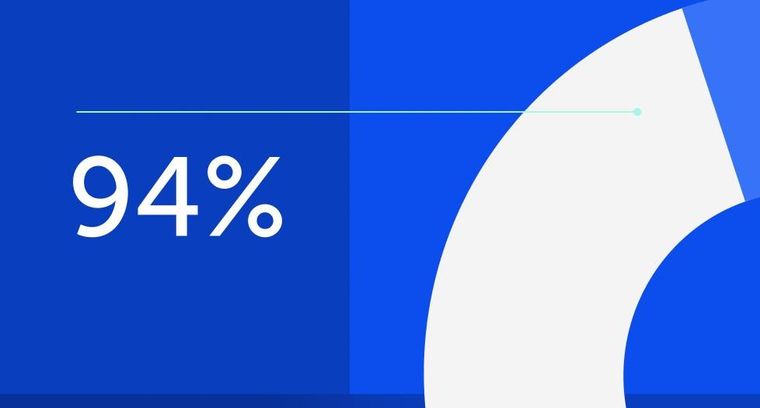
94% of researchers rate our articles as excellent or good
Learn more about the work of our research integrity team to safeguard the quality of each article we publish.
Find out more
ORIGINAL RESEARCH article
Front. Mar. Sci., 28 February 2025
Sec. Coastal Ocean Processes
Volume 12 - 2025 | https://doi.org/10.3389/fmars.2025.1531902
Introduction: Spartina alterniflora invasion may alter the characteristics and functions of the coastal wetland ecosystems. Previous studies have shown that invasion changes the biogeochemical processes and microbial diversity in marsh ecosystems; however, knowledge of the changes in bacterial communities and their function at different soil depths during invasion remains limited.
Methods: In the present study, we investigated S. alterniflora invasion on the dynamic changes of soil bacterial communities using Illumina MiSeq sequencing analyses of 16S rRNA at different soil depths (i.e., 0–10, 10–20, and 20–40 cm) during the invasive process (i.e., 1, 4, 7, and 12 years), as well as the potential correlations between soil physicochemical characteristics and enzyme activities.
Results: The invasion of S. alterniflora did not significantly affect soil bacterial alpha diversity or the functional profiles at the first and second levels of the Kyoto Encyclopedia of Genes and Genomes (KEGG). Furthermore, no significant changes were observed across different soil depths. However, the relative abundances of Chloroplast and Alphaproteobacteria increased from 3.03% and 5.05% in bare mudflat to 13.61% and 8.95% in the 12-year-old S. alterniflora invaded soil, respectively, whereas those of Deltaproteobacteria, Acidimicrobiia, and Bacilli decreased after S. alterniflora invasion for approximately 12 years. The relative abundances of soil bacteria varied with soil depth. Total phosphorus, carbon-to-nitrogen ratio, total carbon, and catalase activity were the key factors affecting soil bacterial community composition.
Discussion: The soil physicochemical characteristics changes caused by invasion may temporarily enhance soil fertility, they can lead to long-term wetland degradation through changed biological community and altered nutrient dynamics. This study provides a comprehensive understanding of the dynamic changes in bacterial communities, soil physicochemical characteristics, and enzyme activities during S. alterniflora invasion on coastal wetlands.
The invasion of alien plant species poses great threats to the biodiversity and function of the native ecosystem, which is considered the primary driving force for the changes in the invaded ecosystem (Zhang et al., 2021; Zhao et al., 2023) and can change soil biochemical characteristics, nutrient cycles, and microbial communities (Nirmala et al., 2023). Among them, Spartina alterniflora is a typical and common invasive species that has been introduced extensively because it can control natural erosion in coastal areas (Kim et al., 2023; Yang et al., 2023). The introduction of S. alterniflora in China began in 1979 and has quickly spread along the coasts, and subsequently became one of the beneficial species within coastal wetland ecosystems (Yang et al., 2016). Compared with other ecosystems, coastal wetlands typically have high productivity and offer valuable ecological services (Ge et al., 2020; Meng et al., 2020). Thus, it is essential to determine the impact of S. alterniflora invasion on soil characteristics to better understand the impact of invasion on coastal wetland ecosystems.
Soil microorganisms are of great significance in various processes within coastal wetland ecosystems, such as element cycles, information transmission, species distribution, and other biochemical processes (Thatoi et al., 2013; Hou et al., 2019; Ge et al., 2019), and microbial communities are sensitive to soil environmental alterations (Card and Quideau, 2010; Deng et al., 2016; An et al., 2020). Bacteria are considered one of the most abundant and diverse microbial communities that play pivotal roles in mediating soil biogeochemical cycles (Freedman and Zak, 2015; Jurburg et al., 2018; He et al., 2023). Understanding the conversion of soil bacterial communities provides a valuable link between plant invasion and soil development because it reveals the complex interactions between plants, soil, and microbes that drive ecosystem change. For instance, S. alterniflora invasion can change soil physicochemical characteristics, alter soil microbial community composition, and significantly affect some special functional bacteria related to nutrient cycles (Li et al., 2020; Lin et al., 2022). Therefore, understanding soil bacterial community and function succession after S. alterniflora invasion is fundamental for illustrating the relationship between S. alterniflora invasion and wetland ecosystem functions. Compared with the bare mudflat, the impact of S. alterniflora invasion on soil bacterial community composition and diversity was inconsistent; it significantly increased (Song et al., 2022) and decreased (Gao et al., 2019), and the changes in bacterial communities were time-dependent (Yang et al., 2019). Soil pH (Li et al., 2020), moisture (Nguyen et al., 2018), salinity (Guo et al., 2018), and nutrient substrates (Ling et al., 2017) play vital roles in driving the diversity of metabolic functions in coastal wetlands. In addition to these factors, the invasion age is an important driver of variation in soil microbial diversity (Zhang et al., 2020).
Plant invasion can alter soil microbial communities and the biogeochemical processes they mediate (Kao-Kniffin and Balser, 2008; Wang et al., 2023), thereby affecting nutrient cycling (Sokol et al., 2022; He et al., 2023). The deep root system of S. alterniflora releases exudates that can significantly alter the properties of deeper soil layers (Liao et al., 2007). The surface soil had higher soil organic carbon (SOC) and moisture content compared to deeper soil layers, and SOC storage initially decreased and then increased with increasing soil depth (Huang et al., 2022). Thus, the soil characteristics in deeper soil layers should also be considered (Nie et al., 2023), which may lead to a more precise explanation of these invasive effects. Although many studies have described the impact of S. alterniflora invasion on aboveground plant communities, soil characteristics, and functions (Davidson et al., 2018), little is known about its influence on soil physicochemical characteristics, enzyme activities, and bacterial communities at different soil depths during invasion.
The Jiangsu coastal zone is the largest S. alterniflora invasion region in China. Most of the natural areas is strictly protected and is rarely disturbed by human activities, making it an ideal research site. Our hypothesis posited that S. alterniflora invasion leads to alterations in soil bacterial communities, which, in turn, may be affected by varying soil characteristics and their respective depth profiles. The primary objectives of our study were as follows: (i) to evaluate the effects of S. alterniflora invasion on soil physicochemical characteristics and enzyme activities in different soil depths along the invasion age; (ii) to assess the alterations in soil bacterial community composition and function along the S. alterniflora invasion age; and (iii) to identify the main drivers that affect soil bacterial communities during the invasive process. Understanding this information is beneficial for improving the comprehension of the impact mechanism of S. alterniflora invasion and for accurately predicting the ecological influences.
