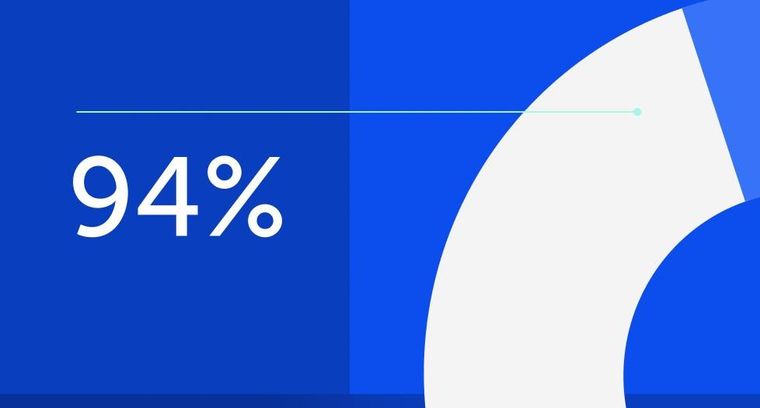
94% of researchers rate our articles as excellent or good
Learn more about the work of our research integrity team to safeguard the quality of each article we publish.
Find out more
ORIGINAL RESEARCH article
Front. Mar. Sci., 07 April 2025
Sec. Marine Biogeochemistry
Volume 12 - 2025 | https://doi.org/10.3389/fmars.2025.1534727
This article is part of the Research TopicDeep-sea Material and Energy Cycles: Insights from Sediments, Fe-Mn Nodules, and Authigenic Carbonates, and Their Carbon Sequestration, Hydrocarbon Accumulation, and Ore-forming SignificancesView all 5 articles
Fossil fish teeth are important carriers of rare earth elements (REEs) in deep-sea sediments. Meanwhile, 87Sr/86Sr-143Nd/144Nd in these fossils have been widely used in paleoceanography. However, when and how REEs enter the fish teeth remains ambiguous, which hinders elucidating the enrichment mechanism of REEs in deep-sea sediments, and the effectiveness of 87Sr/86Sr-143Nd/144Nd in paleoceanography is doubted. This study examined the contents of REEs, major and trace elements, and 87Sr/86Sr-143Nd/144Nd in modern fish teeth and in fossil fish teeth from deep-sea sediments. The results indicated that elemental geochemical properties and a redox environment are the main factors controlling their enrichment process in fish teeth. At least three categories of trace elements are classified during two different stages (physiological process of living fish and post-deposition of fossils): (1) elements of Type I mainly belong to IA, IIA, IIIA, IVA, and transition group with active chemical properties, entering into the fish teeth during life; (2) Type II represents elements from the IA, VA, transition elements, and Actinides groups, and are enriched uninterruptedly from the living fish teeth to the post-deposition fossils with variable valences under different redox conditions; (3) Type III are elements accumulated only after deposition under oxidizing or suboxidizing and are members of Lanthanides and rare dispersed elements groups with a large atomic radius and strong chemical activity. The distinctly different enrichment processes of Sr and Nd, 87Sr/86Sr, and 143Nd/144Nd in fossil fish teeth may represent different material sources and should be cautiously employed in paleoceanography. The continuous rehabilitation of 87Sr/86Sr in fossil fish teeth after deposition would change the original 87Sr/86Sr of seawater recorded near fish teeth but the content of Sr remains unchanged. As for 143Nd/144Nd, when Nd enters the fossils after deposition, the exchange of 143Nd/144Nd between particles and pore liquid gradually ceases due to the saturation of the isomorphism lattice. 143Nd/144Nd of fossil fish teeth may provide more information about deep seawater at the seawater-sediment interface. This study elucidates the enrichment mechanism of REEs in deep-sea sediments and the cautious utilization of 87Sr/86Sr and 143Nd/144Nd of fossils is a prerequisite.
As a group of elements with symbiotic properties, rare earth elements (REEs) have been widely used as tracers in different earth systems (Elderfield et al., 1990; Sholkovitz, 1993; Sun et al., 2023; Vanderstraeten et al., 2023; Weng et al., 2023) and have important significance in mineral resources (Nassar et al., 2015). Since the first report of heavily REE-enriched deep-sea sediment in the Pacific (Piper, 1974), deep-sea sediment containing higher than 400 ppm of REEs has been indicated as potential rare earth mineral resources nearly 50 years later (Kato et al., 2011). Subsequent studies have shown that REE-rich deep-sea sediments mainly develop in the Pacific Ocean and Indian Ocean (Kato et al., 2011; Kon et al., 2014; Yasukawa et al., 2014; Tanaka et al., 2020; Ren et al., 2021, 2022; Liao et al., 2022). The biological apatite (mainly referring to fossil fish teeth/bones) in sediments has been proven to be the main host of REEs with the highest content reaching to 22,000 ppm (Ren et al., 2021). Fossil fish teeth/bones with less than 10% content in sediments can contribute at least 69% of REEs in sediments (Lu et al., 2022; Liao et al., 2022), 1–2 orders of magnitude more than other hosts such as Fe-Mn oxyhydroxides, clay, and phillipsite (Wang et al., 2016; Bi et al., 2021; Liao et al., 2022). Therefore, fossil fish teeth/bones have attracted much attention in studies on the REE mineralization process in the deep sea.
Previous studies have shown that the enrichment of REE by biological apatite in sediments mainly occurs near the seawater/sediment interface in the early diagenetic process (Elderfield and Pagett, 1986; Grandjean et al., 1987; Bi et al., 2021; Ren et al., 2021), and is influenced by bottom water such as South Pacific Gyre and Antarctic bottom water (Ohta et al., 2021; Zhang et al., 2023). Nevertheless, it was found that the diffusion coefficient of REEs in fish bones from sediments in the Pacific is 70 mm2/my (Toyoda and Tokonami, 1990). With such a slow diffusion rate, the hypothesis is that REE directly entered the interior of the fish teeth from seawater during early diagenesis and high concentrations were doubted. Some studies argue that the enrichment of REEs occurs in a relatively longer period after deposition and absorption from pore water during diagenesis may occur (Liao et al., 2019a; Deng et al., 2022). One explanation is that the enrichment of REEs in deep-sea sediments is due to the significant decrease in the amount of REEs imported into seawater through pore water compared with shallow sea sediments, resulting in a large amount of REEs remaining in sediments and eventually being adsorbed by biological apatite (Deng et al., 2022). This process may also be related to the release of some of the initially adsorbed REEs from Ferrum-Manganese (Fe-Mn) minerals into pore water during oxidizing diagenesis (Liao et al., 2019b). In addition, whether clay minerals act as an intermediate in the enrichment process of REEs in biological apatite needs further investigation (Liu et al., 2023).
Being the main host of REEs, fossil fish teeth, which preserve not only neodymium isotopes (143Nd/144Nd) but also strontium isotopes (87Sr/86Sr) information, have been widely used as reliable indices in paleoceanography, geological events, and isotope stratigraphy (Wright et al., 1987; Ingram, 1995; Martin and Scher, 2004). However, it was indicated that the 87Sr/86Sr ratios of fossil fish teeth could change because of teeth recrystallization and exchange with pore fluid Sr2+ during burial and diagenesis, leading to older or younger Sr isotopic stratigraphic age (Martin and Scher, 2004). It has also been pointed out that the fidelity of 87Sr/86Sr in fish teeth is related to the density of the structure, and fish teeth with dense structure and low Lanthanum (La) content have a relative 87Sr/86Sr ratio, indicating that the Sr isotope is exchanged with the pore water when the elements such as La enter the fish tooth in the early diagenesis. However, some fish teeth are resistant to early diagenetic changes due to their dense structure (Wang et al., 2023). Conversely, the Nd absorption capacity of modern fish teeth is extremely low in ppb level, but the content of Nd in fossil fish teeth is three to six orders of magnitude higher than that in living fish teeth (Wright et al., 1984). The increase in Nd after fish teeth deposition coincides with the rapid transition from hydroxyapatite in live fish teeth to hydroxyfluorapatite in fossil fish teeth and the latter facilitate Nd enrichment, which is believed to be the main enrichment mechanism of Nd in fossil fish teeth (Martin and Haley, 2000). In the past, the rapid adsorption of Nd by fossil fish teeth was believed occur when teeth were still in contact with the bottom water, and the adsorption ceased in the burial stage (Martin and Scher, 2004). However, recent research indicated that the 143Nd/144Nd in fossil fish teeth is unevenly distributed and varies widely, increasing gradually from the root and middle to the tip of fish teeth (Deng et al., 2022). It was inferred that the Nd isotopic ratio of biological apatite in deep-sea sediments may be changed by the pore water during diagenesis. The reliability of REEs as tracers in the study of deep-sea sediment material sources needs to be further confirmed, and their usage in paleoceanography needs to be reevaluated as well.
To conclude, the enrichment mechanism of REEs in fossil fish teeth in deep-sea sediments has not been clearly elucidated, which results in doubts about the reliability of 87Sr/86Sr-143Nd/144Nd of fossil fish teeth as indices in paleoceanography and impacts the expounding of the material sources of highly enriched REEs in deep-sea sediments. Systematic comparative studies on the geochemical characteristics of these elements and isotopes in modern and fossil fish teeth may be conducive to understanding the enrichment process and influencing factors of these elements and isotopes in fish teeth. In this study, we detect the major and trace elements, REEs, and Sr-Nd isotopes in fossil fish teeth from the Central Indian Ocean Basin (CIOB) and modern fish teeth from the East China Sea (ECS) and the Qiandaohu Lake in eastern China, trying to answer the following three research questions: (1) with the comparison of REEs and other elements contained in living and fossil fish teeth, when and how the different elements enter the fish teeth and/or fossils will be discussed; (2) according to their enrichment process in modern and fossil fish teeth, different categories of elements will be classified according to their geochemical properties; (3) the 87Sr/86Sr-143Nd/144Nd record in fossil fish teeth from the CIOB as a material source tracer will be re-examined to analyze the impacting factors on its reliability when used to in paleoceanography. This study will explore the enrichment mechanism of REEs in deep-sea sediments and the utility of 87Sr/86Sr-143Nd/144Nd of fossils in paleoceanography.
The locations of the different sampling sites for fossil and modern fish teeth are shown in Figure 1.
Figure 1. Sampling location map (the red stars represent the origin of the fossil fish teeth while the red circles represent the origin of the modern fish teeth). The base map was downloaded from GEBCO_2023 http://www.gebco.net and modified from Mukhopadhyay (1998).
