- 1Northeastern Regional Association of Coastal Ocean Observing Systems (NERACOOS), Portsmouth, NH, United States
- 2Biology Department, Woods Hole Oceanographic Institution, Woods Hole, MA, United States
- 3Department of Civil and Environmental Engineering, Massachusetts Institution of Technology, Cambridge, MA, United States
- 4University of Maine, Darling Marine Center, Walpole, ME, United States
The northern sand lance (Ammodytes dubius), a key species in the food web supporting the Stellwagen Bank National Marine Sanctuary (SBNMS), feeds primarily on the lipid-rich copepod Calanus finmarchicus. Climate change poses a significant threat to this dynamic, as C. finmarchicus populations are at the southern edge of their subarctic distribution and are vulnerable to warming waters and changing oceanographic conditions. Declines in the advective supply of C. finmarchicus to Stellwagen Bank could adversely affect sand lance populations and, consequently, the ecological and economic resources that depend on them. To quantify the connectivity between SBNMS and potential sources of C. finmarchicus, we used the Finite-Volume Community Ocean Model (FVCOM) coupled with Lagrangian particle tracking over the years 1978 to 2016. Numerical experiments revealed that Stellwagen Bank is highly connected to upstream areas in the Maine Coastal Current (MCC), where existing time series monitoring stations observe C. finmarchicus populations. The connectivity exhibited strong seasonal patterns, with peak connectivity occurring during spring and early summer, aligning with the sand lance feeding period on C. finmarchicus. We found significant interannual variability, influenced by changes in the strength of the MCC and circulation patterns in the western Gulf of Maine. Years with stronger MCC flow showed higher connectivity and a greater potential supply of C. finmarchicus particles to Stellwagen Bank. Conversely, periods of reduced flow corresponded with decreased connectivity, potentially limiting the availability of C. finmarchicus to sand lance populations. Meanwhile, observations from drifters and buoys since 2001 have documented decreases in MCC current speed which has been linked to a climate driven strengthening of southwesterly winds. These findings underscore the importance of pelagic habitat connectivity in assessing the climate vulnerability of marine protected areas (MPAs) like SBNMS. Furthermore, monitoring C. finmarchicus populations at upstream time series stations can provide information on downstream foraging habitat in MPAs, and potentially in other vulnerable areas of ecological and socioeconomic interest. By incorporating these indicators of connectivity and upstream C. finmarchicus population abundance into decision support tools, Sanctuary managers and stakeholders can make informed decisions to mitigate potential climate impacts.
Introduction
The Stellwagen Bank National Marine Sanctuary (SBNMS) is one of the most biologically diverse and productive areas in the Gulf of Maine (GoM) region, supporting numerous recreational and commercial activities including whale watching and fishing (Battista et al., 2006; ONMS, 2020). The emblematic feature of the Sanctuary is a shallow sandy bank that provides essential habitat for northern sand lance (Ammodytes dubius), a foundational species of the local food web (Office of National Marine Sanctuaries (ONMS), 2020; Suca et al., 2021). Sand lance are planktivorous forage fish that feed on the lipid rich copepod Calanus finmarchicus (Staudinger et al., 2020; Suca et al., 2021). They exhibit both a diel and seasonal cycle, emerging from the shelter of their coarse-sand habitat to feed during the late winter through early summer (Staudinger et al., 2020; Suca et al., 2021). After sand lance cease feeding, they utilize their lipid reserves for gonad development and spawn as temperatures decline in the late fall through early winter (Murray et al., 2019). In the broader Northwest Atlantic ecosystem sand lance are a common prey item for higher trophic level species, including herring and mackerel (Suca et al., 2018; Staudinger et al., 2020). They are also preferentially targeted by migratory species and due to their non-migratory nature have been shown to attract Atlantic cod (Richardson et al., 2014), great shearwaters (Powers et al., 2020), and humpback whales (Suca et al., 2021) to SBNMS. Thus, the Sanctuary along with its ecological and economic resources are highly dependent on sand lance which in turn is vulnerable to climate change directly and indirectly through its reliance on C. finmarchicus (Office of National Marine Sanctuaries (ONMS), 2020; Pershing et al., 2021; Suca et al., 2021).
The population of C. finmarchicus in the western GoM is among the most abundant across its biogeographic range despite its residence at the southern edge of its subarctic distribution (Melle et al., 2014). Throughout the GoM, C. finmarchicus serves as a critical link in the food web transferring lipid energy up to higher trophic levels, with most characteristic North Atlantic species feeding on them directly or indirectly (Pershing and Stamieszkin, 2020). While other copepod species—such as Centropages and Pseudocalanus spp.—along with krill and amphipods are commonly consumed by small pelagic fishes and other marine predators (Bowman, 2000; Suca et al., 2018), C. finmarchicus is the preferred prey for many, including sand lance (Suca et al., 2021) and North Atlantic right whales (Hudak et al., 2023). C. finmarchicus are targeted for their relatively large size and high nutritional value, derived from the lipids they accumulate as part of their life history strategy (DeLorenzo Costa et al., 2006; Record et al., 2018). They take advantage of the highly productive spring bloom, during which their populations grow significantly. The relatively large late-stage individuals then accumulate lipid reserves for sustenance through their dormancy period (Johnson et al., 2008). The overwintering, diapausing stages of this copepod are particularly abundant in the deep waters of Wilkinson Basin, where they dominate the mesozooplankton, comprising approximately 60-80% of the total biomass (Runge et al., in review; Runge et al., 2015). Because they accumulate and transfer large amounts of lipid energy in time and space, Calanus spp. play a vital role in structuring arctic and subarctic ecosystems (Record et al., 2018) to the extent that their distribution is a defining characteristic of the North Atlantic biome, including the GoM and SBNMS (Pershing and Stamieszkin, 2020). Warming of the GoM, at a rate nearly four times faster than the global average threatens the viability of C. finmarchicus populations in the region and all those species that depend on them, including sand lance (Grieve et al., 2017; Office of National Marine Sanctuaries (ONMS), 2020; Pershing and Stamieszkin, 2020; Pershing et al., 2021).
Starting after 2010, various plankton surveys recorded a transition in GoM C. finmarchicus populations to a decadal pattern of lower abundances, specifically there was a large decline in the fall and winter while spring abundances shifted higher (Sorochan et al., 2019; Ji et al., 2022; Pershing and Kemberling, 2023; Runge et al., in review). This transition coincided with rapid surface warming, and broader shifts in circulation patterns resulting in a greater influx of warm slope water into the GoM rather than cooler Labrador slope water (Meyer-Gutbrod et al., 2021; Pershing and Kemberling, 2023; Townsend et al., 2023). Shifts in the general oceanography and the flux of water masses entering the GoM have been hypothesized to impact late-stage abundance of C. finmarchicus in various ways. The higher temperatures likely impair their ability to overwinter (Maps et al., 2012; Runge et al., 2015), a shift in the timing of the spring bloom may lead to higher mortality rates (Honda et al., 2024), and the supply from sources within and upstream of the GoM may be diminished (Sorochan et al., 2019; Ji et al., 2022; Honda et al., 2023). At the same time, greater phytoplankton food availability in the late winter and early spring in the GoM enables increased early-stage C. finmarchicus abundance (Honda et al., 2024; Runge et al., in review). Moreover, increases in food availability in the fall, possibly due to decreased grazing pressure from older C. finmarchicus, has allowed the expansion of other mesozooplankton, particularly smaller copepods (Pershing and Kemberling, 2023).