We carried out this experiment on the coast of Xiangshui County, Jiangsu Province, China (119.8875–119.8945°E, 34.4623–34.4647°N) (Figure 1). The climate type is transitional warm temperate–subtropical oceanic monsoon. The mean temperature and annual rainfall is 13.9°C and 929.0 mm, respectively. There is no other vascular plant except S. alterniflora in the selected coastal wetland. The soil type is coastal Solonchaks (initial developmental stage) by the World Reference Base for Soil Resources and Halosols by the Chinese Soil Taxonomy. Soil samples were collected from five different habitats: natural mudflat (without S. alterniflora) and 1-, 4-, 7-, and 12-year-old S. alterniflora invasive soil. These soil samples were collected after the tide has receded. Here, the invasion ages were differentiated by the fixed marks and by previous remote sensing image data similar to our previous studies (Ge et al., 2020).
Figure 1. Map of the study area and soil sampling habitats. Notes: BM, bare mudflat; SA1, 1-year-old S. alterniflora; SA4, 4-year-old S. alterniflora; SA7, 7-year-old S. alterniflora; SA12, 12-year-old S. alterniflora.
All soil samples were taken in April 2023. At each of the five sampling habitats, three soil samples (approximately 50 m apart) were collected using 50-cm soil corers (diameter: 5 cm) and separated into three layers: surface soil layer (0–10 cm), middle soil layer (10–20 cm), and subsoil layer (20–40 cm). The soil samples were loaded into sterile Ziplock bags and taken to the laboratory using ice boxes. Soil samples from each habitat were processed as follows: three replicate samples from each habitat were individually homogenized and prepared for analysis. After the soil samples were well mixed, part of the soil was stored at 4°C to measure the enzyme activities, 10 g of the soil was stored at −80°C to analyze soil bacterial communities, and other soil samples were air-dried and passed through a 2-mm sieve to separate roots, large particles of gravel, and litter to analyze the physicochemical characteristics in the laboratory.
Soil moisture was determined by the oven-drying method at 105°C for 24 h. Soil salinity was calculated by determining electrical conductivity (EC); EC and pH were measured after a suspension of 5 g of soil and 25 mL of distilled water using a conductivity meter (Mettler-Toledo S230-B, Switzerland) and pH meter (Mettler-Toledo FE28, Switzerland), respectively. Total carbon (TC) and total nitrogen (TN) concentrations were determined by the CHNS elemental analyzer (Elementar Macrocube, Germany). SOC, total phosphorus (TP), and available phosphorus (AP) were determined with the methods of Avery and Bascomb (1982).
Catalase (CAT; EC 1.11.1.6), sucrase (SC; EC3.2.1.26), and urease (UE; EC 3.5.1.5) activities were measured according to the manufacturer’s specifications. CAT was analyzed using H2O2 as the substrate and determined with a spectrophotometer (UV-2450, SHIMADZU) at 240 nm, and SC was determined with the substrate of sucrose and measured at 508 nm. UE was determined with the substrate of urea and measured at 578 nm.
The total DNA was extracted from 0.5 g of soil (wet weight) using the PowerSoil®DNA Isolation Kit for soil (Mo Bio Laboratories, Inc., Carlsbad, CA, USA), according to the manufacturer’s specifications. Then, the amount of extracted DNA was measured by a NanoDrop TM 2000 spectrophotometer (Thermo Scientific, USA) before polymerase chain reaction (PCR). PCR analysis was implemented to determine the 16S rRNA gene copy number of bacteria by the universal primers F515 (5′-GTGCCAGCMGCCGCGGTAA-3′) together with R907 (5′-CCGTCAATTCMTTTRAGTTT-3′) with the barcode. The PCR cycling parameters were set as follows: 98°C for 1 min, 30 cycles of 10 s at 98°C, 30 s at 50°C and 60 s at 72°C, and finally with an extension at 72°C for 5 min. The PCR products were confirmed with 2% agarose gel and then purified by the DNA Purification Kit (TIANGEN, China) before sequencing. The sequencing was carried out by the Illumina MiSeq platform (Shanghai Majorbio BioPharm Technology Company, China).
Two-way analysis of variance (ANOVA) followed by Tukey’s tests was employed to identify the effect of S. alterniflora invasion ages on soil physicochemical characteristics, enzyme activities, and bacterial community richness and diversity indices in different soil depths. SIMPER was explored to detect the dissimilarity and the taxon contribution for the dissimilarity of bacterial community composition based on the data for the top 10 genera. Bacterial functional pathway modes within different soil samples were predicted with the PICRUSt database (Langille et al., 2013). The matrix of Pearson’s correlations was calculated to find potential relationships among soil physicochemical characteristics, enzyme activities, and the relative abundances of the top 10 classes. The relationships between the potential environmental factors (averaged for each site by three samples) and the soil bacterial communities (top 10 classes) were analyzed by redundancy analysis (RDA). All these statistical analyses were conducted using the software R 4.2.1 (R Core Team, 2022) and PAST 4.03 (Hammer et al., 2001).
Soil physicochemical characteristics changed after the invasion of S. alterniflora and differed among the invasion ages (Table 1). Soil physicochemical characteristics showed a variety of trends during the invasion process. Soil moisture significantly increased with S. alterniflora invasion age in the surface soil (0–10 cm) (p < 0.05) and also significantly increased with soil depth in the bare mudflat and the S. alterniflora-invaded 1-year soil (Table 1). The surface soil pH significantly decreased in the 12-year-old S. alterniflora soil, and deeper soil (10–40 cm) pH first increased with S. alterniflora invasion age, with the highest value in the 7-year-old S. alterniflora soil, and then decreased in the 12-year-old S. alterniflora soil (Table 1). Soil pH was not significantly affected by the soil depth, except in the bare mudflat soil. Soil EC and AP concentrations first increased with S. alterniflora invasion age and then decreased in the 7-year-old S. alterniflora soil, but significantly increased in the 12-year-old S. alterniflora soil (p < 0.05, Table 1). Compared with the bare mudflat soil, TP dramatically decreased after the invasion of S. alterniflora, and the value was the lowest in the 3-year-old S. alterniflora soil. TP and AP were generally unaffected by the soil depth at the same invasion time. The TN dramatically increased with S. alterniflora invasion age (Table 1). TN significantly increased with soil depth in the 1- and 4-year-old S. alterniflora soils. SOC decreased after S. alterniflora invasion for 1 year and then increased progressively with invasion time. TC was not significantly affected by S. alterniflora invasion age or soil depth (Table 1). S. alterniflora invasion age significantly influenced the physicochemical characteristics, and soil depth had an evident impact on the physicochemical characteristics. The interaction between S. alterniflora invasion age and soil depth had a significant impact on soil physicochemical characteristics (Supplementary Table S1).
Table 1. Soil physicochemical characteristics in different soil depths along S. alterniflora invasion ages.
Soil UE, SC, and CAT activities are presented in Figure 2. Compared to the bare mudflat, UE activity decreased at the S. alterniflora initial invasion stage, followed by a gradual increase with increasing invasion age, peaking in the 7-year-old S. alterniflora soil. It was significantly higher in the surface soil than in the subsoil. S. alterniflora invasion had no significant effect on the UE activity in the subsoil (20–40 cm). SC activity increased significantly with S. alterniflora invasion age, peaked in the 12-year-old S. alterniflora soil, and decreased with increasing soil depth. SC activity was the highest in the 12-year-old S. alterniflora surface soil. Soil CAT activity progressively increased after S. alterniflora invasion, peaking in the 4-year-old S. alterniflora soil, whereas no significant differences were observed among soil depths.