The ECS is one of the largest marginal seas in the Northwest Pacific Ocean. The submarine landform is divided from west to east into the East China Sea shelf, East China Sea slope, Okinawa trough, Kyushu-Ryukyu Island slope, and Ryukyu Island arc (Liu et al., 2003, 2020). The hydrodynamic of the ECS is complex with Changjiang (Yangtze River) diluted water, East China Sea coastal current, Kuroshio, Taiwan Warm Current, and Tsushima warm current (Su, 1998; Zhou et al., 2015). The interaction of these currents has a dominant influence on the regional marine environment. Seasonal changes in different water masses and the mixing of riverine runoff and coastal currents with the intrusion of saline currents significantly affect the redox conditions of the ECS (Sun et al., 2017, 2019). Generally, the strong oxidizing seawater distributes discontinuously in the ECS, in contrast to the weak oxidizing condition of the aquatic environment in spring. Two anoxic zones located at the north and the south Yangtze River estuary are developing, particularly in the summer, and can even merge into one large anoxic zone, which endangers both fish and benthos (Wei et al., 2015).
Qiandaohu Lake is located in eastern China and is fed from the East China Sea, in the low reach of the Changjiang River Basin. There are nearly 30 tributaries entering the lake, among them, Xin’an River is the largest water inflow, accounting for approximately 60% of the total runoff (Li et al., 2011). Geologically, the Xin’an River Basin is located on the eastern margin of the Yangtze Plate, the upper reaches of the lake basin are the Neoproterozoic Jiangnan paleo island arc, and the lower reaches are the Early Paleozoic Huaiyushan-Tianmushan passive marginal basin (Pan et al., 2009). The carbonate rocks and clastic rocks of the early Paleozoic are exposed, and intrusive rocks and volcanic rocks of the Yanshan and Jinning periods have been developed (Tang et al., 2023).
A series of NE-SW trending to NS-trending fault deformation belts divides the CIOB into the eastern and the western parts. The eastern CIOB is older (60-90 Ma), characterized by compressional structures, and presents a north-south trending fault zone, while the western is younger (30 Ma), including a NE-SW trending fault zone (Mukhopadhyay, 1998). Many of the isolated seamounts and seamount chains distributed parallel to the north-south trend in the basin are the products of a collision between the Indian plate and the Eurasian plate during the period of 65-49 Ma (Raju and Ramprasad, 1989; Dyment, 1993; Mukhopadhyay et al., 1997; Rajendran and Rao, 2000; Das et al., 2007). The Wharton Basin is located to the east of the CIOB, separated by the Ninety-East Ridge (NER). Based on the Fe-Mn crusts, the sources of detrital material change along the NER and reflect, from north to south, the decreasing influence of the Ganga River system and volcanic arcs located to the east, with the increasing influence of sediment derived from Australia to the south (Hein et al., 2016). The inhomogeneous growth of seamounts in the basin and the large amounts of volcanic-hydrothermal materials in volcanic rocks and sedimentary layers indicate the existence of volcanic hydrothermal activities in the plate (Iyer, 1991 and 2005; Mukhopadhyay, 1998; Iyer et al., 2007). Low-oxygen North Atlantic deep water (NADW) at 2,000–3,800 m and oxygen-rich Antarctic bottom water (AABW) below 3,800m converge to form circumpolar deep water. The redox condition at the sediment-water interface in the Indian Ocean is mainly regulated by export productivity and ventilation of abyssal water mass (Nambiar et al., 2022; Piotrowski et al., 2009).
The modern fish teeth samples from the ECS include a Miichthys miiuy, a Cynoglossus semilaevis, a Scomber japonicus, and three Larimichthys crocea (numbered D1, D2, D3, and D4–D6, respectively). Among them, Miichthys miiuy (D1), Scomber japonicus (D3), and Larimichthys crocea (D4–D6) are normal offshore bottom fishes. They live in a muddy and sandy environment and will migrate from deep water to the shore for long-distance reproductive migration during the reproductive season every year. By contrast, Cynoglossus semilaevis (D2) are usually inert with slow action and only move in a small range. In addition, two Mylopharyngodon piceus (numbered QDH1 and QDH2) were collected from Qiandaohu Lake. They are bottom-living fish in fresh water, with reproductive migration generally from the lower reaches of rivers and their tributaries or affiliated lakes up to the middle and upper reaches.
Two sediment cores (GC04 and GC11) from the CIOB and one sediment core (GC03) from the Wharton Basin were collected using a gravity piston corer by the China Ocean Mineral Resources R & D Association (COMRA) during the 30th and 34th Dayang Cruises in 2014 and 2015.
GC04 was collected at 80.10° E, 17.54° S at a water depth of 4,826 m, 350 cm long, homogeneous, and was light reddish brown. In total, 14 subsamples were collected at intervals of 20–30 cm. GC11 was collected at 80.49° E, 19.54° S with a water depth of 5,106 m, 200 cm long, and was homogeneously reddish brown. In total, 21 subsamples were collected at 10 cm intervals. GC03 was collected at 92.41° E, 13.35° S with a water depth of 5072 m, 200 cm long, and was a heterogeneous texture with the upper part a yellowish-brown and the lower part a reddish-brown. In total, nine subsamples were collected at 10 cm intervals in the top 90 cm. The samples were cryopreserved at -20°C after collection and transported back to the laboratory for subsequent tests. In this study, 15 samples of debris of deposited fish teeth in three cores were identified and sorted under a binocular microscope.
An OLYMPUS CX43 microscope is used for transreflection photography of fish teeth at 5× magnification. After mounting in epoxy and polishing, samples were put into the TESCAN MIRA3 field emission microscope to take backscattered electron images (BSE).
Modern fish teeth D5 and QDH2 were selected as representatives for the laser Raman spectroscopy test (Horiba JY 800). The laser beam was directly aimed at the fish teeth for detection with an objective magnification of 50 and an excitation wavelength of 532 nm. The spectral acquisition beam range was 200–1,200 cm-1 with a slit of 100 μm, an aperture of 400 μm, and a grating of 1,800 gr/nm. The exposure time was 30 s and the data were interpreted using Origin 2018 software after the test.
The major oxide compositions of the modern and fossil fish teeth were determined with an electron micro probe analysis (EMPA, JEOL JXA-iSP 100). The microprobe was operated at an accelerating voltage of 15.0 kV, a beam current of 10.0 nA, and a beam size of 5.0 μm. The experimental conditions for the surface analysis of D5 were accelerated at an accelerating voltage of 15.0 kV, a beam current of 20.0 nA, and a minimum beam size. Data were corrected online using a modified ZAF [atomic number (Z) effect, absorption (A) effect, and fluorescence excitation (F) effect] correction procedure.
A laser ablation inductively coupled plasma mass spectrometer (LA-ICP-MS) was used to analyze trace elements in fossil and modern fish teeth. The Resolution SE 193 nm excimer laser was coupled to an Agilent Model 7900 inductively coupled plasma mass spectrometer. Samples analysis was conducted at or near the EMPA spot locations under laser conditions with a beam spot diameter of 38 μm, denudation frequency of 10 Hz, and energy density of 4 J/cm2. The trace element concentrations of the fish teeth were calibrated against the standard reference materials (National Institute of Standards and Technology (NIST) 610 and NIST 612). Suitable internal standards (BHVO-2G and BIR-1G from the United States Geological Survey) were also carried out for the data calibration. During the test, two NIST 610 standard samples and one of each NIST612, BHVO-2G, and BIR-1G standard samples were analyzed every eight sample points. The relative standard deviations of most of the trace elements of the standards were within 5%. The concentration of trace elements was calculated using the Calcium (Ca) element as the internal standard based on the electron probe data at each measuring point. Specifically, we performed an in-situ LA-ICP-MS test at the same point as the electron probe test. This test and data correction method can ensure the consistency and reliability of trace element data, and avoid the systematic error caused by a fixed internal standard. The software Iolite V4.0 was adopted for data processing (Paton et al., 2011).
87Sr/86Sr and 143Nd/144Nd isotopic ratios were analyzed in situ in low resolution and static mode using a Neptune Plus Multi-Collector Inductively Coupled Plasma Mass Spectrometer (MC-ICP-MS) (Thermo Fisher Scientific, Germany) coupled to a J-200 343 nm femto second laser ablation system (Applied Spectra, USA).
At the beginning of every analytical session, the fs-LA-ICP-MS system was optimized using NIST 612 to achieve the maximum signal intensity and low oxide rates. The samples were ablated in line mode with a spot size of 30 μm, a line length of 20 μm, a stage movement speed of 0.65 μm/s, a laser repetition rate of 8 Hz, and a beam energy density of 1.5 J/cm2.
The instrumental mass bias for the Sr isotopes was corrected using an exponential function based on the 86Sr/88Sr value of 0.1194. Correction of the interferences of the Krypton (84Kr) and 86Kr isotopes was successfully accomplished by background subtraction. The interferences of the doubly charged ions of Erbium (168Er2+) on 84Sr, 170Er2+ and Ytterbium (170Yb2+) on Rubidium (85Rb), 172Yb2+ on 86Sr, and 174Yb2+ on 87Sr were corrected based on the measured signal intensities of 167Er2+ (m/z 83.5) and 173Yb2+ (m/z 86.5) and the natural isotopic compositions of Rb, Er, and Yb (Li et al., 2018). As for the Nd and Samarium (Sm) isotopes, the instrumental mass biases were corrected by an exponential function based on the 146Nd/144Nd value of 0.7219 and the 147Sm/149Sm value of 1.0868, respectively (Yang et al., 2014). The interference of 143Sm on 143Nd was corrected for the mass bias coefficient and the natural isotopic composition based on the measured signal intensity of 147Sm. To monitor the instrument’s stability and for external correction of the 147Sm/144Nd ratios, the Durango and MAD apatite reference standards were analyzed. The relative standard deviations of the Sr and Nd isotopes of standards were within 2%.
Due to the contents of Nd in marine and lake fish teeth being below the limit of detection, the Nd isotopes in modern fish teeth were not obtained. The Nd isotopic compositions are generally expressed as ϵNd (t=0) (), where (143Nd/144Nd)Sample is the value of the actual sample and (143Nd/144Nd)CHUR is the value of the chondritic uniform reservoir, calculated using (143Nd/144Nd)CHUR=0.512630 (Bouvier et al., 2008).