Declines in the C. finmarchicus population have significant implications for dependent higher trophic levels. The oceanographic changes in the GoM and subsequent decline in C. finmarchicus have been linked to shifts in the migratory patterns of the critically endangered North Atlantic right whale (NARW). The NARW, which selectively preys on lipid-rich C. finmarchicus (Hudak et al., 2023), responded by moving to foraging grounds in the Gulf of Saint Lawrence, leading to an increased risk of gear entanglements and ship strikes (Record et al., 2019; Meyer-Gutbrod et al., 2021, 2023). The NARW population, which had been recovering, is now declining and classified as undergoing an unusual mortality event. Recruitment of Atlantic herring, another C. finmarchicus predator, has also been low (Northeast Fisheries Science Center (NEFSC), 2018), and while this decline is consistent with other climate related changes, it has not been directly linked to changes in C. finmarchicus abundance (Pershing et al., 2021). Likewise, the emblematic groundfish Atlantic cod feeds on C. finmarchicus in its larval stages and later larger prey in SBNMS including herring and sand lance, among others (Richardson et al., 2014; Pershing et al., 2021). Cod stocks have been at historic lows in the GoM, and while population abundance has been linked to C. finmarchicus elsewhere, it is a challenge to separate the decline here from overfishing (Beaugrand and Kirby, 2010; Pershing et al., 2015). Meanwhile, lobster recruitment declines in the GoM have been linked to lower abundances of C. finmarchicus and shifts in phenology, resulting in a mismatch between larvae released in the water column and food availability (Carloni et al., 2018, 2024). At the scale of the entire Northeast Shelf, sand lance populations are significantly correlated with C. finmarchicus abundance in years prior to 2010 (Suca et al., 2021), but it is unclear whether this relationship still holds, especially when focused on Stellwagen Bank. NARW, Atlantic herring, Atlantic cod, lobster, and sand lance are all focal species of SBNMS and are all vulnerable to climate change via C. finmarchicus, which is a foundational species for the whole GoM, including SBNMS (Office of National Marine Sanctuaries (ONMS), 2020). The NARW case especially exemplifies how rapid climate-driven changes in C. finmarchicus abundances can have downstream effects on sanctuary focal species, including those which feed on sand lance like humpback whales and great shearwaters. However, the potential climate impact on sand lance is unique and presents an opportunity for investigating circulation-mediated vulnerability because sand lance are non-migratory and thus narrowly dependent on C. finmarchicus abundance advected over the bank.
The GoM is connected to the broader North Atlantic Ocean by a circulation pattern that brings a mix of different water masses into its northeastern sector. Water masses enter through the Northeast Channel at depth and are generally transported west to the deep basins of the GoM: Georges Basin, Jordan Basin, and Wilkinson Basin (Townsend et al., 2015). The surface currents enter from the western Scotian Shelf in Canadian waters, turning west and then follow the coastline in a counter-clockwise direction, eventually exiting the GoM through the Great South Channel and southern flank of Georges Bank (Figure 1, Pettigrew et al., 2005). The transport of water centered on the 100 m isobath along the coast is named the Maine Coastal Current (MCC). The MCC is strongly influenced by the influx of fresh cold waters from the Scotian Shelf as well as the many inputs of riverine water and contains rich nutrient concentrations that sustains high primary productivity through the spring and summer months (Churchill et al., 2005). The MCC enters Massachusetts Bay, where it diverges into two branches, the main portion of which passes over the eastern edge of Stellwagen Bank towards the outside of Cape Cod (Jiang et al., 2007). While this describes the general pattern of currents in the GoM they can vary depending on broader climate driven oceanographic changes in the GoM (Townsend et al., 2023), and variability in freshwater inflow (Churchill et al., 2005; Pettigrew et al., 2005) and wind forcing (Jiang et al., 2007; Burkholder et al., 2024).
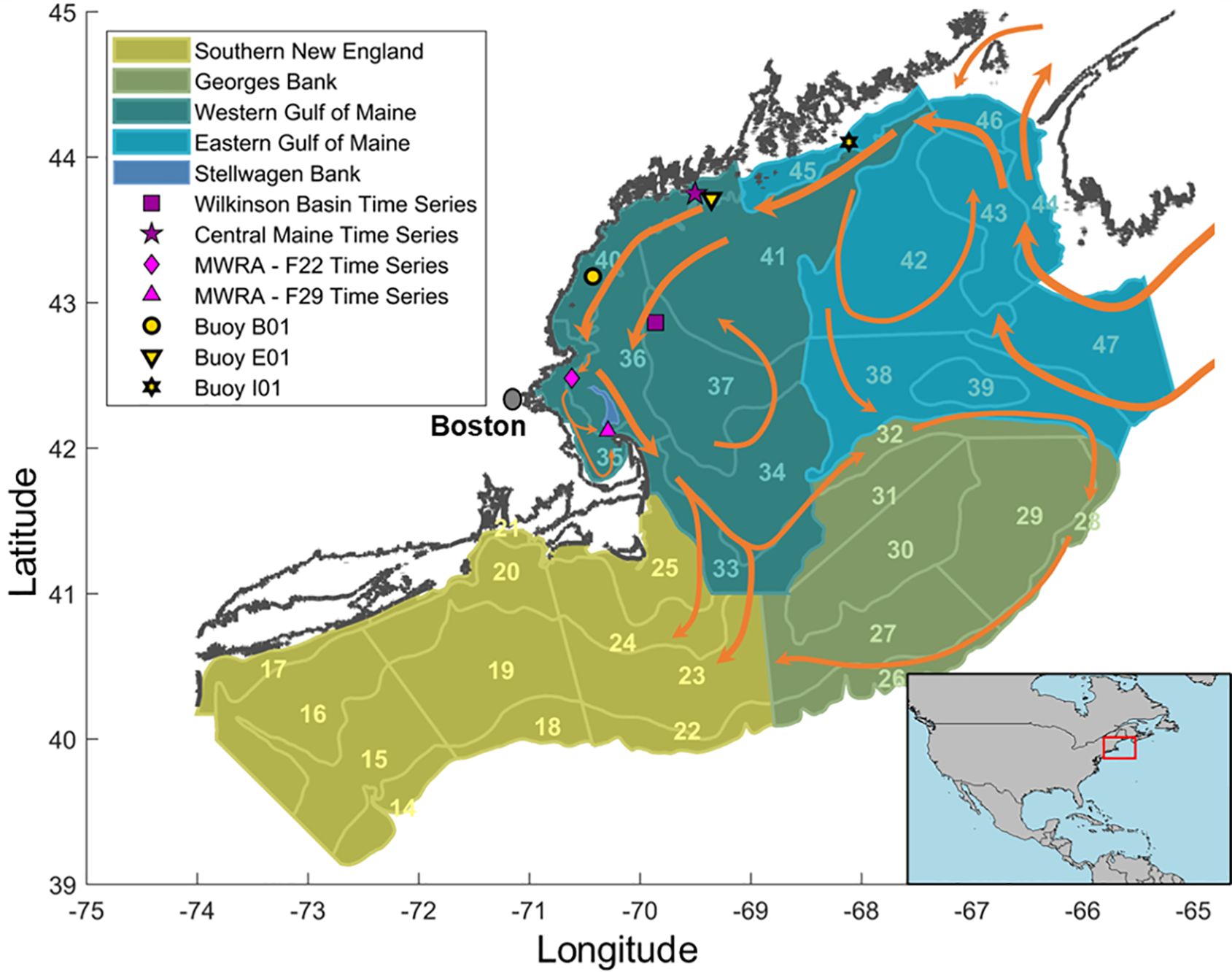
Figure 1. The Gulf of Maine, illustrating NOAA EcoMon survey strata divided by region, MBON and MWRA time-series station locations, NERACOOS buoy positions, and surface currents (<75 m depth) represented by orange arrows. The inset map highlights the study area (red box) within the broader geography.