Figure 2. Soil enzyme activities in different soil depths along S. alterniflora invasion ages. (a) Urease; (b) catalase; (c) sucrase. Values are means ± SD. Different letters mean significant difference (p < 0.05) between soil depths and S. alterniflora invasion ages. BM, bare mudflat; SA1, 1-year-old S. alterniflora; SA4, 4-year-old S. alterniflora; SA7, 7-year-old S. alterniflora; SA12, 12-year-old S. alterniflora.
Chao1, ACE, Shannon, and Simpson values were measured to analyze bacterial community diversity (Table 2). In bare mudflat, Chao1 values were 1,406.7 ± 79.0 in the surface soil and 1,429.4 ± 195.9 in the subsoil, and invasive S. alterniflora substantially decreased the Chao1 values; they were 1,175.2 ± 191.9 in the surface soil and 1,090.9 ± 62.0 in the subsoil of 12-year-old S. alterniflora. Compared with bare mudflat, ACE values decreased with S. alterniflora invasion; they were 1,606.8 ± 202.0 and 1,593.7 ± 337.7 in bare mudflat surface soil and subsoil, respectively, and they were 1,372.0 ± 59.0 in the surface soil and 1,230.9 ± 127.6 in the subsoil of 12-year-old S. alterniflora. Shannon values were 5.6 ± 0.3 in the surface soil and 5.8 ± 0.3 in the subsoil of the bare mudflat. Invasive S. alterniflora did not significantly influence Shannon and Simpson values (Table 2). These results illustrate that S. alterniflora invasion did not significantly affect the soil bacterial alpha diversity.
Table 2. Alpha diversity indices of soil bacterial community of different soil depths in different sampling habitats.
The soil bacteria in the coastal wetland were mainly identified as the phylum Proteobacteria, Actinobacteria, Firmicutes, and Bacteroidetes (Figure 3). Proteobacteria was the main phylum in all bacterial communities and was dominated by Gammaproteobacteria (8.2%–14.3%), Deltaproteobacteria (8.0%–12.6%), and Alphaproteobacteria (5.1%–10.2%) (Figure 3). Compared with the bare mudflat, S. alterniflora invasion significantly increased the relative abundance of Chloroplast (p<0.05), and the relative abundance of Alphaproteobacteria increased by approximately twofold in the soil of 4-year-old S. alterniflora (Figure 3). The relative abundance of Chloroplast increased from 4.3% ± 1.5% and 0.7% ± 0.1% in the mudflat surface soil and deeper soil to 12.7% ± 4.5% and 22.8% ± 6.1% in the 7-year-old S. alterniflora surface soil and subsoil, respectively. In the subsoil, the relative abundance of Clostridia increased from 7.5% ± 2.5% in the bare mudflat to 12.7% ± 3.5% in 7-year-old S. alterniflora soils (Figure 3). However, S. alterniflora invasion substantially decreased the relative abundances of Acidimicrobiia. The relative abundance of Acidimicrobiia affiliated with Actinobacteria decreased from 11.0%–13.8% in the bare mudflat to 7.2%–10.1% in the 12-year-old S. alterniflora soil. The relative abundance of Bacilli affiliated with Firmicutes first increased with S. alterniflora invasion for 1 year, and then decreased by approximately 3.7-fold in the 7-year-old S. alterniflora soil (Figure 3). Chloroplast, Gammaproteobacteria, and Acidimicrobiia contributed 22.38%, 15.45%, and 13.56% of the total community difference, respectively, and they shared 51.38% of the total community difference (Table 3). The results of the 10 most abundant classes in each sample illustrated an evident variation in the soil bacterial community composition across different invasion ages of S. alterniflora (Supplementary Table S2). The relative abundances of the top 10 classes increased with invasion age, accounting for 69.8%, 72.6%, 74.0%, 72.3%, and 79.7% of the bacterial communities in the mudflats and 1-, 4-, 7-, and 12-year-old S. alterniflora soils, respectively (Supplementary Table S2).
Figure 3. The relative abundance of soil bacterial community composition. (a) 0–10 cm; (b) 10–20 cm; (c) 20–40 cm. Those with a relative abundance of less than 1% were grouped as others. BM, bare mudflat; SA1, 1-year-old S. alterniflora; SA4, 4-year-old S. alterniflora; SA7, 7-year-old S. alterniflora; SA12, 12-year-old S. alterniflora.
Table 3. Dissimilarity percentages (SIMPER) with the taxon contributions based on S. alterniflora invasion age (overall average dissimilarity = 38.72).
Pearson’s correlation analysis of soil bacterial communities with soil physicochemical characteristics and enzyme activities is shown in Figure 4. The results showed that moisture was significantly and positively correlated with EC, TC, TN, C/N, SOC, AP, and SC in the surface soils (correlation coefficients ranging from 0.67 to 0.93); it also had positive relationships with EC, TC, and AP in the subsoil (correlation coefficients ranging from 0.62 to 0.89). EC had a significantly positive association with TC, TN, C/N, and AP in both the surface soil and subsoil (correlation coefficients ranging from 0.62 to 0.92). EC was positively correlated with SOC and SC in the surface soil (r = 0.85 and 0.94) and CAT in the subsoil (r = 0.64). In addition, TP was negatively correlated with AP, CAT, UE, and SC in the surface soil (correlation coefficients ranging from 0.64 to 0.83), while it was negatively correlated with AP, CAT), UE, and SC in the subsoil (r = 0.55, 0.96, and 0.61, respectively). The results showed that Alphaproteobacteria was significantly and negatively correlated with pH, while positively correlated with TN in the surface soil. Bacilli was significantly and negatively correlated with TP (r = −0.58), and Deltaproteobacteria was positively correlated with TP (r = 0.52). Gammaproteobacteria had a significantly negative correlation with C/N in the surface soil (Figure 4A). Bacilli had negative relationships with moisture, EC, TC, C/N, SOC, AP, CAT, and SC in the middle soil, while being positively correlated with TP. Chloroplast had positive relationships with pH and CAT, while being negatively correlated with TP in the middle soil (Figure 4B). Deltaproteobacteria had a significantly negative correlation with TC, C/N, and CAT, while being positively correlated with UE in the subsoil (Figure 4C).
Figure 4. Pearson correlation analyses between soil physicochemical characteristics and the relative abundances of major bacterial class. (a) 0–10 cm; (b) 10–20 cm; (c) 20–40 cm. The color of the circle depends on the direction of the correlation (blue = positive; red = negative). The size of the circle is proportional to the r value. p-values in white are significant (*p < 0.05; **p < 0.01; ***p < 0.001). EC, electrical conductivity; TC, total carbon; TN, total nitrogen; C/N, carbon-to-nitrogen ratio; SOC, soil organic carbon; TP, total phosphorus; AP, available phosphorus; CAT, catalase; UE, urease; SC, sucrase.
The correlation indices among the soil physicochemical characteristics, enzyme activity, and relative abundances of bacterial communities also varied with soil depth (Figure 4). For instance, pH was negatively associated with Alphaproteobacteria (r = 0.52) in the surface soil, but positively correlated with Chloroplast (r = 0.61) in the subsoil. Deltaproteobacteria were positively correlated with TP (r = 0.52) in the surface soil but negatively correlated with TC (r = 0.52), C/N (r = 0.57), and CAT (r = 0.53) in the subsoil. UE was significantly positively correlated with Bacilli (r = 0.64) and negatively correlated with Acidimicrobiia (r = 0.59) in the surface soil. In comparison, in the subsoil, UE was positively correlated with Anaerolineae (r = 0.54) and Deltaproteobacteria (r = 0.60). The SC was positively correlated with Chloroplast (r = 0.59) in the surface soil and negatively correlated with Bacilli (r = 0.61) in the subsoil.