Fossil and modern fish teeth show distinct morphological characteristics respectively. The teeth of the Mylopharyngodon piceus from Qiandaohu Lake are approximately 5 mm in width and length showing a nightcap style (Figure 2b). In contrast, the teeth of marine fish from the ECS are tapered, and smaller with a length of approximately 1 mm (Figure 2a). The fossil fish teeth show similar morphology and structure to the modern marine fish teeth but were much smaller with only 100–250 μm and/or less. Beside cones, the fossil fish teeth also showed ellipses, spindles, long stripes, and other shapes (Figure 2c). All fish teeth have a two-layer structure of enamel and dentin. The dentin is inside the fish teeth with obvious pores, indicating a relatively loose texture. The enamel is a thin light-colored dense outer layer of the fish teeth (Figure 2), which exhibits the typical growth structure clearly.
Figure 2. Comparison of the morphological features of fish teeth based on BSE photos: (a) Modern fish teeth from the ECS; (b) Modern fish teeth from Qiandaohu Lake; (c) Representative fossil fish teeth from the CIOB.
The marine fish teeth and lake fish teeth in this study had similar Raman spectra with four vibration modes of apatite [PO4]3-, indicating that the main component is hydroxyphosphate. The enamel showed a generally higher peak intensity than that of dentin (Figure 3). The main peak of marine fish teeth enamel was 1,030 cm-1 (Figures 3a, b), representing the A-type substitution of carbonate (that is, the substitution of hydroxyl), while the main peak of lake fish teeth enamel was 1070 cm-1 (Figures 3e, f), representing the B-type substitution of carbonate (that is, the substitution of phosphate). There were both A-type and B-type substitutions in the dentin of the marine and lake fish teeth.
Figure 3. Raman spectra of modern fish teeth [(a–d): marine fish teeth D5, where (a, b) are in the enamel; (c, d) are in the dentin; (e–h): lake fish teeth QDH2, where (e, f) are in the enamel, (g, h) are in the dentin].
A similar double-layer structure in fossil fish teeth was indicated, and both the enamel and dentin of fossil fish teeth are composed of hydroxyapatite. Moreover, the x-ray diffraction (XRD) of the enamel also showed a higher peak than that of dentin, indicating higher crystallinity of enamel (Liao et al., 2019a).
Elements mapping of the living fish teeth (sample D5 as an example) showed that the major elements vary in the profile (Figure 4). The contents of fluorine (F), natrium (Na), phosphorus (P), calcium (Ca), and ferrum (Fe) increased from the root to the tip and decreased from the enamel to the central dentin. In contrast, the contents of magnesium (Mg) increased from the root to the tip, and from the lateral enamel to the medial dentin. Sr and sulfur (S) were evenly distributed in the dentin and enamel of fish teeth without an obvious tendency.
The main element compositions of both fossil and modern fish teeth are CaO and P2O5, and there is a significant positive correlation between them (Figure 5a). Generally, the fossil fish teeth are characterized by higher contents but narrower fluctuations of CaO (44.32%–51.96%) and P2O5 (36.03%–40.73%) than the modern fish teeth (29.00%–48.49% for CaO and 24.77%–42.36% for P2O5) as shown in Table 1. CaO/P2O5 in the fish teeth was broader than the stoichiometric ratio of apatite (10/6) but was basically within the range of CaO/P2O5 in fossil fish teeth and mammal bones samples studied previously [from 1.2 to 2.0, Nemliher et al. (2004)]. CaO/P2O5 in the fossil fish teeth varied in the range of 1.19 to 1.39, while CaO/P2O5 in the modern fish teeth varied in the range of 1.00 to 1.27. The higher CaO/P2O5 in the fossil fish teeth may indicate the influence of the fossilization and diagenesis with the replacement of orthophosphate-ion by carbonate-ion in the apatite crystal lattice (Nemliher et al., 2004).
Figure 5. (a) CaO/P2O5 and (b) Sr/Ca ratios of fish teeth in this study. CaO and P2O5 data are from major element concentrations tested by EMPA and summarized in Table 1, while Sr data are from trace element concentrations tested by LA-ICP-MS and summarized in Table 2.
Except for CaO and P2O5, F had the highest content in the fossil fish teeth, ranging from 0.94% to 3.12%. It was much higher than the modern fish teeth with 0.00%–1.05%. Furthermore, the fossil fish teeth were also distinctly more enriched in Na2O, FeO, and SiO2 (Table 1), which may be due to the porous particles with inclusions of terrigenous material after deposition (Nemliher et al., 2004). The lowest CaO/P2O5 was observed in GC03 (as shown in Table 1), together with significantly higher contents of Al2O3, SiO2, and K2O than GC04 and GC11, suggesting intense influence from terrigenous materials in core GC03 as well. Inversely, the modern fish teeth had higher MgO, K2O, and chlorine (Cl) (Table 1). As essential mineral elements for fish life, teeth, bone, and scales are important sinks of these elements (Bijvelds et al., 1998).
Generally, most trace elements were detected in fossil fish teeth and varied greatly. Some trace elements including beryllium (Be), germanium (Ge), cadmium (Cd), indium (In), thallium (Tl), bismuth (Bi), and most REEs (except lanthanum, cerium, yttrium) were below the limit of detection in both the marine and lake fish teeth. Vanadium (V), nickel (Ni), arsenic (As), stannum (Sn), and thorium (Th) only in the marine fish teeth while Hf was detected only in the lake fish teeth (Table 2).
The marine and lake fish teeth had different contents of trace elements. Using the ratio of element contents of marine fish teeth to those in lake fish teeth (Mi/Li = element i in marine fish teeth/in lake fish teeth) as shown in Figure 6, the trace elements can be divided into three categories:
1. Strontium (Sr), copper (Cu), scandium (Sc), cobalt (Co), uranium (U), titanium (Ti), ferrum (Fe), niobium (Nb), lead (Pb), barium (Ba), gallium (Ga), manganese (Mn), chromium (Cr), lithium (Li), cesium (Cs), nickel (Ni), vanadium (V), and arsenic (As) had higher contents in marine fish teeth than in lake fish teeth. Among them, Mn and U had Mi/Li ratios higher than 5, reflecting the obvious enrichment in marine fish teeth. Nb, Pb, Li, and Sr were moderately enriched with Mi/Li ratios of 2–5. Cu, Sc, Co, Ti, Fe, Ba, Ga, and Cr were slightly enriched in marine fish teeth with Mi/Li ratios of 1–2.
2. Zirconium (Zr), molybdenum (Mo), tungsten (W), zinc (Zn), and rubidium (Rb), with Mi/Li ratios of <1, were deficient in marine fish teeth and could be sorted as W (0.07) < Mo (0.17) < Rb (0.51) < Zn (0.55) < Zr (0.90).
3. The Mi/Li ratios of Beryllium (Be), germanium (Ge), stannum (Sn), cadmium (Cd), indium (In), hafnium (Hf), thallium (Tl), thorium (Th), bismuth (Bi), and rare earth elements (REEs), were unavailable due to their absence in marine fish teeth and/or lake fish teeth.
To analyze the ability of fish to aggregate trace elements over the life cycle, the enrichment index of trace elements of modern marine fish teeth was calculated relative to the seawater around Yangshan Island (Zeng et al., 2012) (Mi/Wi = element i in marine fish teeth/seawater). The lake fish teeth were not considered due to a lack of reliable background values of trace element content in the water bodies of the Xin’an River Basin. The elements that were absent in marine fish teeth were also not included. It was indicated that the Mi/Wi ratio for marine fish teeth varied greatly in the range from lower than 10-1 to higher than 103, as shown in Table 3 and Figure 6. Most of them were higher than 1, indicating obvious enrichment. Rb was the only deficit trace element with a Mi/Wi of 0.39. Cu, Co, Pb, Ba, W, Zn, Cr, and Sr were highly enriched with a Mi/Wi higher than 102, and Mn, Ga, Zr, Sn, and Th had a Mi/Wi higher than 103.
The trace element composition of fossil fish teeth in core sediments of the Indian Ocean was compared with that of modern marine fish teeth (Fi/Mi = element i in fossil fish teeth/marine fish teeth). To analyze the trace elements enrichment degree of fish teeth after deposition, grading with a 10-fold gradient was used, as shown in Figure 6.
Type I: slightly enriched with Fi/Mi of 1–10, including Sr, Li, Sn, Rb, Zn, Cr, Mn, Ga, As, and Ba. Among them, Sr had the lowest ratio of 1.13.
Type II: moderately enriched with Fi/Mi between 10–100, including W, Pb, V, Ni, Nb, Fe, Ti, Cs, and Mo.
Type III: highly enriched with Fi/Mi between 100–1,000, including U, Co, Sc, and Cu.
Type IV: extremely enriched with Fi/Mi higher than 1,000, with only two elements, Zr and Th, belonging to this type.
Besides the above four categories, there were special types of trace elements that were absent in modern marine fish teeth but present in fossil fish teeth. Among them, REEs were extremely high in fossil fish teeth, reaching 7,546.72 ppm (Table 4). Be and Ge had high concentrations in fossil fish teeth as well, with 4.03 ppm and 25.87 ppm (Table 2), respectively. In addition, the concentrations of Cd (0.65 ppm), In (0.03 ppm), Hf (0.5 ppm), Tl (0.11 ppm), and Bi (0.07 ppm) were observed to be low in the fossil fish teeth. It was deduced that these elements were aggregated in the fossil fish teeth after deposition.
In summary, trace elements with enhanced concentrations after deposition include the rare and rare earth elements family, represented by Zr, Th, Sc, and REEs with significant enrichment, followed by iron family elements, such as Ti, V, Fe, Co, Ni and Cu, with no obvious enrichment of rock-forming family elements such as Ba, Rb, Li, and Sr, in fossil fish teeth. It is noteworthy that REEs were highly enriched after deposition, which was obviously different from the enrichment of Sr during the life cycle of fish. The distinctly different enrichment procedures of REEs and Sr are an important basis for source tracing when using their respective isotopes.
Furthermore, compared with GC04 and GC11, the fossil fish teeth from GC03 contained higher concentrations of Sc, V, Zn, Sr, Zr, Mo, Hf, W, and U but significantly lower concentrations of Fe, Ni, Ga, Rb, Sn, and Cs (Table 2).
Mi/Wi represents the ratio of marine fish teeth/Yangshan sea water (data from Zeng et al. (2012)); Fi/Mi represents the ratio of fossil fish teeth/marine fish teeth; Mi/Li represents the ratio of marine fish teeth/lake fish teeth.
