- 1Posgrado en Ciencias del Mar y Limnología, Universidad Nacional Autónoma de México, Mexico City, Mexico
- 2MARUM - Center for Marine Environmental Sciences, University of Bremen, Bremen, Germany
- 3Department of Earth, Ocean & Atmospheric Science, Florida State University, Tallahassee, FL, United States
- 4Laboratorio de Biodiversidad y Macroecología, Instituto de Ciencias del Mar y Limnología, Universidad Nacional Autónoma de México, Mexico City, Mexico
The Chapopote Knoll at 3200 m depth, in the southern Gulf of Mexico harbors highly diverse benthic habitats, including massive asphalt flows and surficial gas hydrates with gas seepage. Its associated benthic megafauna includes endemic cold-seep species and background species. This study describes the benthic habitat preferences, distribution patterns and diets of three crustacean species, the caridean shrimp Alvinocaris muricola and the galatheids Munidopsis geyeri and M. exuta. High-resolution imaging recorded eight habitats and helped depict their spatial distributions. A. muricola aggregates on Siboglinidae clusters and in gas seepage sites. M. geyeri and M. exuta are less selective and occur in almost all habitats. The carbon (δ13C) and nitrogen (δ15N) values of A. muricola show a nutritional preference of bacteria from mats and water column detritus retained among the Sibolindiae, whereas the two Munidopsis species have wider spectrum diets. Gut content analysis in all three species, validate the stable isotope values, food sources and confirm the secondary consumer’s trophic level. This study recognizes coexistence of A. muricola and the two Munidopsis species in the benthic habitats while using different resources. Compound specific isotope analyses of galatheid guts revealed females to have more 13C-depleted lipids (-35‰) compared to males (-28‰), calling for more detailed analyses to clarify this trophic segregation.
1 Introduction
The Chapopote Knoll is an asphalt volcano with diverse cold seep habitats occurring at 2914-3300 m depth (MacDonald et al., 2004; Sahling et al., 2016). Similar asphalt accumulations have been reported from other settings: Santa Barbara basin at 185-210 m depth (Valentine et al., 2010); the Angola margin asphalt mounds at 1350-2150 m depth (Jones et al., 2014); North São Paulo Plateau at 2652–2752 m depth (Fujikura et al., 2017) and from Northern Gulf of Mexico at 975-1524 m (Puma site; Williamson et al., 2008), 1264-1365 m (Shenzi site; Weiland et al., 2008), 1150 m (Henderson site; NOAA, 2017), and 1925 m (Tar Lily sites; MacDonald, 2014). The Chapopote Knoll remains the deepest site and is distinguished by its dynamic geologic and geochemical processes that contribute to its biodiversity (Marcon et al., 2018; MacDonald et al., 2020). The chemosynthetic fauna is dominated by Siboglinidae tubeworms (Escarpia spp.), that occur among fissures in, or protruding from below the asphalt and authigenic carbonates crusts associated with gas hydrate mounds (MacDonald et al., 2004; Sahling et al., 2016). Modiolid bivalves (Bathymodiolus heckerae and B. brooksi) are less abundant and concentrate at methane venting soft sediment habitats (Raggi et al., 2012). Crustaceans include small peracarids that live among the Siboglinidae and on the asphalt, decapods that are dispersed over the Chapopote asphalt spill area and the neighboring background soft sediment habitat (MacDonald et al., 2004, 2020). Other fauna associated with asphalts and exposed gas hydrates includes snails, sponges, and hydroids. The microbial community is supported by the aerobic and anaerobic oxidation of hydrocarbons in association with asphalt deposits and gas hydrate (Naehr et al., 2009; Schubotz et al., 2011a, b; Raggi et al., 2012; Rubin-Blum et al., 2017; Wegener et al., 2020). The free-living microorganisms provide an important source of primary production to background and seep associated biota.
Digital images and video provide resolution to identify the faunal components and delineate their habitats. Mosaics of multiple images can increase the visual coverage obtained (Ludvigsen et al., 2007) and help understand the spatial distribution, associations among species and relationship to the cold seep habitats including potential food sources (Sibuet and Olu-Le Roy, 2002; Olu et al., 2009). These direct observations of the organisms in their ecosystem have facilitated the study of the deep-sea benthos thereby producing an integrated view of the ecosystem. The influence of fluid venting patterns and geological features define the community structure of cold seeps and dominant endemic megafauna that varies among sites (Olu et al., 2009). These criteria have been used to classify cold seeps into large field sites with conspicuous biological activity where dense clusters of endemic fauna aggregate (>1600 ind.m-2); large areas with conspicuous biological activity where fauna occurs in dispersed clusters; and sites of relatively low biological activity with dispersed organisms (Sibuet and Olu-Le Roy, 2002). Carney (1994) classified the cold seep megabenthic resident community into endemic (chemosynthesis-based organisms), colonist and vagrant. The latter are background visiting fauna that benefit from the seepage microorganisms, mats and biota. Among the decapod Crustacea that occur associated to methane seepage is the caridean shrimp Alvinocaris muricola that has been recorded as an endemic component, and the Munidopsis squat lobsters referred to as colonists (Olu et al., 2009). Both species have amphi-Atlantic distribution (Komai and Segonzac, 2005; Macpherson and Segonzac, 2005; Ramirez-Llodra and Segonzac, 2006; Olu et al., 2010; Pereira et al., 2020) and coexist in the Chapopote Knoll habitats (shown in Supplementary Material). In the Regab site in the Gulf of Guinea, Komai and Segonzac (2005) described that A. muricola feeds from protists fragments on dark mucus, and fine mineral particles. Munidopsis geyeri’s diet is sediment and faunal fragments as recorded by Macpherson and Segonzac (2005).
Traits associated with niche differences, presented as trade-offs among species on a local scale, include differential use of resources, susceptibility to predators, use of the abiotic environment and response to disturbance or stress (Kneitel and Chase, 2004). In particular, research of cold seep fauna diet, based on gut content, stable isotopes and fatty acids, has recorded different intake of chemosynthetic carbon and has related it to the use of the habitat in which it occurs (Olu et al., 2009; Fujikura et al., 2017). This study describes the occurrence and diet of A. muricola, M. geyeri and M. exuta on the Chapopote Knoll and contributes with new knowledge to an understanding of the interaction between species with differentiated life history and use of the cold seep habitats.
1.1 Study area
The Chapopote Knoll (meaning “tar” in Nahuatl language) is located in the Campeche Bay sub-province of the Gulf of Mexico (Figure 1A) (CONACyT, 1982). The regional bathymetry is characterized by knolls and ridges. A “craterlike graben near the crest of the structure” (MacDonald et al., 2004) characterizes the “asphalt volcano”, together with the visual similarities of the asphalt discharges to a´a or pa´hoehoe basaltic lava flows (Brüning et al., 2010). This site is 5-10 km in diameter and rises 350-800 m above the surrounding lower bathyal and abyssal plain at a depth of 3000 m below sea level. The lava-like flows of solidified asphalt on Chapopote cover more than 1 km2 of the rim. The main asphalt deposits occurred at the south-western border of the central crater-like depression (MacDonald et al., 2004; Brüning et al., 2010). Recent research on Chapopote has yielded information on the nature of asphalt seepage as a secondary result of salt tectonism, on general physicochemical features of Chapopote, and on petroleum hydrocarbon degradation (Ding et al., 2008; Brüning et al., 2010; Schubotz et al., 2011a, b; Sahling et al., 2016; Smrzka et al., 2016; Wegener et al., 2020). The bottom water that enters the Gulf of Mexico via the Caribbean Sea is North Atlantic Deep Water (NADW, 34.97‰, 4.36°C, and 5ml L-1) (Vidal et al., 1994; Rivas et al., 2005) with a suggested residence time of about 250 yr (Rivas et al., 2005). The Sigsbee Abyssal Gyre (SAG) seems to be a persistent pattern of deep circulation (average speed: 5 cm s-1; Pérez-Brunius et al., 2018).
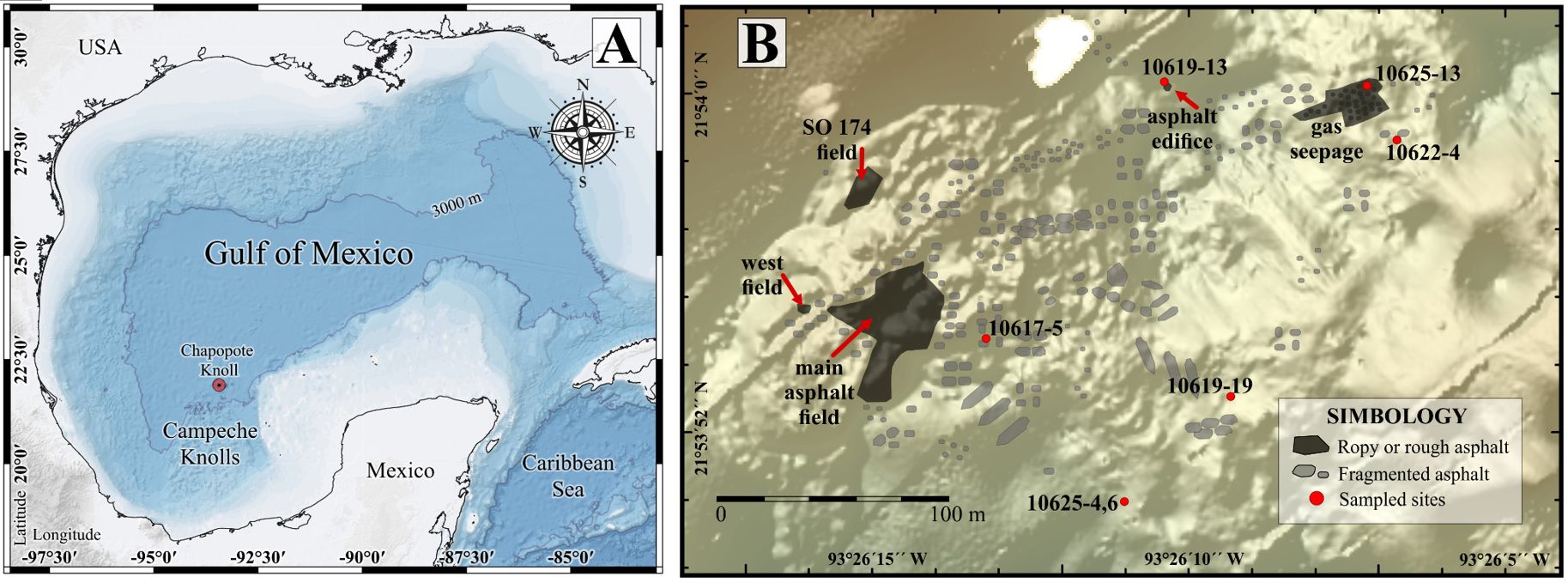
Figure 1. Study area and sample locations on the Chapopote asphalt volcano. (A) The Gulf of Mexico (base map from UNINMAR, 2011), (B) Insert of the Campeche Knolls within the Southwestern sector (modified from Brüning et al., 2010; Sahling et al., 2016).
