- 1Consolidated Safety Services, Inc., Fairfax, VA, United States
- 2National Centers for Coastal Ocean Science, National Oceanic and Atmospheric Administration, Beaufort, NC, United States
- 3U.S. Geological Survey, Pacific Coastal and Marine Science Center, Santa Cruz, CA, United States
- 4Earth Resources Technology (ERT) Inc. Contractor, Restoration Center, National Oceanic and Atmospheric Administration, Aguadilla, Puerto Rico
Coastal resilience has become a pressing global issue due to the growing vulnerability of coastlines to the effects of climate change. Nature-based solutions have emerged as a promising approach to coastal protection to not only enhance coastal resilience, but also restore critical ecosystems. Coral reef restoration has the potential to provide ecosystem services benefits; however, there are still key uncertainties in linking restoration design to reductions in coastal flood hazard under current and future climate conditions. In this study, we applied one-dimensional and two-dimensional numerical coastal engineering models, calibrated and validated using field data, to evaluate the effectiveness of coral restoration scenarios on coastal waves, water levels, and flooding along the coast of San Juan, Puerto Rico, U.S.A. Model results indicate a small reduction in maximum water levels under the proposed restoration scenarios. This underscores the importance of these endeavors, not only for ecological preservation but also for preventing further reef deterioration. Such preservation is essential for mitigating the increased coastal risks anticipated in the future. Results from this study provide information to guide policymakers and coastal managers in making informed decisions on viable restoration project design options. By systematically evaluating how restoration location impacts coastal flood hazards, communities can develop and implement proactive strategies to mitigate flood-related risk. In addition, by restoring coral reefs, communities can contribute to environmental preservation while ensuring sustainable development and protection of coastal environments.
1 Introduction
Coral reefs are one of the most biodiverse ecosystems and provide valuable ecosystem services such as fisheries habitat, tourism opportunities, and shoreline protection (Burke et al., 2011). Acting as natural barriers, they absorb and dissipate wave energy, offering protection to adjacent coastal communities and infrastructure from storm surges and flooding (Ferrario et al., 2014; Reguero et al., 2021). However, coral reefs are facing major challenges, including coral disease and bleaching (Eakin et al., 2018), climate change (Hoegh-Guldberg et al., 2007), and anthropogenic stressors (Hughes et al., 2017), which significantly increase the vulnerability of coastal areas to wave-driven flooding and erosion (e.g., Elliff and Silva, 2017; Reguero et al., 2018; Storlazzi et al., 2019).
Coastal flooding, driven by extreme events, has become a growing concern for vulnerable coastal communities (IPCC, 2012). The increasing frequency and intensity of extreme events, along with accelerating sea-level rise (SLR) due to climate change, are exacerbating the risks of coastal flooding (Kirezci et al., 2020; Sweet et al., 2022) and associated socio-economic impacts (Hallegatte et al., 2013; Vitousek et al., 2017). This flooding also leads to environmental degradation, such as erosion of coastal habitats and pollution of marine ecosystems (e.g., IPCC, 2014; Neumann et al., 2015). In response, there is growing interest in effective and sustainable measures to mitigate coastal flood risks and enhance resilience of vulnerable coastal communities. Nature-based solutions (NbS) are one promising approach to reduce these risks (Temmerman et al., 2013; Elko et al., 2021). NbS practices involve the strategic use of natural systems to minimize risk, such as enhancing wetlands or restoring mangroves to bolster coastal resilience. These modifications are coupled with the introduction of native biological components through human intervention or accelerated succession (Perricone et al., 2023).
Coral reef restoration can act as a NbS by reducing the risks of wave-driven run-up, coastal flooding, and erosion by dissipating wave energy through modifications of the bathymetry and seabed roughness, while concurrently enhancing ecological stability (Viehman et al., 2023). Reef restoration can also provide environmental, economic, and social benefits (Spalding et al., 2017), which have been the focus of most restoration efforts (Bayraktarov et al., 2019). Recent studies have demonstrated that hybrid coral reef restoration can significantly reduce flood risk by minimizing economic losses and saving lives, particularly in low-lying coastal areas where vulnerable communities are most at risk (Storlazzi et al., 2025). Despite the increasing interest in using coral restoration as a means of reducing flood risk, there remain significant uncertainties, such as how restoration siting and design (e.g., height and corresponding roughness) influence the overall effectiveness of such endeavors (Roelvink et al., 2021; Norris et al., 2023).
Prior to restoration implementation, modeling studies are essential to identify how potential design options for a restored reef can optimize protective benefits and reduce coastal flood risk (Viehman et al., 2023). The importance of reef geometry and topography, such as reef slope, width, and profile shape, on wave dynamics have been highlighted in previous studies, as these factors significantly influence wave energy distribution, total wave energy, and run-up in harbors (e.g., Gao et al., 2019, 2020). By incorporating these factors, numerical models serve as valuable tools to simulate and forecast coastal flooding scenarios. Regional-scale hydrodynamic models can inform potential restoration placement (e.g., Reguero et al., 2021; Storlazzi et al., 2021a), but reef-scale hydrodynamic models are also needed to more precisely relate restoration design and location to the potential reduction of hazard risks (e.g., Reguero et al., 2018). These forecasts enable mitigation planning for NbS that could reduce damages and enhance resilience in the face of coastal flood events. However, few reef-scale hydrodynamic models have yet been implemented to inform coral restoration planning and design.
In this study, we investigated the effects of potential coral reef restoration design and siting on coastal flood reduction along the coast of San Juan, Puerto Rico, U.S.A., for extreme events and projected SLR. We first provide a brief overview of the study area and field data. We next show the calibration and validation process of one-dimensional (1D) numerical models encompassing the field data and expanded into a two-dimensional (2D) model of the study area. We then present results of the 1D and 2D model simulations and discuss the effects of coral restoration on wave run-up and flood reduction along the coast. This study provides a template for planning coral reef restoration as a NbS practice in coastal areas to reduce coastal flooding caused by combination of extreme events and SLR.
2 Study area
Puerto Rico’s northern coastline facing the North Atlantic Ocean experiences strong wave action, predominantly from the northeast, with waves typically moving westward (Barreto-Orta et al., 2019). Our study area, extending from the Escambrón area (or Bahía de Condado) to the region adjacent to Luis Muñoz Marín International Airport (Figure 1a), includes nearshore littoral habitats and sandy beaches. The study area was exposed to severe wind and wave conditions during Hurricanes Irma and Maria in 2017, resulting in coastal flooding and erosion that threatened critical infrastructure, tourism facilities, and property (Cangialosi et al., 2018; Pasch et al., 2019). The coral reefs north of San Juan provide critical natural infrastructure to defend against storm waves and coastal flooding by dissipating wave energy and thus safeguarding critical coastal infrastructure. However, the primary reef building coral species in this geography have suffered significant declines caused by multiple stressors including coral bleaching, disease, hurricanes, and degraded water quality, reducing the structural complexity of the reef, and therefore, the effectiveness of the reefs for coastal protection. Consequently, large-scale coral restoration of Acropora palmata, a primary coral species for building shallow reefs that attenuate waves, is being evaluated in this coastal region by local managers (Viehman et al., 2020). Goals of a large-scale coral reef restoration would include increasing the coastal protection benefits of these reefs; therefore, the site is ideal for modeling the complex interactions between coral restoration and coastal wave-driven flood reduction.