(Due to their non-detection in fish teeth, the concentration coefficients of Be, Ge, Cd, In, Hf, Tl, Bi, Th, Ti, Nd, and REE could not be calculated.
The REE in the fossil fish teeth is further discussed in detail. Table 4 shows the REE content in fossil fish teeth from the three cores. The concentrations of ΣREEs in the fossil fish teeth varied greatly from hundreds to thousands of ppm. The average values of REEs can be sorted as GC03 (8,971.93 ppm) > GC11 (7,690.59 ppm) > GC04 (5,707.63 ppm).
The highest REE concentration in GC03 was aligned with higher concentrations of Sc, V, Zn, Sr, Zr, Hf, Mo, W, and U (Table 2). The symbiosis of these elements in fossil fish teeth is obviously closely related to the isomorphism of these elements to Ca2+ in fish tooth apatite. Generally, U in the apatite is positively related to P by absorbance, co-deposits, or substitution with Ca (Hughes and Rakovan, 2002; Pan and Fleet, 2002). Therefore, U together with REEs, can be accumulated by fish teeth in deep-sea sediments (Li et al., 2023). The relatively low Ca/P ratio of the fossil fish teeth from the GC03 may be important in explaining the enriched REEs and U, which is consistent with the distinct negative correlation between REEs and CaO/P2O5 in REE-rich sediments (Zhang et al., 2017).
Similar to previous studies, distinct fractionation of REEs was observed in the fossil fish teeth. The normalized REE patterns of the post-Archean mean shales of Australia [PAAS, Taylor and McLennan (1985)] (as shown in Figure 7) have been shown to be consistent with fossil fish teeth in the Pacific Ocean and the Indian Ocean in previous studies (Liao et al., 2019a; Sun et al., 2022; Liao et al., 2022; Deng et al., 2022) with (La/Yb)N < (La/Sm)N < (Sm/Yb)N. The REE distribution pattern was consistent with the bottom seawater of the Indian Ocean [data from Nozaki and Alibo (2003)], with strong negative Ce anomalies (range from 0.01 to 0.26) and slight positive Y anomalies (ranging from 1.04 to 1.46).
Figure 7. REE pattern in fossil fish teeth normalized by PAAS with a comparison with previous studies.
It is noteworthy that the REE normalization pattern between fossil fish teeth was significantly different from that of deep-sea sediments in this study (Figure 7). The sediments of GC04 and GC11 in the CIOB showed a slight left-leaning flat type, and the sediments of GC03 from the Wharton Basin showed a right-leaning flat type. But all the fossil fish teeth showed an obvious strong negative Ce anomaly and a weak positive Y anomaly. The distinct differences in REE normalization patterns between the sediments and fossil fish teeth in this study were different from those from the Pacific Ocean where the REE normalization patterns in the sediments and fossil fish teeth were consistent and showed an obvious negative Ce anomaly and a positive Y anomaly (Bi et al., 2021; Ren et al., 2022; Sa et al., 2018; Liao et al., 2019a).
The bulk sediments data of GC03, GC04, and GC11 are from Zhang et al. (2023); the bulk sediments data of the Pacific Ocean are from Sa et al. (2018); the Indian Ocean fish teeth data are from Sun et al. (2022); the North Pacific fish teeth data are from Liao et al. (2019a); the Western Pacific fish teeth data are from Deng et al. (2022); the Southeast Pacific fish teeth data are from Liao et al. (2022); the Indian ocean deep water data are from Nozaki and Alibo (2003).
Due to the extremely low Nd content in the modern fish teeth, the 143Nd/144Nd ratio was available only in the fossil fish teeth. The 87Sr/86Sr values of the modern fish teeth are shown in Table 5 and 6 exhibits the 143Nd/144Nd ratio and 87Sr/86Sr values for the fossil fish teeth.
The 87Sr/86Sr of marine fish teeth was less radiogenic but fluctuated in a wider range (from 0.70633 to 0.70913, with an average of 0.70832) than that of the lake fish teeth (from 0.70918 to 0.70973, with an average of 0.70953). Both the Sr content and 87Sr/86Sr isotopic ratio in different marine fish varied in a wide range, as shown in Table 5 and Figure 8. The Cynoglossus semilaevis (D2) had the highest Sr content and range of variation, and also had the highest average 87Sr/86Sr ratio, while the Miichthys miiuy (D1) and Larimichthys crocea (D4) had the lowest Sr contents and the least radioactive but biggest fluctuation of 87Sr/86Sr. The values of the Scomber japonicus (D3) were located in the transition region.
The 87Sr/86Sr ratios of the fossil fish teeth ranged from 0.70808 to 0.71075, with an average of 0.70942, close to that of the lake fish teeth but significantly more radioactive than that of the marine fish teeth. Among the fossil fish teeth from different cores, 87Sr/86Sr can be ranked as GC03 < GC04 < GC11 from the point of view of minimum, maximum, and average values. The ϵNd of the fossil fish teeth from the three cores ranged from -8.1 to -2.2 with an average of -4.7, in a sequence of GC04 (-4.2) > GC03 (-4.3) > GC11(-5.7). The fossil fish teeth in the GC03 showed the widest ϵNd range (-7.0–2.2), while the ϵNd of GC04 (-6.7–2.2) and GC11 (-8.1–3.9) varied in a relatively narrow range, as shown in Table 6.
Except for Mo, W, Zn, and Rb (the contents of which were significantly higher in lake fish teeth), the contents of most elements were higher in the marine fish teeth and further increased by different degrees in the fossil fish teeth after deposition. To evaluate the aggregation capability of the trace elements in the fish teeth during different processes, including the life cycle and post-deposition, Mi/Wi and Fi/Mi were introduced in this study for comparison. Mi/Wi represents the ability of fish teeth to absorb and accumulate trace elements from the water in which they live, while Fi/Mi represents the degree of trace elements diffusion and adsorption to fish teeth after death. Both the amount and the magnitude of trace element enrichment were considered to evaluate the enrichment degree of trace element during the life and after deposition. Then, trace elements were divided into three categories based on their geochemical properties.
1. Type I represents a category of elements that were mainly accumulated in the fish teeth during life with Mi/Wi greatly higher than Fi/Mi, including Sr, Li, Ba, Cr, Mn, Zn, Ga, Sn, Pb, and W. The amount of this type of element was significantly enhanced with a larger magnitude mainly through absorbance by the fish during the life cycle. These elements belong to IA, IIA, IIIA, and IVA groups respectively in the periodic table of elements. The outer electron shells are basically ns1 (Li, Cr, and Ga), ns2 (Sr, Ba, Mn, Zn, and W), and ns2np2 (Sn and Pb). Their outer electrons are apt to lose and remain stable under oxidation and weak-reduction conditions. It is hypothesized that these elements enter into the organism during life from seawater by ion absorption. Isomorphism with Ca, Mg, Fe, and K may be the reason for their main occurrence in bioapatite.
2. Type II is a group of elements that accumulated in the fish teeth during both the life cycle and post-deposition, including Mo, Rb, U, V, As, Co, Ti, Fe, Cu, Zr, and Th. They exhibited similar degrees of Fi/Mi and Mi/Wi. From the view of magnitude change, the ability of modern fish teeth to absorb these elements from ambient water is similar to that of fossils to adsorb after deposition. These elements belong to the IA, VA, transition elements, and Actinides groups with unsaturated outer electron and/or inner electron shells. Most of them are redox-sensitive elements exhibiting variable valences under different redox conditions. These elements can be accumulated through ion absorption in fish teeth during the life cycle of the fish with a lower valence and can be enriched continuously through adsorption into the fossils in the deep seawater-sediment interface after the deposition period. A higher valence is indicated in the later stage but needs further investigation.
3. Type III represents elements that are enriched in fossils only after deposition, including REEs, Be, Ge, Cd, Sc, Hf, and Tl. They are mainly members of Lanthanides and rare dispersed elements groups with a large atomic radius and strong chemical activity. Except for Be, which has the atomic electronic structure of 1s22s2 with a saturated inner electron shell, the rest have an unsaturated inner electron shell of nd10-n(nf14-n) (n+1)s2 or unsaturated outer electron shell of ns2(n+1)p6-(n+1). Ions with a +3 or +4 valence are their main occurrence state. These elements are undetectable in living fish teeth but are highly enriched after fossilization, and, among them, REEs were the most enriched with the highest concentration of 7,546.72 ppm detected in fossil fish teeth in this study. Their large ionic radius and extremely low content in seawater hinder them from entering into the organism and accumulating. However, as the collagen in the fish teeth decomposes during early diagenesis after deposition (Tütken et al., 2008), a large number of pores are generated in the phosphate skeleton, leading to the recrystallization of nanoscale biogenic apatite in the diagenetic fluid and the adsorption of lanthanides from the surrounding environment with other rare dispersed elements (Trueman et al., 2004). This process occurs under oxidizing or suboxidizing environments at the deep seawater-sediment interface, accompanied by low sedimentation rates and strong adsorption, resulting in the extreme accumulation of REEs in particular.
It should be noted that F in fossil fish teeth was much higher than that in modern fish teeth with 0.00%–1.05%, which can be attributed to the transformation of hydroxyapatite into hydroxyfluorapatite after deposition in which the hydroxide is replaced by F. This transformation was indicated to be the main factor accelerating the adsorption of Nd, resulting in 3 to 6 orders of magnitude more Nd in fossil fish teeth than in living fish teeth (Wright et al., 1984; Shaw and Wasserburg, 1985; Staudigel et al., 1985). The extreme enrichment of REE in bio-apatite is related to ion exchange with REE3++Na+→2Ca2+; REE3++Si4+→Ca2++P5+. It has been shown that biological apatite is composed of elongated nanocrystals arranged with hexagonal hydroxyapatite cross sections, with large porosity and extremely high specific surface area, which is conducive to REE adsorption. In addition, Si-rich regions appear along the nanoboundary, showing element exchange along the mineral nanocrystal boundary, confirming the presence of Na, Si, Ce, and Y in the biological apatite nanocrystals, and indicating that atoms heavier than Ca (Ln, such as Ce and Y) are substituted into the lattice, with weaker peaks associated with the exchange of lighter Na atoms (Liao et al., 2019a).
In general, the occurrence of trace elements in fish teeth is influenced by the geochemical properties of elements, the crystal structure of bio-apatite, and the redox conditions in different processes. This can be shown in a two-stage three-classification model, as shown in Figure 9.
