1029/2011EO270001
Smith K. E., Burrows M. T., Hobday A. J., King N. G., Moore P. J., Sen Gupta A., et al. (2023). Biological impacts of marine heatwaves. Annu. Rev. Mar. Sci. 15, 119–145. doi: 10.1146/annurev-marine-032122-121437
Smith N., Kessler W. S., Cravatte S., Sprintall J., Wijffels S., Cronin M. F., et al. (2019). Tropical pacific observing system. Front. Mar. Sci. 6. doi: 10.3389/fmars.2019.00031
Smyth W. D., Moum J. N., Li L., Thorpe S. A. (2013). Diurnal shear instability, the descent of the surface shear layer, and the deep cycle of equatorial turbulence. J. Phys. Oceanogr. 43, 2432–2455. doi: 10.1175/JPO-D-13-089.1
Sprintall J., Gordon A., Koch-Larrouy A., Lee T., Potemra J. T., Pujiana K., et al. (2014). The Indonesian seas and their role in the coupled ocean–climate system. Nat. Geosci 7, 487–492. doi: 10.1038/ngeo2188
Sprintall J., Gordon A. L., Wijffels S. E., Feng M., Hu S., Koch-Larrouy A., et al. (2019). Detecting change in the Indonesian seas. Front. Mar. Sci. 6. doi: 10.3389/fmars.2019.00257
Sprintall J., Nagura M., Hermes J., Roxy M. K., McPhaden M. J., Pattabhi E., et al. (2024). COVID impacts cause critical gaps in the Indian Ocean observing system. Bull. Am. Meteorol. Soc 105, E725–E741. doi: 10.1175/BAMS-D-22-0270.1
Stramma L., Johnson G. C., Sprintall J., Mohrholz V. (2008). Expanding oxygen-minimum zones in the tropical oceans. Science 320, 655–658. doi: 10.1126/science.1153847
Strutton P. G., Ryan J. P., Chavez F. P. (2001). Enhanced chlorophyll associated with tropical instability waves in the equatorial Pacific. Geophys. Res. Lett. 28, 2005–2008. doi: 10.1029/2000GL012166
Stuecker M. F. (2018). Revisiting the pacific meridional mode. Sci. Rep. 8, 3216. doi: 10.1038/s41598-018-21537-0
Subramaniam A., Yater P. L., Carpenter E. J., Capone D. G. (2008). Amazon River enhances diazotrophy and carbon sequestration in the tropical North Atlantic Ocean. Proc. Nat. Acad. Sci. 105, 10460–10465. doi: 10.1073/pnas.0710279105
Subramanian A. C., Balmaseda M. A., Centurioni L., Chattopadhyay R., Cornuelle B. D., DeMott C., et al. (2019). Ocean observations to improve our understanding, modeling, and forecasting of subseasonal-to-seasonal variability. Front. Mar. Sci. 6. doi: 10.3389/fmars.2019.00427
Sutton A. J., Sabine C. L., Maenner-Jones S., Lawrence-Slavas N., Meinig C., Feely R. A., et al. (2014). A high-frequency atmospheric and seawater pCO2 data set from 14 open-ocean sites using a moored autonomous system. Earth Syst. Sci. Data 6, 353–366. doi: 10.5194/essd-6-353-2014
Takahashi T., Feely R. A., Weiss R. F., Wanninkhof R. H., Chipman D. W., Sutherland S. C. (1997). Global air-sea flux of CO2: An estimate based on measurements of sea-air pCO2 difference. Proc. Natl. Acad. Sci. 94, 8292–8299. doi: 10.1073/pnas.94.16.8292
Takahashi T., Sutherland S. C., Wanninkhof R., Sweeney C., Feely R. A., Chipman D. W., et al. (2009). Climatological mean and decadal change in surface ocean pCO2, and net sea–air CO2 flux over the global oceans. Deep Sea Res. Part II: Topical Stud. Oceanography 56, 554–577. doi: 10.1016/j.dsr2.2008.12.009
Tanhua T., Pouliquen S., Hausman J., O’Brien K., Bricher P., de Bruin T., et al. (2019). Ocean FAIR data services. Front. Mar. Sci. 6. doi: 10.3389/fmars.2019.00440
Teixeira J., Piepmeier J. R., Nehrir A. R., Ao C. O., Chen S. S., Clayson C. A., et al. (2021). Toward a global planetary boundary layer observing system: The NASA PBL Incubation Study Team report (Pasadena, CA USA: NASA PBL Incubation Study Team). Available at: https://science.nasa.gov/earth-science/decadal-surveys/decadal-pbl/ (Accessed June 1, 2024).