2 Material and methods
The Chapopote Knoll was explored during the GeoB M67/2b cruise “Fluid seepage of Chile and in the Gulf of Mexico” on board the R/V Meteor in April 2006. In an initial phase, gas signatures in the water column, swath-mapping with the MBES Kongsberg 2040 with the AUV SEAL 5000 at an altitude of 80 m and combined with bathymetry data collected during two earlier cruises to the Campeche Knolls, and AUV surveys were used to localize active seeps and to generate high resolution bathymetric and backscatter maps. Swath mapping generates a two-dimensional area or map of the seafloor resulting from a deep- water multibeam echosounder that provide information on water depth, the roughness and smoothness of the sea floor. Areas of interest were explored with the ROV QUEST that provided the video and high-resolution images from the sea floor, assisted with the collection of organisms with scavenger traps and suction sampler (Table 1; Figure 1B).
2.1 Habitat description: image and mosaic analysis
The video recorded by the ROV was converted into a continuous image mosaic with the programs Adobe Premiere Pro, Adelie GIS (Arc View) and Adelie Video (VCR remote controller). This method is not replicable with new higher resolution strategies used but can complement those used in the Campeche Knolls. The Chapopote habitats recognized in 500 m2 were named following the terminology and geological classification of Brüning et al. (2010) and Fink and Fletcher (1978) and compared to other cold seep sites (Sibuet and Olu-Le Roy, 2002; Ondréas et al., 2005) standardizing the habitat terminology.
2.2 Spatial distribution
The abundance of A. muricola, M. geyeri and M. exuta was estimated from the video images and the mosaics. The species M. geyeri and M. exuta were subsumed into Munidopsis spp. due to the limitations imposed by the scale of the images and impossibility to identify the two species. The relative species abundance (total number of individuals divided by the number of analyzed images in an area of 500 m2 were reported as mean values (μ) and the ratio of variance (s2). The species spatial distribution was classified in the two-dimensional space and named as random (μ=s2), uniform (μ>s2) and aggregated or clustered (μ<s2) (Zar, 2010). The Correspondence Analysis (CA) was used to test the affinity of species to each habitat (Legendre and Legendre, 1998), and computed using the ‘FactorMineR’ library on the R statistical language and RStudio (R Core Team, 2001–2022).
2.3 Potential and ingested diet: gut content, stable isotope measurements and lipid analyses
Qualitative analysis of gut contents was based on samples observed under a stereoscopic microscope (10x magnification). The gut contents from five caridean shrimp and 17 galatheid crabs was recorded as total number of items, relative abundance, mean and standard deviation (μ±σ), from smear preparations in ten subsamples prepared per sample and observed with optical microscopy. A total of 210 fields (4 cm2 area) was observed, 50 fields in the five A. muricola caridean shrimps, 150 fields in the 16 M. geyeri and 10 fields in one M. exuta galatheid crabs.
The dietary preferences of the caridean shrimps and galatheid crabs were assessed in tissue and gut content by using 13C/12C and 15N/14N stable isotopic composition (Peterson and Fry, 1987). Tissue fragments were processed for elemental analysis: freeze-dried and acidified with HCl 0.1 N solution and subsequently washed with deionized water and freeze-dried before analysis, and processed in a gas chromatography (NA 2500) isotope-ratio mass spectrometer (Finnigan Delta plusXL), with values expressed in the delta notation as ‰ difference from a standard. The standard reference used for δ15N was atmospheric nitrogen and the standard reference for carbon was the Vienna PeeDee Belemnite Standard with an assigned value of 0.0‰. Changes in trophic levels were identified by the 1‰ and 3.4‰ decrease from the original isotope values, for δ13C and δ15N, respectively (McCutchan et al., 2003).
For lipid analyses, the entire gut contents of six individuals assigned to M. geyeri and two M. exuta was solvent-extracted using a modified Bligh and Dyer method following protocols described in Sturt et al. (2004). Soft tissue samples were freeze-dried and homogenized prior to dispersion in 10 ml of a solvent mixture of DCM: MeOH:buffer (1:2:0.8; v/v) and ultrasonically extracted for 10 minutes in four steps. For the first two extraction steps, a phosphate buffer was used (pH 7.4), for the last two steps the phosphate buffer was replaced by a TCA buffer (50 g/L, pH 2). Separation of the total lipid extract into free fatty acids and intact polar lipid-bound fatty acids and monoalkyl glycerolethers (MAGE) was achieved by preparative LC-MS using an Intersil Diol column (5 um, 10 x 150 mm) after Zhu et al. (2013). Subsequently, “free” and “bound” lipids were derivatized with 2.5% methanolic HCl at 70°C for 12 hrs. yielding fatty acid methyl esters (FAMEs) and bis-(trimethylsilyl) trifluoroacetamide (BSTFA, Merck, Germany) in pyridine at 70°C for 1 h yielding trimethylsilyl-(TMS)-derivatives of alcohols and ether lipids. Analysis of FAMEs and TMS-derivatives was performed by gas chromatography (GC) coupled to mass spectrometry (MS) as described previously (Schubotz et al., 2011a). Compound-specific stable carbon isotopic compositions were measured on a ThermoFinningan GC coupled to a ThermoFinnigan Deltaplus XP isotope ratio MS. The isotopic compositions of the TMS-derivatives were corrected for the additional methyl groups introduced during derivatization. The standard deviation of replicated analysis was <1‰. All isotopic values are reported in the delta notation (δ13C PDB).
3 Results
3.1 Habitat description: image and mosaic analysis
In total, 319 images and 23 mosaics were analyzed. The Chapopote Knoll study site was represented by eight habitats (Table 2; Figure 2), describing asphalt flow features, Siboglinidae clusters, gas and hydrocarbon seepages in which biota aggregate. The Siboglinidae aggregate densities of more than 50 individuals per cluster, forming a habitat for smaller species. These conspicuous clusters are restricted to the methane seepage. Megafauna that was identified associated to the seepage includes Porifera Hymedesmia (Stylopus) methanophila Rubin-Blum et al., 2019 and Acarnidae Iophon methanophila Rubin-Blum et al., 2019, Mollusca Bivalvia Bathymodiolus brooksi Gustafson et al., 1998 and Bathymodiolus heckerae Gustafson et al., 1998; Mollusca Gastropoda Fucaria Warén and Bouchet, 1993; Provanna sculpta Warén & Ponder, 1991 and Provanna cf chevalieri Warén and Bouchet, 2009; Mollusca Lepetellida Lepetodrilus shannonae Warén and Bouchet, 2009; Annelida Polychaeta Siboglinidae Escarpia laminata Jones, 1985; Malacostraca Decapoda Alvinocarididae Alvinocaris muricola Williams, 1988; Munidopsidae Munidopsis exuta Macpherson and Segonzac, 2005 and Munidopsis geyeri Pequegnat and Pequegnat, 1970; Echinodermata Holothuroidea Chiridota heheva Pawson and Vance, 2004, Ophiuroidea Ophioctenella acies Tyler et al., 1995; Chordata Zoarcidae Pachycara Zugmayer, 1911.
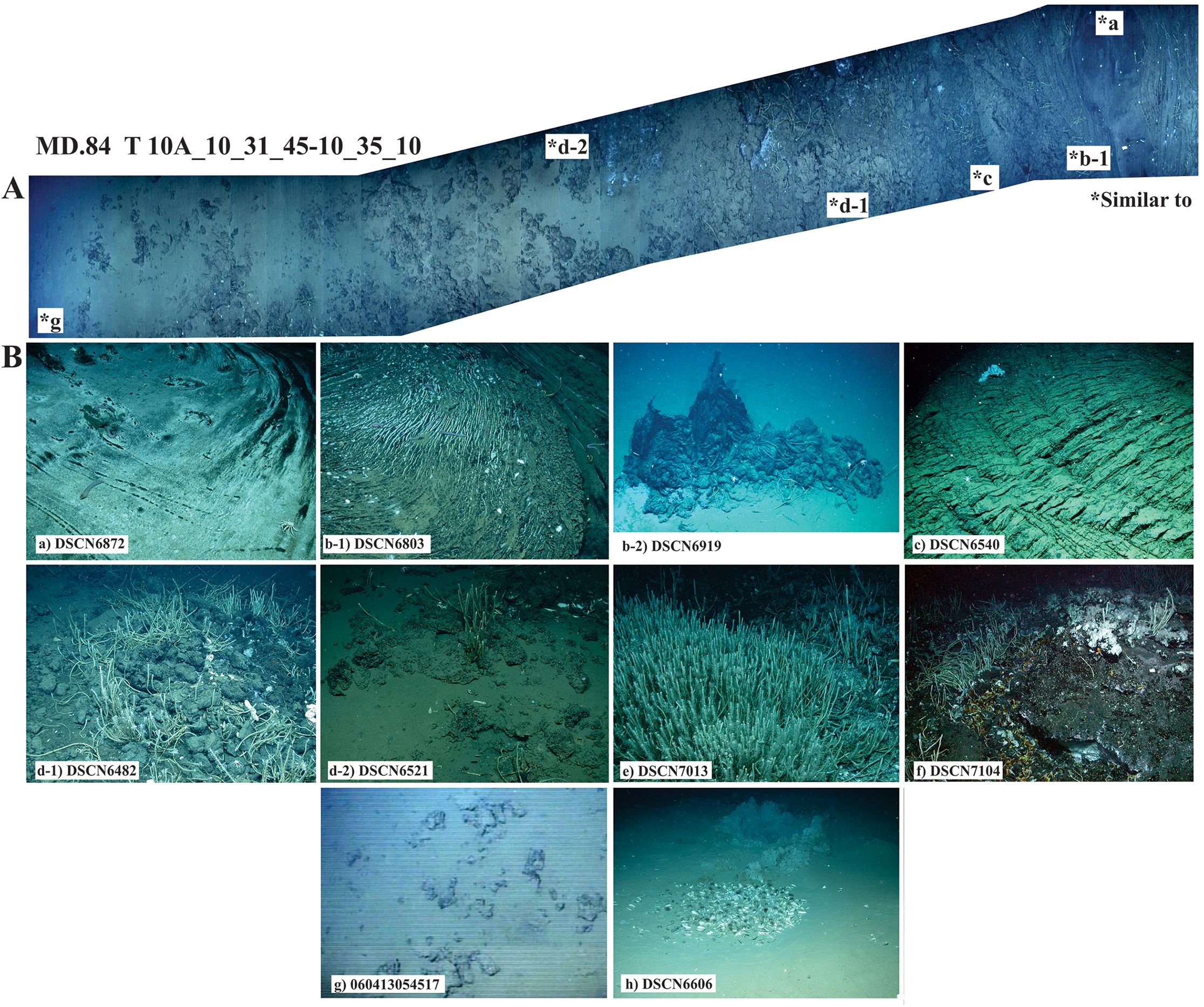
Figure 2. Habitats recognized on the Chapopote asphalt volcano. (A) mosaic showing the proximity of five different habitats. (B) a) Continuous asphalt flow, b-1) Ropy asphalt flow and b-2) as edifice, c) Rough asphalt flow, d-1) Breccia (less sediment covering), d-2) Breccia (more sediment covering), e) Siboglinidae clusters, f) Gas seepage, g) Asphalt fragments, h) Hydrocarbon seepage on soft sediment. “MD.84 T10A_10_31_45-10_35_10” number corresponds to video identification; DSCN= Digital Still Camera – Nikon, this is followed by the sequential number that identifies the image in the cruise and dive. All photos MARUM Copyright.