As a highly enriched trace element in modern fish (Mi/Wi=293.6, Figure 6), Sr is indicated to be mainly absorbed by the fish teeth during life and is slightly enriched by recrystallization exchange after deposition (with the smallest Fi/Mi of 1.13, Figure 6). Previous research has proved that the deposition of some trace elements in otolith is related to the history of fish migration in addition to their contents in water and the environmental temperature (Beck et al., 1992; Izzo et al., 2018; Hüssy et al., 2021; Arai and Kimura, 2022). In this study, the much higher Sr content in the marine fish teeth (1425.18 ppm) than lake fish teeth (455.10 ppm) is consistent with the large difference of Sr content in seawater (7.88 ppm) and in rivers (0.09 ppm) (Anadón et al., 2002). In addition, the Sr/Ca ratio was significantly positively correlated with Sr content in fish teeth (R2 = 0.87) (Figure 5b), but differences in Sr/Ca and 87Sr/86Sr were also found among different modern marine fish species (Figure 8). It is reasonable to assume that element composition (especially Sr and Ca) was also recorded in the fish teeth, similar to those in the otolith (Avigliano et al., 2018; Bock et al., 2017). This means that in the process of material exchange between fish and the external environment, some trace elements are continuously ingested into the fish, and after a series of metabolisms and circulations, finally also deposited in fish teeth.
In addition, the observed lower 87Sr/86Sr of marine fish teeth (0.70832) than that of lake fish teeth (0.70953) (Figure 8) is consistent with the difference of 87Sr/86Sr between seawater [modern seawater average 87Sr/86Sr=0.70917 (McArthur et al., 2020)] and inland freshwater [world river average 87Sr/86Sr=0.71190 (Palmer and Edmond, 1989)], while the 87Sr/86Sr of the lower reaches of Yangtze River (Datong station) was 0.71063 (Luo et al., 2014). Both the 87Sr/86Sr of the lake fish teeth and marine fish teeth were lower than the ambient waters, and the 87Sr/86Sr ratios of all the fish teeth were lower than that of modern seawater (Figure 8). It was noted that the Sr/Ca and 87Sr/86Sr of D1 and D4 overlapped greatly, while D2 exhibited significantly higher Sr/Ca and 87Sr/86Sr, and D3 was located in the transition region of Sr/Ca-87Sr/86Sr (Figures 5b, 8). The significantly different Sr/Ca-87Sr/86Sr among these marine fish teeth should be closely related to their different migration habit. As mentioned, D1, D3, and D4 usually migrate from deep water to nearshore for relatively long distances, thus recording a chemical composition and 87Sr/86Sr ratios in a wide range. In comparison, due to its lack of nearshore migration, D2 has narrow 87Sr/86Sr ratios. A trigonometric diagram of Sr/Ca- 87Sr/86Sr-Sr was then created to distinguish different aquatic environments, as shown in Figure 8.
Previous studies have pointed out that andesite on Taiwan Island (87Sr/86Sr=0.70489) and basalt on the coast of Zhejiang and Fujian provinces (87Sr/86Sr=0.70805) are important material sources for the ECS (Mi et al., 2017). Moreover, the Okinawa Trough, located at the eastern boundary of the ECS, is tectonically active with frequent volcanism. The acidic volcanic rock in the north-central trough has 87Sr/86Sr ranging from 0.70406 to 0.70544 (Huang et al., 2006). These potential sources of Sr isotopes may result in the less radioactive 87Sr/86Sr composition of the regional seawater of the ECS and are recorded in the fish teeth during migration. 87Sr/86Sr in the lake fish teeth varied in a narrow range but was higher than the 87Sr/86Sr of carbonate rocks in the Yangtze River Basin, varying from 0.70800 to 0.70900 (Chetelat et al., 2008), also suggesting that the weathering of exposed marine carbonate rocks in the area around Qiandaohu Lake may result in relatively low 87Sr/86Sr in the lake water and subsequent adsorbed to fish teeth.
In comparison, the correlation between Sr/Ca-Sr was more significant in the fossil fish teeth (R2 = 0.98, Figure 5b), and similar consistent characteristics could be found in 87Sr/86Sr (Figure 8). In the case of the main component remaining unchanged under an open system, a strong replacement of Sr after deposition accompanies the significantly strong correlation between Sr/Ca-Sr. However, it is interesting that a negative correlation of Sr-87Sr/86Sr was observed for the fossil fish teeth. It was proved that Sr changed because of teeth recrystallization and exchange with pore fluid Sr2+ during burial and diagenesis (Martin and Scher, 2004). In this process, the interference of the Sr isotope from different sources with the original Sr isotope in fish teeth cannot be ignored.
The average 87Sr/86Sr in fossil fish teeth from the CIOB is 0.70942 (from 0.70808 to 0.71075), significantly more radiogenic than the ancient and modern seawater (increasing from 0.70770 at about 40 Ma to 0.70920 at present) (McArthur et al., 2001). Previous studies have indicated that the Sr isotope may be recrystallized and exchanged with Sr in pore water after deposition under the influence of diagenesis, which affected the original Sr isotope record (Martin and Scher, 2004). The 87Sr/86Sr of the fossil fish teeth in the 2-m cores from the CIOB was higher than the fossil fish teeth (87Sr/86Sr=0.70853) and foraminifera (87Sr/86Sr=0.70849) buried deep in the Indian Ocean but was very close to the 87Sr/86Sr of the silicate components (87Sr/86Sr=0.70944) in the surface sediments of the CIR and has a strong terrigenous property (Table 7). The results of chemical leaching experiments from Western Pacific sediments show that although the 87Sr/86Sr of phosphate components (87Sr/86Sr=0.70830–0.70917) is between the range of seawater since 25 Ma, it does not decrease with the increase of depth, which also indicates a strong terrigenous attribution (Ren et al., 2022).
Table 7. Comparison of Sr-Nd isotopes and different components in fossil fish teeth from different sea areas.
It is interesting that the corresponding lower ϵNd under the influence of terrigenous debris was not observed in the fossil fish teeth, and, on the contrary, the ϵNd was more radioactive with an average of -4.7 (from -8.1– -2.2) (Table 7). It was also more radioactive than the Antarctic circumpolar current (ACC) and the Indian Ocean mean water [ϵNd=-7–9, Albarède et al. (1997)] recorded by iron manganese oxides in the seabed, and appears to be very close to the Pacific Ocean [ϵNd=-5–4, Ling et al. (2005)] and the silicate components in the surface sediments of the SWIR [ϵNd=-4.6, Subha Anand et al. (2019)], indicating the uncertainty of the paleoceanographic information record in the samples of this study.
Recent studies have found that REEs in pore water near the sediment-seawater interface may be an important source of rare earth elements in rare earth-rich sediments (Liao et al., 2019b; Deng et al., 2022). Bioapatite can obtain REEs from terrigenous detrital deposits through pore water (Elderfield and Pagett, 1986) and the Nd isotope of biological apatite in volcanic detrital deposits has obvious volcanic origin characteristics (Zhao et al., 2013). A study found that ϵNd in fossil fish teeth was unevenly distributed and varied widely, gradually increasing from the root to the tip of fish teeth and was significantly different from the seawater (Deng et al., 2022). An inference from this is that the original ϵNd values of bioapatite in deep-sea sediment may be altered by pore water with high Nd concentrations and low ϵNd values during diagenesis. The difference was also found in this study, but the trend was reversed so that the ϵNd of the fossil fish teeth was more radioactive than the Indian Ocean water. This may be related to the same high Nd concentrations but different ϵNd of pore water under the influence of sediment components.
For the sediment input of the CIOB, a higher 87Sr/86Sr ratio and lower ϵNd near Antarctica indicate old granitic rocks as the source, and the opposite is observed in sediments from the Central and the Southwest Indian Ridges, indicating seafloor spreading and mid‐ocean ridge hydrothermal activity as the source (Subha Anand et al., 2019). It is noteworthy that the 87Sr/86Sr ratio increases along with decreasing Sr content in the fossil fish teeth from GC03 (0.70924) and GC04 (0.70940) to GC11 (0.70964). It is possible that the GC11 core, at a lower latitude than the GC03 and GC04 cores, was more influenced by continental detritus from Antarctica sources with high 87Sr/86Sr. Furthermore, high field strength elements Hf and Zr, large ion lithophile element Sr, and rare earth elements exhibited a decreasing trend in the fossil fish teeth with GC03>GC04>GC11 (Table 2), which indicates a weakened terrigenous signal from GC11 and GC04 to GC03. It is reasonable to speculate that fine terrestrial debris with high 87Sr/86Sr may enter the fine pores of fish teeth or through exchange of Sr isotopes at the microscopic level after deposition but with minor impact on the Sr content. If so, fossil fish teeth may preserve the influence from enhanced continental weathering signal and prove the long diagenesis influence after deposition (Martin and Scher, 2004).
As for the significantly more radioactive ϵNd, it may be closely related to the release of Nd from volcanic hydrothermal components in sediments into pore water. While cores GC03 and GC04 were less affected by Antarctic continental debris, they received more from seafloor spreading and mid-ocean ridge hydrothermal activity (Subha Anand et al., 2019), and the radioactive ϵNd of these materials may have been absorbed by fish teeth through pore water after deposition. This means that fossil fish teeth may also preserve the influence from volcanic hydrothermal weathering signal and the influence is multi-factor controlled. In general, the suspected sources or continuous absorption of Sr and REEs from pore water could occur, which may cast doubt on the reliability of fish teeth fossils as valid records of deep-sea environments in Earth’s history over millions of years. The fractionation of Sr-Nd isotopes in fish teeth and the related diagenetic change after burial are worthy of further study.