Terray P., Masson S., Prodhomme C., Roxy M. K., Sooraj K. P. (2016). Impacts of Indian and Atlantic oceans on ENSO in a comprehensive modeling framework. Climate Dynamics 46, 2507–2533. doi: 10.1007/s00382-015-2715-x
Thompson P. A., Paerl H. W., Campbell L., Yin K., McDonald K. S. (2023). Tropical cyclones: what are their impacts on phytoplankton ecology? J. Plankton Res. 45, 180–204. doi: 10.1093/plankt/fbac062
Tivig M., Keller D. P., Oschlies A. (2024). Riverine nutrient impact on global ocean nitrogen cycle feedbacks and marine primary production in an Earth System Model. Biogeosciences 21, 4469–4493. doi: 10.5194/bg-21-4469-2024
Todd R. E., Asher T. G., Heiderich J., Bane J. M., Luettich R. A. (2018). Transient response of the Gulf Stream to multiple hurricanes in 2017. Geophys. Res. Lett. 45, 509–510. doi: 10.1029/2018GL079180
Todd R. E., Chavez F. P., Clayton S., Cravatte S., Goes M., Graco M., et al. (2019). Global perspectives on observing ocean boundary current systems. Front. Mar. Sci. 6. doi: 10.3389/fmars.2019.00423
Torres H., Wineteer A., Klein P., Lee T., Wang J., Rodriguez E., et al. (2023). Anticipated capabilities of the ODYSEA wind and current mission concept to estimate wind work at the air–sea interface. Remote Sens. 15, 3337. doi: 10.3390/rs15133337
Trenary L. L., Han W. (2008). Causes of decadal subsurface cooling in the tropical Indian Ocean during 1961-2000. Geophys. Res. Lett. 35, 13–17. doi: 10.1029/2008GL034687
Trenary L. L., Han W. (2013). Local and remote forcing of decadal sea level and thermocline depth variability in the South Indian Ocean. J. Geophys. Res. Oceans 118, 381–398. doi: 10.1029/2012JC00831
Ummenhofer C. C., Biastoch A., Böning C. W. (2017). Multidecadal Indian Ocean variability linked to the Pacific and implications for preconditioning Indian Ocean dipole events. J. Climate 30, 1739–1751. doi: 10.1175/JCLI-D-16-0200.1
Ummenhofer C. C., Ryan S., England M. H., Scheinert M., Wagner P., Biastoch A., et al. (2020). Late 20th century Indian Ocean heat content gain masked by wind forcing. Geophys. Res. Lett. 47, e2020GL088692. doi: 10.1029/2020GL088692
Valsala V., Sreeush M. G., Anju M., Sreenivas P., Tiwari Y. K., Chakraborty K., et al. (2021). An observing system simulation experiment for Indian Ocean surface pCO2 measurements. Prog. Oceanogr. 194, 102570. doi: 10.1016/j.pocean.2021.102570
Van Vranken C., Vastenhoud B. M. J., Manning J. P., Plet-Hansen K. S., Jakoboski J., Gorringe P., et al. (2020). Fishing gear as a data collection platform: Opportunities to fill spatial and temporal gaps in operational sub-surface observation networks. Front. Mar. Sci. 7. doi: 10.3389/fmars.2020.485512
Vecchi G. A., Harrison M. J. (2007). An observing system simulation experiment for the Indian Ocean. J. Climate 20, 3300–3319. doi: 10.1175/JCLI14147.1
Verdy A., Mazloff M. R. (2017). A data assimilating model for estimating Southern Ocean biogeochemistry. J. Geophys. Res. 122, 6968–6988. doi: 10.1002/2016JC012650
Vialard J., Delecluse P. (1998). An OGCM study for the TOGA decade. Part I: Role of salinity in the physics of the western Pacific fresh pool. J.Phys. Oceanogr. 28, 1071–1088. doi: 10.1175/1520-0485(1998)028<1071:aosftt>2.0.co;2