3.2 Spatial distribution
The analysis of 319 images recognized a total of 648 specimens of the shrimp A. muricola and 1594 of Munidopsis spp. (M. geyeri and M. exuta) galatheids (Table 3). Abundances differed significantly between the shrimp and galatheid crabs (T test: t=-6.11; df=318, p=<0.0001). A. muricola was recorded in five habitats, with highest abundance on the gas seepage and Siboglinidae clusters (Table 3). In habitats with isolated tube worms (e.g. continuous along the asphalt flows), A. muricola cling to their tubes, often near the tube opening. Abundance differed between habitats (ANOVA F(7,311)=25.85, p<0.001, n=319). In general A. muricola showed an aggregated distribution (26.02<1,731.33; μ<s2).
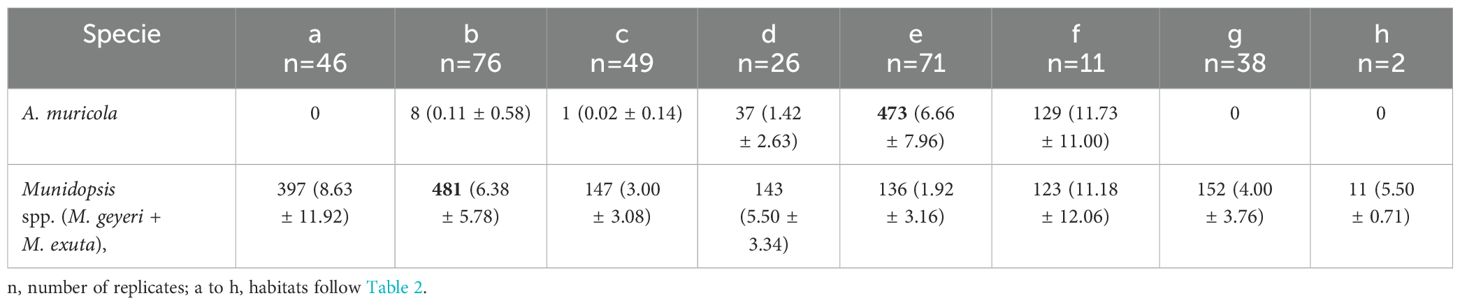
Table 3. Abundances of A. muricola and Munidopsis spp. (M. geyeri + M. exuta), values reported as mean and standard deviation (μ ±σ); higher values in bold.
The Munidopsis group (M. geyeri and M. exuta) was recorded in all eight habitats, abundance differed among habitats being highest on the continuous and ropy asphalt flow habitats (ANOVA F(7,311)=7.53, p<0.001, n=319). The Munidopsis group (M. geyeri and M. exuta) also showed an aggregated distribution (8.07<66.20; μ<s2). Correspondence analysis placed the two genera on the habitat centroids (Figure 3) and supports a dependence on variables (X2 = 30.1685; p=0.0072) showing the association between A. muricola and habitats with Siboglinidae clusters and gas seepage, whereas the Munidopsis group (M. geyeri and M. exuta) was closely associated with the continuous and ropy asphalt flows, and the breccia habitats (Figure 3).
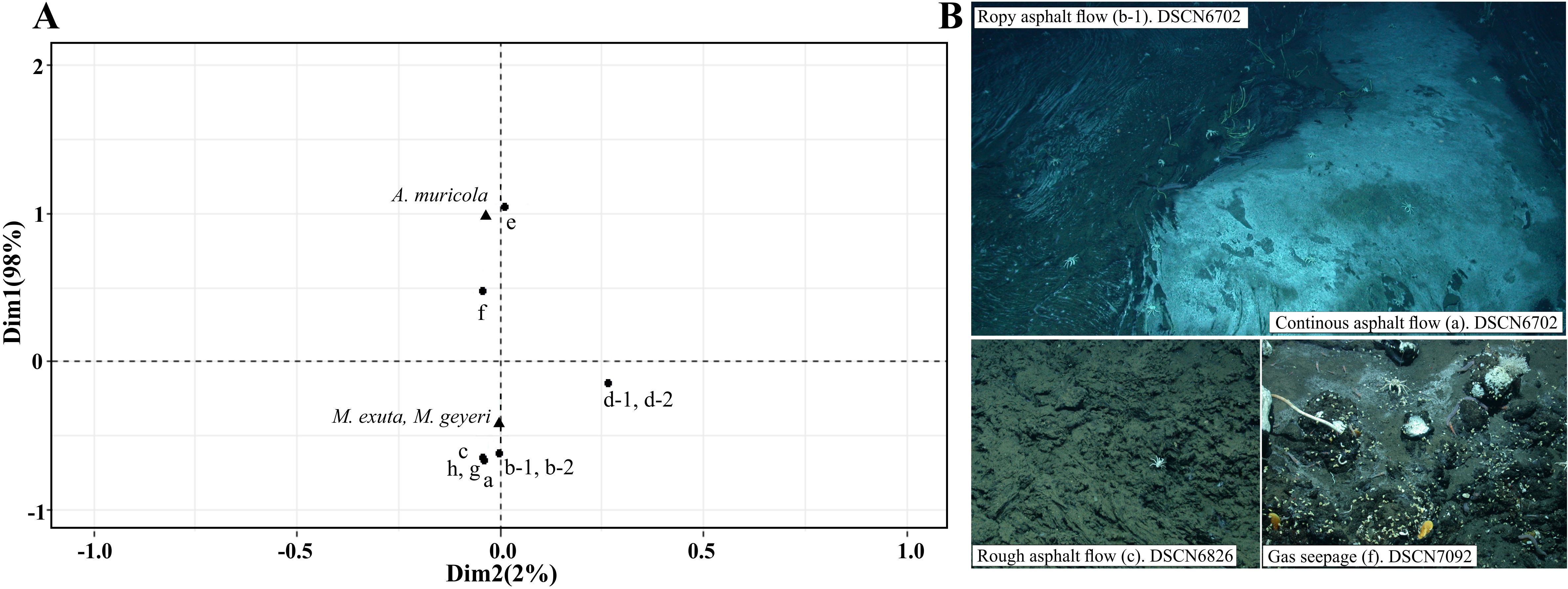
Figure 3. (A) Correspondence analysis (CA) for A. muricola and the two Munidopsis species on Chapopote, circles represent habitats: a) Continuous asphalt flow, b-1) Ropy asphalt flow and b-2) as edifice, c) Rough asphalt flow, d-1) Breccia (less sediment covering), d-2) Breccia (more sediment covering), e) Siboglinidae clusters, f) Gas seepage, g) Asphalt fragments, h) Hydrocarbon seepage on soft sediment. Markers. Circles are the benthic habitats; triangles are the species. Axes are the first two orthogonal components reproducing the distances or dimensions between the habitats and species. Dim= Dimension. (B) Graphic examples of distribution patterns on 4 habitats. DSCN= Digital Still Camera – Nikon, this is followed by the sequential number that identifies the image in the cruise and dive. All photos MARUM Copyright.
3.3 Potential and ingested diet: gut content, stable isotope, and lipid analyses
The gut content in all three species recognized twelve types of items (Figure 4). The gut contents included detritus (light brown, brown and black Figures 4A–C), filamentous material (Figure 4D), foraminifera (Figure 4E), phytodetritus (Figure 4F), diatoms (Figure 4G) and sponge ectodermal tylotes type spicules (Figure 4H). Crustacean fragments (Figure 4I) and nematodes (Figure 4J) were recognized exclusively in galatheids. Annelids (Figure 4K) and soft-walled foraminifera (Figure 4L) occurred in M. geyeri but were not registered in M. exuta.
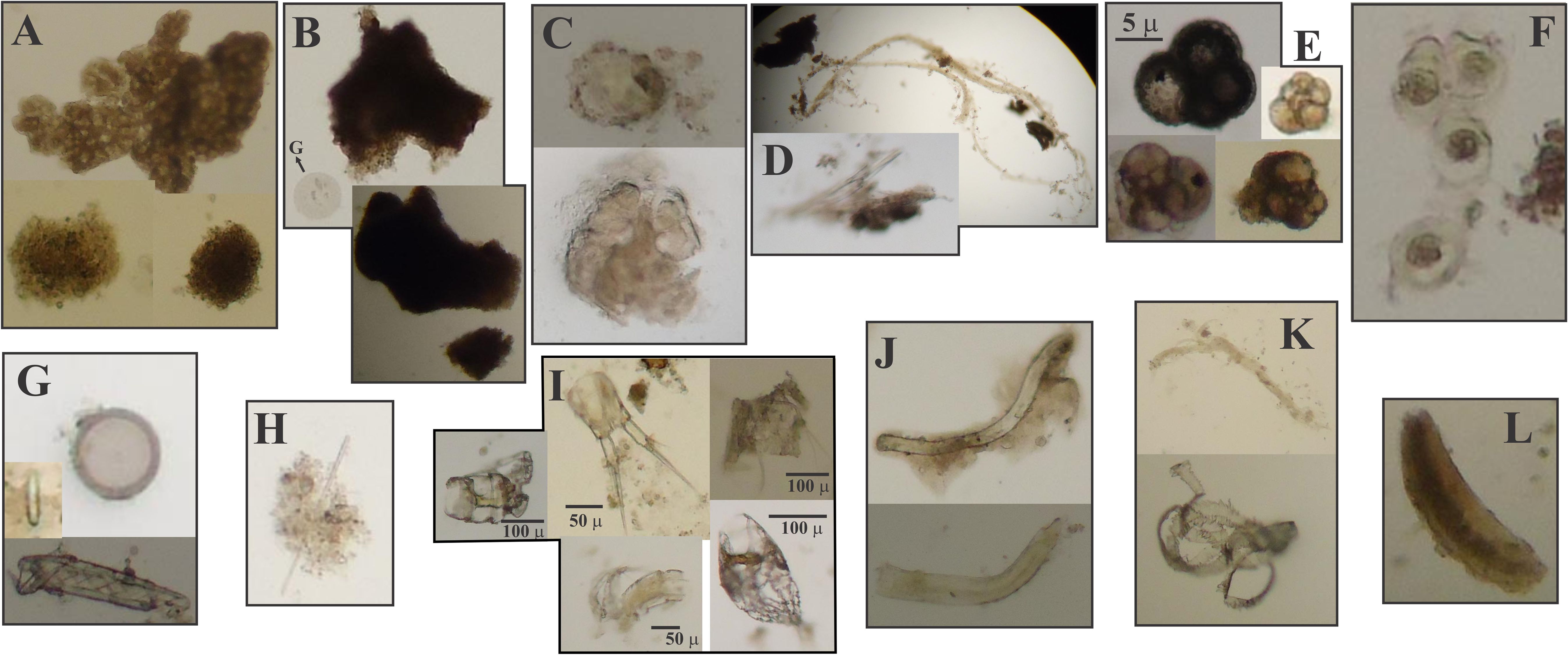
Figure 4. Items recorded in gut contents of Alvinocaris muricola and Munidopsis spp., optical microscopy images: Detritus (light brown, brown and black (A–C), filamentous material (D), foraminifera (E), phytodetritus (F), diatoms (G) and spicules (H). Crustacean fragments (I) and nematodes (J), annelids (K) soft-walled foraminifera (L).