It is hypothesized that the high abundances of C. finmarchicus found in Wilkinson Basin result from the combination of high primary productivity in the GoM and a favorable circulation pattern. This mechanism, known as CAST (Coastal Amplification and Supply and Transport), describes how abundant spring-through-summer food availability in the Maine Coastal Current (MCC) leads to rapid C. finmarchicus population growth, which is then advected to Wilkinson Basin, where the copepods enter diapause and accumulate at depth (Ji et al., 2017). Recent analyses of fixed-station time series data in the MCC and Wilkinson Basin in the western GoM have revealed that the population decline of C. finmarchicus over the past 15 years has been restricted to the fall and winter, while summer and spring abundances have remained the same or higher (Runge et al., in review). This finding supports the CAST mechanism and may also result from an increase in late winter/early spring phytoplankton in Wilkinson Basin (Runge et al., in review; Honda et al., 2024; Ji et al., 2017). In this study, we explore whether the CAST mechanism may also be responsible for supplying Stellwagen Bank with C. finmarchicus, thus linking SBNMS productivity and the foraging habitat of sand lance to upstream sources of C. finmarchicus. Since both C. finmarchicus abundance and GoM circulation patterns are impacted by climate change, this linkage also presents a possible indicator for monitoring SBNMS climate vulnerability. Our investigation employs ocean simulation modeling with Lagrangian particle tracking to conduct numerical experiments. Our specific objectives are to identify the upstream sources and to investigate seasonal and interannual variability in connectivity. Furthermore, we evaluate the connectivity between Stellwagen Bank and time series stations, from which observations of C. finmarchicus could serve as useful indicators of upstream productivity.
Methods
Ocean simulation model
Following Ji et al. (2017), we utilized the Finite-Volume Community Ocean Model (FVCOM) to simulate hydrographic and hydrodynamic conditions in the GoM region for our particle tracking numerical experiments. FVCOM is a prognostic, unstructured-grid, finite-volume, free-surface, three-dimensional primitive equation ocean model developed by the University of Massachusetts Dartmouth and the Woods Hole Oceanographic Institution (WHOI) (Chen et al., 2007). Designed to accurately represent complex coastal geometries and bathymetries, FVCOM is well-suited for modeling the intricate coastline and variable bathymetry of the GoM.
The model incorporates forcing fields specified from observational data and outputs from meteorological models to simulate realistic ocean conditions. In this study, we employed the third-generation Gulf of Maine-FVCOM (GoM-FVCOM) hindcasts covering the years 1978 to 2016, which are available through the Northeast Coastal Ocean Forecast System (NECOFS). GoM-FVCOM is a high-resolution circulation model of the northeast coastal system, with horizontal resolutions ranging from 0.3 to 10 km (Cowles et al., 2008; Chen et al., 2011). The FVCOM outputs from NECOFS provided forcing conditions necessary for running our individual-based model (IBM) simulations of copepod Lagrangian tracking.
Individual-based model – particle tracking
An IBM was employed to conduct Lagrangian particle tracking experiments representing the advective transport of C. finmarchicus within the study area. The IBM solves the advection equations using a fourth-order Runge-Kutta method, utilizing 10 second interpolated hourly flow fields from FVCOM to simulate the trajectories of individual particles (Ji et al., 2012; Boucher et al., 2013; Liu et al., 2015). The model operates in an offline mode, meaning that the physical oceanographic simulations were conducted prior to the IBM runs, and the stored flow fields from the FVCOM were used for particle tracking. The IBM generates a NetCDF file for each day of the numerical experiment containing the location of each particle. In our experiments, particles at fixed depths were tracked both forwards and backwards in time, allowing us to examine potential source regions and destinations of C. finmarchicus relative to Stellwagen Bank. If a particle’s depth exceeded the local bathymetry (i.e., if the water depth was less than the particle’s depth), the particle was adjusted to remain 1 m above the seafloor to prevent grounding. Note that in the backwards tracking experiments time is reversed such that the particle locations depend upon their earlier position in a sequence that is a later position in time. For consistency, we aim to describe the results from a forward time perspective.
Particle release locations and timeframes
In the backwards tracking experiments, all particles were released from Stellwagen Bank, which for purposes of the model was defined as the area within the sanctuary where depths are less than 40 m. This 182 km2 area corresponds to the sandy bank habitat of sand lance (Figure 1). In the forward tracking experiments, the particles were released from 25 – 140 km2 polygons centered over the location of time series stations where there has been long-term monitoring of C. finmarchicus population abundance. By examining connectivity between these stations and Stellwagen Bank, we aimed to determine whether data collected at these stations could inform us about the C. finmarchicus populations advected over the bank, thereby providing an indicator of foraging habitat for the SBNMS.
The time series stations fall under the Massachusetts Water Resources Authority’s (MWRA) outfall monitoring program or the Northeastern Region Association of Coastal Ocean Observing System’s (NERACOOS) Gulf of Maine Marine Biological Observation Network (MBON) program. The MWRA has been conducting environmental monitoring since 1992 to detect changes in the water column which may be caused by the effluent outfall of treated wastewater. The monitoring program includes monthly observation of several different biological and environmental measures including net tows for identification and enumeration of mesozooplankton (Werme and Hunt, 2001). Since its inception 29 stations have been sampled for zooplankton, 14 of which were still in use as of 2020, and from that group two stations were selected based on presence of C. finmarchicus, proximity to the bank, and water depth. Station F22 is northwest of the bank at 42.480°N, 70.618°W in 80 m of water, and Station F29 is to the south at 42.117°N, 70.290°W in 65 m of water.