Villas Bôas A. B., Ardhuin F., Ayet A., Bourassa M. A., Brandt P., Chapron B., et al. (2019). Integrated observations of global surface winds, currents, and waves: Requirements and challenges for the next decade. Front. Mar. Sci. 6. doi: 10.3389/fmars.2019.00425
Wang C. (2019). Three-ocean interactions and climate variability: a review and perspective. Clim. Dyn. 53, 5119–5136. doi: 10.1007/s00382-019-04930-x
Wang R., He J., Luo J.-J., Chen L. (2024). Atlantic warming enhances the influence of Atlantic Niño on ENSO. Geophys. Res. Lett. 51, e2023GL108013. doi: 10.1029/2023GL108013
Wang F., Hu S., Hu D., Yuan D. (2018). Recent progress in the western Pacific Ocean Circulation and Climate Study from IOCAS and Contribution to NPOCE/CLIVAR. CLIVAR Exchanges 75, 12–18. doi: 10.36071/clivar.75.2018
Wang J., Ma Q., Wang F., Zhang D. (2021). Linking seasonal-to-interannual variability of intermediate currents in the southwest tropical Pacific to wind forcing and ENSO. Geophys. Res. Lett. 48, e2021GL092440. doi: 10.1029/2021GL092440
Wang F., Wang J., Guan C., Ma Q., Zhang D. (2016). Mooring observations of equatorial currents in the upper 1000 m of the western Pacific Ocean during 2014. J. Geophys. Res. 121, 3730–3740. doi: 10.1002/2015JC011510
Wang F., Wang J., Xu L., Zhang X., Yan S., Chen Y. (2020). The development of a new real-time subsurface mooring. J. Oceanology Limnology 38, 1080–1091. doi: 10.1007/s00343-020-0144-0
Wanninkhof R., Pickers P. A., Omar A. M., Sutton A., Murata A., Olsen A., et al. (2019). A Surface Ocean CO2 Reference Network, SOCONET and associated marine boundary layer CO2 measurements. Front. Mar. Sci. 6. doi: 10.3389/fmars.2019.00400
Watanabe M., Jin F. (2002). Role of Indian Ocean warming in the development of Philippine Sea anticyclone during ENSO. Geophys Res. Lett. 29, 116-1–116-4. doi: 10.1029/2001GL014318
Webster P. J., Moore A. M., Loschnigg J. P., Leben R. R. (1999). Coupled ocean-atmosphere dynamics in the Indian Ocean during 1997-98. Nature 401, 356–360. doi: 10.1038/43848
Whalen C. B., de Lavergne C., Naveira Garabato A. C., Klymak J. M., Mackinnon J. A., Sheen K. L. (2020). Internal wave-driven mixing: governing processes and consequences for climate. Nat. Rev. Earth Environ. 1, 606–621. doi: 10.1038/s43017-020-0097-z
Whitt D. B., Cherian D. A., Holmes R. M., Bachman S. D., Lien R. C., Large W. G., et al. (2022). Simulation and scaling of the turbulent vertical heat transport and deep-cycle turbulence across the equatorial Pacific cold tongue. J. Phys. Oceanogr. 52, 981–1014. doi: 10.1175/JPO-D-21-0153.1
Wyatt A. S. J., Leichter J. J., Washburn L., Kui L., Edmunds P. J., Burgess S. C., et al. (2023). Hidden heatwaves and severe coral bleaching linked to mesoscale eddies and thermocline dynamics. Nat. Commun. 14, 25. doi: 10.1038/s41467-022-35550-5
Wyrtki K. (1962). The oxygen minima in relation to ocean circulation. Deep Sea Res. Oceanographic Abstracts 9, 11–23. doi: 10.1016/0011-7471(62)90243-7
Wyrtki K., Kilonsky B. (1984). Mean water and current structure during the Hawaii-to-Tahiti shuttle experiment. J. Phys. Oceanogr. 14, 242–254. doi: 10.1175/1520-0485(1984)014<0242:MWACSD>2.0.CO;2