Detritus aggregates were the most abundant item. The objects recorded in the total of sample preparations reviewed on optical microscope include brown detritus aggregates with relative abundances of 18.6 ± 7.3 (μ±σ) in A. muricola (n=50), 34.0 ± 8.2 in M. geyeri (n=150), and 45 in M. exuta (n=10) (Figure 5). Black aggregates with 46.4 ± 4.0 in A. muricola (n=50), 36.3 ± 8.5 in M. geyeri (n=150), and 34 in M. exuta (n=10); and light brown aggregates with 13.2 ± 3.7 in A. muricola (n=50), 13.3 ± 6.6 in M. geyeri (n=150), and 5.8 in M. exuta (n=10) (Figure 5). The relative abundances of filamentous materials were 7.8 ± 2.0 in A. muricola, 9.4 ± 4.1 in M. geyeri (n=150), and 8.1 in M. exuta (n=10) (Figure 5). The percentage of phytodetritus in A. muricola (9.5 ± 3.9) was higher than in the galatheid crabs (M. geyeri: 1.4 ± 1.2, M. exuta: 1.9; Figure 5). Standard deviation data are not available for M. exuta as the data are from observation of 10 fields in one sample.
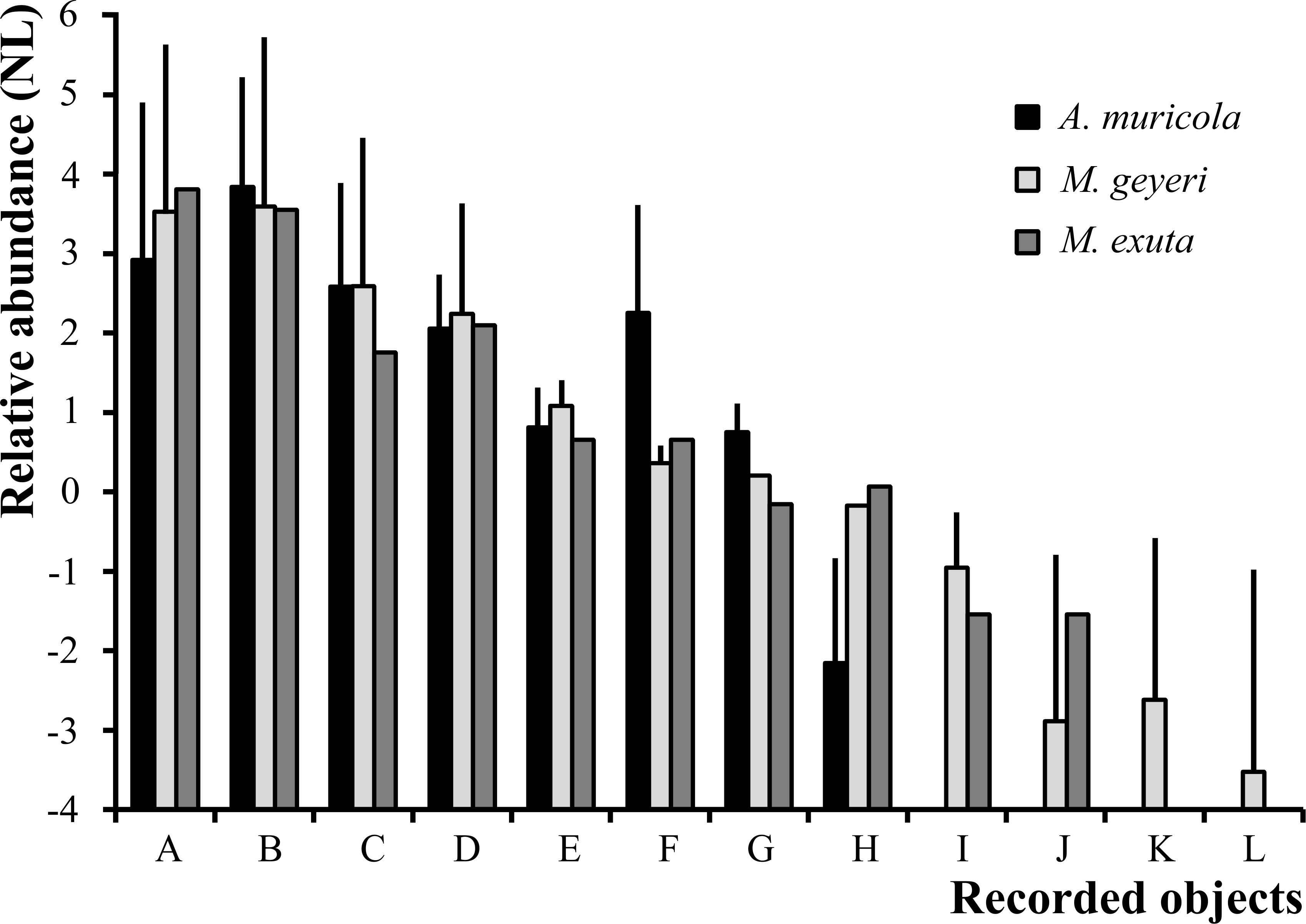
Figure 5. Relative abundance (Y axis: relative abundance, values in natural logarithm (NL), ln scale) of items recorded in gastric contents of A. muricola (n = 50), M. geyeri (n = 150) and M. exuta (n = 10); letters on the X-axis correspond to those of the gut content optical microscopy images detritus (light brown, brown and black (A–C), filamentous material (D), foraminifera (E), phytodetritus (F), diatoms (G) and spicules (H). Crustacean fragments (I) and nematodes (J), annelids (K) soft-walled foraminifera (L). The bars are standard deviations. Standard deviation data are not available for M. exuta as the data are from observation of 10 fields in one sample.
The bulk δ13C‰ values differed between species (ANOVA F(2,16)=8.48 p=0.003; Table 4). The carbon isotopic signature of muscle tissue for A. muricola had a range that included photo- and chemo-autotrophic carbon sources (-34.73 to -23.94). Depleted isotope values in the two galatheid species indicate a potential diet that includes biota from cold seeps (-41.13 to -28.25). The δ13C‰ values of the gut contents in M. exuta were slightly enriched (Table 4, Figure 6). The δ15N‰ values in muscle tissue did not differ between species (ANOVA F(2,16)=1.04 p=0.38; Table 4), both have the same trophic level and are with a range of 6.17 ± 0.96 and 6.99 ± 1.27‰. The δ15N‰ values in the gut contents of M. geyeri were slightly enriched.
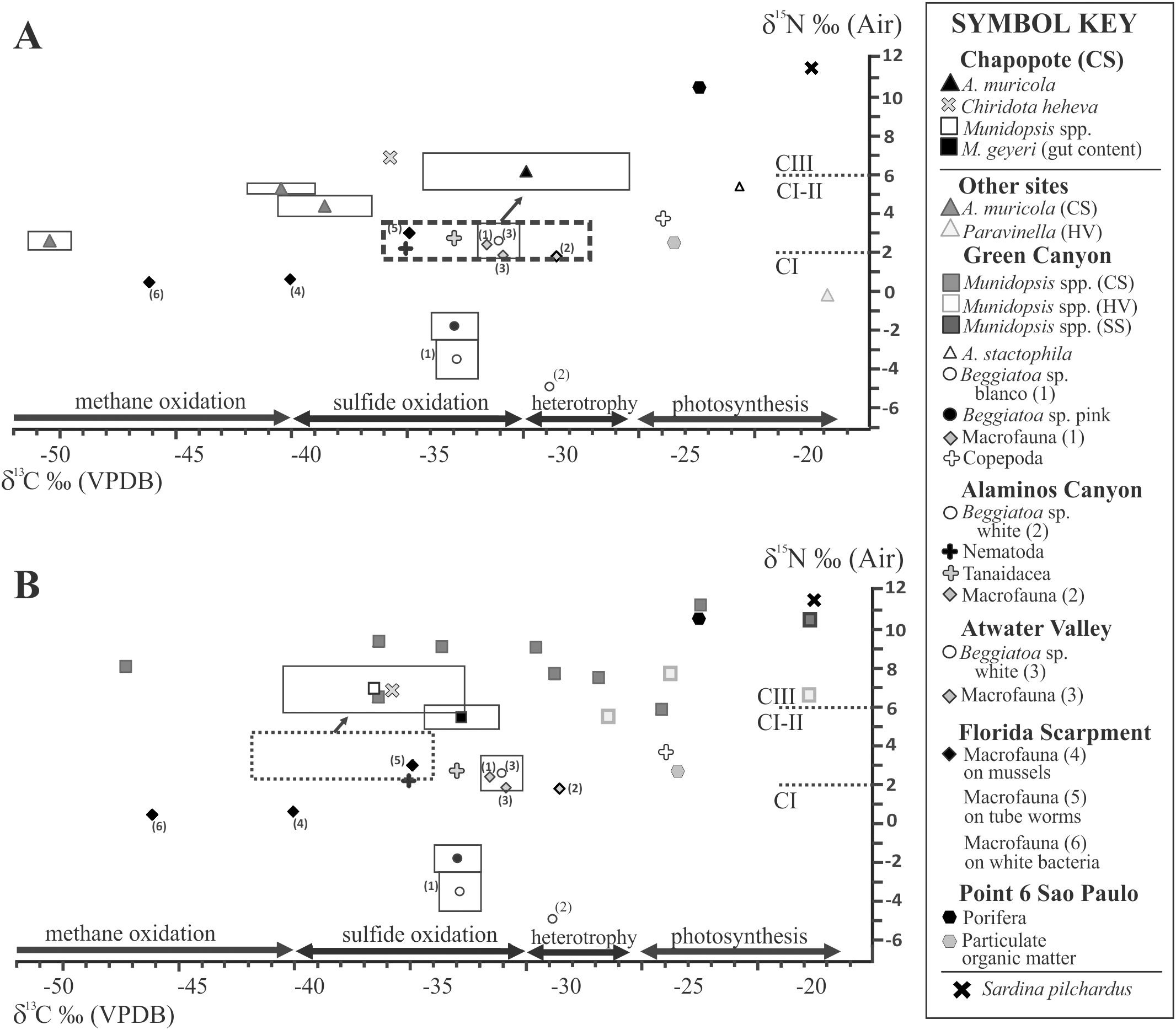
Figure 6. Values of δ13C vs. δ15N for A. muricola (A), Munidopsis spp. (M. geyeri and M. exuta) (B) from Chapopote Knoll and data from other ecosystems (Bode et al., 2004; Demopoulos et al., 2010; Fujikura et al., 2017; Levin and Mendoza, 2007; MacAvoy et al., 2005; Olu et al., 2009; Van Dover and Fry, 1994; Velázquez Luna, 2009). Rectangles represent mean and standard deviation. The potential diet is denoted by a dotted rectangle with an arrow. VPDB: Vienna Pee Dee Belemnite (calibration standard), CS: cold seep; HV: hydrothermal vent; SS: soft sediment.