Soil characteristics had different relationships with bacterial communities. RDA illustrated the relationships between soil physicochemical characteristics, enzyme activity, and bacterial community composition. Soil characteristics in the first and second RDA dimensions accounted for 62.45% of the total variance in bacterial community structure (Figure 5). The RDA results clearly revealed that alterations in the soil bacterial community composition at the class level were most strongly associated with soil TP (Figure 5).
Figure 5. Relationships between soil physicochemical characteristics and bacterial community in different soil depths indicated by RDA ordination plots for the first two dimensions. The extent of correlations between soil characteristics and RDA axes is represented by the length and angle of arrows (soil characteristic vectors). BM, bare mudflat; SA1, 1-year-old S. alterniflora; SA4, 4-year-old S. alterniflora; SA7, 7-year-old S. alterniflora; SA12, 12-year-old S. alterniflora.
PICRUSt was employed to analyze the functional content of bacterial colonies in S. alterniflora invasion soil of different ages. However, no significant differences were observed among the five groups at KEGG level 1 and KEGG level 2 (p > 0.05) (Figure 6).
Figure 6. The different functional categories predicted by PICRUSt for the bacterial colonies. (a) KEGG levels 1; (b) KEGG levels 2. BM, bare mudflat; SA1, 1-year-old S. alterniflora; SA4, 4-year-old S. alterniflora; SA7, 7-year-old S. alterniflora; SA12, 12-year-old S. alterniflora.
Previous studies have shown that plant invasion can significantly change soil physicochemical characteristics (e.g., soil salinity, pH, and moisture) (Yuan et al., 2015); however, the effects are not constant (Souza-Alonso et al., 2015). In this study, soil moisture changed in an orderly manner and increased in the surface soil (0–10 cm) with the increasing age of invasion (Table 1). The increase in soil moisture following the invasion of S. alterniflora can be attributed to its extensive root system, which enhances water retention capacity in the soil. Additionally, the dense canopy formed by S. alterniflora reduced evaporation rates, further contributing to higher soil moisture. Yang et al. (2020) also found that soil moisture increased significantly after the invasion of S. alterniflora. Soil pH varies with the invasion of S. alterniflora and may increase (Gao et al., 2017), decrease (Yang et al., 2019; Zhang et al., 2020; Lin et al., 2021), or not be altered (Huang et al., 2016) by S. alterniflora invasion, which may be attributed to soil types and invasion history (Carey et al., 2015). In our study, pH significantly decreased in the surface soil after S. alterniflora invasion for approximately 12 years, whereas it significantly increased in the middle and subsoil layers after S. alterniflora invasion for approximately 7 years, and then decreased or remained unaffected after S. alterniflora invasion for approximately 12 years (Table 1). The soil physicochemical characteristics at different soil depths also showed inconsistent responses during invasion (Gao et al., 2017; Zhang et al., 2020).
The spatial and temporal distribution of phosphorus is a key index for assessing the risk, productivity, and function of wetland ecosystems (Cui et al., 2019). In this study, 12-year-old S. alterniflora invasion significantly enhanced the AP concentration (Table 1). This may be due to microbial decomposers related to S. alterniflora roots (Wang et al., 2021). The TN concentration increased with S. alterniflora invasion age, and there was no significant difference among soil depths (Table 1), which is in accordance with other coastal wetlands (Qi et al., 2019; Lin et al., 2021; Zhang et al., 2023). This may illustrate that S. alterniflora invasion plays a critical role in the soil nitrogen cycle. S. alterniflora can increase nitrogen distribution during photosynthesis and, thus, stimulate carbon and nutrient accumulation in the soil (Wang et al., 2018; Chen et al., 2018; Hou et al., 2019; Ge et al., 2020). Zhang et al. (2020) reported that S. alterniflora invasion increased SOC linearly with invasion age. SOC decreased at the initial invasion age of S. alterniflora and then increased with increasing invasion age at any soil depth (Table 1). The biodegradable carbon in S. alterniflora litter had a higher litter decomposition rate and induced more SOC (Huang et al., 2022; Liu et al., 2022). However, S. alterniflora invasion had no significant effect on TC (Table 1). This may be related to the invasion age, as plant invasion age is also a crucial element in determining the supply of nutrients in the soil (Souza-Alonso et al., 2015; Yang et al., 2023).
Alterations in the diversity of soil bacterial communities are closely correlated with the multifunctionalities of the ecosystem (Jing et al., 2015). Compared to the bare mudflats, S. alterniflora invasion significantly increased soil bacterial abundance and diversity (Yang et al., 2016; Rodríguez-Caballero et al., 2017; Yang et al., 2019; Lin et al., 2022). Interestingly, alpha diversity indices (Chao1, ACE, and Shannon) were not greatly affected by S. alterniflora invasion in this study (Table 2), suggesting that S. alterniflora invasion had no significant effect on soil bacterial alpha diversity. Soil bacterial diversity has varied in different studies. Other researchers have found that the Shannon index decreased after S. alterniflora invasion (Gao et al., 2019; Li et al., 2020). Carey et al. (2015) showed that there was a minor response in bacterial community composition and diversity after 5–6 years of Aegilops triuncialis and Euphorbia caput-medusae invasion in field experiments, although the soil characteristics changed. This discrepancy may be explained by the invasion stage (Liu et al., 2017), soil C and N contents, nutrient availability (Lin et al., 2022), and climatic conditions (Li et al., 2020). Soil microorganisms play crucial roles in maintaining the balance and stability of essential processes of nutrient cycling of soil; thus, the stable microbial structures and functions in soil are beneficial for the stability of coastal wetland ecosystems (Ali and Xie, 2020).
Compared to the bare mudflat, the relative abundances of Flavobacteriia and Bacteroidia, which are affiliated with Bacteroidetes, were higher in S. alterniflora invasive soil (Figure 3). Bacteroidetes are ubiquitous in anaerobic environments (Xu et al., 2017) and are generally considered as functional groups for decomposing complex organic compounds (Fernandez-Gomez et al., 2013). Moreover, S. alterniflora litter contained more recalcitrant substances, such as lignocellulosic and lignin materials (Zhang et al., 2010), which increased the relative abundance of Bacteroidetes, thus enhancing the degradation of S. alterniflora residues and promoting the accumulation of TN in the soil (Table 1). Likewise, Lin et al. (2022) reported that the relative abundances of Bacteroidetes and Firmicutes commonly increased as the invasion age of S. alterniflora increased. Proteobacteria was the predominant phylum in all soil bacterial communities in the wetland ecosystem (Figure 3) and has been broadly recognized in other studies (Liu et al., 2017; Lin et al., 2022). Among Proteobacteria, Gamma- and Delta-Proteobacteria were the two primary classes, which is in line with other reports (Zheng et al., 2019; Lin et al., 2022). Anaerolineae can respire multiple carbon compounds (Hug et al., 2013) and increased after S. alterniflora invasion of a salt marsh in China (Yang et al., 2020). However, this disagrees with our study, and we found that the relative abundance of Anaerolineae decreased after S. alterniflora invasion (Supplementary Table S2). The decrease in the relative abundance of Anaerolineae after S. alterniflora invasion may be due to changes in soil redox conditions. As S. alterniflora increased soil aeration through its dense root system, the soil became more aerobic, which was less favorable for anaerobic bacteria like Anaerolineae (Li et al., 2020; Dai et al., 2024). The relative abundances of the top 10 classes increased progressively with S. alterniflora invasion age (Supplementary Table S2). SOC accumulation in the invaded wetlands, along with invasion age, was beneficial for increasing the relative abundance of bacteria (Liu et al., 2017). Although soil bacterial alpha diversity was not significantly altered with the soil depth (Table 2), the relative abundance of Gammaproteobacteria, Alphaproteobacteria, Chloroplast, Clostridia, and Bacilli altered with soil depth (Figure 3). As the physicochemical characteristics of the deep soil layer changed (Table 1), the bacterial community composition can alter with the changed environmental characteristics (Song et al., 2022). In addition to the observed changes in soil bacterial community composition, the invasion of S. alterniflora also significantly impacts the beta diversity of bacterial communities. Beta diversity, which reflects the variation in community composition among different sites, is often altered by plant invasions due to changes in environmental conditions and resource availability (Zhang et al., 2020; Yang et al., 2016). In the context of S. alterniflora invasion, previous studies have shown that the beta diversity of soil bacterial communities tends to increase with the progression of invasion, likely due to the enhanced heterogeneity in soil physicochemical properties and the introduction of new organic substrates from plant litter (Yang et al., 2016; Rodríguez-Caballero et al., 2017).