Xue Y., Wen C., Yang X., Behringer D., Kumar A., Vecchi G., et al. (2017). Evaluation of tropical Pacific observing system using NCEP and GFDL ocean data assimilation systems. Clim. Dyn. 49, 843–868. doi: 10.1007/s00382-015-2743-6
Yin J. (2023). Rapid decadal acceleration of sea level rise along the U.S. East and Gulf Coasts during 2010–22 and its impact on hurricane-induced storm surge. J. Climate 36, 4511–4528. doi: 10.1175/JCLI-D-22-0670.1
Yu L. (2019). Global air–sea fluxes of heat, fresh water, and momentum: energy budget closure and unanswered questions. Annu. Rev. Mar. Sci. 11, 227–248. doi: 10.1146/annurev-marine-010816-060704
Yu L., Jin X. (2018). A regime-dependent retrieval algorithm for near-surface air temperature and specific humidity from multi-microwave sensors. Remote Sens. Environ. 215, 199–216. doi: 10.1016/j.rse.2018.06.001
Yu L., Weller R. A. (2007). Objectively analyzed air-sea heat fluxes for the global ice-free oceans, (1981-2005). Bull. Am. Meteorol. Soc 88, 527–540. doi: 10.1175/BAMS-88-4-527
Zawislak J., Rogers R. F., Aberson S. D., Alaka G. J., Alvey G. R., Aksoy A., et al. (2022). Accomplishments of NOAA’s airborne hurricane field program and a broader future approach to forecast improvement. Bull. Am. Meteorol. Soc 103, E311–E338. doi: 10.1175/BAMS-D-20-0174.1
Zhang D., Cronin M. F., Meinig C., Farrar J. T., Jenkins R., Peacock D., et al. (2019). Comparing air-sea flux measurements from a new unmanned surface vehicle and proven platforms during the SPURS-2 field campaign. Oceanography 32, 122–133. doi: 10.5670/oceanog.2019.220
Zhang Y., Du Y., Feng M., Hobday A. J. (2023d). Vertical structures of marine heatwaves. Nat. Commun. 14, 6483. doi: 10.1038/s41467-023-42219-0
Zhang C., Foltz G. R., Chiodi A., Mordy C., Edwards C., Meinig C., et al. (2023a). Hurricane observations by uncrewed systems. Bull. Am. Meteorol. Soc 104, E1893–E1917. doi: 10.1175/BAMS-D-21-0327.1
Zhang L., Han W. (2021). Indian ocean dipole leads to atlantic niño. Nat. Commun. 12, 5952. doi: 10.1038/s41467-021-26223-w
Zhang L., Han W., Sienzn F. (2018). Unraveling causes for the changing behavior of the tropical Indian Ocean in the past few decades. J. Climate 31, 2377–2388. doi: 10.1175/JCLI-D-17-0445.1
Zhang Q., Liu B., Li S., Zhou T. (2023c). Understanding models’ global sea surface temperature bias in mean state: From CMIP5 to CMIP6. Geophys. Res. Lett. 50, e2022GL100888. doi: 10.1029/2022GL100888
Zhang H., Tian D., Sun Y., Yang M., Yang S., Zhou Y., et al. (2024). Unmanned vehicles probed inner-core air-sea conditions during Super Typhoon Koinu, (2023). Sci. Bull. 69, 3789–3792. doi: 10.1016/j.scib.2024.10.018
Zhang H., Wang J., Wang F., Zhang Z., Ma Q. (2023b). Observed upper deep branch of the pacific meridional overturning circulation north of new Guinea. J. Phys. Oceanogr. 53, 1375–1386. doi: 10.1175/JPO-D-22-0180.1
Zhang Y., Zhang Z., Chen D., Qiu B., Wang W. (2020). Strengthening of the Kuroshio current by intensifying tropical cyclones. Science 368, 6494. doi: 10.1126/science.aax5758
Zhao Y., Capotondi A. (2024). The role of the tropical Atlantic in tropical Pacific climate variability. NPJ Clim Atmos Sci. 7, 140. doi: 10.1038/s41612-024-00677-3