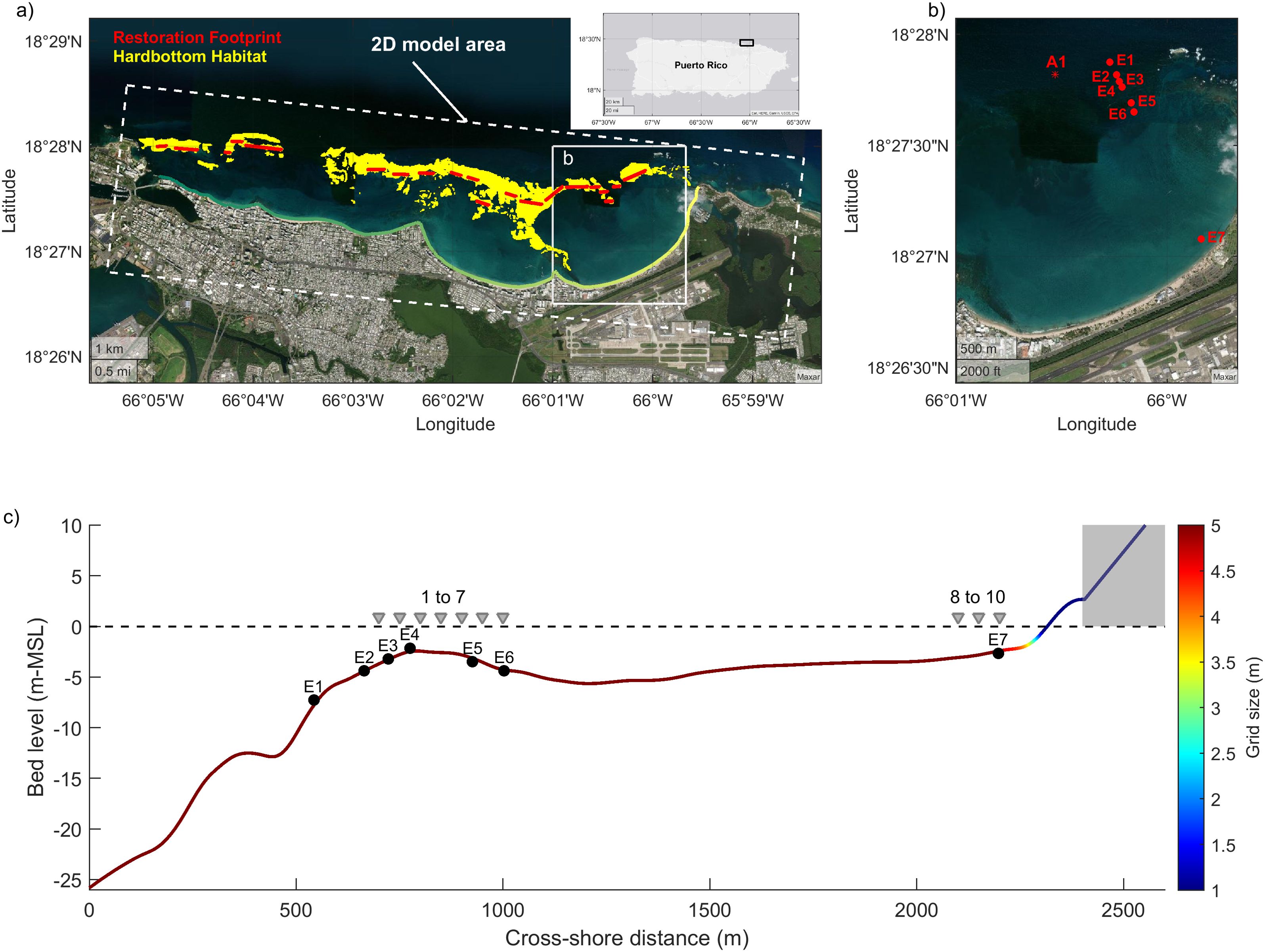
Figure 1. Map of the study area in San Juan, Puerto Rico, U.S.A., and the one-dimensional and two-dimensional XBeach hydrodynamic model domains. (a) Plan view of the study area highlighting the hard bottom habitat (yellow area) and the location of the proposed restoration marked in red within the 2D model. The extent of the coastline along the shore is represented by a gradient color line. (b) Location of the field measurements used for the 1D XBeach model calibration and validation. (c) Profile view of the cross-shore transect, indicating the locations of observation points aligned with field deployments (E1 to E7) and location of proposed reef restoration (1 to 10, see Sec. 3.5). The gray area indicates artificially raised land elevation for model purposes; the horizontal dashed line represents the mean sea level at the start of the model simulation.
3 Data and methods
3.1 Field measurements
We used field data collected from 8 instruments deployed off San Juan from November 2018 to April 2019 from Rosenberger et al. (2020), as shown in Figure 1b. Field measurements were obtained from a cross-shore transect (E1-E7, Figure 1b). Field data provided the offshore wave forcing, as well as wave and water level data across the fore reef, reef flat, shallow back-reef and lagoon. The dataset consisted of a Nortek Acoustic Wave and Current profiler that was deployed at a depth of 12.8 m (A1 in Figure 1b) on the middle fore reef to record directional incident wave conditions at 1 Hz for 34 min every hour and 7 Nortek RBR Solo-D|wave pressure sensors (E1 – E7 in Figures 1b, c) that collected measurements at 2 Hz for a burst time of 34 min every hour on the upper fore reef, reef flat, lagoon, and upper shoreface.
The RBR sensor measurements were corrected for atmospheric pressure and then converted to water levels. Wave measurements were analyzed to calculate significant wave heights () and root-mean-squared wave heights () of sea-swell (SS, frequency range of 0.20–0.04 Hz) and infragravity (IG, frequency range of 0.040–0.004 Hz) waves. Two high-energy wave events during 28-30 December 2018 and 23-26 January 2019 (Supplementary Material) were used for model calibration and validation, respectively. The E5 sensor was unable to record data throughout the entire deployment due to battery issues, resulting in a lack of data for the 2019 event. Wave height transformations over the reef toward the coast demonstrate a general trend of decreasing wave height. Substantial wave energy was dissipated between the E2 and E5 locations, which are characterized by shallow depths (~<5 m, Figure 1c), primarily due to wave breaking that resulted in an increase in the mean water level.
3.2 Numerical model
We used the physics-based, coastal hydrodynamic model XBeach to simulate scenarios of coral reef restoration location and height in conditions of extreme events. XBeach is a 2D, open-source, phase-averaged wave numerical model that solves equations for wave propagation, mean flow including long waves, sediment transport, and morphological changes (Roelvink et al., 2009). XBeach has widely been used to model 1D and 2D coastal flooding and has been validated against field measurements (e.g., Quataert et al., 2015; Elsayed and Oumeraci, 2016; Storlazzi et al., 2018). We utilized the surfbeat mode, which solves short wave fluctuations at the wave group scale (Roelvink et al., 2009). This mode employs a reduced wave-action equation to capture these variations while also resolving the associated long waves. This means that it focuses on the overall behavior of the group of waves, rather than simulating each individual wave within the group. The surfbeat mode, capable of resolving both short and long waves, offers a comprehensive depiction of coastal processes, enhancing the overall fidelity of the simulations to provide a more efficient and accurate simulation of short-wave behavior (Quataert et al., 2020).
A digital elevation model of the study area from USACE FEMA topobathy lidar (OCM Partners, 2024) was used to create bathymetric files for the modeling efforts. We developed a 1D XBeach model along the cross-shore instrument transect for model calibration and validation (Figures 1b, c). This simplified model facilitated efficient calibration efforts and enabled focused analysis of key parameters. The model utilized a non-equidistant grid, with resolution optimized based on depth, ranging from 5 m in deeper offshore regions to 1 m in the nearshore and surf zone. This approach ensured finer resolution in areas of active wave transformation while maintaining computational efficiency offshore. The grid refinement followed XBeach’s built-in grid generation settings, which adaptively adjust resolution according to hydrodynamic conditions. Additionally, we assumed no changes in the bed level within the model, indicating no morphological alterations throughout the simulation.