Soil physicochemical characteristics are widely recognized as one of the key driving elements affecting soil bacterial community composition and structure (Nguyen et al., 2018; Rath et al., 2019). In this study, we found that pH, TP, TN, CAT, and UE were the predominant factors influencing the relative abundance of bacteria in the surface soil (Figure 4A). Likewise, TP, TN, and pH are vital environmental factors that regulate the sediment bacterial community structure after S. alterniflora invasion (Song et al., 2022). pH was significantly negatively correlated with Alphaproteobacteria in the surface soil, but positively correlated with Chloroplast in deep soil (Figure 4). The results suggested that Alphaproteobacteria may thrive in more neutral conditions, which aligns with previous studies showing that Alphaproteobacteria are often abundant in low-pH environments. pH is an important factor affecting soil bacterial community composition in various soil types (Lauber et al., 2009; Tripathi et al., 2012; Ding et al., 2017). UE catalyzes the hydrolysis of carbon–nitrogen bonds between some amides and urea (Madejón et al., 2001) and is thus closely related to organic matter turnover and transformations (Baddam et al., 2016). UE activity gradually increased with S. alterniflora invasion age and peaked in the 7-year-old S. alterniflora soil (Figure 2). UE activity was significantly positively correlated with Bacilli, Anaerolineae, and Deltaproteobacteria, and negatively correlated with Acidimicrobiia (Figure 4). RDA illustrated that changes in the soil bacterial community composition were closely related to soil TP, C/N, TC, and CAT (Figure 5). P is the primary limiting factor of microbial growth (Sun et al., 2019; Wang et al., 2021). Likewise, TC and TN play major roles in the regulation of bacterial community structure in coastal wetlands (Lin et al., 2022).
The observed shifts in bacterial community composition, particularly the increased abundance of Chloroplast and Alphaproteobacteria, may be driven by root exudates from S. alterniflora, which can alter soil nutrient availability and pH (Zeleke et al., 2013). These exudates often contain organic compounds that selectively promote the growth of certain bacterial groups while inhibiting others. Over the long term, such changes in bacterial communities could enhance nutrient cycling and organic matter decomposition, potentially leading to increased soil fertility (Wang et al., 2024). However, they may also disrupt the balance of native microbial communities, affecting overall ecosystem stability and function. Here, we found that S. alterniflora invasion significantly altered soil properties such as pH, moisture, and nutrient availability, which, in turn, shaped the structure and function of bacterial communities. For instance, the increase in soil moisture and EC likely created more favorable conditions for certain bacterial taxa, such as Gammaproteobacteria and Deltaproteobacteria, which were known for their roles in nutrient cycling and organic matter decomposition. Additionally, changes in SOC and TP content may influence microbial metabolic pathways, as indicated by shifts in bacterial functional groups (Xu et al., 2025). These interconnected changes suggested that S. alterniflora invasion not only affects individual soil parameters but also restructured the soil microbiome in ways that can have cascading effects on ecosystem processes.
Our findings revealed that S. alterniflora invasion significantly impacted soil properties and microbial communities in coastal wetlands, with implications for conservation and management. The invasion altered soil physicochemical characteristics, increasing soil moisture, SOC, EC, and AP, among others. While these changes may temporarily enhance soil fertility, they can lead to long-term wetland degradation through changed biological community and altered nutrient dynamics. Management efforts should prioritize early detection and control of S. alterniflora, especially in areas with intact native plant communities. Additionally, shifts in microbial community composition, such as increased abundance of Chloroplast and Alphaproteobacteria, highlight S. alterniflora’s potential to disrupt ecosystem functions through changes in nutrient cycling. To counteract these effects, management strategies should be taken to control S. alterniflora, such as biological control agents, or promote native plants to support diverse and stable microbial communities. Moreover, our results emphasized the importance of considering soil depth in assessing invasion impacts, as deeper soil layers may respond differently compared to surface soils.
The present study illustrated that S. alterniflora invasion had no significant impact on soil bacterial alpha diversity but shifted the bacterial community composition, and the bacterial community composition changed with soil depths. TP, TC, C/N, and CAT were the primary limiting factors for bacterial community composition. The invasion of S. alterniflora modified the soil physicochemical characteristics at different soil depths, particularly by increasing soil moisture, EC, AP, and TN. However, characteristics at all soil depths showed unstable responses during the invasion process. Compared with the bare mudflat, UE activity first decreased and then increased with S. alterniflora invasion age. CAT and SC activity gradually increased with the invasion age of S. alterniflora. The 7-year-old S. alterniflora invasive soil had a better nutrient status. These results provide a comprehensive understanding of the effects of S. alterniflora invasion on coastal wetland ecosystems.
The original contributions presented in the study are included in the article/Supplementary Material. Further inquiries can be directed to the corresponding author.
LY: Data curation, Investigation, Validation, Writing – original draft, Writing – review & editing. YP: Data curation, Investigation, Writing – original draft. SW: Data curation, Formal analysis, Visualization, Writing – original draft. CR: Data curation, Investigation, Writing – original draft. HD: Data curation, Project administration, Writing – original draft. HL: Software, Supervision, Writing – original draft. BG: Conceptualization, Formal analysis, Supervision, Writing – original draft, Writing – review & editing.
The author(s) declare financial support was received for the research, authorship, and/or publication of this article. The work was supported by the Natural Science Foundation of Jiangsu Province (BK20211363 and BK20230718), the Natural Science Foundation of Yancheng (YCBK2023013), and the Marine Science and Technology Special Project of Jiangsu Province (JSZRHYKJ202211). Yancheng Teachers University Educational Reform Project (2023YCTCJGZ13).
The authors declare that the research was conducted in the absence of any commercial or financial relationships that could be construed as a potential conflict of interest.
The author(s) declare that no Generative AI was used in the creation of this manuscript.
All claims expressed in this article are solely those of the authors and do not necessarily represent those of their affiliated organizations, or those of the publisher, the editors and the reviewers. Any product that may be evaluated in this article, or claim that may be made by its manufacturer, is not guaranteed or endorsed by the publisher.