Analysis of the composition and stable carbon isotopes of free fatty acids in the gut contents of M. geyeri (n=6) and M. exuta (n=2) showed low amounts of detected free fatty acids characteristic of bacteria, such as C15:0, C16:1ω5, C17:1 and cyclo-C19:0 in the gut content. These bacterial fatty acids were often most depleted in 13C (up to -58.6‰) and indicate a partly chemoautotrophic food source for female Munidopsis (Tables 5, 6). In contrast, male individuals contained high amounts of fatty acids enriched in 13C, which can be assigned to planktonic debris, such as C16:0, C18:0 and C20:5 with δ13C values ranging from -27 to -24‰.
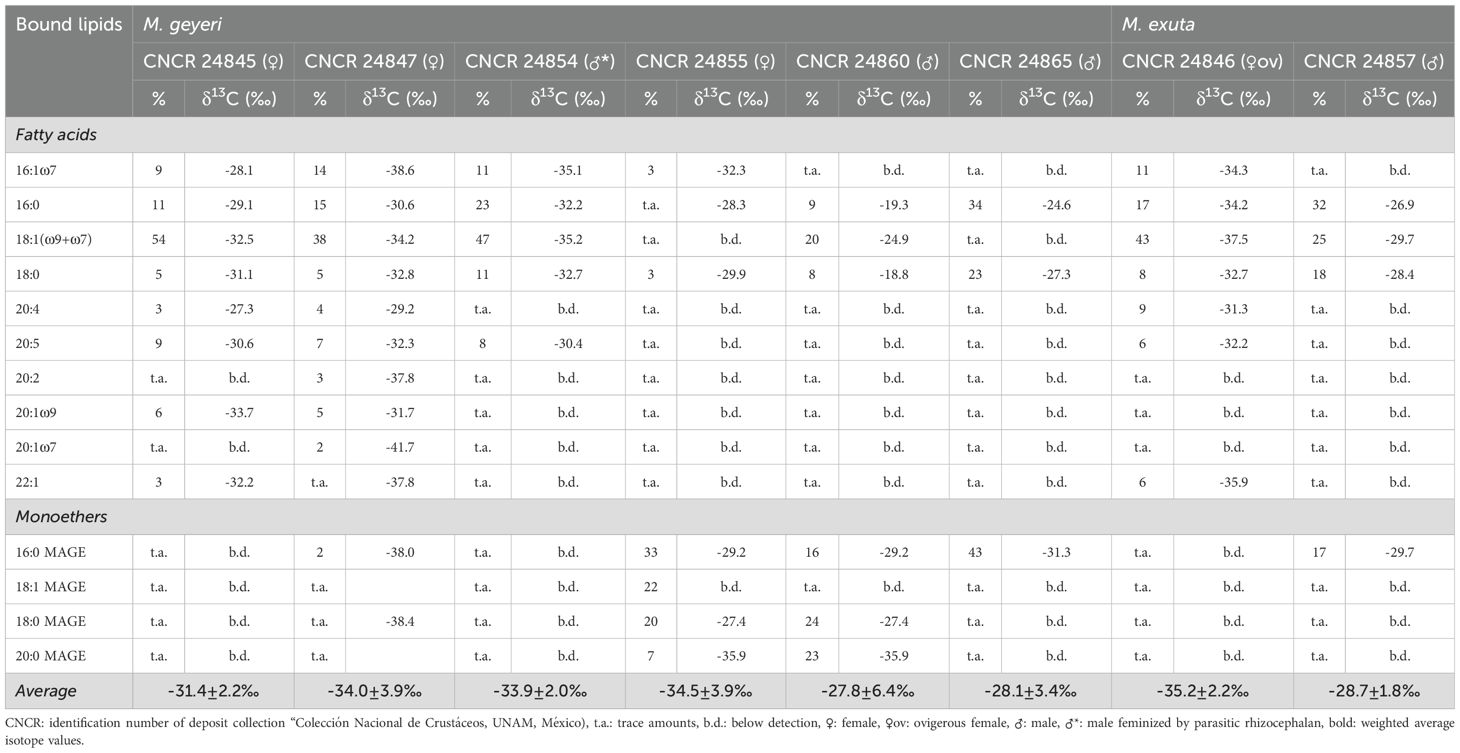
Table 6. Relative abundances and stable carbon isotope values of bound fatty acids and monoalkyl glycerol ethers (MAGE).
4 Discussion
4.1 Habitat description: image and mosaic analysis
Chapopote asphalt morphology varies across wide areas from fresh and ductile to old and fragmented increasing habitat heterogeneity by providing substratum for benthic biota (Jones et al., 2014). Thus, the heterogeneity of Chapopote habitats and the different types of seepage (gas and asphalt) support characteristic faunal assemblages (Table 2). Sibuet and Olu-Le Roy (2002) recognized well-defined, areas of organisms in large abundance that are clearly associated to carbonated structures. At Chapopote Knoll, we recognize two carbonated habitats: carbonate hosting Siboglinidae clusters and carbonate with breccia. Similar habitats have been observed at other chemosynthetic sites (Bergquist et al., 2003).
The megabenthic components on Chapopote are represented by families common to other described seeps sites (Ondréas et al., 2005) and contrast with other asphalt-associated ecosystems (Santa Barbara basin extinct asphalts and Angola margin asphalt mounds) where more than 50% of their biodiversity corresponds to vagrant and colonist biota (Valentine et al., 2010; Jones et al., 2014).
4.2 Spatial distribution
Both A. muricola and the two Munidopsis species displayed preference to specific habitats with an aggregated spatial distribution. In summary, Table 3 shows that M. geyeri and M. exuta occurred in all habitats in contrast with A. muricola. The statistical results showed patterns of occurrence in Chapopote Knoll: a larger abundance of the caridean shrimp A. muricola in active seepage areas which supports the biota with chemoautotrophic symbionts that provide 3D structures, Escarpia laminata that forms Siboglinidae clusters habitat and Bathymodiolus heckerae - B. brooksi mussel aggregations in the gas seeping habitat (Figure 3). These distribution patterns agree with A. muricola from Regab pockmark (Gulf of Guinea; Olu et al., 2009). In contrast, Munidopsis species had higher abundance on asphalt flows, followed by asphalt fragments and hydrocarbon seepage (Figure 3). Their presence on all habitats, as showed in mosaic of Figure 2A is similar to other deep sea background benthic species (e.g. Echinus affinis sea urchin and Hyalinoecia sp. polychaete worm; Grassle et al., 1975) that forage without preference in the diverse habitats.
4.3 Potential and ingested diet: gut content, stable isotope measurements, and lipid analyses
A. muricola and M. geyeri coexist in a similar way as the analogue species that have been reported on the Regab pockmark (Olu et al., 2009). Munidopsis exuta is a second galatheid species that occurs on Chapopote Knoll. Although A. muricola showed high percentages of detritus in their gut contents, our results agree with previous reports for a similar ecosystem (Regab pockmark) (Komai and Segonzac, 2005) where their diet is chemoautotrophic-dependent in contrast with M. geyeri and M. exuta characterized by a wide-spectrum diet. We suggest a trade-off of the two taxa in differentiated life histories (Supplementary Material), where A. muricola is a specialist species and M. geyeri and M. exuta are generalist organisms, able to use the resources available from diverse habitats opportunistically (MacArthur, 1972).
Muscle tissues from the three species of decapod Crustacea analyzed had δ13C stable isotope values that suggest a partial chemoautotrophic carbon source (δ13C ¾ -25‰; Demopoulos et al., 2010). This contrasts with fauna strongly dependent on photosynthetically fixed carbon, with enriched δ13C values (-25‰ to -15‰, Fry and Sherr, 1989; -22.4 ± 0.3; Fujikura et al., 2017). The slightly enriched signal for δ13C in tissues from A. muricola inhabiting Chapopote Knoll contrasts with previous reports from the Regab pockmark (δ13C -38.5 ± 2.03 to -50.4‰ ± 0.92, n=17; Olu et al., 2009), and this supports the wide spectrum of potential food sources including chemoautotrophic organisms, detritus and/or recycled organic matter on Chapopote sediments or retained among the Siboglinidae. Related species from shallower cold seeps of the Gulf of Mexico also have enriched δ13C values (e.g. -20.6‰ on A. stactophila at 1500 m; MacAvoy et al., 2005). In particular, M. geyeri had δ13C depleted values, similar to those reported from the Regab pockmark (δ13C -36.33 ± 1.05, n=3; Olu et al., 2009). The depleted values in M. exuta tissues (Table 4) as well as the presence of 13C-depleted bacterial fatty acids (Tables 5, 6) reaffirm that part of the diet in Munidopsis is of chemoautotrophic origin in addition with other background and pelagic carbon sources. The large differences in lipid δ13C values observed between individuals of one of the Munidopsis species (Tables 5, 6), suggest that they are highly adaptable to the available food sources, and use bacterial mats as well as water column debris. The similar δ15N values recorded in all three crustacean species suggest a similar trophic position, as secondary consumers as recorded by Olu et al. (2009) for A. muricola: δ15N 2.6 ± 0.45 to 5.3 ± 0.26‰, n=17; in the Regab pockmark. Small invertebrate and mat e.g., Beggiatoa sp. is potential carbon source for A. muricola, whereas macrofauna and meiofaunal nematodes are potential carbon sources for Munidopsis species. A shift in trophic position is recorded as an enrichment of δ13C and δ15N (Figure 6) in A. muricola and Munidopsis. Our stable isotope values lie within those recorded for similar species by Demopoulos et al. (2010) in other seep locations in the Gulf of Mexico (Figure 6) and for M. geyeri in the Regab pockmark (Macpherson and Segonzac, 2005; Olu et al., 2009). Munidopsis species seem to maintain a similar type of diet elsewhere with δ13C values within the range from -1‰ to -53.30‰ in chemosynthetic ecosystems (Levin and Mendoza, 2007) and δ15N values from 5.5‰ to 12.4‰ (Fisher et al., 1994; Escobar-Briones et al., 2002).