The field data from sites E1-E7 were used to calibrate and validate a 1D XBeach model along the instrument transect (Figure 1b). For calibration, the model ran over a two-day period to simulate the 2018 event, whereas for validation, it ran over a three-day period to simulate the 23-26 January 2019 event (see Supplementary Figure 1). These simulations were conducted with storm-induced waves represented at the offshore boundary of the model domain (x=0 in Figure 1c) by a Joint North Sea Wave Project (JONSWAP) spectrum with significant wave height and peak period obtained from field measurements (Sec. 3.1). Time series of short-wave heights and surface elevations at each instrument location were output from the 1D simulations (E1-E7 in Figure 1c) for comparison with field measurements.
The calibrated and validated 1D model was used to evaluate the effects of coral restoration (i.e., location and height) on wave run-up (i.e., the vertical distance between the still water level and the maximum height of the wave up-rush on the shoreline) reduction under extreme forcing conditions. We specified a numerical run-up gauge to track the moving waterline with a minimum depth of 0.02 m. We extended the beach, maintaining a consistent slope of 1:20 from the natural beach slope, up to +10 m above mean sea level (MSL, x> 2300 m, see also gray area in Figure 1c) to allow for the run-up to be evaluated across the entire range of hydrodynamic forcing, which are applied as offshore boundary conditions at the seaward extent of the transect (i.e., at cross-shore equal to zero) of the model. We analyzed the run-up time series, identified individual run-up peaks above the still water level, and then calculated a 98th percentile of the peaks in the vertical run-up in order to determine the , which is the value exceeded by 2% of the run-up events.
The 1D model calibration and validation results were used to develop a fully 2D numerical model (Figure 1a) to study the effects of variations in coral reef restoration structure (i.e., height) and oceanographic forcing on coastal flooding. The model was forced with extreme event scenarios (Table 1) as JONSWAP wave spectrum at the offshore boundary and absorbing-generating conditions onshore and no-flux conditions at the lateral boundaries. Large scale 2D XBeach models require extensive computational time (Rautenbach et al., 2022; Gaido-Lasserre et al., 2024) and thus we increased the computational efficiency of the simulations by implementing a parallel message passing interface version of XBeach on an 80-core Linux cluster for faster processing and execution of the simulations.
3.3 Model calibration and validation
We calibrated the model by adjusting the short-wave friction coefficient () and the current and infragravity wave friction coefficient () to minimize model-data differences in waves and water levels. We conducted a set of simulations to identify the sensitivity of the model to hydrodynamic friction parameters (i.e., and ) and to identify optimum values that can be used to reproduce key hydrodynamic processes along the profile. A total of 56 simulations were conducted with different combinations of short-wave friction coefficients and current friction coefficients. The range of varied from 0.1 to 0.8 in increments of 0.1, whereas values tested included 0.01, 0.05, 0.10, 0.15, 0.20, 0.25, and 0.30 (see Supplementary Material).
For any constant value, as increased, mean water level and infragravity wave height () had smaller root-mean-square error (RMSE), which means that the model can reproduce observed values more accurately across the transect. This improvement in model performance was gained at the expense of lower accuracy in sea-swell wave height (). The effects of on sea-swell wave height for a constant was negligible; however, the lower value of would lead to a higher mean water level RMSE. A smaller wave friction coefficient () resulted in larger mean water level RMSE, but also increased setup on the reef flat (see Supplementary Figure 2).
For all simulations, differences between modeled and observed mean water levels had RMSE under 0.1 m. The lowest summation of the RMSE values for mean water level, , and were determined. The sensitivity analysis resulted in a spatially-variable combination of friction coefficients that could most accurately reproduce variations in SS waves, IG waves, and mean water levels observed across the cross-shore transect. We then used those values to validate the model performance for the 2019 event. We determined the performance of the calibrated model by calculating RMSE and mean bias error (e.g., Roelvink et al., 2009) for the 23-26 January 2019 event.
The validation results indicate an average RMSE of 0.05 m for the mean water level. The bias varies across the transect from -0.02 m (indicating underestimation) over the fore reef to 0.03 m (indicating overestimation) over the reef flat (Figure 2). The predicted values for SS and IG waves have an average RMSE of ~0.09 and ~0.05 m, respectively. Overall, the validation results indicate that the model can reproduce the spatial variability in wave heights across the transect, as well as temporal changes in response to the varying offshore/boundary wave and water level conditions.
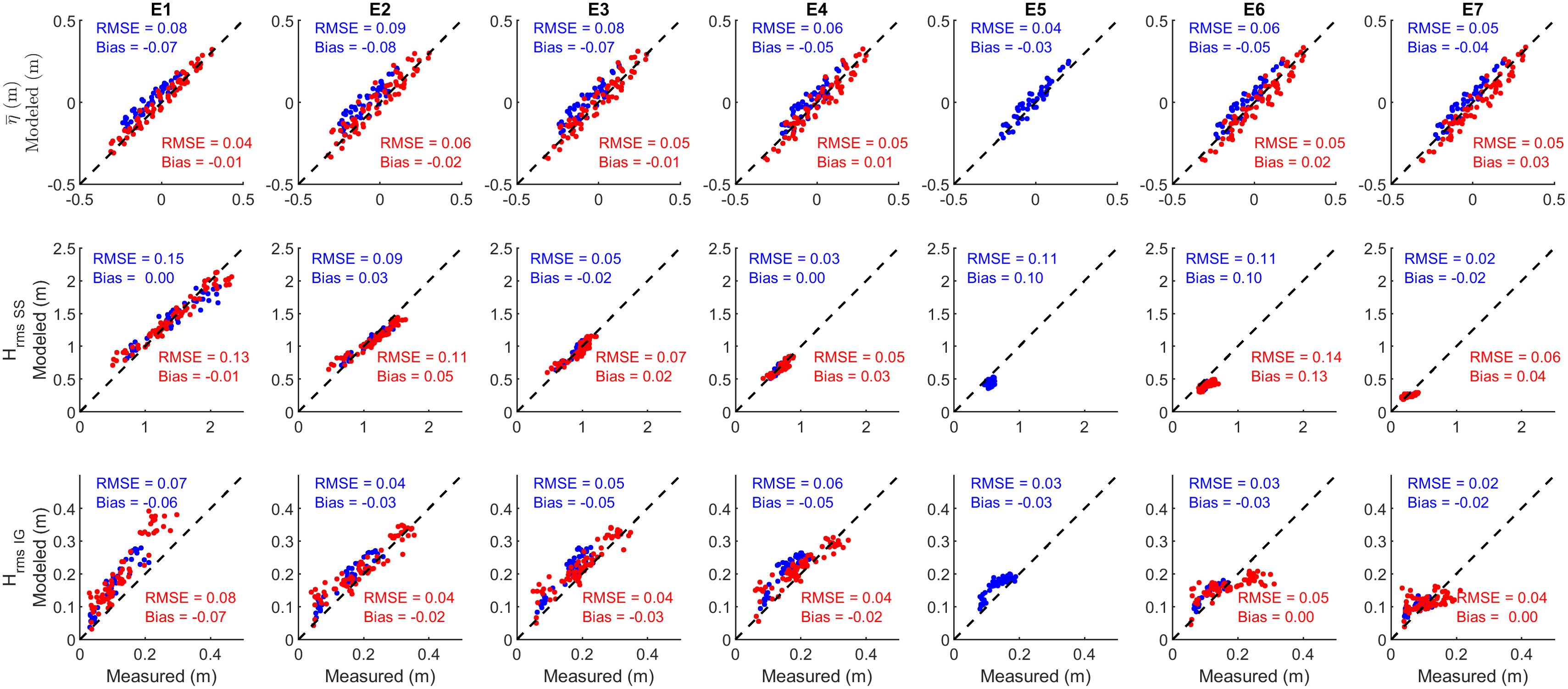
Figure 2. Comparison of model results with oceanographic field data. Measured burst-averaged mean water level (top row), sea-swell wave height (, mid row), and infragravity wave height (, bottom row) for each instrument along the transect. Model results for calibration and validation are plotted in blue and red, respectively. Positive bias indicates over-prediction and negative bias indicates under-prediction.