The Supplementary Material for this article can be found online at: https://www.frontiersin.org/articles/10.3389/fmars.2025.1531902/full#supplementary-material
Ali S., Xie L. (2020). Plant growth promoting and stress mitigating abilities of soil born microorganisms. Recent Patents Food Nutr. Agric. 11, 96–104. doi: 10.2174/2212798410666190515115548
An S. U., Cho H., Jung U. J., Kim B., Lee H., Hyun J. H. (2020). Invasive Spartina anglica greatly alters the rates and pathways of organic carbon oxidation and associated microbial communities in an intertidal wetland of the Han River Estuary, Yellow Sea. Front. Mar. Sci. 7, 1–15. doi: 10.3389/fmars.2020.00059
Avery B. W., Bascomb C. L. (1982). Soil survey laboratory methods (Harpenden, Hertfordshire: Rothamsted Experimental Station, UK).
Baddam R., Reddy G. B., Raczkowski C., Cyrus J. S. (2016). Activity of soil enzymes in constructed wetlands treated with swine wastewater. Ecol. Eng. 91, 24–30. doi: 10.1016/j.ecoleng.2016.01.021
Card S. M., Quideau S. A. (2010). Microbial community structure in restored riparian soils of the Canadian prairie pothole region. Soil Biol. Biochem. 42, 1463–1471. doi: 10.1016/j.soilbio.2010.05.010
Carey C. J., Beman J. M., Eviner V. T., Malmstrom C. M., Hart S. C. (2015). Soil microbial community structure is unaltered by plant invasion, vegetation clipping, and nitrogen fertilization in experimental semi-arid grasslands. Front. Microbiol. 6, 466. doi: 10.3389/fmicb.2015.00466
Chen L., Gao J., Zhu Q., Wang Y., Yang Y. (2018). Accumulation and output of heavy metals by the invasive plant Spartina alterniflora in a coastal salt marsh. Pedosphere 28, 884–894. doi: 10.1016/S1002-0160(17)60369-2
Cui H., Ou Y., Wang L. X., Wu. H. T., Yan B. X., Li Y. X. (2019). Distribution and release of phosphorus fractions associated with soil aggregate structure in restored wetlands. Chemosphere 223, 319–329. doi: 10.1016/j.chemosphere.2019.02.046
Dai Z., Zhang N., Wang F., Li Y., Peng J., Xiang T., et al. (2024). Loss of microbial functional diversity following Spartina alterniflora invasion reduces the potential of carbon sequestration and nitrogen removal in mangrove sediments - from a gene perspective. J. Environ. Manage. 365, 121569. doi: 10.1016/j.jenvman.2024.121569
Davidson I. C., Cott G. M., Devaney J. L., Simkanin C. (2018). Differential effects of biological invasions on coastal blue carbon: A global review and meta-analysis. Global Change Biol. 24, 5218–5230. doi: 10.1111/gcb.2018.24.issue-11
Deng Q., Cheng X. L., Hui D. F., Zhang Q., Li M., Zhang Q. F. (2016). Soil microbial community and its interaction with soil carbon and nitrogen dynamics following afforestation in central China. Sci. Total Environ. 541, 230–237. doi: 10.1016/j.scitotenv.2015.09.080
Ding L. J., Su J. Q., Li H., Zhu Y. G., Cao Z. H. (2017). Bacterial succession along a long-term chronosequence of paddy soil in the Yangtze River delta, China. Soil Biol. Biochem. 104, 59–67. doi: 10.1016/j.soilbio.2016.10.013
Fernandez-Gomez B., Richter M., Schuler M., Pinhassi J., Acinas S. G., Gonzalez J. M., et al. (2013). Ecology of marine bacteroidetes: a comparative genomics approach. ISME J. 7, 1026–1037. doi: 10.1038/ismej.2012.169
Freedman Z., Zak D. R. (2015). Soil bacterial communities are shaped by temporal and environmental filtering: evidence from a long-term chronosequence. Environ. Microbiol. 17, 3208–3218. doi: 10.1111/emi.2015.17.issue-9
Gao D. Z., Li X. F., Lin X. B., Wu D. M., Jin B. S., Huang Y. P., et al. (2017). Soil dissimilatory nitrate reduction processes in the Spartina alterniflora invasion chronosequences of a coastal wetland of southeastern China: dynamics and environmental implications. Plant Soil 421, 383–399. doi: 10.1007/s11104-017-3464-x
Gao G. F., Li P. F., Zhong J. X., Shen Z. J., Chen J., Li Y. T., et al. (2019). Spartina alterniflora invasion alters soil bacterial communities and enhances soil N2O emissions by stimulating soil denitrification in mangrove wetland. Sci. Total Environ. 653, 231–240. doi: 10.1016/j.scitotenv.2018.10.277
Ge B., Jiang S., Liu Q., Zhou C., Tang B. (2019). Density, but not distribution pattern of Assiminea latericea varies on tidal flats with smooth cordgrass Spartina alterniflora invasion stage. Regional Stud. Mar. Sci. 27, 100528. doi: 10.1016/j.rsma.2019.100528
Ge B. M., Jiang S. H., Yang L., Zhang H. B., Tang B. P. (2020). Succession of macrofaunal communities and environmental properties along a gradient of smooth cordgrass Spartina alterniflora invasion stages. Mar. Environ. Res. 156, 104862. doi: 10.1016/j.marenvres.2019.104862
Guo X. P., Lu D. P., Niu Z. S., Feng J. N., Chen Y. R., Tou F. Y., et al. (2018). Bacterial community structure in response to environmental impacts in the intertidal sediments along the Yangtze Estuary, China. Mar. pollut. Bull. 126, 141–149. doi: 10.1016/j.marpolbul.2017.11.003
Hammer Ø., Harper D. A. T., Ryan P. D. (2001). PAST: paleontological statistics software package for education and data analysis. Palaeontologia Electronica 4, 1–9. Available online at: http://palaeo-electronica.org/2001_1/past/issue1_01.htm.