The stable carbon isotope composition of the intact polar lipid bound fatty acids and monoalkylglycerolethers, which are most likely derived from eukaryotic plasmalogens and phospholipids of the gut membrane, are very similar to the free lipids (Tables 5, 6). Females of M. geyeri and M. exuta had relatively depleted δ13C in Chapopote while males had more enriched δ13C lipid values (Tables 5, 6). This apparent difference, previously recorded only in albatross Phoebastria irrorata (Awkerman et al., 2007); skimmer bird Rynchops niger intercedens (Mariano-Jelicich et al., 2007); seals Callorhinus ursinus and rats Rattus norvegicus (Kurle et al., 2014) has been associated to a larger protein intake but has not been recorded before in Crustacea. Trophic partitioning has been recorded in shrimp species (de Aquino Ferreira et al., 2022) and dietary shifts with growth have been reported in squid (Cherel et al., 2009). It will require a more detailed analysis with a larger sample set to clarify the trophic segregation recorded in males and females in the two Munidopsis species.
The importance of the bacterial filaments in crustacean diets has been discussed since the late 1990s (Pond et al., 1997; Polz et al., 1998). However, the nutritional role of bacterial mat or of ectobiont bacteria that grow on decapod appendices has remained enigmatic in observations made in seeps and hydrothermal vents. It is not a constant practice in all published studies to have data from direct feeding records, from experimental work, from gut content, from fatty acid and stable isotope analysis, and the presence of enzymes necessary for carbon fixation. Crustacea that potentially feed on bacterial derived carbon at chemosynthetic sites include lithodid crabs Paralomis sp (Niemann et al., 2013), Shinkaia crosnieri galatheid (Tsuchida et al., 2011; Zhao et al., 2020; Feng et al., 2023), squat lobsters Munidopsis spp (Zhao et al., 2020), M. alvisca and M. verrilli (Hoyoux et al., 2012), Kiwa hirsuta (Goffredi, 2010) and K. puravida crab (Thurber et al., 2011), and intermediate size shrimp Chorocaris chacei and adult Rimicaris exoculata (Gebruk et al., 2000).
Bacteria may be an important food source for seep infauna too, proving to be an essential nutritional item for seep-associated infaunal macrobenthos (Demopoulos et al., 2010). Lipid and isotope analyses provide evidence food sources (Thurber et al., 2011; Tsuchida et al., 2011), direct observation provide the behavior of feeding strategies, and specific fatty acids help trace bacterial assimilation into tissues (Pond et al., 1997). Morphological structures and tegument types are used to host, harvest or feed on bacteria. The host’s behavior can benefit from a more efficient use of chemical fluxes near seeps explaining a different distribution of species using the bacteria (Feng et al., 2023).
5 Conclusions
Chapopote Knoll encompasses diverse benthic abyssal habitats. Among these the Siboglinidae clusters represent a dominant faunal component restricted to areas with methane seepage.
The decapod Crustacea species A. muricola, M. geyeri and M. exuta coexist spatially and feed in the asphalt volcano habitats. A. muricola has an aggregated distribution in the Siboglinidae clusters and gas seepage sites, and M. geyeri and M. exuta are distributed in all habitats with a preference on asphalt flows. The diet of the three species, A. muricola, M. geyeri and M. exuta, with contrasting carbon sources is explained by the spatial distribution patterns.
The stable isotope composition of A. muricola indicates a preferential intake of chemoautotrophic organisms, detritus and/or recycled organic matter on Chapopote sediments or retained among the Siboglinidae. In contrast the stable isotopic composition of M. geyeri and M. exuta records a wider spectrum of food items. A trophic segregation between sexes on Munidopsis species was recorded that will need to be verified with a larger sample set.
Data availability statement
The datasets presented in this study can be found in online repositories. The names of the repository/repositories and accession number(s) can be found in the article/Supplementary Material.
Ethics statement
The manuscript presents research on animals that do not require ethical approval for their study.
Author contributions
AG: Conceptualization, Data curation, Formal analysis, Investigation, Methodology, Visualization, Writing – review & editing. SF: Data curation, Formal analysis, Investigation, Methodology, Writing – review & editing. MI: Writing – review & editing, Conceptualization, Project administration, Resources, Validation, Visualization. EE: Conceptualization, Funding acquisition, Project administration, Resources, Validation, Visualization, Writing – review & editing, Data curation, Formal analysis, Investigation, Methodology, Software, Supervision, Writing – original draft.
Funding
The author(s) declare financial support was received for the research, authorship, and/or publication of this article. The national and international projects that funded the present research, are a collaborative project between the Center for Marine and Environmental Sciences (MARUM) of the Bremen University, Germany, Study of the process related with fluid seepage in oceanic ground (E project of the former RCOM – Research center Ocean Margins and current Cluster of Excellence, EXC 2077-390741603, funded by the German Research Foundation (DFG) under Germany’s Excellence Strategy); Central Research Development Fund (CRDF), University of Bremen; Instituto de Ciencias del Mar y Limnología, UNAM, Mexico, Factores que definen la variabilidad de la diversidad biológica y biomasa en el mar profundo del Golfo de México (PAPIIT), CONACyT 40158F; and Texas University (TAMU), USA.
Acknowledgments
We thank the captain and crew of R/V Meteor for their valuable help during research cruise M67/2b. Thanks to Dr. Markus Brüning (MARUM, Bremen) for advice on Ifremer’s ADELIE software use to process mosaics from videotapes; M. in C. León Felipe Álvarez for his advice and supplies for cartographic representation; Professor Dr. Kai-Uwe Hinrichs for the use of his laboratory facilities (MARUM, Bremen) and Xavier Prieto-Mollar and Jenny Wendt for the help in the laboratory. In Memoriam to Dr. Heiko Sahling who contributed to the development of this manuscript. Instituto de Ciencias del Mar y Limnología, UNAM, Mexico for partial coverage of the article processing fee.
Conflict of interest
The authors declare that the research was conducted in the absence of any commercial or financial relationships that could be construed as a potential conflict of interest.
The author(s) declared that they were an editorial board member of Frontiers, at the time of submission. This had no impact on the peer review process and the final decision.
Generative AI statement
The authors declare that no Gen AI was used in the creation of this manuscript.
Publisher’s note
All claims expressed in this article are solely those of the authors and do not necessarily represent those of their affiliated organizations, or those of the publisher, the editors and the reviewers. Any product that may be evaluated in this article, or claim that may be made by its manufacturer, is not guaranteed or endorsed by the publisher.
Supplementary material
The Supplementary Material for this article can be found online at: https://www.frontiersin.org/articles/10.3389/fmars.2025.1534328/full#supplementary-material
References
Awkerman J. A., Hobson K. A., Anderson D. J. (2007). Isotopic (δ15N and δ13C) evidence for intersexual foraging differences and temporal variation in habitat use in waved albatrosses. Can. J. Zool. 85, 273–279. doi: 10.1139/z06-202
Bergquist D. C., Ward T., Cordes E. E., McNelis T., Howlett S., Kosoff R., et al. (2003). Community structure of vestimentiferan-generated habitat islands from Gulf of Mexico cold seeps. J. Exp. Mar. Bio. Ecol. 289, 197–222. doi: 10.1016/S0022-0981(03)00046-7
Bode A., Álvarez-Ossorio M. T., Carrera P., Lorenzo J. (2004). Reconstruction of trophic pathways between plankton and the North Iberian sardine (Sardina pilchardus) using stable isotopes. Sci. Mar. 68, 165–178. doi: 10.3989/scimar.2004.68n1165
Brüning M., Sahling H., MacDonald I. R., Ding F., Bohrmann G. (2010). Origin, distribution, and alteration of asphalts at Chapopote Knoll, Southern Gulf of Mexico. Mar. Pet. Geol. 27, 1093–1106. doi: 10.1016/j.marpetgeo.2009.09.005
Carney R. S. (1994). Consideration of the oasis analogy for chemosynthetic communities at Gulf of Mexico hydrocarbon vents. Geo-Marine Lett. 14, 149–159. doi: 10.1007/BF01203726
Cherel Y., Fontaine C., Jackson G. D., Jackson C. H., Richard P. (2009). Tissue, ontogenic and sex-related differences in δ13C and δ15N values of the oceanic squid Todarodes filippovae (Cephalopoda: Ommastrephidae). Mar. Biol. 156, 699–708. doi: 10.1007/s00227-008-1121-x
CONACyT (1982). Ciencia y Tecnología para el aprovechamiento de los recursos marinos (situación actual, problemática y políticas indicativas) (Mexico: Centro Cultural Universitario C.U).
de Aquino Ferreira K., Araújo Braga A., Madeira Di Beneditto A. P. (2022). Interspecific and intraspecific comparison of the isotopic niche of shrimps targets of fishing in south-eastern Brazil. J. Mar. Biol. Assoc. United Kingdom 102, 338–344. doi: 10.1017/S0025315422000558
Demopoulos A. W. J., Gualtieri D., Kovacs K. (2010). Food-web structure of seep sediment macrobenthos from the Gulf of Mexico. Deep Sea Res. Part II Top. Stud. Oceanogr. 57, 1972–1981. doi: 10.1016/j.dsr2.2010.05.011
Ding F., Spiess V., Brüning M., Fekete N., Keil H., Bohrmann G. (2008). A conceptual model for hydrocarbon accumulation and seepage processes around Chapopote asphalt site, southern Gulf of Mexico: from high resolution seismic point of view. J. Geophys. Res. 113, 1–15. doi: 10.1029/2007JB005484
Escobar-Briones E., Morales P., Cienfuegos E., G. M. (2002). “Carbon sources and trophic position of two abyssal species of Anomura, Munidopsis alvisca (Galatheidae) and Neolithodes diomedae (Lithodidae),” in Contributions to the Study of East Pacific Crustaceans. Ed. Hendrickx M. (Universidad Nacional Autónoma de México, Mexico), 37–43.