3.4 Extreme events scenarios
We obtained the wave heights, periods, and projected SLR associated with the different levels of extreme events (10-, 50-, and 100-yr return periods) from the Caribbean coastal ocean observing system wave model (Anselmi-Molina et al., 2012; http://caricoos.org). Significant wave heights of 4.97-7.39 m with peak periods of 14.8-17.8 s correspond to extreme events that occur at return periods between 10 years and 100 years (Table 1). These values were applied as boundary conditions (i.e., the corresponding JONSWAP spectra) with friction values determined from the calibration process (Sec. 3.3) to both the 1D and 2D XBeach models to investigate the effects of restored reef on coastal flooding and evaluate its effects on the coastal system.
3.5 Restoration scenarios
The size, depth, and location of restored reefs, along with the density of outplanted corals added to the seabed in restoration (which translates into hydrodynamic roughness) were varied in the 1D model. Depths of locations for potential restorations were restricted to a range of 2.5-4.0 m to provide an ideal real-world scenario for restoration of A. palmata coral. A. palmata is particularly suited for restoration for coastal resilience since it has naturally dominated shallow fore-reef, crest, and shallow back-reef habitats; while capable of growing to a height of 50 cm or more within 5 years (Acropora Biological Review Team, 2005).
Forty potential restoration scenarios were tested in the 1D model. Ten locations were included across the transect (Figure 1c), with four restoration heights of 25, 50, 100, and 150 cm. Although it is unlikely for the reef to reach 150 cm height, this choice would provide a more comprehensive analysis. The reef restoration starting point was set at 700, 750, 800, 850, 900, 950, 1000, 2100, 2150 and 2200 m in the cross-shore along the transect (i.e., 1 to 10 in Figure 1c) and extend 10 m onshore.
For the 2D models, we utilized friction coefficients derived from the 1D model calibration and mapped these values onto the habitat maps to determine the friction characteristics for each habitat type. Applying the 1D-derived friction values in the 2D model allowed us to capture habitat-specific variations in wave dissipation, reflecting the diverse frictional properties of coastal habitats (e.g., coral reefs, sandy areas). By integrating the NOAA 2017 hard bottom habitat map (Viehman et al., 2020) with the NOAA benthic habitat map (Kendall et al., 2001), we created a spatially varying friction file that assigns specific friction values to different habitats. Additionally, we used the hard bottom habitat map to guide the placement of proposed restorations (Figure 1a).
4 Results
4.1 Wave run-up
Using the validated and calibrated 1D model, we investigated how coral reef restoration could impact wave run-up under extreme wave and water level conditions. The percent change in , which is defined as the percent difference between the current unrestored and simulated restored reef, for the different model simulations, is presented in Figure 3. Dissipation of waves across a restored reef is the main factor influencing wave transformation compared to an unrestored reef. Restored coral reefs can result in a decrease in at the shoreline because of reductions in both the wave heights and water levels, resulting in less inland penetration of wave-induced flooding.
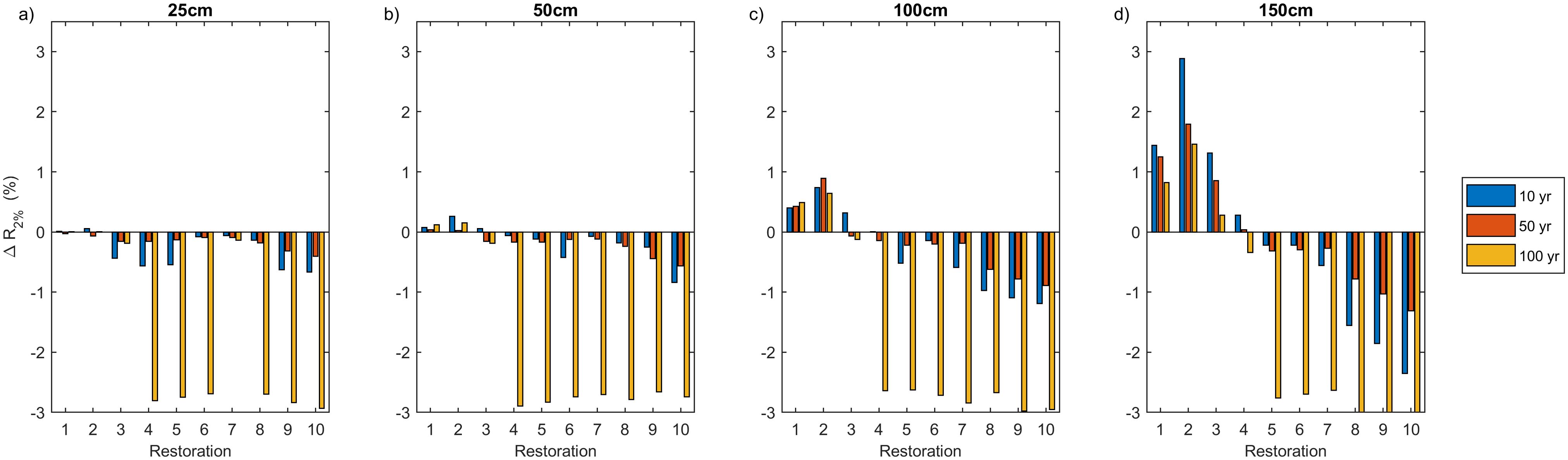
Figure 3. The percent change in run-up, , at 10 restoration locations (Figure 1c) with (a) 25, (b) 50, (c) 100, and (d) 150 cm restoration height for three storm scenarios (Table 1) compared to unrestored conditions. Positive values indicate an increase in wave run-up, negative values indicate a decrease. Larger restoration heights closer to the shore lead to a greater reduction in wave run-up for all storm scenarios.
The model results indicate that reduction increases as coral reef restoration height and offshore wave height increases (Figure 3), due to increased wave energy dissipation. However, the reduction in is also dependent on the restoration location. Restoration locations 1 and 2 (Figure 1) at 50 cm restoration height, and restoration locations 1 to 3 with 100 and 150 cm restoration height, lead to increases in by initiating the wave-breaking process farther offshore. This process also increases wave setup inshore of the breakpoint over a larger portion of the reef. Consequently, this amplified setup contributes to higher levels along the coastline. In contrast, restorations closer to the shore (i.e., locations 8, 9, and 10 in Figure 2) more effectively reduce wave run-up. These locations, positioned after initial wave breaking, enhance wave dissipation and mitigate the impact of waves more efficiently, leading to a notable reduction in levels. This finding supports previous studies (Reguero et al., 2018; Roelvink et al., 2021), which indicate that restorations near the shore are more effective at reducing wave run-up due to their role in dissipating wave energy after wave breaking.
4.2 2D wave-driven flooding
Based on our 1D calibrated and validated model results, we developed a 2D model to study effects of restoration scenarios on coastal flooding. The top 2% of the maximum wave-driven water levels () along the coastline are presented in Figure 4. Nearly the entire coastline within the study area is vulnerable to coastal hazards from flooding (Figure 4), emphasizing the need for effective coastal management strategies that can mitigate these risks.