The NERACOOS MBON (Marine Biodiversity Observation Network) time series stations follow protocols similar to the Atlantic Zone Monitoring Program (Mitchell et al., 2002) and are strategically positioned for monitoring C. finmarchicus population dynamics in the GoM (see Runge et al., in review). The Coastal Maine Timeseries Station (CMTS: 43.747° N, 69.502° W; depth 110 m) is situated 8 km offshore of the Damariscotta estuary at the margin of the highly productive Maine Coastal Current. The Wilkinson Basin Time series Station (WBTS: (42.914° N, 69.786° W); depth 257 m) is located 60 km east of Portsmouth, NH. at the northwest corner of Wilkinson Basin, the deep basin in the western GoM where overwintering populations of C. finmarchicus accumulate in great numbers. The time series started in 2005 and 2008, respectively at the WBTS and CMTS stations.
As summarized in Table 1, particles were released every year from 1978 to 2016 daily during the period when sand lance are primarily feeding on C. finmarchicus according to Suca et al. (2021). The backwards run releases at Stellwagen Bank were from March 1st to June 30th and at depths of 1, 15, and 50 m. The particles were then allowed to be advected backwards in time according to the flow fields for 30 days. In the forward release runs from the time series stations the releases were done for all years, daily between February 1st and May 31st with particle tracking lasting 60 days. The release days reflect the approximate time it is expected to take a C. finmarchicus to develop from egg to adult, according to the Belehradek temperature function at 5°C it takes 66 days and at 10°C it takes 35 (Campbell et al., 2001). During the early stages the copepods are near the surface but as they develop they begin to vertically migrate each day between layers of greater food concentration and depth. The release depths attempt to capture this variability across their life history. At the MWRA stations the release depth was 15 m while particles at the MBON stations were released at 1, 15, 50 and 150 m with the deepest depth representing those diapausing pre-adults.
Connectivity analysis
Given the extensive data generated from the experimental runs (over 162 million individual time stamped particle positions) it was too computationally intensive to track each particle’s exact location continuously. To manage this complexity, we examined particle locations at discrete time points and aggregated the spatial data. For the backwards run originating from Stellwagen Bank and for the nearfield forward releases at the MWRA sites, the particle locations were evaluated every 5 days. The particles originating from the MBON time series stations were evaluated every 15 days. Particle locations were then binned according to 35 sampling strata defined by the NOAA Northeast Fisheries Science Center’s ecosystem monitoring (EcoMon) program (Figure 1). The EcoMon program conducts 2-4 broad-scale plankton surveys annually across the Northeast shelf and the GoM, using a stratified random design and including hydrographic and mesozooplankton observations. The strata are based on bathymetric and ecological similarities across the region (Sherman et al., 1984; Meise and O’Reilly, 1996; Kane, 2007), with an additional stratum created in this study for Stellwagen Bank to capture particles within this area. Connectivity was calculated as the percentage of particles in each stratum that originated from a specific release location and day. To account for the bias introduced by larger strata having a higher likelihood of containing a particle, we then normalized the percentage by the area of each stratum. This approach allowed us to quantify the relative connectivity between Stellwagen Bank and the various release locations seasonally and interannual. IBM numerical experiments and particle tracking using output NetCDF files was done in MATLAB (2022) using the WHOI high performance computing environment, all data analysis and visualization was performed using the R programming software (R Core Team, 2023), and the ggplot2 package.
Lagrangian and Eulerian comparison
Further analysis compared the results of the Lagrangian particle tracking experiments to Eulerian measures of currents from NERACOOS buoys E01, I01 and B01 upstream of Stellwagen Bank (Figure 1). The easternmost buoy, I01, is situated within the eastern Maine coastal current (EMCC). Further downstream, on the opposite side of the Penobscot Estuary, is E01, which lies in the western MCC just upstream of the CMTS station. Buoy B01 is the furthest west and south, but still within the MCC and well upstream of Stellwagen Bank (Pettigrew et al., 2011; Burkholder et al., 2024). The historic record of 14 m depth ADCP measured current at the buoys was downloaded from the University of Maine data portal for the years 2001 to 2022 (University of Maine 2023). Following Burkholder et al. (2024), the hourly velocity measures of the u (east-west) and v (north-south) components were low pass filtered to remove the tidal signal by applying a fifth order Butterworth filter with a cutoff period of 23.5 hours and the alongshore current for each buoy location was then calculated. A weekly mean for each measure of the current was calculated over all years to create a climatology, and for each year to use as an explanatory variable in statistical modeling.
For the model comparing the Lagrangian and Eulerian observations, we fitted a series of zero-inflated negative binomial models using the glmmTMB package in R (Brooks et al., 2017), which is suitable for count data exhibiting overdispersion and an excess of zeros. The general model structure had the response variable as the number of particles released from CMTS reaching Stellwagen Bank after 45 days, and any number and combination of buoy-measured current components as explanatory variables. A total of 124 model combinations were generated by systematically including the 9 explanatory variables in different combinations with a constraint that if an alongshore component was included in the model neither of the corresponding components from that buoy would be included. The best performing model was then chosen according to the Akaike information criteria (AIC) and parameter parsimony and evaluated for model assumptions using the DHARMa (Hartig, 2023) residual diagnostics package.
Results
Backward release, particle tracking experiments
The particles released from Stellwagen Bank and advected backward in time for 30 days originated mostly from within the western GoM. Among all the variations of release depth and number of days, the particles released at 1 m that were allowed to drift for 30 days had the lowest normalized mean percentage of particles originating from western GoM with 90.4%. Likewise, a negligible number of particles came from Georges Bank and southern New England, and less than 1.6% on average were from the eastern GoM. As many as 8% originated from out-of-bounds locations (i.e., not within a stratum), likely inner coastal areas.
A closer examination of the strata from which the particles came 30 days prior shows that they are mostly upstream of Stellwagen Bank and follow the general flow of the MCC (Figures 1, 2). The majority of particles are from stratum 36, which envelops Stellwagen Bank and extends upstream to the north; the next most are found in stratum 35, which abuts 36 to the west and south. Among those further off, stratum 40 is the most connected; this stratum contains the CMTS station and the western MCC, which hugs the coast on its path towards Stellwagen Bank. Generally, the upstream strata in the path of the MCC (strata, 41, 45) see a decline of particles with fewer drifting days, while the number of particles in and around Stellwagen Bank steadily increases. Presumably longer drifting times would enable more particles to originate from these further off upstream strata, but by then a C. finmarchicus copepod would more likely be diapausing at depth or contributing to the next generation. The particle depth enhances the trend, with shallow water particles being more connected to further-off strata than deep particles. Stratum 37, which is defined by Wilkinson Basin, has notably little connectivity to Stellwagen Bank compared to the other strata, with the highest mean percentage (1%) origin found at a depth of 15 m, 30 days prior to arrival at Stellwagen Bank. There appears to be some retention on Stellwagen Bank, with 10.6–11.1% of the particles found there from the three release depths at day -30, but these particles may have been transported away and then returned.