Feng W., Wang M., Dong D., Hui M., Zhang H., Fu L., et al. (2023). Variation in epibiotic bacteria on two squat lobster species of Munidopsidae. Front. Microbiol. 14, 1197476. doi: 10.3389/fmicb.2023.1197476
Fink J. H., Fletcher R. C. (1978). Ropy pahoehoe: surface folding of a viscous fluid. J. Volcanol. Geotherm. Res. 4, 151–170. doi: 10.1016/0377-0273(78)90034-3
Fisher C. R., Childress J. J., Macko S. A., Brooks J. M. (1994). Nutritional interactions in Galapagos Rift hydrothermal vent communities: inferences from stable carbon and nitrogen isotope analyses. Mar. Ecol. Prog. Ser. 103, 45–55. doi: 10.3354/meps103045
Fry B., Sherr E. B. (1989). “δ13C Measurements as indicators of carbon flow in marine and freshwater ecosystems,” in Stable isotopes in ecological research. Ecological Studies (Analysis and Synthesis). Eds. Rundel P. W., Ehleringer J. R., Nagy K. A. (Springer, New York, NY, New York), 196–229. doi: 10.1007/978-1-4612-3498-2_12
Fujikura K., Yamanaka T., Sumida P. Y. G., Bernardino A. F., Pereira O. S., Kanehara T., et al. (2017). Discovery of asphalt seeps in the deep Southwest Atlantic off Brazil. Deep Sea Res. Part II Top. Stud. Oceanogr. 146, 35–44. doi: 10.1016/j.dsr2.2017.04.002
Gaytán-Caballero A., MacDonald I., Morales-Domínguez E., Escobar-Briones E. (2015). “Biology,” in METEOR-Berichte, Natural hydrocarbon seepage in the southern Gulf of Mexico, Cruise No. M114. February 12 – March 28, 2015. Eds. Sahling H., Bohrmann G. (Editorial Assistance: DFG-Senatskommission für Ozeanographie, MARUM – Zentrum für Marine Umweltwissenschaften der Universität Bremen, Kingston (Jamaica) – Kingston (Jamaica), 39–40.
Gebruk A. V., Southward E. C., Kennedy H., Southward A. J. (2000). Food sources, behaviour, and distribution of hydrothermal vent shrimps at the Mid-Atlantic Ridge J. Mar. Biol. Ass. U.K. 80, 485–499. doi: 10.1017/S0025315400002186
Goffredi S. K. (2010). Indigenous ectosymbiotic bacteria associated with diverse hydrothermal vent invertebrates. Environ. Microbiol. Rep. 2, 479–488. doi: 10.1111/j.1758-2229.2010.00136.x
Grassle J. F., Sanders H. L., Hessler R. R., Rowe G. T., McLellan T. (1975). Pattern and zonation: a study of the bathyal megafauna using the research submersible Alvin. Deep Sea Res. Oceanogr. Abstr. 22, 457–481. doi: 10.1016/0011-7471(75)90020-0
Gustafson R. G., Turner R. D., Lutz R. A., Vrijenhoek R. C. (1998). A new genus and five new species of mussels (Bivalvia: Mytilidae) from deep-sea sulfide/hydrocarbon seeps in the Gulf of Mexico. Malacologia 40 (1-2), 63–112. Available online at: http://biodiversitylibrary.org/page/13112012
Hoyoux C., Zbinden M., Samadi S., Gaill F., Compère P. (2012). Diet and gut microorganisms of Munidopsis squat lobsters associated with natural woods and mesh-enclosed substrates in the deep South Pacific. Mar. Biol. Res. 8, 28–47. doi: 10.1080/17451000.2011.605144
Jones D. O. B., Walls A., Clare M., Fiske M. S., Weiland R. J., O’Brien R., et al. (2014). Asphalt mounds and associated biota on the Angolan margin. Deep. Res. Part I Oceanogr. Res. Pap. 94, 124–136. doi: 10.1016/j.dsr.2014.08.010
Jones M. L. (1985). On the Vestimentifera, new phylum: six new species, and other taxa, from hydrothermal vents and elsewhere. Bull. Biol. Soc. Wash. 6, 117–158.
Kneitel J. M., Chase J. M. (2004). Trade-offs in community ecology: linking spatial scales and species coexistence. Ecol. Lett. 7, 69–80. doi: 10.1046/j.1461-0248.2003.00551.x
Komai T., Segonzac M. (2005). A revision of the genus Alvinocaris Williams and Chace (Crustacea: Decapoda: Caridea: Alvinocarididae), with descriptions of a new genus and a new species of Alvinocaris. J. Nat. Hist. 39, 1111–1175. doi: 10.1080/00222930400002499
Kurle C. M., Koch P. L., Tershy B. R., Croll D. A. (2014). The effects of sex, tissue type, and dietary components on stable isotope discrimination factors (δ13C and δ15N) in mammalian omnivores. Isotopes Environ. Health Stud. 50, 307–321. doi: 10.1080/10256016.2014.908872
Levin L. A., Mendoza G. F. (2007). Community structure and nutrition of deep methane-seep macrobenthos from the North Pacific (Aleutian) Margin and the Gulf of Mexico (Florida Escarpment). Mar. Ecol. 28, 131–151. doi: 10.1111/j.1439-0485.2006.00131.x
Ludvigsen M., Sortland B., Johnsen G., Singh H. (2007). Applications of georeferenced underwater photo mosaics in Marine Biology and Archaeology. Oceanography 20, 140–149. doi: 10.5670/oceanog.2007.14
MacArthur R. H. (1972). Geographical ecology: patterns in the distribution of species (New York: Harper & Row).
MacAvoy S. E., Fisher C. R., Carney R. S., Macko S. A. (2005). Nutritional associations among fauna at hydrocarbon seep communities in the Gulf of Mexico. Mar. Ecol. Prog. Ser. 292, 51–60. doi: 10.3354/meps292051
MacDonald I. (2014). Exploration of the Gulf of Mexico 2014: The Asphalt Ecosystem of the Gulf of Mexico (Silver Spring: NOAA Ocean Exploration). Available at: https://oceanexplorer.noaa.gov/okeanos/explorations/ex1402/logs/apr24/apr24.html. (Accessed June 16, 2024)
MacDonald I. R., Bohrmann G., Escobar E., Abegg F., Blanchon P., Blinova V., et al. (2004). Asphalt volcanism and chemosynthetic life in the Campeche Knolls, Gulf of Mexico. Science 304, 999–1002. doi: 10.1126/science.1097154
MacDonald I., Gaytán-Caballero A., Escobar Briones E. (2020). “The asphalt ecosystem of the southern Gulf of Mexico: abyssal habitats across space and time,” in Scenarios and Responses to Future Deep Oil Spills. Eds. Murawski S. A., Ainsworth C. H., Gilbert S., Hollander D. J., Paris C. B., Schlüter M., Wetzel D. L. (Cham: Springer International Publishing), 132–146. doi: 10.1007/978-3-030-12963-7_8
Macpherson E., Segonzac M. (2005). Species of the genus Munidopsis (Crustacea, Decapoda, Galatheidae) from the deep Atlantic Ocean, including cold-seep and hydrothermal vent areas. Zootaxa 1095, 1–60. doi: 10.11646/zootaxa.1095.1.1
Marcon Y., Sahling H., MacDonald I. R., Wintersteller P., Ferreira C., dos S., et al. (2018). Slow volcanoes: The intriguing similarities between marine asphalt and basalt lavas. Oceanography 31, 1–12. doi: 10.5670/oceanog.2018.202
Mariano-Jelicich R., Madrid E., Favero M. (2007). Sexual dimorphism and diet segregation in the black skimmer Rynchops Niger. Ardea 95, 115–124. doi: 10.5253/078.095.0113
McCutchan J. H. Jr., Lewis W. M. Jr., Kendall C., McGrath C. C. (2003). Variation in trophic shift for stable isotope ratios of carbon, nitrogen, and sulfur. OIKOS 102, 378–390. doi: 10.1034/j.1600-0706.2003.12098.x
Naehr T. H., Birgel D., Bohrmann G., MacDonald I. R., Kasten S. (2009). Biogeochemical controls on authigenic carbonate formation at the Chapopote “asphalt volcano”, Bay of Campeche. Chem. Geol. 266, 390–402. doi: 10.1016/j.chemgeo.2009.07.002
Niemann H., Linke P., Knittel K., MacPherson E., Boetius A., et al. (2013). Methane-carbon flow into the benthic food web at cold seeps – A case study from the Costa Rica subduction zone. PloS One 8 (10), e74894. doi: 10.1371/journal.pone.0074894
NOAA, Ocean Explorer (2017). NOAA Ship Okeanos Explorer: Gulf of Mexico 2017, Dive 09: “Henderson Ridge Mid South”. Available online at: https://oceanexplorer.noaa.gov/okeanos/explorations/ex1711/dailyupdates/dailyupdates.htmlcbpi=dec11.html (Accessed January 10, 2024).
Olu K., Caprais J. C., Galéron J., Causse R., von Cosel R., Budzinski H., et al. (2009). Influence of seep emission on the non-symbiont-bearing fauna and vagrant species at an active giant pockmark in the Gulf of Guinea (Congo-Angola margin). Deep. Res. Part II Top. Stud. Oceanogr. 56, 2380–2393. doi: 10.1016/j.dsr2.2009.04.017
Olu K., Cordes E. E., Fisher C. R., Brooks J. M., Sibuet M., Desbruye D. (2010). Biogeography and potential exchanges among the Atlantic Equatorial Belt cold-seep faunas. PloS One 5, 1–11. doi: 10.1371/journal.pone.0011967
Ondréas H., Olu K., Fouquet Y., Charlou J. L., Gay A., Dennielou B., et al. (2005). ROV study of a giant pockmark on the Gabon continental margin. Geo-Marine Lett. 25, 281–292. doi: 10.1007/s00367-005-0213-6
Pawson D. L., Vance D. J. (2004). Chiridota heheva, new species, from Western Atlantic deep-sea cold seeps and anthropogenic habitats (Echinodermata: Holothuroidea: Apodida). Zootaxa 534, 1–12. doi: 10.11646/zootaxa.534.1
Pequegnat L. H., Pequegnat W. E. (1970). “Deep-sea anomurans of superfamily Galatheoidea with description of three new species”, in: Contributions on the Biology of the Gulf of Mexico. Eds. Pequegnat W. E., Chace F. A. (College Station: Texas A & M University).