He S., Lin J., Liu X., Jia S., Chen S. (2023). Cordgrass Spartina alterniflora acts as a key carbon source to support macrozoobenthos in the salt marsh and nearby mudflat communities. Ecol. Indic. 148, 110052. doi: 10.1016/j.ecolind.2023.110052
Hou X. L., Han H., Tigabu M., Cai L. P., Meng F. R., Liu A. Q., et al. (2019). Changes in soil physico-chemical properties following vegetation restoration mediate bacterial community composition and diversity in Changting, China. Ecol. Eng. 138, 171–179. doi: 10.1016/j.ecoleng.2019.07.031
Huang J. X., Xu X., Wang M., Nie M., Qiu S. Y., Wang Q., et al. (2016). Responses of soil nitrogen fixation to Spartina alterniflora invasion and nitrogen addition in a Chinese salt marsh. Sci. Rep. 6, 20384. doi: 10.1038/srep20384
Huang X., Duan Y. T., Tao Y. H., Wang X. P., Long H. L., Luo C. S., et al. (2022). Effects of Spartina alterniflora invasion on soil organic carbon storage in the Beihai Coastal Wetlands of China. Front. Mar. Sci. 9, 890811. doi: 10.3389/fmars.2022.890811
Hug L. A., Castelle C. J., Wrighton K. C., Thomas B. C., Sharon I., Frischkorn K. R., et al. (2013). Community genomic analyses constrain the distribution of metabolic traits across the Chloroflexi phylum and indicate roles in sediment carbon cycling. Microbiome 1, 1–17. doi: 10.1186/2049-2618-1-22
Jing X., Sanders N. J., Shi Y., Chu H. Y., Classen A. T., Zhao K., et al. (2015). The links between ecosystem multifunctionality and above- and belowground biodiversity are mediated by climate. Nat. Commun. 6, 8159. doi: 10.1038/ncomms9159
Jurburg S. D., Natal-da-Luz T., Raimundo J., Morais P. V., Sousa J. P., Elsas J. D., et al. (2018). Bacterial communities in soil become sensitive to drought under intensive grazing. Sci. Total Environ. 618, 1638–1646. doi: 10.1016/j.scitotenv.2017.10.012
Kao-Kniffin J., Balser T. C. (2008). Soil fertility and the impact of exotic invasion on microbial communities in Hawaiian forests. Microbial Ecol. 56, 55–63. doi: 10.1007/s00248-007-9323-1
Kim S., Yu C., Ruesink J., Hong J. S. (2023). Vertical distribution of the salt marsh invader Spartina alterniflora and native halophytes on the west coast of Korea in relation to tidal regimes. Aquat. Invasions 18, 331–349. doi: 10.3391/ai.2023.18.3.104556
Langille M. G. I., Zaneveld J., Caporaso J. G., McDonald D., Knights D., Reyes J. A., et al. (2013). Predictive functional profiling of microbial communities using 16S rRNA marker gene sequences. Nat. Biotechnol. 31, 814–821. doi: 10.1038/nbt.2676
Lauber C. L., Hamady M., Knight R., Fierer N. (2009). Pyrosequencing-based assessment of soil pH as a predictor of soil bacterial community structure at the continental scale. Appl. Environ. Microbiol. 75, 5111–5120. doi: 10.1128/AEM.00335-09
Li Y. X., Laborda P., Xie X. L., Zhou R., Chen Y., Li T., et al. (2020). Spartina alterniflora invasion alters soil microbial metabolism in coastal wetland of China. Estuarine Coast. Shelf Sci. 245, 106982. doi: 10.1016/j.ecss.2020.106982
Liao C. Z., Luo Y. Q., Jiang L. F., Zhou X. H., Wu X. W., Fang C. M., et al. (2007). Invasion of Spartina alterniflora enhanced ecosystem carbon and nitrogen stocks in the Yangtze Estuary, China. Ecosystems 10, 1351–1361. doi: 10.1007/s10021-007-9103-2
Lin G. M., He Y. N., Lu J. G., Chen H., Feng J. X. (2021). Seasonal variations in soil physicochemical properties and microbial community structure influenced by Spartina alterniflora invasion and Kandelia obovata restoration. Sci. Total Environ. 797, 149213. doi: 10.1016/j.scitotenv.2021.149213
Lin Y. X., Hu H. W., Yang P., Ye G. P. (2022). Spartina alterniflora invasion has a greater impact than non-native species, Phragmites australis and Kandelia obovata, on the bacterial community assemblages in an estuarine wetland. Sci. Total Environ. 822, 153517. doi: 10.1016/j.scitotenv.2022.153517
Ling N., Chen D. M., Guo H., Wei J. X., Bai Y. F., Shen Q. R., et al. (2017). Differential responses of soil bacterial communities to long-term N and P inputs in a semi-arid steppe. Geoderma 292, 25–33. doi: 10.1016/j.geoderma.2017.01.013
Liu J. Q., Wang W. Q., Shen L. D., Yang Y. L., Xu J. B., Tian M. H., et al. (2022). Response of methanotrophic activity and community structure to plant invasion in China’s coastal wetlands. Geoderma 407, 115569. doi: 10.1016/j.geoderma.2021.115569
Liu M., Yu Z., Yu X., Xue Y., Huang B., Yang J. (2017). Invasion by cordgrass increases microbial diversity and alters community composition in a mangrove nature reserve. Front. Microbiol. 8, 2503. doi: 10.3389/fmicb.2017.02503
Madejón E., Burgos P., López R., Cabrera F. (2001). Soil enzymatic response to addition of heavy metals with organic residues. Biol. Fertility Soils 34, 144–150. doi: 10.1007/s003740100379
Meng W., Feagin R. A., Innocenti R. A., Hu B., He M., Li H. (2020). Invasion and ecological effects of exotic smooth cordgrass Spartina alterniflora in China. Ecol. Eng. 143, 105670. doi: 10.1016/j.ecoleng.2019.105670
Nguyen L. T. T., Osanai Y., Lai K., Anderson I. C., Bange M. P., Tissue D. T., et al. (2018). Responses of the soil microbial community to nitrogen fertilizer regimes and historical exposure to extreme weather events: flooding or prolonged-drought. Soil Biol. Biochem. 118, 227–236. doi: 10.1016/j.soilbio.2017.12.016
Nie S. Q., Mo S. M., Gao T. W., Yan B., Shen P. H., Kashif M., et al. (2023). Coupling effects of nitrate reduction and sulfur oxidation in a subtropical marine mangrove ecosystem with Spartina alterniflora invasion. Sci. Total Environ. 862, 160930. doi: 10.1016/j.scitotenv.2022.160930
Nirmala L., Reghu R. J., Santhosh R. S., Sugathan S., Chithra A. A. K., Sophy A. J. K. (2023). Plant invasion by Chromolaena odorata alters the soil microbiome and provides insight into the role of copiotrophs. Ecol. Genet. Genomics 26, 100157. doi: 10.1016/j.egg.2022.100157
Qi X., Liu H., Lin Z., Liu X., Gong H. (2019). Impacts of age and expansion direction of invasive Spartina alterniflora on soil organic carbon dynamics in coastal salt marshes along eastern China. Estuaries Coasts 42, 1858–1867. doi: 10.1007/s12237-019-00611-4
R Core Team. (2022). R: A language and environment for statistical computing. R Foundation for Statistical Computing, Vienna, Austria. Available online at: https://www.R-project.org/.