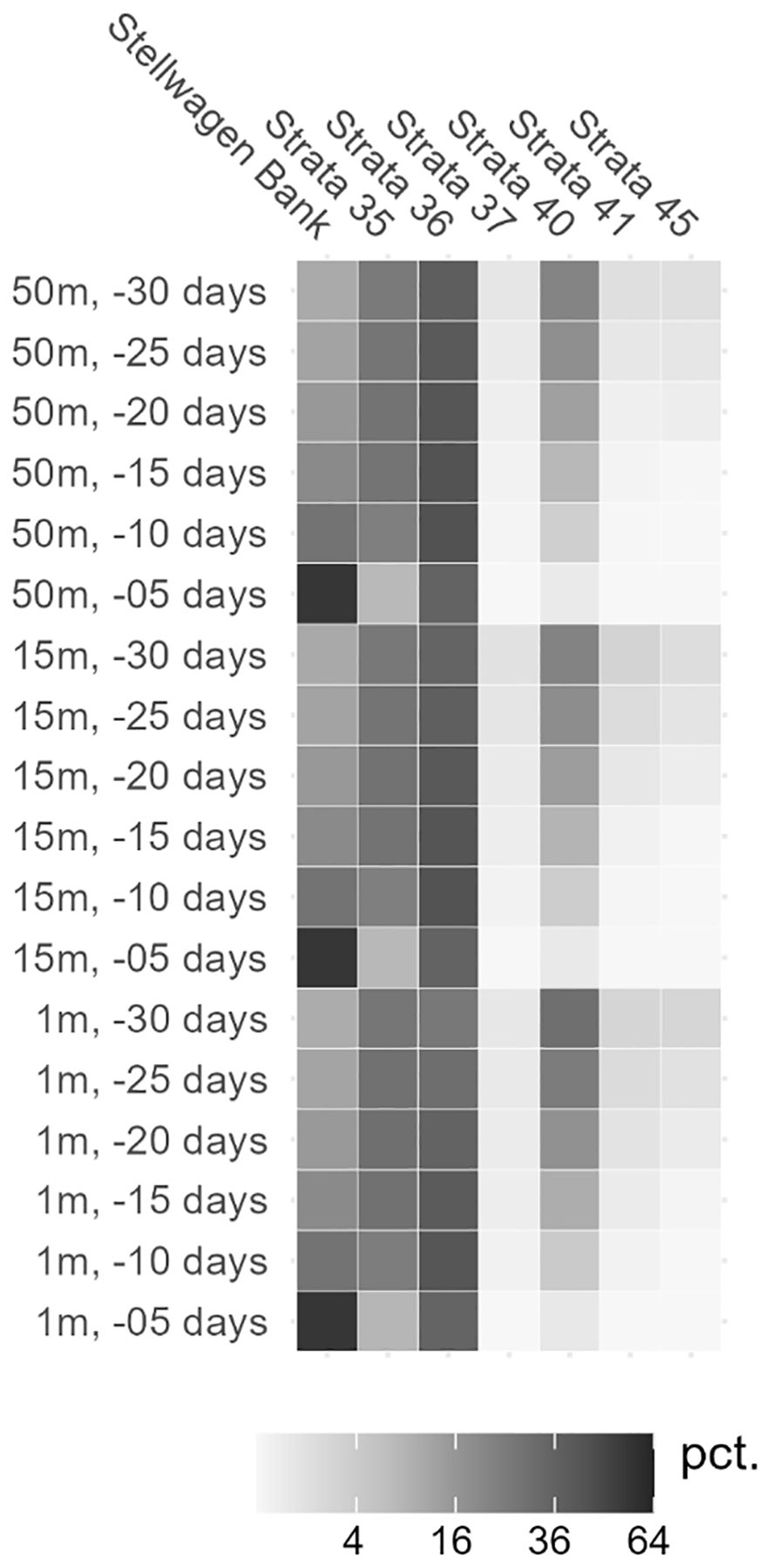
Figure 2. Particle origin in Backward Tracking Release from Stellwagen Bank. The normalized mean percentage of particles in select strata across all release dates is displayed according to particle depth and days since release. Percentages were square-root transformed to better display the differences between strata, with the legend indicating the untransformed value.
The connectivity between strata and Stellwagen Bank has a seasonal pattern, as shown by the percentage of particles originating from the strata 30 days prior to release (Figure 3). The area just upstream, stratum 36, is most highly connected in early spring, then its connectivity decreases through early summer before increasing again. Meanwhile, the coastal strata 35 and 40 show the opposite trend of low connectivity in early spring, which then increases towards summer. For the less connected strata, seasonal patterns are not as clearly indicated in the backward tracking experimental data. Still, in those more connected strata where seasonality is apparent, the variability across the 39 years of experimental runs is quite high, as indicated by the rapid day-to-day changes in connectivity and the standard error, which can span nearly 10 percentage points.
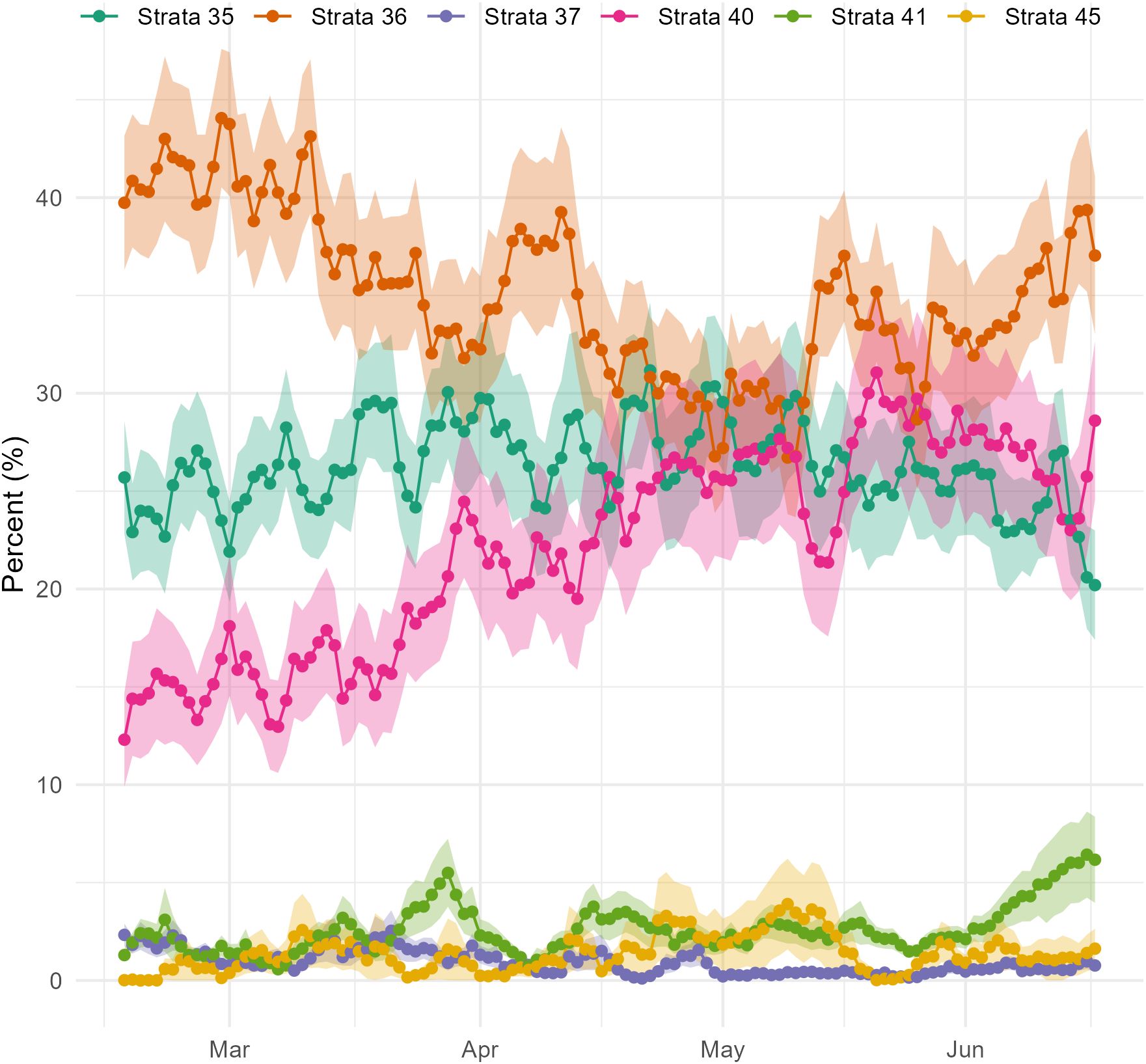
Figure 3. Seasonal Pattern of Particle Origin in Backward Tracking Release from Stellwagen Bank. The normalized mean percentage of particles at 15 m depth (solid line) and the standard error (shaded area) in selected strata 30 days prior to advection onto Stellwagen Bank, with the release date indicated on the x-axis (e.g., percent of particles in strata on April 1 corresponding to a release date of May 1). The midpoint of each month is indicated.