Zhao Y., Di Lorenzo E., Newman M., Capotondi A., Stevenson S. (2023a). A Pacific tropical decadal variability challenge for climate models. Geophys. Res. Lett. 50, e2023GL104037. doi: 10.1029/2023GL104037
Zhao Y., Jin Y., Capotondi A., Li J., Sun D. (2023b). The role of tropical Atlantic in ENSO predictability barrier. Geophys. Res. Lett. 50, e2022GL101853. doi: 10.1029/2022GL101853
Zhao S., Jin F.-F., Stuecker M. F., Thompson P. R., Kug J.-S., McPhaden M. J., et al. (2024). Explainable El Nino predictability from climate mode interactions. Nature 630, 891–898. doi: 10.1038/s41586-024-07534-6
Zhao M., Knutson T. (2024). Crucial role of sea surface temperature warming patterns in near-term high-impact weather and climate projection. NPJ Clim Atmos Sci. 7, 130. doi: 10.1038/s41612-024-00681-7
Zhu Y., Zhang R.-H. (2019). A modified vertical mixing parameterization for its improved ocean and coupled simulations in the tropical Pacific. J. Phys. Oceanogr. 49, 21–37. doi: 10.1175/JPO-D-18-0100.1
Zohdi E., Abbaspour M. (2019). Harmful algal blooms (red tide): a review of causes, impacts and approaches to monitoring and prediction. Int. J. Environ. Sci. Technol. 16, 1789–1806. doi: 10.1007/s13762-018-2108-x
Zuo H., Balmaseda M. A., Tietsche S., Mogensen K., Mayer M. (2019). The ECMWF operational ensemble reanalysis-analysis system for ocean and sea-ice: a description of the system and assessment. Ocean Sci. 15, 779–808. doi: 10.5194/os-15-779-2019
Keywords: ocean observations, carbon cycle, climate, extreme weather, marine heatwaves, boundary currents, biogeochemistry, air-sea interaction
Citation: Foltz GR, Eddebbar YA, Sprintall J, Capotondi A, Cravatte S, Brandt P, Sutton AJ, Morris T, Hermes J, McMahon CR, McPhaden MJ, Looney LB, Tuchen FP, Roxy MK, Wang F, Chai F, Rodrigues RR, Rodriguez-Fonseca B, Subramanian AC, Dengler M, Stienbarger C, Bailey K and Yu W (2025) Toward an integrated pantropical ocean observing system. Front. Mar. Sci. 12:1539183. doi: 10.3389/fmars.2025.1539183
Received: 03 December 2024; Accepted: 17 January 2025;
Published: 07 February 2025.
Edited by:
Philipp Friedrich Fischer, Alfred Wegener Institute Helmholtz Centre for Polar and Marine Research (AWI), GermanyReviewed by:
Shuhei Masuda, Japan Agency for Marine-Earth Science and Technology (JAMSTEC), JapanHan Zhang, Ministry of Natural Resources, China
Copyright © 2025 Foltz, Eddebbar, Sprintall, Capotondi, Cravatte, Brandt, Sutton, Morris, Hermes, McMahon, McPhaden, Looney, Tuchen, Roxy, Wang, Chai, Rodrigues, Rodriguez-Fonseca, Subramanian, Dengler, Stienbarger, Bailey and Yu. This is an open-access article distributed under the terms of the Creative Commons Attribution License (CC BY). The use, distribution or reproduction in other forums is permitted, provided the original author(s) and the copyright owner(s) are credited and that the original publication in this journal is cited, in accordance with accepted academic practice. No use, distribution or reproduction is permitted which does not comply with these terms.
*Correspondence: Gregory R. Foltz, Z3JlZ29yeS5mb2x0ekBub2FhLmdvdg==