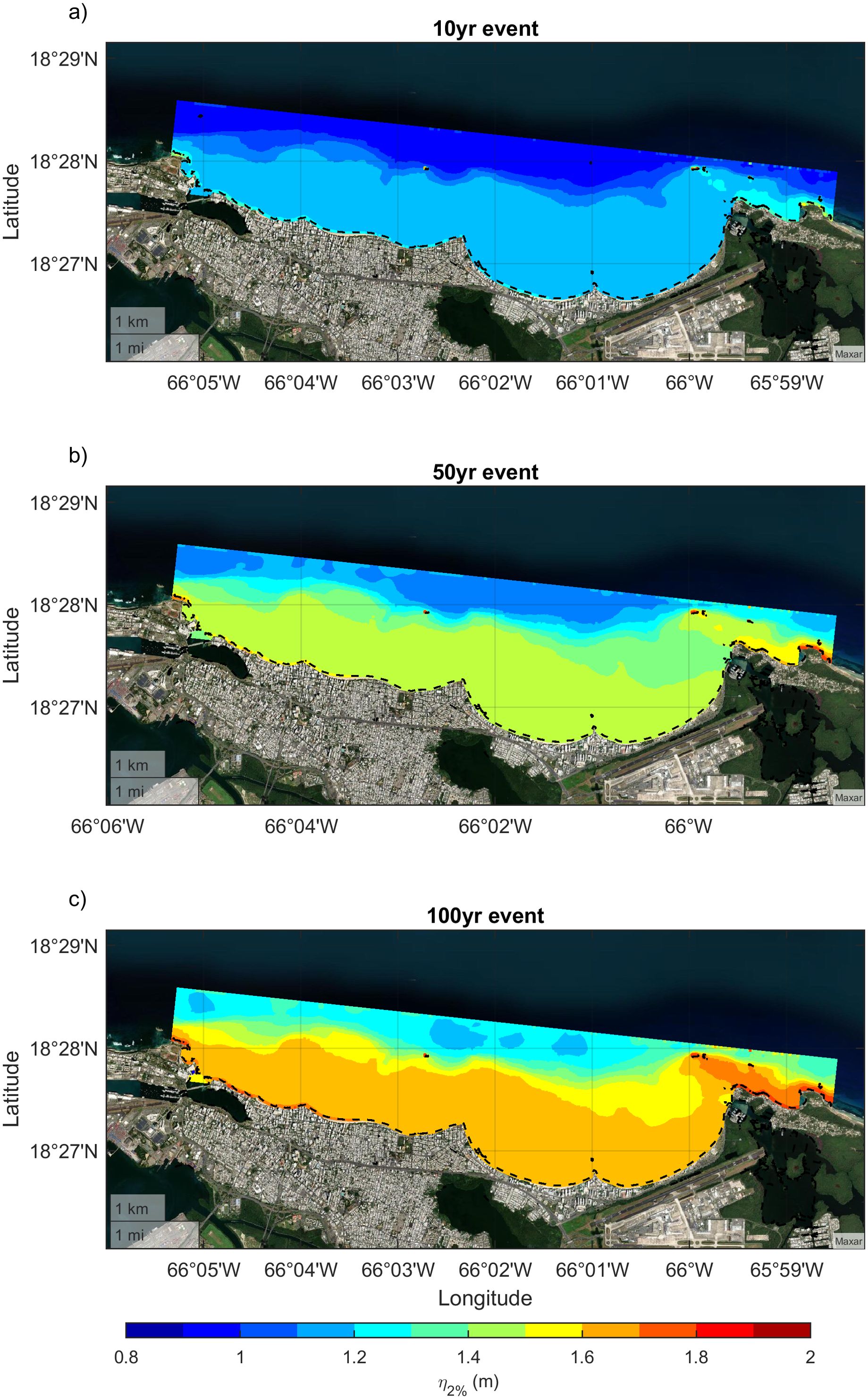
Figure 4. Maps displaying the top 2% of the maximum total wave-driven water levels for a (a) 10-, (b) 50-, and (c) 100-yr return period storm scenarios with no coral reef restoration (i.e., present day bathymetry). The flood extent is compared to the coastline (black dashed line). The entire coastline is vulnerable to flooding and for greater return-period storms, the amplitude of total water levels and inland extent of flooding increase.
The primary factors contributing to the variability in the difference of maximum water levels between the current unrestored and simulated restored reefs () are the changes in bathymetry and the enhanced hydrodynamic roughness resulting from coral restoration, which significantly influence waves and wave-driven water levels (Figure 5).
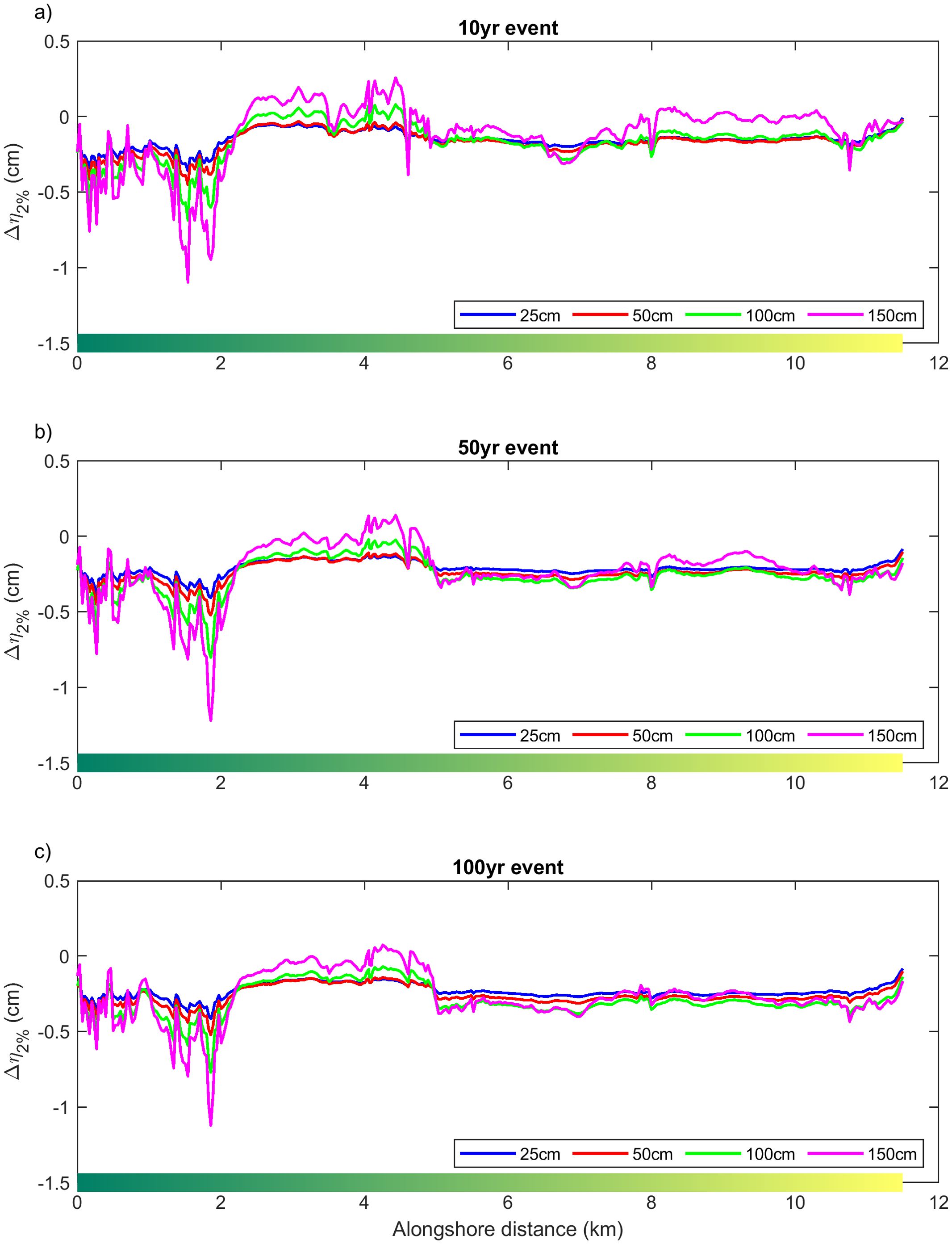
Figure 5. Difference between the planned coral reef restoration (Figure 1a) and no-restoration maximum water levels for the (a) 10-, (b) 50-, and (c) 100-yr return-period storms and four restoration height (25, 50, 100, 150 cm) scenarios along the coastline represented by a gradient color line (see Figure 1a). Restoration efforts have a greater impact on smaller storms with shorter return periods. Restorations closer to the coast (~300-700 m offshore) over 0-2 km alongshore distance result in greater reductions in wave-driven coastal flooding.