To further explore the potential capability of trace elements, 87Sr/86Sr, and ϵNd in modern and fossil fish teeth to be indicators in aquatic environments, material sources tracing, and paleoceanography reconstruction, the mineralogy, major and trace elements, and isotopic composition of fossil fish teeth from the Central Indian Ocean and modern marine and lake fish teeth from East China Sea and Qiandaohu Lake in eastern China were examined and compared. The main conclusions we reached are as follows:
1. According to the element contents in living and fossil fish teeth, the Mi/Wi and Fi/Mi ratios were calculated, which indicated that elements enter into fish teeth during the life cycle of the organism and/or into fossil fish teeth after deposition. Three types of trace elements were classified according to their different manners of enrichment. Type I: Sr, Li, Ba, Cr, Mn, Zn, Ga, Sn, Pb, and W, belonging to the IA, IIA, IIIA and IVA groups, respectively, in the periodic table of elements, can be easily oxidized and accumulate during life cycle into the fish teeth through absorption and remain stable under the oxidation and weak-reduction conditions of the modern marine environment. Type II: Mo, Rb, U, V, As, Co, Ti, Fe, Cs, Cu, Zr, and Th, belonging to the IA, VA, transition elements, and Actinides groups, exhibit variable valence under different redox conditions. These elements can be enriched through ion absorption in fish teeth during the life cycle with a lower valence, and also can be aggregated continuously through adsorption into fish teeth with a high valence in the deep seawater-sediment interface after deposition. Type III: REEs, Be, Ge, Cd, Sc, In, Hf, Tl, and Bi, mainly members of Lanthanides and rare dispersed elements groups with a large atomic radius and strong chemical activity. These elements are enriched only after deposition with a large number of pores generated in the phosphate skeleton, leading to the recrystallization of nanoscale biogenic apatite in the diagenetic fluid and the adsorption of lanthanides from the surrounding environment with other rare dispersed elements. It is indicated the enrichment processes are closely associated with the geochemical properties of these elements, the isomorphism and mineral crystal structure, and the redox environments.
2. The Sr/Ca and 87Sr/86Sr in the modern fish teeth reflect the influence of different aquatic environments and fish migration. The generally lower 87Sr/86Sr in the modern fish teeth may be affected by the contribution of Sr isotopes from regional sediment sources. As for the differentiation of Sr/Ca and 87Sr/86Sr in the modern fish teeth, it is mainly related to the migration of the fish. Changes in geochemical elements and Sr isotope composition of swimming fish between seawater and freshwater during migration have been recorded in fish teeth.
3. The more radioactive 87Sr/86Sr ratios in fossil fish teeth than in modern seawater reflect the influence of terrigenous sources on fossil fish teeth. The fact that there was increased 87Sr/86Sr in the fossil fish teeth from GC03, GC04 and GC11 suggests more Sr isotope contributions from Antarctic continental detritus. Furthermore, the higher ϵNd values of the fossil fish teeth than those of the Indian Ocean may reflect the effect of pore water on the enrichment of REEs in fossil fish teeth after deposition. The release of radioactive ϵNd from the mantle-derived volcanic hydrothermal components into pore water may be a possible explanation but needs further study.
The original contributions presented in the study are included in the article/supplementary material. Further inquiries can be directed to the corresponding author.
The manuscript presents research on animals that do not require ethical approval for their study.
XZ: Data curation, Formal analysis, Methodology, Software, Validation, Visualization, Writing – original draft, Writing – review & editing. ZX: Conceptualization, Data curation, Formal analysis, Funding acquisition, Methodology, Project administration, Resources, Supervision, Writing – original draft, Writing – review & editing. KZ: Data curation, Methodology, Resources, Writing – original draft. MH: Data curation, Investigation, Writing – original draft. MY: Data curation, Investigation, Writing – original draft. ZL: Data curation, Writing – original draft. ZQ: Methodology, Investigation, Writing – original draft. YL: Data curation, Formal analysis, Methodology, Writing – original draft.
The author(s) declare that financial support was received for the research and/or publication of this article. This study was supported by the National Natural Science Foundation of China (41773005) and the 13th Five-Year Plan Project for the Investigation and Development of International Marine Resources (DY135-R2-1-01).
We thank Prof. Songming Zhu and Zhangying Ye of the College of Biosystems Engineering and Food Science, Zhejiang University of China for the lake fish teeth samples for this study, and Prof. Jingsong Bao of College of Agriculture and Biotechnology, Zhejiang University of China for providing experiment supervision.
The authors declare that the research was conducted in the absence of any commercial or financial relationships that could be construed as a potential conflict of interest.
The author(s) declare that no Generative AI was used in the creation of this manuscript.
All claims expressed in this article are solely those of the authors and do not necessarily represent those of their affiliated organizations, or those of the publisher, the editors and the reviewers. Any product that may be evaluated in this article, or claim that may be made by its manufacturer, is not guaranteed or endorsed by the publisher.
Albarède F., Goldstein S. L., Dautel D. (1997). The neodymium isotopic composition of manganese nodules from the Southern and Indian oceans, the global oceanic neodymium budget, and their bearing on deep ocean circulation. Geochimica Cosmochimica Acta 61, 1277–1291. doi: 10.1016/S0016-7037(96)00404-8
Anadón P., Gliozzi E., Mazzini I. (2002). Paleoenvironmental reconstruction of marginal marine environments from combined paleoecological and geochemical analyses on ostracods. Ostracoda: Appl. quaternary Res. 131, 227–247. doi: 10.1029/131GM12
Arai T., Kimura S. (2022). Spatiotemporal variability of trace elements fingerprints in otoliths of Japanese eel (Anguilla japonica) and its use in tracing geographic origin. Biology 11, 1733. doi: 10.3390/biology11121733
Avigliano E., Miller N., Volpedo A. V. (2018). Silversides (Odontesthes bonariensis) reside within freshwater and estuarine habitats, not marine environments. Estuarine Coast. Shelf Science. 205, 123–130. doi: 10.1016/j.ecss.2018.03.014
Banakar V. K., Galy A., Sukumaran N. P., Parthiban G., Volvaiker A. Y. (2003). Himalayan sedimentary pulses recorded by silicate detritus within a ferromanganese crust from the Central Indian Ocean. Earth Planetary Sci. Lett. 205 (3-4), 337–348. doi: 10.1016/S0012-821X(02)01062-2
Beck J. W., Edwards R. L., Ito E., Taylor F. W., Recy J., Rougerie F., et al. (1992). Sea-surface temperature from coral skeletal strontium/calcium ratios. Science 257, 644–647. doi: 10.1126/science.257.5070.644
Bi D., Shi X., Huang M., Yu M., Zhou T., Zhang Y., et al. (2021). Geochemical and mineralogical characteristics of deep-sea sediments from the western North Pacific Ocean: Constraints on the enrichment processes of rare earth elements. Ore Geology Rev. 138, 104318. doi: 10.1016/j.oregeorev.2021.104318
Bijvelds M. J., van der Velden J. A., Kolar Z. I., Flik G. (1998). Magnesium transport in freshwater teleosts. J. Exp. Biol. 201, 1981–1990. doi: 10.1242/jeb.201.13.1981
Bock L. R., Whitledge G. W., Pracheil B., Bailey P. (2017). Relationships between water and paddlefish Polyodon spathula dentary elemental and stable-isotopic signatures: potential application for reconstructing environmental history. J. Fish Biol. 90, 595–610. doi: 10.1111/jfb.2017.90.issue-2
Bouvier A., Vervoort J., Patchett P. (2008). The Lu-Hf and Sm-Nd isotopic composition of CHUR: Constraints from unequilibrated chondrites and implications for the bulk composition of terrestrial planets. Earth Planetary Sci. Lett. 273, 48–57. doi: 10.1016/j.epsl.2008.06.010
Chetelat B., Liu C. Q., Zhao Z. Q., Wang Q. L., Li S. L., Li J., et al. (2008). Geochemistry of the dissolved load of the Changjiang Basin rivers: anthropogenic impacts and chemical weathering. Geochimica Cosmochimica Acta 72, 4254–4277. doi: 10.1016/j.gca.2008.06.013
Das P., Iyer S. D., Kodagali V. N. (2007). Morphological characteristics and emplacement mechanism of the seamounts in the Central Indian Ocean Basin. Tectonophysics 443, 1–18. doi: 10.1016/j.tecto.2007.08.002
Deng Y., Guo Q., Liu C., He G., Cao J., Liao J., et al. (2022). Early diagenetic control on the enrichment and fractionation of rare earth elements in deep-sea sediments. Sci. Adv. 8, eabn5466. doi: 10.1126/sciadv.abn5466
Dyment J. (1993). Evolution of the Indian ocean triple junction between 65 and 49 ma (anomalies 28 to 21). J. Geophysical Research: Solid Earth. 98, 13863–13877. doi: 10.1029/93JB00438
Elderfield H., Pagett R. (1986). Rare earth elements in ichthyoliths: variations with redox conditions and depositional environment. Sci. Total Environment. 49, 175–197. doi: 10.1016/0048-9697(86)90239-1
Elderfield H., Upstill-Goddard R., Sholkovitz E. R. (1990). The rare earth elements in rivers, estuaries, and coastal seas and their significance to the composition of ocean waters. Geochimica Cosmochimica Acta 54, 971–991. doi: 10.1016/0016-7037(90)90432-K
Gourlan A. T., Meynadier L., Allègre C. J. (2008). Tectonically driven changes in the Indian Ocean circulation over the last 25 Ma: Neodymium isotope evidence. Earth Planetary Sci. Lett. 267 (1-2), 353–364. doi: 10.1016/j.epsl.2007.11.054