Interannually, there is likewise a high degree of variability year to year for each stratum, but there are also some apparent longer-term trends (Figure 4). Between 2000 and 2011, the connectivity between Stellwagen Bank and stratum 40 is elevated relative to other years, as is stratum 41 to a lesser degree. Similarly, stratum 36 has more years of higher connectivity prior to 1995 than after. The data also indicate some variable connectivity between Wilkinson Basin (stratum 37) and Stellwagen Bank that ranges from 0.4% to 4.3%. The difference between overall mean connectivity and any given year can be substantial; for example, in 2005, the mean percentage of particles originating from stratum 40 was 48.5%, a 26.4 percentage point difference from the mean. Less connected strata can also have years of high connectivity, with the difference between stratum 45’s mean and 1980 being 12.3 percentage points.
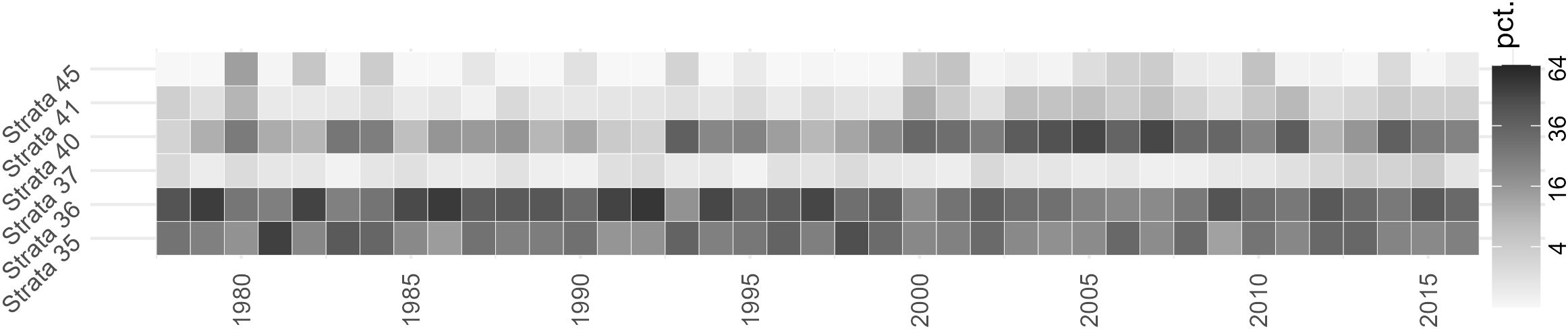
Figure 4. Interannual variability of particle origin in Backward Tracking Release from Stellwagen Bank, showing the normalized mean percentage of particles at 15 m depth in selected strata 30 days prior to advection onto Stellwagen Bank. Percentages were square root transformed to better display the differences between strata, with the legend indicating the untransformed value.
Forward release particle tracking experiments
Once released from the zones around the time series stations, the daily progressing mean percentage of particles in the strata reflects the general flow pattern (Figures 1, 5). As with the backward releases, the depth of the particle modulates the pattern, with those at the surface being transported faster. Particles released from CMTS at 1 m are most concentrated in stratum 40 at the start but then decline while their numbers in other strata increase. Particles at 1 m depth increase in strata 36 and 37 up to day 30 before declining, but those at deeper depths continue to increase. At all depths, the concentration of CMTS particles in the strata of 33, 34, 35, and Stellwagen Bank is highest 45 days after release. Particles released from the WBTS station likewise increase in concentration at Stellwagen Bank over time, but the number in stratum 36 decreases at all depths over time, and the relatively high concentration of particles that are advected to Georges Bank (GB) and southern New England (SNE) from WBTS indicates a more southern and eastern flow. To a much higher degree than the other release locations, particles from F22 and F29 can be found in their origin stratum 35, which is a relatively shallow coastal stratum and indicates some amount of retention. A surprising number of particles released from F22 and F29 are transported against the general flow of currents towards the north and east into strata 40 and 37. Like the WBTS release, many of the particles are further transported to SNE. Likely owing to their proximity, the connectivity between the MWRA stations and Stellwagen Bank is relatively high; for F29, the percentage varies little with time, while those released from F29 reach their peak on day 15 for the 15 m release. Focusing just on the 15 m depth, we can identify the time periods for the greatest connectivity between the stations and Stellwagen Bank for further analysis: 45 days for CMTS, 45 days for WBTS, 15 days for F22, and 5 days for F29.