Restoration sites located closer to the shore, particularly on the west side of the study area, spanning the western-most 2 km of the coastline (0-2 km alongshore in Figure 5), result in greater reductions in maximum water levels () due to both the proximity to shore and the height of the restoration. This pattern is consistent with the results from the 1D model simulations (see Section 4.1), which indicate that restorations near shore are more effective at reducing .
However, an increase in maximum water levels () is observed in areas without restoration efforts. Specifically, over the alongshore distance of ~2-4 km in the study area (Figure 5), where the absence of restoration results in a lack of wave attenuation, leading to an increase in flooding. This region represents a sandy area between the western and eastern proposed hardbottom restoration sites, and thus no restoration is proposed for this section. Additionally, along the eastern portion of the study area (approximately 8-10 km alongshore), the restoration efforts lead to an increase in water levels. This could result in an elevated and further flooding, as shown in Figure 5, which may be exacerbated by increased offshore restoration. The amplification of water levels in these areas supports the findings of the 1D models, which suggest that offshore restorations can contribute to increased wave energy and flooding in these regions.
5 Discussion and conclusion
Enhancing coastal resilience is essential for mitigating the impacts of increased storm frequency and intensity, as well as SLR, in the context of a changing climate. Although natural coral reefs can provide coastal protection, reefs with reduced structural complexity - from death of reef-building coral species and the erosion of the underlying reef structure - may not provide the coastal protection benefit possible in each situation. Ecological restoration as part of NbS may be able to improve the coastal protection functions, particularly on degraded reefs resulting from climate change (Sheppard et al., 2005) or other stressors, such as land-based pollution (Carlson et al., 2019). In this study, we utilized a combination of field observations and numerical coastal engineering modeling to assess the effectiveness of simulated reef restoration efforts (i.e., location and characteristics of the restoration features) to serve as NbS by improving the resilience of the San Juan coastline to the impacts of storms and wave-driven flooding. By incorporating future projections of SLR, this research addresses critical gaps in understanding how restoration design parameters—such as reef depth, location, and height—affect flood risk reduction. Additionally, this study moves beyond theoretical approaches by employing practical, case-specific modeling within a real-world context.
The sensitivity analysis conducted on a 1D model reveals that the dimensions and location of coral reef restoration efforts, as well as hydrodynamic forcing conditions, can significantly impact coastal flooding. Restoration scenarios extending onto the reef flat (locations 4-7) decrease wave run-up, whereas restoration implemented offshore of the reef flat (locations 1-3) is likely to lead to increased wave-driven flooding. This increase in flooding occurs due to the creation of elevated water levels (i.e., setup) onshore of the reef flat. This effect promotes higher wave run-up, though it should be noted that the model oversimplifies this process by representing the restoration as an impermeable, elevated bed. Furthermore, the 1D model does not include the 2D effects of flow and longshore currents, which have the potential to modify wave-induced setup. When considering the relative comparison of flood levels rather than an absolute representation of flood risk, it becomes apparent that restorations conducted on the flat reef and farther inshore in closer proximity to the beach shoreline exhibit a relatively larger influence in reducing wave run-up than farther offshore, as was proposed by Roelvink et al. (2021). However, the scope of restoration locations in this exercise were limited to areas with hard bottom habitat in depths optimized for A. palmata restoration, which restricted the spatial areas for restoration.
To address the 1D approach limitation and capture the complete dynamics of wave-induced changes in the context of restoration, we conducted a 2D modeling approach. This enhanced methodology made it possible to provide a more comprehensive representation of the complex interactions between waves, wave-driven water levels, coral reef restoration efforts, and their impact on coastal flooding. Our results indicate that increased hydrodynamic roughness from reef restoration can reduce wave heights, wave run-up, and wave-driven flooding. However, the specific location of restoration efforts can influence these effects and can also potentially create conditions for amplification of waves, wave-driven water levels, and resulting coastal flooding. In addition, effects of restoration on run-up and flooding decrease for higher energy, but less, frequent extreme events. In these scenarios, the variability in the underwater topography (bathymetric variability) becomes relatively smaller with increased water depth due to SLR, resulting in reduced frictional wave dissipation and thus lower variation in maximum wave-driven water levels.
The model results indicate that the preliminary design scenarios for the potential coral reef restoration may not lead to a substantial reduction in the maximum water levels, implying a limited contribution to coastal protection. This result emphasizes the need to include scenario modeling when designing restoration for coastal protection: not all potential restoration locations or designs may reduce coastal hazards. However, it is important to recognize that the restoration of degraded coral reefs not only enhances their coastal protection function but also helps prevent further loss of coastal hazard risk reduction due to anthropogenic activities and promotes ecological functions. Ecologically-oriented restoration efforts that enhance reef habitats and restore threatened coral populations can also contribute to maintaining or restoring the geologic reef structure through accretion from deposition of coral skeletal material. The continuing degradation of coral reef structure poses a significant risk to coastal areas, as highlighted by Storlazzi et al. (2021b). Successful restoration efforts are crucial as they can prevent or slow down further degradation, thereby reducing the risk to coastal communities.
This study’s approach and findings have practical implications for coastal planning and management and provide decision-makers with key insights into identifying the most appropriate locations and designs for restoration projects in terms of accounting for their potential to mitigate flood risks. By quantitatively comparing multiple restoration locations and elevations, this study helps identify which designs offer the greatest flood reduction benefits and contribute to enhancing coastal resilience, property protection, and minimizing loss of life. Further studies are needed to fully understand the realized functional benefits and cost-effectiveness of coral restoration for flood reduction, as well as to develop successful strategies for scaling up coral restoration efforts in a sustainable manner. Recent studies provide valuable insights into the cost-effectiveness of coral restoration for flood risk reduction on a regional scale (e.g., Storlazzi et al., 2025). By incorporating these findings into local-scale models, policymakers and stakeholders can be better equipped to make informed decisions regarding coral restoration and its broader economic and social impacts.
Data availability statement
The datasets presented in this study can be found in online repositories. The names of the repository/repositories and accession number(s) can be found below: https://www.sciencebase.gov/catalog/item/5d7ab2fee4b0c4f70d02a493.
Author contributions
RF: Formal analysis, Methodology, Visualization, Writing – original draft, Writing – review & editing. CS: Conceptualization, Writing – review & editing. MN: Writing – review & editing. SV: Writing – review & editing, Conceptualization.
Funding
The author(s) declare that financial support was received for the research and/or publication of this article. This work was supported by NOAA’s Coral Reef Conservation Program (project 31231), U.S. Geological Survey’s Coastal Marine Hazards and Resources Program, and NOAA’s National Centers for Coastal Ocean Science.