Grandjean P., Cappetta H., Michard A., Albarede F. (1987). The assessment of REE patterns and 143Nd/144Nd ratios in fish remains. Earth Planetary Sci. Letters. 84, 181–196. doi: 10.1016/0012-821X(87)90084-7
Hein J. R., Conrad T., Mizell K., Banakar V. K., Frey F. A., Sager W. W. (2016). Controls on ferromanganese crust composition and reconnaissance resource potential, Ninetyeast Ridge, Indian Ocean. Deep Sea Res. Part I: Oceanographic Res. Papers. 110, 1–19. doi: 10.1016/j.dsr.2015.11.006
Houedec S. L., Meynadier L., Allègre C. J. (2012). Nd isotope systematics on ODP Sites 756 and 762 sediments reveal major volcanic, oceanic and climatic changes in South Indian Ocean over the last 35 Ma. Earth Planetary Sci. Lett. 327-328, 29–38. doi: 10.1016/j.epsl.2012.01.019
Huang P., Li A., Hu N., Fu Y., Ma Z. (2006). Isotopic feature and uranium dating of the volcanic rocks in the Okinawa Trough. Sci. China Ser. D. 49, 375–383. doi: 10.1007/s11430-006-0375-8
Hughes J. M., Rakovan J. (2002). The crystal structure of apatite, Ca5(PO4)3(F, OH, Cl). Rev. mineralogy geochemistry. 48, 1–12. doi: 10.2138/rmg.2002.48.1
Hüssy K., Limburg K. E., De Pontual H., Thomas O. R., Cook P. K., Heimbrand Y., et al. (2021). Trace element patterns in otoliths: the role of biomineralization. Rev. Fisheries Sci. Aquaculture. 29, 445–477. doi: 10.1080/23308249.2020.1760204
Ingram B. L. (1995). High-resolution dating of deep-sea clays using Sr isotopes in fossil fish teeth. Earth Planetary Sci. Letters. 134, 545–555. doi: 10.1016/0012-821X(95)00151-2
Iyer S. D. (1991). Comparison of internal features and microchemistry of ferromanganese crusts from the Central Indian Basin. Geo-marine letters. 11, 44–50. doi: 10.1007/BF02431054
Iyer S. D. (2005). Evidences for incipient hydrothermal event (s) in the Central Indian Basin: a review. Acta Geologica Sinica-English Edition. 79, 77–86. doi: 10.1111/j.1755-6724.2005.tb00869.x
Iyer S. D., Mascarenhas-Pereira M. B. L., Nath B. N. (2007). Native aluminium (spherules and particles) in the Central Indian Basin sediments: Implications on the occurrence of hydrothermal events. Mar. Geology. 240, 177–184. doi: 10.1016/j.margeo.2007.02.004
Izzo C., Reis-Santos P., Gillanders B. M. (2018). Otolith chemistry does not just reflect environmental conditions: A meta-analytic evaluation. Fish Fisheries. 19, 441–454. doi: 10.1111/faf.2018.19.issue-3
Kato Y., Fujinaga K., Nakamura K., Takaya Y., Kitamura K., Ohta J., et al. (2011). Deep-sea mud in the Pacific Ocean as a potential resource for rare-earth elements. Nat. geoscience. 4, 535–539. doi: 10.1038/ngeo1185
Kon Y., Hoshino M., Sanematsu K., Morita S., Tsunematsu M., Okamoto N., et al. (2014). Geochemical characteristics of apatite in heavy REE-rich deep-sea mud from minami-torishima area, southeastern Japan. Resource Geology. 64, 47–57. doi: 10.1111/rge.2014.64.issue-1
Li D., Peng J., Chew D., Liang Y., Hollings P., Fu Y., et al. (2023). Dating rare earth element enrichment in deep-sea sediments using U-Pb geochronology of bioapatite. Geology 51, 428–433. doi: 10.1130/G50938.1
Li P., Shi W., Liu Q., Yu Y., He G., Chen L., et al. (2011). Spatial and temporal distribution patterns of chlorophyll-a and the correlation analysis with environmental factors in Lake Qiandao. J. Lake Sci. (in Chinese). 23, 568–574. doi: 10.18307/2011.0412
Li C., Zhou L., Zhao Z., Zhang Z., Zhao H., Li X., et al. (2018). In-situ Sr isotopic measurement of scheelite using fs-LA-MC-ICPMS. J. Asian Earth Sci. 160, 38–47. doi: 10.1016/j.jseaes.2018.03.025
Liao J., Chen J., Sun X., Wu Z., Deng Y., Shi X., et al. (2022). Quantifying the controlling mineral phases of rare-earth elements in deep-sea pelagic sediments. Chem. Geology. 595, 120792. doi: 10.1016/j.chemgeo.2022.120792
Liao J., Sun X., Li D., Sa R., Lu Y., Lin Z., et al. (2019a). New insights into nanostructure and geochemistry of bioapatite in REE-rich deep-sea sediments: LA-ICP-MS, TEM, and Z-contrast imaging studies. Chem. Geology. 512, 58–68. doi: 10.1016/j.chemgeo.2019.02.039
Liao J., Sun X., Wu Z., Sa R., Guan Y., Lu Y., et al. (2019b). Fe-Mn (oxyhydr) oxides as an indicator of REY enrichment in deep-sea sediments from the central North Pacific. Ore Geology Rev. 112, 103044. doi: 10.1016/j.oregeorev.2019.103044
Ling H., Jiang S., Frank M., Zhou H., Zhou F., Lu Z., et al. (2005). Differing controls over the Cenozoic Pb and Nd isotope evolution of deepwater in the central North Pacific Ocean. Earth Planetary Sci. Letters. 232, 345–361. doi: 10.1016/j.epsl.2004.12.009
Liu Z., Chen Y., Ding J., Zhang W., Wu Y., Guo F. (2003). Study on zoned characteristics and formation cause of the East China Sea submarine topography. Adv. Mar. Science. 21, 160–173.
Liu Y., Jing Y., Zhao W. (2023). Distribution of rare earth elements and implication for Ce anomalies in the clay-sized minerals of deep-sea sediment, Western Pacific Ocean. Appl. Clay Science. 235, 106876. doi: 10.1016/j.clay.2023.106876
Liu J., Xu H., Jiang Y., Wang J., He J. (2020). Mesozoic and Cenozoic shelf structure and tectonic evolution in the East China Sea Basin. Acta Petrologica Sin. (in Chinese). 94, 675–691. doi: 10.19762/j.cnki.dizhixuebao.2020115
Lu Y., Zhang X., Zhu K., Wang J., Yu M., Huang M., et al. (2022). Occurrence characteristics of rare earth elements in core sediments of the Central Indian Ocean Basin. J. Rare Earths (in Chinese). 41, 1006–1021.
Luo C., Zheng H., Tada R., Wu W., Irino T., Yang S., et al. (2014). Tracing Sr isotopic composition in space and time across the Yangtze River basin. Chem. Geology. 388, 59–70. doi: 10.1016/j.chemgeo.2014.09.007
Martin E., Haley B. (2000). Fossil fish teeth as proxies for seawater Sr and Nd isotopes. Geochimica Cosmochimica Acta 64, 835–847. doi: 10.1016/S0016-7037(99)00376-2
Martin E. E., Scher H. D. (2004). Preservation of seawater Sr and Nd isotopes in fossil fish teeth: bad news and good news. Earth Planetary Sci. Letters. 220, 25–39. doi: 10.1016/S0012-821X(04)00030-5
Martin E. E., Scher H. (2006). A Nd isotopic study of southern sourced waters and indonesian throughflow at intermediate depths in the Cenozoic Indian Ocean. Geochemistry Geophysics Geosystems 7 (9). doi: 10.1029/2006GC001302
McArthur J. M., Howarth R. J., Bailey T. R. (2001). Strontium isotope stratigraphy: LOWESS version 3: best fit to the marine Sr-isotope curve for 0–509 Ma and accompanying look-up table for deriving numerical age. J. Geology. 109, 155–170. doi: 10.1086/319243
McArthur J. M., Howarth R. J., Shields G. A., Zhou Y. (2020). Chapter 7 - Strontium isotope stratigraphy. Geologic time scale 1, 211–238.
Mi B., Liu S., Dou Y., Shi X. (2017). Sr-Nd-Pb isotopic compositions in the sediments of the middle and small rivers around the East China Sea and the implications for tracing sediment sources. Mar. Sci. Bull. (in Chinese). 36, 440–448.
Mukhopadhyay R. (1998). Post-Cretaceous intraplate volcanism in the central Indian Ocean Basin. Mar. geology. 151, 135–142. doi: 10.1016/S0025-3227(98)00073-5
Mukhopadhyay R., George P., Ranade G. R. (1997). Spreading rate dependent seafloor deformation in response to India-Eurasia collision: results of a hydrosweep survey in the Central Indian Ocean Basin. Mar. geology. 140, 219–229. doi: 10.1016/S0025-3227(97)00024-8
Nambiar R., Bhushan R., Raj H. (2022). Paleoredox conditions of bottom water in the northern Indian Ocean since 39 ka. Palaeogeography Palaeoclimatology Palaeoecology. 586, 110766. doi: 10.1016/j.palaeo.2021.110766
Nassar N. T., Du X., Graedel T. E. (2015). Criticality of the rare earth elements. J. Ind. Ecol. 19, 1044–1054. doi: 10.1111/jiec.2015.19.issue-6
Nemliher J. G., Baturin G. N., Kallaste T. E., Murdmaa I. O. (2004). Transformation of hydroxyapatite of bone phosphate from the ocean bottom during fossilization. Lithology Mineral Resources. 39, 468–479. doi: 10.1023/B:LIMI.0000040736.62014.2d
Nozaki Y., Alibo D. S. (2003). Importance of vertical geochemical processes in controlling the oceanic profiles of dissolved rare earth elements in the northeastern Indian Ocean. Earth Planetary Sci. Letters. 205, 155–172. doi: 10.1016/S0012-821X(02)01027-0
Ohta J., Yasukawa K., Nakamura K., Fujinaga K., Iijima K., Kato Y. (2021). Geological features and resource potential of deep-sea mud highly enriched in rare-earth elements in the Central Pacific Basin and the Penrhyn Basin. Ore Geology Rev. 139, 104440. doi: 10.1016/j.oregeorev.2021.104440
Palmer M. R., Edmond J. M. (1989). The strontium isotope budget of the modern ocean. Earth Planetary Sci. Letters. 92, 11–26. doi: 10.1016/0012-821X(89)90017-4
Pan Y., Fleet M. E. (2002). Compositions of the apatite-group minerals: substitution mechanisms and controlling factors. Rev. mineralogy geochemistry. 48, 13–49. doi: 10.2138/rmg.2002.48.2
Pan G., Xiao Q., Lu S., Deng J., Feng Y., Zhang K., et al. (2009). Subdivision tectonic units of China. Geology China (in Chinese). 36, 1–28.
Paton C., Hellstrom J., Paul B., Woodhead J., Hergt J. (2011). Iolite: FREEware for the visualisation and processing of mass spectrometric data. J. Analytical Atomic Spectrometry. 26, 2508–2518. doi: 10.1039/c1ja10172b
Piotrowski A. M., Banakar V. K., Scrivner A. E., Elderfield H., Galy A., Dennis A. (2009). Indian Ocean circulation and productivity during the last glacial cycle. Earth Planetary Sci. Letters. 285, 179–189. doi: 10.1016/j.epsl.2009.06.007
Piper D. Z. (1974). Rare earth elements in the sedimentary cycle: a summary. Chem. geology. 14, 285–304. doi: 10.1016/0009-2541(74)90066-7
Rajendran S., Rao T. P. (2000). Analysis of a north-south magnetic profile over the Central Indian Ocean. Curr. Sci. 78, 1378–1381. Available online at: https://www.jstor.org/stable/24104048.