Rath K. M., Fierer N., Murphy D. V., Rousk J. (2019). Linking bacterial community composition to soil salinity along environmental gradients. ISME J. 13, 836–846. doi: 10.1038/s41396-018-0313-8
Rodríguez-Caballero C. F., Alguacil M. M., Fernández-López M., Fernández-González A. J., Roldán A. (2017). Striking alterations in the soil bacterial community structure and functioning of the biological N cycle induced by Pennisetum setaceum invasion in a semiarid environment. Soil Biol. Biochem. 109, 176–187. doi: 10.1016/j.soilbio.2017.02.012
Sokol N. W., Slessarev E., Marschmann G. L., Nicolas A., Blazewicz S. J., Brodie E. L., et al. (2022). Life and death in the soil microbiome: how ecological processes influence biogeochemistry. Nat. Rev. Microbiol. 20, 415–430. doi: 10.1038/s41579-022-00695-z
Song L., Wang Q. X., Zhu Y. J., Christakos G., Wu J. P. (2022). Changes to the structure and function of microbial communities in Spartina alterniflora and Kandelia obovata sediments as a factor of stand age. Appl. Soil Ecol. 177, 104544. doi: 10.1016/j.apsoil.2022.104544
Souza-Alonso P., Guisande-Collazo A., González L. (2015). Gradualism in Acacia dealbata Link invasion: impact on soil chemistry and microbial community over a chronological sequence. Soil Biol. Biochem. 80, 315–323. doi: 10.1016/j.soilbio.2014.10.022
Sun H., Jiang J., Cui L., Feng W., Wang Y., Zhang J. (2019). Soil organic carbon stabilization mechanisms in a subtropical mangrove and salt marsh ecosystems. Sci. Total Environ. 673, 502–510. doi: 10.1016/j.scitotenv.2019.04.122
Thatoi H., Behera B., Mishra R., Dutta S. (2013). Biodiversity and biotechnological potential of microorganisms from mangrove ecosystems: a review. Ann. Microbiol. 63, 1–19. doi: 10.1007/s13213-012-0442-7
Tripathi B. M., Kim M., Singh D., Lee-Cruz L., Lai-Hoe A., Ainuddin A. N., et al. (2012). Tropical soil bacterial communities in Malaysia: pH dominates in the equatorial tropics too. Microbial Ecol. 64, 474–484. doi: 10.1007/s00248-012-0028-8
Wang Q., Li J., Chen J., Hong H., Lu H., Liu J., et al. (2018). Glomalin-related soil protein deposition and carbon sequestration in the old Yellow River delta. Sci. Total Environ. 625, 619–626. doi: 10.1016/j.scitotenv.2017.12.303
Wang K., Wang S., Zhang X., Wang W., Li F., Dong L., et al. (2023). Potential ecological impacts of physical control on Spartina alterniflora in coastal wetland: Migration and transformation of nutrients and the response of bacterial community structure. J. Cleaner Production 398, 136556. doi: 10.1016/j.jclepro.2023.136556
Wang Y. F., Xu J. Y., Liu Z. L., Cui H. L., Chen P., Cai T. G., et al. (2024). Biological interactions mediate soil functions by altering rare microbial communities. Environ. Sci. Technol. 58, 5866–5877. doi: 10.1021/acs.est.4c00375
Wang L., Yuan J. H., Wang Y., Butterly C. R., Tong D. L., Zhou B., et al. (2021). Effects of exotic Spartina alterniflora invasion on soil phosphorus and carbon pools and associated soil microbial community composition in coastal wetlands. ACS Omega 6, 5730–5738. doi: 10.1021/acsomega.0c06161
Xu S., Lu W. J., Liu Y. T., Ming Z. Y., Liu Y. J., Meng R. H., et al. (2017). Structure and diversity of bacterial communities in two large sanitary landfills in China as revealed by high-throughput sequencing (MiSeq). Waste Manage. 63, 41–48. doi: 10.1016/j.wasman.2016.07.047
Xu S., Na M., Huang Y., Zhang J., Zhou J., Li L. J. (2025). Changes in microbial carbon cycling functions along rice cultivation chronosequences in saline-alkali soils. Soil Biol. Biochem. 202, 109699. doi: 10.1016/j.soilbio.2024.109699
Yang W., Cai A. D., Wang J. S., Luo Y. Q., Cheng X. L., An S. Q. (2020). Exotic Spartina alterniflora Loisel. invasion significantly shifts soil bacterial communities with the successional gradient of saltmarsh in eastern China. Plant Soil 449, 97–115. doi: 10.1007/s11104-020-04470-y
Yang W., Jeelani N., Leng X., Cheng X., An S. Q. (2016). Spartina alterniflora invasion alters soil microbial community composition and microbial respiration following invasion chronosequence in a coastal wetland of China. Sci. Rep. 6, 26880. doi: 10.1038/srep26880
Yang L., Shi J. Y., Pan R., Yang R. P., Li H. S., Wang S., et al. (2023). Distribution of mudsnail Bullacta caurina along smooth cordgrass Spartina alterniflora invasion stages on a coast of the Yellow Sea, China. Mar. Environ. Res. 192, 106248. doi: 10.1016/j.marenvres.2023.106248
Yang W., Jeelani N., Xia L., Zhu Z.H., Luo Y. Q., Cheng X. L., et al. (2019). Soil fungal communities vary with invasion by the exotic Spartina alternifolia Loisel. in coastal salt marshes of eastern China. Plant Soil 442, 215–232. doi: 10.1007/s11104-019-04184-w
Yuan J. J., Ding W. X., Liu D. Y., Kang H., Freeman C., Xiang J., et al. (2015). Exotic Spartina alterniflora invasion alters ecosystem–atmosphere exchange of CH4 and N2O and carbon sequestration in a coastal salt marsh in China. Global Change Biol. 21, 1567–1580. doi: 10.1111/gcb.2015.21.issue-4
Zeleke J., Sheng Q., Wang J. G., Huang M. Y., Xia F., Wu J. H., et al. (2013). Effects of Spartina alterniflora invasion on the communities of methanogens and sulfate-reducing bacteria in estuarine marsh sediments. Front. Microbiol. 4, 243. doi: 10.3389/fmicb.2013.00243
Zhang X. H., Bai J. L., Zhang Z. S., Xie T., Zhang G. L., Liu Y., et al. (2023). Plant invasion strengthens the linkages between dissolved organic matter composition and the microbial community in coastal wetland soils. Catena 198, 107449. doi: 10.1016/j.catena.2023.107449
Zhang G. L., Bai J. H., Zhao Q. Q., Jia J., Wang W., Wang X. (2020). Bacterial succession in salt marsh soils along a short-term invasion chronosequence of Spartina alterniflora in the Yellow River estuary China. Microbial Ecol. 79, 644–661. doi: 10.1007/s00248-019-01430-7
Zhang G. L., Bai J. H., Zhao Q. Q., Jia J., Wang X., Wang W., et al. (2021). Soil carbon storage and carbon sources under different Spartina alterniflora invasion periods in a salt marsh ecosystem. Catena 196, 104831. doi: 10.1016/j.catena.2020.104831
Zhang Y., Ding W., Luo J., Donnison A. (2010). Changes in soil organic carbon dynamics in an eastern Chinese coastal wetland following invasion by a C4 plant Spartina alterniflora. Soil Biol. Biochem. 42, 1712–1720. doi: 10.1016/j.soilbio.2010.06.006
Zhao S., Wang T., Li X., Xu H., Liu N., Xu A., et al. (2023). Comparing different management strategies of river mouths for waterbird conservation: A case study along the Yellow Sea coast. Global Ecol. Conserv. 47, e02659. doi: 10.1016/j.gecco.2023.e02659
Keywords: plant invasion, microbial community, coastal wetland, invasion age, biodiversity
Citation: Yang L, Peng Y, Wang S, Rong C, Dong H, Li H and Ge B (2025) Soil bacterial community composition but not alpha diversity altered along a gradient of Spartina alterniflora invasion on the coast of Yellow Sea, China. Front. Mar. Sci. 12:1531902. doi: 10.3389/fmars.2025.1531902
Received: 21 November 2024; Accepted: 03 February 2025;
Published: 28 February 2025.
Edited by:
Meilin Wu, Chinese Academy of Sciences (CAS), ChinaReviewed by:
Gaoling Shi, Jiangsu Academy of Agricultural Sciences (JAAS), ChinaCopyright © 2025 Yang, Peng, Wang, Rong, Dong, Li and Ge. This is an open-access article distributed under the terms of the Creative Commons Attribution License (CC BY). The use, distribution or reproduction in other forums is permitted, provided the original author(s) and the copyright owner(s) are credited and that the original publication in this journal is cited, in accordance with accepted academic practice. No use, distribution or reproduction is permitted which does not comply with these terms.
*Correspondence: Baoming Ge, Z2VibUB5Y3R1LmVkdS5jbg==
Disclaimer: All claims expressed in this article are solely those of the authors and do not necessarily represent those of their affiliated organizations, or those of the publisher, the editors and the reviewers. Any product that may be evaluated in this article or claim that may be made by its manufacturer is not guaranteed or endorsed by the publisher.
Research integrity at Frontiers
Learn more about the work of our research integrity team to safeguard the quality of each article we publish.