Pereira O. S., Shimabukuro M., Bernardino A. F., Sumida P. Y. G. (2020). Molecular affinity of Southwest Atlantic Alvinocaris muricola with Atlantic Equatorial Belt populations. Deep Sea Res. Part I Oceanogr. Res. Pap. 163, 103343. doi: 10.1016/j.dsr.2020.103343
Pérez-Brunius P., Furey H., Bower A., Hamilton P., Candela J., García-Carrillo P., et al. (2018). Dominant circulation patterns of the deep Gulf of Mexico. J. Phys. Oceanogr. 48, 511–529. doi: 10.1175/JPO-D-17-0140.1
Peterson B. J., Fry B. (1987). Stable Isotopes in ecosystem studies. Annu. Rev. Ecol. Syst. 18, 293–320. doi: 10.1146/annurev.es.18.110187.001453
Polz M. F., Robinson J. J., Cavanaugh C. M., Van Dover C. L. (1998). Trophic ecology of massive shrimp aggregations at a Mid-Atlantic Ridge hydrothermal vent site. Limnol. Oceanogr. 43, 1631–1638. doi: 10.4319/lo.1998.43.7.1631
Pond D. W., Dixon D. R., Bell M. V., Fallick A. E., Sargent J. R. (1997). Occurrence of 16: 2(n-4) and 18: 2(n-4) fatty acids in the lipids of the hydrothermal vent shrimps Rimicaris exoculata and Alvinocaris markensis: nutritional and trophic implications. Mar. Ecol. Prog. Ser. 156, 167–174. doi: 10.3354/meps156167
Raggi L., Schubotz F., Hinrichs K.-U., Dubilier N., Petersen J. M. (2012). Bacterial symbionts of Bathymodiolus mussels and Escarpia tubeworms from Chapopote, an asphalt seep in the southern Gulf of Mexico. Environ. Microbiol. 15 (7), 1969–1987. doi: 10.1111/1462-2920.12051
Ramirez-Llodra E., Segonzac M. (2006). Reproductive biology of Alvinocaris muricola (Decapoda: Caridea: Alvinocarididae) from cold seeps in the Congo Basin. J. Mar. Biol. Assoc. UK 86, 1347. doi: 10.1017/S0025315406014378
R Core Team (2001–2022). R: a language and environment for statistical computing (Vienna: R Found. Stat. Comput). Available at: http://www.r-project.org/ (Accessed November 10, 2023).
Rivas D., Badan A., Ochoa J. (2005). The ventilation of the deep Gulf of Mexico. J. Phys. Oceanogr. 35, 1763–1781. doi: 10.1175/jpo2786.1
Rubin-Blum M., Antony C. P., Borowski C., Sayavedra L., Pape T., Sahling H., et al. (2017). Short-chain alkanes fuel mussel and sponge Cycloclasticus symbionts from deep-sea gas and oil seeps. Nat. Microbiol. 2, 1–11. doi: 10.1038/nmicrobiol.2017.93
Rubin-Blum M., Antony C. P., Sayavedra L., Martínez-Pérez C., Birgel D., Peckmann J., et al. (2019). Fueled by methane: deep-sea sponges from asphalt seeps gain their nutrition from methane-oxidizing symbionts. ISME J. 13, 1209–1225. doi: 10.1038/s41396-019-0346-7
Sahling H., Borowski C., Escobar-Briones E., Gaytán-Caballero A., Hsu C.-W., Loher M., et al. (2016). Massive asphalt deposits, oil seepage, and gas venting support abundant chemosynthetic communities at the Campeche Knolls, southern Gulf of Mexico. Biogeosciences 13, 4491-4512. doi: 10.5194/bg-13-4491-2016
Schubotz F., Lipp J. S., Elvert M., Hinrichs K.-U. (2011a). Stable carbon isotopic compositions of intact polar lipids reveal complex carbon flow patterns among hydrocarbon degrading microbial communities at the Chapopote asphalt volcano. Geochim. Cosmochim. Acta 75, 4399–4415. doi: 10.1016/j.gca.2011.05.018
Schubotz F., Lipp J. S., Elvert M., Kasten S., Mollar X. P., Zabel M., et al. (2011b). Petroleum degradation and associated microbial signatures at the Chapopote asphalt volcano, Southern Gulf of Mexico. Geochim. Cosmochim. Acta 75, 4377–4398. doi: 10.1016/j.gca.2011.05.025
Sibuet M., Olu-Le Roy K. (2002). “Cold Seep communities on continental margins: structure and quantitative distribution relative to geological and fluid venting patterns,” in Ocean Margin Systems. Eds. Wefer G., Billett D., Hebbeln D., Jørgensen B. B., Schlüter M., van Weering T. C. E. (Springer Berlin Heidelberg, Berlin, Heidelberg), 235–225. doi: 10.1007/978-3-662-05127-6_15
Smrzka D., Zwicker J., Klügel A., Monien P., Bach W., Bohrmann G., et al. (2016). Establishing criteria to distinguish oil-seep from methane-seep carbonates. Geology 44, 667–670. doi: 10.1130/G38029.1
Sturt H. F., Summons R. E., Smith K., Elvert M., Hinrichs K.-U. (2004). Intact polar membrane lipids in prokaryotes and sediments deciphered by high-performance liquid chromatography/electrospray ionization multistage mass spectrometry—new biomarkers for biogeochemistry and microbial ecology. Rapid Commun. Mass Spectrom. 18, 617–628. doi: 10.1002/rcm.1378
Thurber A. R., Jones W. J., Schnabel K. (2011). Dancing for food in the deep sea: bacterial farming by a new species of yeti crab. PloS One 6, e26243. doi: 10.1371/journal.pone.0026243
Tsuchida S., Suzuki Y., Fujiwara Y., Kawato M., Uematsu K., Yamanaka T., et al. (2011). Epibiotic association between filamentous bacteria and the vent-associated galatheid crab, Shinkaia crosnieri (Decapoda: Anomura). J. Mar. Biol. Assoc. U K 91, 23–32. doi: 10.1017/S0025315410001827
Tyler P. A., Paterson G. J.L., Sibuet M., Guille A., Murtons B. J., Segonzac M. (1995). A new genus of ophiuroid (Echinodermata: Ophiuroidea) from hydrothermal mounds along the Mid-Atlantic Ridge. J. Mar. Biol. Ass. U.K. 75, 977–986. doi: 10.1017/S0025315400038303
UNINMAR (2011). Base map from Unidad de Informática Marina (Mexico City: Instituto de Ciencias del Mar y Limnología). Available at: http://uninmar.icmyl.unam.mx/geoportal/ (Accessed July 17, 2024).
Valentine D. L., Reddy C. M., Farwell C., Hill T. M., Pizarro O., Yoerger D. R., et al. (2010). Asphalt volcanoes as a potential source of methane to late Pleistocene coastal waters. Nat. Geosci. 3, 345–348. doi: 10.1038/ngeo848
Van Dover C. L., Fry B. (1994). Microorganisms as food resources at deep-sea hydrothermal vents. Limnol. Oceanogr. 39, 51–57. doi: 10.4319/lo.1994.39.1.0051
Velázquez Luna R. G. (2009). Ecología de Chiridota heheva holoturia asociada a un fondo abisal con volcanismo de asfalto en el sector Suroeste del Golfo de México (Master Thesis, Mexico City: Universidad Nacional Autónoma de México).
Vidal V. M. V., Vidal F. V., Hernández A. F., Meza E., Zambrano L. (1994). Winter water mass distributions in the western Gulf of Mexico affected by a colliding anticyclonic ring. J. Oceanogr. 50, 559–588. doi: 10.1007/bf02235424
Warén A., Bouchet P. (1993). New records, species, genera, and a new family of gastropods from hydrothermal vents and hydrocarbon seeps. Zool. Scr. 22, 1–90.
Warén A., Bouchet P. (2009). New gastropods from deep-sea hydrocarbon seeps off West Africa. Deep-Sea Res. II: Top. Stud. Oceanogr. 56(23), 2326–2349. doi: 10.1016/j.dsr2.2009.04.013
Warén A., Ponder W. F. (1991). New species, anatomy, and systematic position of the hydrothermal vent and hydrocarbon seep gastropod family Provannidae fam.n. (Caenogastropoda). Zool. Scr. 20(1), 27–56.
Wegener G., Knittel K., Bohrmann G., Schubotz F. (2020). “Benthic deep-sea life associated with asphaltic hydrocarbon emissions in the southern Gulf of Mexico,” in Marine hydrocarbon seeps. Eds. Teske A., Carvalho V. (Springer, Cham), 101–123. doi: 10.1007/978-3-030-34827-4_5
Weiland R. J., Adams G. P., McDonald R. D., Rooney T. C., Wills L. M. (2008). “Geological and biological relationships in the Puma appraisal area: from salt diapirism to chemosynthetic communities,” in Offshore Technology Conference (Offshore Technology Conference, Houston), 1–16. doi: 10.4043/19360-MS
Williams A. B. (1988). New marine decapod crustaceans from waters influenced by hydrothermal discharge, brine, and hydrocarbon seepage. Fish. Bull. 86, 263–287.
Williamson S. C., Zois N., Hewitt A. T. (2008). “Integrated site investigation of seafloor features and associated fauna, Shenzi field, deepwater Gulf of Mexico,” in Offshore Technology Conference “Waves of Change” (Offshore Technology Conference, Houston), 1–8. doi: 10.4043/19356-MS
Zar J. (2010). Biostatistical analysis. 4th ed (Hoboken: Prentice Hall). doi: 10.1017/CBO9781107415324.004
Zhao Y., Xu T., Sheung Law Y., Feng D., Li N., Xin R., et al. (2020). Ecological characterization of cold-seep epifauna in the South China Sea. Deep Sea Res Part I 163, 103361. doi: 10.1016/j.dsr.2020.103361
Zhu C., Lipp J. S., Wörmer L., Becker K. W., Schröder J., Hinrichs K.-U. (2013). Comprehensive glycerol ether lipid fingerprints through a novel reverse-phase liquid chromatography-mass spectrometry protocol. Org. Geochem. 65, 53–62. doi: 10.1016/j.orggeochem.2013.09.012
Keywords: asphalt, knoll, crustacean, cold seep, deep sea, Gulf of Mexico, habitat, local distribution
Citation: Gaytán-Caballero A, Schubotz F, MacDonald IR and Escobar-Briones EG (2025) Descriptive ecology of abyssal decapods from Chapopote Knoll (southwestern Gulf of Mexico). Front. Mar. Sci. 12:1534328. doi: 10.3389/fmars.2025.1534328
Received: 25 November 2024; Accepted: 09 January 2025;
Published: 07 February 2025.
Edited by:
Tamara Frank, Nova Southeastern University, United StatesReviewed by:
Danielle M DeLeo, Florida International University, United StatesEnrique Macpherson, Spanish National Research Council (CSIC), Spain
Copyright © 2025 Gaytán-Caballero, Schubotz, MacDonald and Escobar-Briones. This is an open-access article distributed under the terms of the Creative Commons Attribution License (CC BY). The use, distribution or reproduction in other forums is permitted, provided the original author(s) and the copyright owner(s) are credited and that the original publication in this journal is cited, in accordance with accepted academic practice. No use, distribution or reproduction is permitted which does not comply with these terms.
*Correspondence: Elva G. Escobar-Briones, ZXNjb2JyaUBjbWFybC51bmFtLm14