Raju K. K., Ramprasad T. (1989). Magnetic lineations in the Central Indian Basin for the period A24–A21: a study in relation to the Indian Ocean Triple Junction trace. Earth Planetary Sci. Letters. 95, 395–402. doi: 10.1016/0012-821X(89)90113-1
Ren J., Jiang X., He G., Wang F., Yang T., Luo S., et al. (2022). Enrichment and sources of REY in phosphate fractions: Constraints from the leaching of REY-rich deep-sea sediments. Geochimica Cosmochimica Acta 335, 155–168. doi: 10.1016/j.gca.2022.08.035
Ren J., Liu Y., Wang F., He G., Deng X., Wei Z., et al. (2021). Mechanism and influencing factors of REY enrichment in deep-sea sediments. Minerals 11, 196. doi: 10.3390/min11020196
Sa R., Sun X., He G., Xu L., Pan Q., Liao J., et al. (2018). Enrichment of rare earth elements in siliceous sediments under slow deposition: A case study of the central North Pacific. Ore Geology Rev. 94, 12–23. doi: 10.1016/j.oregeorev.2018.01.019
Scher H. D., Martin E. E. (2006). Timing and climatic consequences of the opening of drake passage. Science 312, 428–430. doi: 10.1126/science.1120044
Shaw H. F., Wasserburg G. J. (1985). Sm-Nd in marine carbonates and phosphates: Implications for Nd isotopes in seawater and crustal ages. Geochimica Cosmochimica Acta 49, 503–518. doi: 10.1016/0016-7037(85)90042-0
Sholkovitz E. R. (1993). The geochemistry of rare earth elements in the Amazon River estuary. Geochimica Cosmochimica Acta 57, 2181–2190. doi: 10.1016/0016-7037(93)90559-F
Staudigel H., Doyle P., Zindler A. (1985). and Nd isotope systematics in fish teeth. Earth Planetary Sci. Letters. 76, 45–56. doi: 10.1016/0012-821X(85)90147-5
Subha Anand S., Rahaman W., Lathika N., Thamban M., Patil S., Mohan R. (2019). Trace elements and Sr, Nd isotope compositions of surface sediments in the Indian Ocean: an evaluation of sources and processes for sediment transport and dispersal. Geochemistry Geophysics Geosystems. 20, 3090–3112. doi: 10.1029/2019GC008332
Sun X., Fan D., Liu P., Pang Y., Tian Y. (2017). The states of Eh, Ph of water from Yangtze River Estuary and its adjacent areas and their implications. Adv. Mar. Sci. (in Chinese). 35, 96–106.
Sun X., Hu Z., Liu M., Pang Y., Fan. D. (2019). Distributions and influence factors of Eh and pH in the East China Sea in autumn. Mar. Environ. Sci. (in Chinese). 38, 211–220.
Sun Z., Liu J., Zhang Y., Song J., Xiao Y., Yuan H., et al. (2023). Compositional characteristics of sediment from Jiaozhou Bay in North China and the implication to the provenance. J. Oceanology Limnology 41, 1–13. doi: 10.1007/s00343-022-2019-z
Sun Y., Shi X., Yan Q., Liu X., Yu M., Huang M., et al. (2022). The study on geochemical characteristics and enrichment mechanism of deep sea REY-rich sediments in the Central Indian Ocean Basin. Acta Oceanologica Sin. (in Chinese). 44, 42–62.
Tanaka E., Nakamura K., Yasukawa K., Mimura K., Fujinaga K., Iijima K., et al. (2020). Chemostratigraphy of deep-sea sediments in the western North Pacific Ocean: Implications for genesis of mud highly enriched in rare-earth elements and yttrium. Ore Geology Rev. 119, 103392. doi: 10.1016/j.oregeorev.2020.103392
Tang Z., Zhang X., Zhang M., Zhou M., Tian F., Wang S., et al. (2023). Geochemical characteristics of soil elements in Xin’an River Basin: constrains from rock formation types. East China Geology (in Chinese). 44, 172–185.
Taylor S. R., McLennan S. M. (1985). The continental crust: its composition and evolution. (Oxford: Blackwell Scientific Publications).
Toyoda K., Tokonami M. (1990). Diffusion of rare-earth elements in fish teeth from deep-sea sediments. Nature 345, 607–609. doi: 10.1038/345607a0
Trueman C. N. G., Behrensmeyer A. K., Tuross N., Weiner S. (2004). Mineralogical and compositional changes in bones exposed on soil surfaces in Amboseli National Park, Kenya: Diagenetic mechanisms and the role of sediment pore fluids. J. Archaeological Science. 31, 721–739. doi: 10.1016/j.jas.2003.11.003
Tütken T., Vennemann T. W., Pfretzschner H. U. (2008). Early diagenesis of bone and tooth apatite in fluvial and marine settings: Constraints from combined oxygen isotope nitrogen and REE analysis. Palaeogeography Palaeoclimatology Palaeoecology. 266, 254–268. doi: 10.1016/j.palaeo.2008.03.037
Vanderstraeten A., Mattielli N., Laruelle G. G., Gili S., Bory A., Gabrielli P., et al. (2023). Identifying the provenance and quantifying the contribution of dust sources in EPICA Dronning Maud Land ice core (Antarctica) over the last deglaciation (7–27 kyr BP): A high-resolution, quantitative record from a new Rare Earth Element mixing model. Sci. Total Environment. 881, 163450. doi: 10.1016/j.scitotenv.2023.163450
Wang T., Dong Y., Chu F., Zhang W., Li X., Su R., et al. (2023). In situ Strontium isotope stratigraphy of fish teeth in deep-sea sediments from the western Clarion-Clipperton Fracture Zone, eastern Pacific Ocean. Chem. Geology. 636, 121624. doi: 10.1016/j.chemgeo.2023.121624
Wang F., He G., Sun X., Yang Y., Zhao T. (2016). Study on rare earth elements occurrence carriers in REY-rich deep-sea sediments in the Pacific Ocean. Acta Petrologica Sin. (in Chinese). 32, 2057–2068.
Wei Q., Wang B., Chen J., Xia C., Qu D., Xie L. (2015). Recognition on the forming-vanishing process and underlying mechanisms of the hypoxia off the Yangtze River estuary. Sci. China Earth Sci. 58, 628–648. doi: 10.1007/s11430-014-5007-0
Weng B. S., Wan R. A., Yu R. L., Hu G. R., Yan Y., Lin C. Q., et al. (2023). Characteristics and provenances of rare earth elements and Nd isotopes in surface sediments of mangrove wetlands in the Jiulong River Estuary, China. Environ. Sci. pollut. Res. 30, 73890–73898. doi: 10.1007/s11356-023-27558-1
Wright J., Schrader H., Holser W. T. (1987). Paleoredox variations in ancient oceans recorded by rare earth elements in fossil apatite. Geochimica Cosmochimica Acta 51, 631–644. doi: 10.1016/0016-7037(87)90075-5
Wright J., Seymour R. S., Shaw H. F. (1984). REE and Nd isotopes in conodont apatite: variations with geological age and depositional environment. Conodont biofacies provincialism. 196, 325–340. doi: 10.1130/SPE196
Yang Y., Wu F., Yang J., Chew D., Xie L., Chu Z., et al. (2014). and Nd isotopic compositions of apatite reference materials used in U–Th–Pb geochronology. Chem. Geology. 385, 35–55. doi: 10.1016/j.chemgeo.2014.07.012
Yasukawa K., Liu H., Fujinaga K., Machida S., Haraguchi S., Ishii T., et al. (2014). Geochemistry and mineralogy of REY-rich mud in the eastern Indian Ocean. J. Asian Earth Sci. 93, 25–36. doi: 10.1016/j.jseaes.2014.07.005
Zeng J., Long S., Bao M., Li Y., Li Y. (2012). Analysis of dominant ingredients and trace elements of offshore sea water in Yangshan, Shanghai. Mar. Environ. Sci. (in Chinese) 31, 186–189+200.
Zhang X., Lu Y., Miao Y., Huang M., Zhu K., Cai L., et al. (2023). Radiogenic Nd in bioapatite from rare earth elements rich deep sea sediments from Central Indian Oceanic Basin and its implication in material sources. Ore Geology Rev. 154, 105295. doi: 10.1016/j.oregeorev.2023.105295
Zhang X., Tao C., Shi X., Li H., Huang M., Huang D. (2017). Geochemical characteristics of REY-rich pelagic sediments from the GC02 in central Indian Ocean Basin. J. Rare Earths 35, 1047–1058. doi: 10.1016/S1002-0721(17)61012-3
Zhao L., Chen Z. Q., Algeo T. J., Chen J., Chen Y., Tong J., et al. (2013). Rare-earth element patterns in conodont albid crowns: evidence for massive inputs of volcanic ash during the latest Permian biocrisis? Global Planetary Change. 105, 135–151. doi: 10.1016/j.gloplacha.2012.09.001
Keywords: fossil and modern fish teeth, Central Indian Ocean Basin, trace elements enrichment process, 87Sr/86Sr-143Nd/144Nd, geochemical indicative significance, paleoceanography
Citation: Xu Z, Zhang X, Zhu K, Huang M, Yu M, Li Z, Qiu Z and Lu Y (2025) Comparison of elemental composition and 87Sr/86Sr-143Nd/144Nd between fossil and modern fish teeth and the significance of the enrichment of REE in deep-sea sediments. Front. Mar. Sci. 12:1534727. doi: 10.3389/fmars.2025.1534727
Received: 26 November 2024; Accepted: 11 February 2025;
Published: 07 April 2025.
Edited by:
Zhongxian Zhao, Chinese Academy of Sciences (CAS), ChinaReviewed by:
Jiangbo Ren, Guangzhou Marine Geological Survey, ChinaCopyright © 2025 Xu, Zhang, Zhu, Huang, Yu, Li, Qiu and Lu. This is an open-access article distributed under the terms of the Creative Commons Attribution License (CC BY). The use, distribution or reproduction in other forums is permitted, provided the original author(s) and the copyright owner(s) are credited and that the original publication in this journal is cited, in accordance with accepted academic practice. No use, distribution or reproduction is permitted which does not comply with these terms.
*Correspondence: Xiaoyu Zhang, emhhbmdfeGlhb3l1QHpqdS5lZHUuY24=
Disclaimer: All claims expressed in this article are solely those of the authors and do not necessarily represent those of their affiliated organizations, or those of the publisher, the editors and the reviewers. Any product that may be evaluated in this article or claim that may be made by its manufacturer is not guaranteed or endorsed by the publisher.
Research integrity at Frontiers
Learn more about the work of our research integrity team to safeguard the quality of each article we publish.