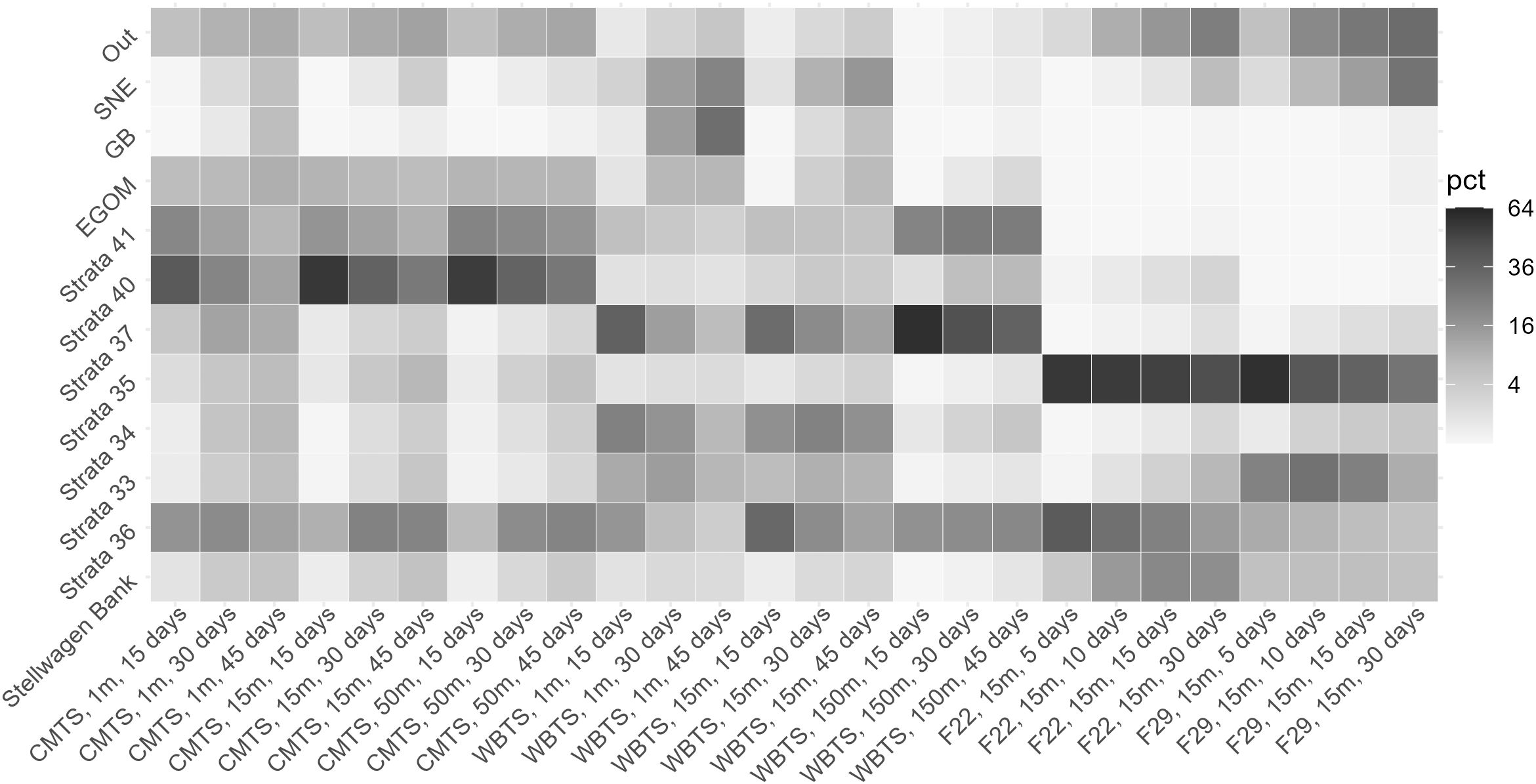
Figure 5. Time series station connectivity to strata in Forward Tracking Experiments. The normalized mean percentage of particles released, from locations shown on x-axis reaching select strata on y-axis, according to particle depth and days since release. While the individual strata were normalized by area, the regions were not: Southern New England (SNE), Georges Bank (GB), Eastern Gulf of Maine (EGOM). Percentages were square root transformed to better display the differences between strata, with the legend indicating the untransformed value.
As with the backward tracking experiments, connectivity in the forward releases displays seasonality (Figure 6) and significant interannual variability (Figure 7). In this analysis, we examined only particles released at 15 m depth during days when connectivity with Stellwagen Bank was highest. From late March through mid-May, particles released from CMTS have an increasing chance of reaching Stellwagen Bank. The proportion of particles from CMTS reaching stratum 36 peaks at the beginning of April and decreases for release dates earlier or later in the year. During times when the percentage reaching stratum 36 is elevated, we observe a decrease in the percentage transported to stratum 40. Meanwhile, particles released from WBTS have their highest connectivity with stratum 36 and Stellwagen Bank in late winter, which decreases through the spring as more particles are advected southeast toward stratum 34. Particles released from F22 showed very little seasonality, while connectivity between F29 and stratum 36 was higher in late winter.
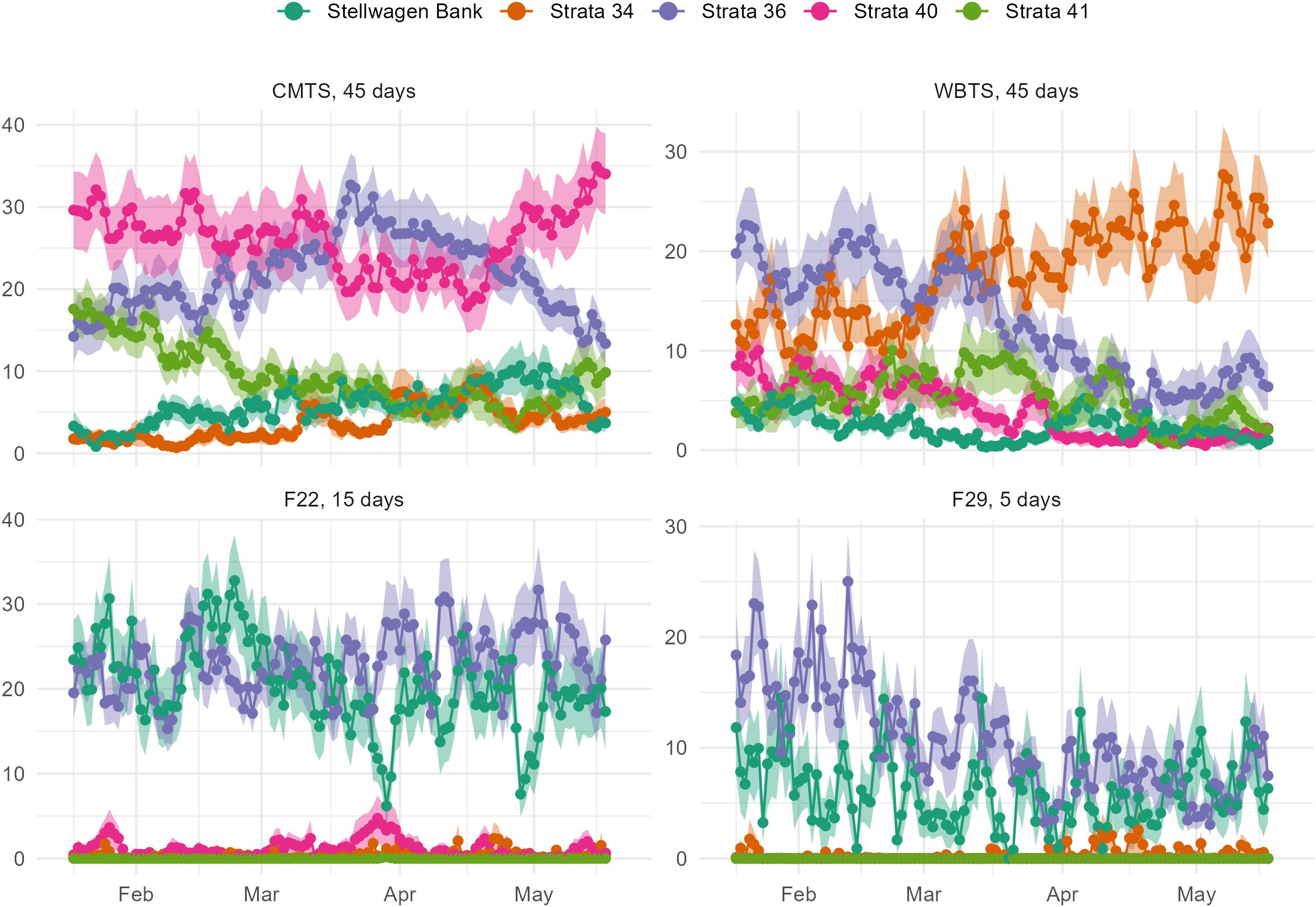
Figure 6. Seasonal pattern of connectivity in Forward Tracking Release from Time Series Stations. The normalized mean percentage of particles at 15 m depth (solid line) and the standard error (shaded area) in selected strata after drifting the indicated number of days from the station release zones: CMTS, 45 days; WBTS, 45 days; F22, 15 days; F29, 5 days. The release date is shown on the x-axis with the midpoint of each month.