Acknowledgments
The views and conclusions contained in this document are those of the authors and should not be interpreted as representing the opinions or policies of NOAA. This document has been peer reviewed and approved for publication consistent with USGS Fundamental Science Practices (https://pubs.usgs.gov/circ/1367). Any use of trade, firm, or product names is for descriptive purposes only and does not imply endorsement by the U.S. Government.
Conflict of interest
Author RF was employed by Consolidated Safety Services, Inc., and MN was employed by Earth Resources Technology Inc.
The remaining authors declare that the research was conducted in the absence of any commercial or financial relationships that could be construed as a potential conflict of interest.
Generative AI statement
The author(s) declare that no Generative AI was used in the creation of this manuscript.
Publisher’s note
All claims expressed in this article are solely those of the authors and do not necessarily represent those of their affiliated organizations, or those of the publisher, the editors and the reviewers. Any product that may be evaluated in this article, or claim that may be made by its manufacturer, is not guaranteed or endorsed by the publisher.
Supplementary material
The Supplementary Material for this article can be found online at: https://www.frontiersin.org/articles/10.3389/fmars.2025.1528460/full#supplementary-material
References
Acropora Biological Review Team (2005). Atlantic Acropora Status Review Document. (Report to National Marine Fisheries Service, Southeast Regional Office), 152.
Anselmi-Molina C. M., Canals M., Morell J., Gonzalez J., Capella J., Mercado A. (2012). Development of an operational nearshore wave forecast system for Puerto Rico and the U.S. Virgin Islands J. Coast. Res. 1049-1056, 1048. doi: 10.2112/JCOASTRES-D-11-00132.1'
Barreto-Orta M., Méndez-Tejeda R., Rodríguez E., Cabrera N., Díaz E., Pérez K. (2019). State of the beaches in puerto rico after Hurricane Maria (2017). Shore Beach 87 (1), 16.
Bayraktarov E., Stewart-Sinclair P. J., Brisbane S., Boström-Einarsson L., Saunders M. I., Lovelock C. E., et al. (2019). Motivations, success, and cost of coral reef restoration. Restor. Ecol. 27, 981–991. doi: 10.1111/rec.12977
Burke L., Reytar K., Spalding M., Perry A. (2011). Reefs at risk revisited (Washington, DC: Rep., World Resour. Inst.).
Cangialosi J. P., Latto A. S., Berg R. (2018). National Hurricane Center Tropical Cyclone Report: Hurricane Irma (AL112017). Available online at: http://www.nhc.noaa.gov/data/tcr/AL112017_Irma.pdf.
Carlson R. R., Foo S. A., Asner G. P. (2019). Land use impacts on coral reef health: A ridge-to-reef perspective. Front. Mar. Sci. 6. doi: 10.3389/fmars.2019.00562
Eakin C. M., Liu G., Gomez A. M., de la Couri J. L., Heron S. F., Skirving W. J., et al. (2018). Unprecedented three years of global coral bleaching 2014–17. Sidebar 3.1. [in State of the Climate in 2017. Bull. Am. Meteorol. Soc. 99, S74–S75.
Elko N., Briggs T. R., Benedet L., Robertson Q., Thomson G., Webb B. M., et al. (2021). A century of U.S. beach nourishment. Ocean Coast. Manage. 199, 105406. doi: 10.1016/j.ocecoaman.2020.105406
Elliff C. I., Silva I. R. (2017). Coral reefs as the first line of defense: shoreline protection in face of climate change. Mar. Env. Res. 127, 148–154. doi: 10.1016/j.marenvres.2017.03.007
Elsayed S. M., Oumeraci H. (2016). Combined modelling of coastal barrier breaching and induced flood propagation using XBeach. Hydrology 3 (4), 32. doi: 10.3390/hydrology3040032
Ferrario F., Beck M. W., Storlazzi C. D., Micheli F., Shepard C. C., Airoldi L. (2014). The effectiveness of coral reefs for coastal hazard risk reduction and adaptation. Nat. Commun. 5, 3794. doi: 10.1038/ncomms4794
Gaido-Lasserre C., Nederhoff K., Storlazzi C. D., Reguero B. G., Beck M. W. (2024). Improved efficient physics-based computational modeling of regional wave-driven coastal flooding for reef-lined coastlines. Ocean Model. 189, 102358. doi: 10.1016/j.ocemod.2024.102358
Gao J., Ma X., Dong G., Zang Y., Ma Y., Zhou L. (2019). Effects of offshore fringing reefs on the transient harbor resonance excited by solitary waves. Ocean Eng. 190, 106422. doi: 10.1016/j.oceaneng.2019.106422
Gao J., Ma X., Zang J., Dong G., Ma X., Zhu Y., et al. (2020). Numerical investigation of harbor oscillations induced by focused transient wave groups. Coast. Eng. 158, 103670. doi: 10.1016/j.coastaleng.2020.103670
Hallegatte S., Green C., Nicholls R. J., Corfee-Morlot J. (2013). Future flood losses in major coastal cities. Nat. Climate Change 3, 802–806. doi: 10.1038/nclimate1979
Hoegh-Guldberg O., Mumby P. J., Hooten A. J., Steneck R. S., Greenfield P., Gomez E., et al. (2007). Coral reefs under rapid climate change and ocean acidification. Science 318, 1737–1742. doi: 10.1126/science.1152509
Hughes T. P., Barnes M. L., Bellwood D. R., Cinner J. E., Cumming G. S., Jackson J. B. C., et al. (2017). Coral reefs in the Anthropocene. Nature 546, 82–90. doi: 10.1038/nature22901
IPCC (2012). Managing the Risks of Extreme Events and Disasters to Advance Climate Change Adaptation. A Special Report of Working Groups I and II of the Intergovernmental Panel on Climate Change. Eds. Field C. B., Barros V., Stocker T. F., Qin D., Dokken D. J., Ebi K. L., Mastrandrea M. D., Mach K. J., Plattner G.-K., Allen S. K., Tignor M., Midgley P. M. (Cambridge, UK, and New York, NY, USA: Cambridge University Press), 582.
IPCC (2014). Climate Change 2014: Synthesis Report. Contribution of Working Groups I, II and III to the Fifth Assessment Report of the Intergovernmental Panel on Climate Change Vol. 151 (Geneva: IPCC).
Kendall M. S., Monaco M. E., Buja K. R., Christensen J. D., Kruer C. R., Finkbeiner M., et al. (2001). Methods used to map the benthic habitats of Puerto Rico and the U.S. Virgin Islands: National Oceanic and Atmospheric Administration. 45. Available online at: http://www2.coastalscience.noaa.gov/publications/.
Kirezci E., Young I. R., Ranasinghe R., Muis S., Nicholls R. J., Lincke D., et al. (2020). Projections of global-scale extreme sea levels and resulting episodic coastal flooding over the 21st Century. Sci Rep. 10 (1), 11629. doi: 10.1038/s41598-020-67736-6
Neumann B., Vafeidis A. T., Zimmermann J., Nicholls R. J. (2015). Future coastal population growth and exposure to sea-level rise and coastal flooding - A global assessment. PloS One 10, e0118571. doi: 10.1371/journal.pone.0118571
Norris B. K., Storlazzi C. D., Pomeroy A. W. M., Rosenberger K. J., Logan J. B., Cheriton O. M. (2023). Combining field observations and high-resolution numerical modeling to demonstrate the effect of coral reef roughness on turbulence and its implications for reef restoration design. Coast. Eng. 184, 104331. doi: 10.1016/j.coastaleng.2023.104331
OCM Partners (2024). 2018 USACE FEMA Topobathy Lidar DEM: Main Island, Culebra, and Vieques, Puerto Rico from 2010-06-15 to 2010-08-15 (NOAA National Centers for Environmental Information). Available at: https://www.fisheries.noaa.gov/inport/item/53098 (Accessed February 1, 2024).
Pasch R. J., Penny A. B., Berg R. (2019). National Hurricane Center Tropical Cyclone Report: Hurricane Maria (AL152017). Available online at: https://www.nhc.noaa.gov/data/tcr/AL152017_Maria.pdf.
Perricone V., Mutalipassi M., Mele A., Buono M., Vicinanza D., Contestabile P. (2023). Nature-based and bioinspired solutions for coastal protection: an overview among key ecosystems and a promising pathway for new functional and sustainable designs. ICES J. Mar. Sci. 80, 1218–1239. doi: 10.1093/icesjms/fsad080
Quataert E., Storlazzi C., van Dongeren A., McCall R. (2020). The importance of explicitly modeling sea-swell waves for runup on reef-lined coasts. Coast. Eng. 160, 103704. doi: 10.1016/j.coastaleng.2020.103704
Quataert E., Storlazzi C., van Rooijen A., Cheriton O., van Dongeren A. (2015). The influence of coral reefs and climate change on wave-driven flooding of tropical coastlines. Geophys. Res. Lett. 42, 6407–6415. doi: 10.1002/2015GL064861
Rautenbach C., Trenham C., Benn D., Hoeke R., Bosserelle C. (2022). Computing efficiency of XBeach hydro- and wave dynamics on Graphics Processing Units (GPUs). Environ. Model. Softw. 157, 105532. doi: 10.1016/j.envsoft.2022.105532
Reguero B. G., Beck M. W., Agostini V. N., Kramer P., Hancock B. (2018). Coral reefs for coastal protection: A new methodological approach and engineering case study in Grenada. J. Env. Manage. 210, 146–161. doi: 10.1016/j.Jenvman.2018.01.024
Reguero B. G., Storlazzi C. D., Gibbs A. E., Shope J. B., Cole A. D., Cumming K. A., et al. (2021). The value of US coral reefs for flood risk reduction. Nat. Sustainabil. 4, 688–698. doi: 10.1038/s41893-021-00706-6
Roelvink D., Reniers A., van Dongeren A., van Thiel de Vries J., McCall R., Lescinski J. (2009). Modelling storm impacts on beaches, dunes and barrier islands. Coast. Eng. 56, 1133–1152. doi: 10.1016/j.coastaleng.2009.08.006
Roelvink F. E., Storlazzi C. D., van Dongeren A. R., Pearson S. G. (2021). Coral reef restorations can be optimized to reduce coastal flooding hazards. Front. Mar. Sci. 8. doi: 10.3389/fmars.2021.653945
Rosenberger K. J., Cheriton O. M., Storlazzi C. D. (2020). Cross-reef wave and water level data from coral reef environments (ver. 3.0, January 2024): U.S. Geological Survey data release. doi: 10.5066/P9RYN5NH
Sheppard C., Dixon D. J., Gourlay M., Sheppard A., Payet R. (2005). Coral mortality increases wave energy reaching shores protected by reef flats: examples from the Seychelles. Estuar. Coast. Shelf. Sci. 64, 223–234. doi: 10.1016/j.ecss.2005.02.016
Spalding M., Burke L., Wood S. A., Ashpole J., Hutchison J., zu Ermgassen P. (2017). Mapping the global value and distribution of coral reef tourism. Mar. Policy 82, 104–113. doi: 10.1016/j.marpol.2017.05.014
Storlazzi C. D., Gingerich S. B., van Dongeren A., Cheriton O. M., Swarzenski P. W., Quataert E., et al. (2018). Most atolls will be uninhabitable by the mid-21st century because of sea-level rise exacerbating wave-driven flooding. Sci. Adv. 4, eaap9741. doi: 10.1126/sciadv.aap9741
Storlazzi C. D., Reguero B. G., Alkins K. C., Shope J. B., Gaido-Lassarre C., Viehman T. S., et al. (2025). Hybrid coral reef restoration can be a cost-effective nature-based solution to provide protection to vulnerable coastal populations. Sci. Adv. 11, eadn4004. doi: 10.1126/sciadv.adn4004
Storlazzi C. D., Reguero B. G., Cole A. D., Lowe E., Shope J. B., Gibbs A. E., et al. (2019). Rigorously valuing the role of U.S. coral reefs in coastal hazard risk reduction: U.S. Geological Survey Open-File Report 2019–1027. 42. doi: 10.3133/ofr20191027
Storlazzi C. D., Reguero B. G., Cumming K. A., Cole A. D., Shope J. B., Gaido L. C., et al. (2021a). Rigorously valuing the coastal hazard risks reduction provided by potential coral reef restoration in Florida and Puerto Rico: U.S. Geological Survey Open-File Report 2021–1054. 35. doi: 10.3133/ofr20211054
Storlazzi C. D., Reguero B. G., Yates K. K., Cumming K. A., Cole A. D., Shope J. B., et al. (2021b). Rigorously valuing the impact of projected coral reef degradation on coastal hazard risk in Florida: U.S. Geological Survey Open-File Report 2021–1055. 27. doi: 10.3133/ofr20211055
Sweet W. V., Hamlington B. D., Kopp R. E., Weaver C. P., Barnard P. L., Bekaert D., et al. (2022). Global and Regional Sea Level Rise Scenarios for the United States: Updated Mean Projections and Extreme Water Level Probabilities Along U.S. Coastlines. NOAA Technical Report NOS 01 (Silver Spring, MD: National Oceanic and Atmospheric Administration, National Ocean Service), 111.
Temmerman S., Meire P., Bouma T. J., Herman P. M. J., Ysebaert T., De Vriend H. J. (2013). Ecosystem-based coastal defence in the face of global change. Nature 504, 79–83. doi: 10.1038/nature12859
Viehman T. S., Nemeth M., Groves S. H., Buckel C. A., Griffin S., Field D., et al. (2020). Coral assessment and restoration in the U.S. Caribbean after 2017 hurricanes (Silver Spring, MD: NOAA National Ocean Service, National Centers for Coastal Ocean Science), 64. NOAA Technical Memorandum 278. doi: 10.25923/7r0b-wc52
Viehman T. S., Reguero B. G., Lenihan H. S., Rosman J. H., Storlazzi C. D., Goergen E. A., et al. (2023). Coral restoration for coastal resilience: integrating ecology, hydrodynamics, and engineering at multiple scales. Ecosphere 14, e4517. doi: 10.1002/ecs2.4517
Keywords: coastal resilience, climate change, restoration, numerical models, coral reef, flood hazard
Citation: Familkhalili R, Storlazzi CD, Nemeth M and Viehman S (2025) Assessing the effect of coral reef restoration location on coastal flood hazard along the San Juan Coastline, Puerto Rico. Front. Mar. Sci. 12:1528460. doi: 10.3389/fmars.2025.1528460
Received: 14 November 2024; Accepted: 27 February 2025;
Published: 04 April 2025.
Edited by:
Xiaoxia Sun, Chinese Academy of Sciences (CAS), ChinaReviewed by:
Junliang Gao, Jiangsu University of Science and Technology, ChinaDilip Kumar Jha, National Institute of Ocean Technology, India
Copyright © 2025 Familkhalili, Storlazzi, Nemeth and Viehman. This is an open-access article distributed under the terms of the Creative Commons Attribution License (CC BY). The use, distribution or reproduction in other forums is permitted, provided the original author(s) and the copyright owner(s) are credited and that the original publication in this journal is cited, in accordance with accepted academic practice. No use, distribution or reproduction is permitted which does not comply with these terms.
*Correspondence: Ramin Familkhalili, cmFtaW4uZmFtaWxraGFsaWxpQG5vYWEuZ292