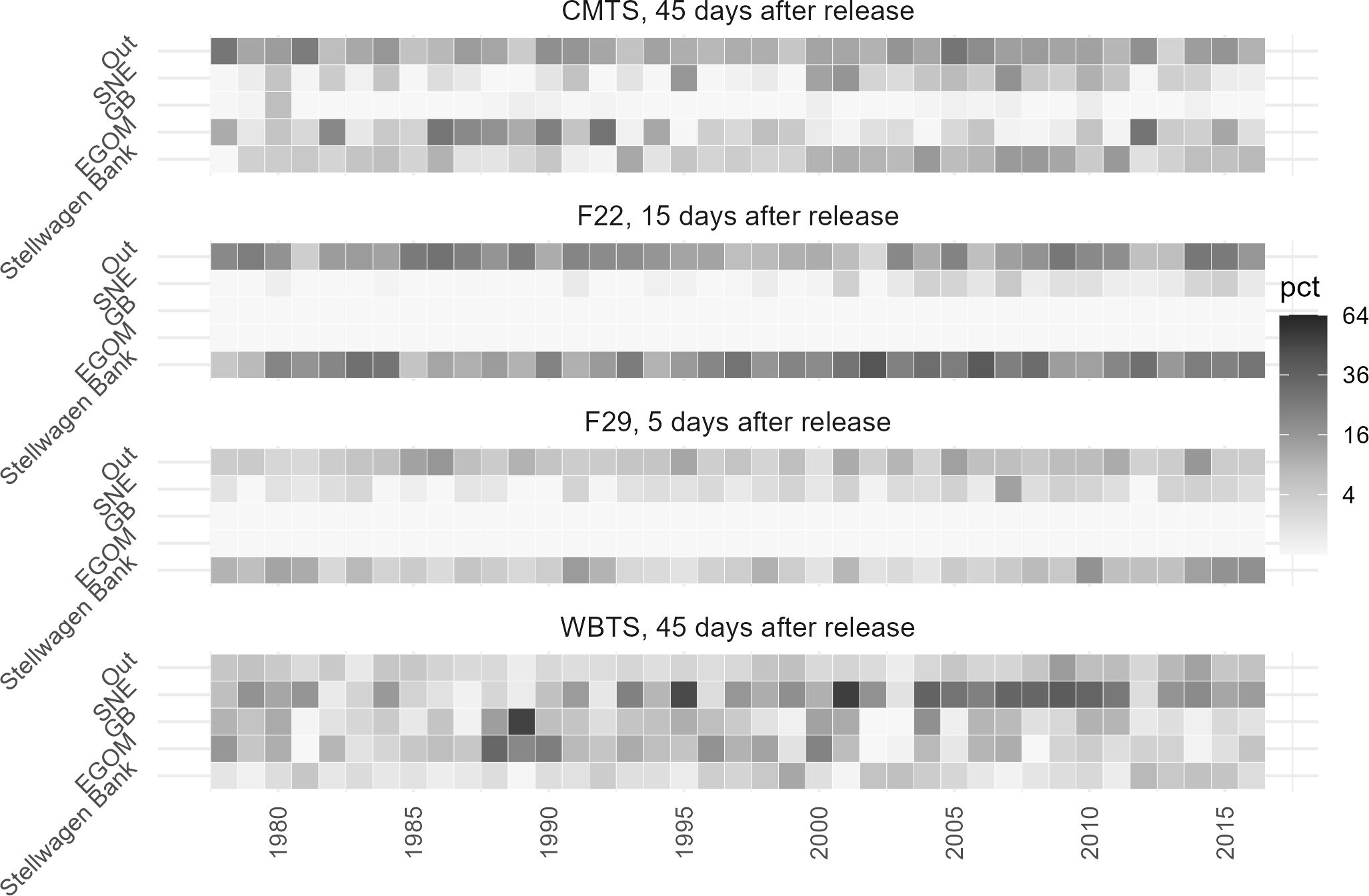
Figure 7. Interannual Variability of Particle Origin in Forward Tracking Release from Time Series Stations. The normalized mean percentage of particles at 15 m depth in selected strata, after the indicated number days released. Data were square root transformed to better display the differences between strata, with the legend indicating the untransformed value.
Since there are four different release zones in the forward-release scenarios, rather than just Stellwagen Bank in the backward releases, we are able to see indications that the interannual variability across them is not random. Rather, it appears that there are perturbations in the larger-scale pattern of circulation that simultaneously affect connectivity of all releases within a year. For example, as with the backward release we see elevated connectivity between CMTS in stratum 40 and Stellwagen Bank between 2000 and 2011 (Figure 7). In those same years, there is an increase in connectivity between F22 and F29 with Stellwagen Bank, while the number of particles released from WBTS heading to southern New England increases. Another notable pattern was the increase in the number of particles from CMTS heading to the eastern GoM between 1986 and 1992, and for a shorter period in the same timeframe, particles from WBTS were transported to southern New England and Georges Bank. Thus, we see that on longer timescales, there are larger changes in advective flow in the GoM which can affect the connectivity between Stellwagen Bank and upstream time series stations.
Lagrangian and Eulerian comparison
The 2001–2023 climatology of alongshore currents at the three buoys demonstrates seasonality that corresponds to patterns seen in the particle tracking experiments. In late spring, there is a nearly 10 cm/s increase in current speed at Buoy I01, which lasts from mid-April through the end of October (Figure 8). Buoy B01 likewise sees an increase in current speed in the spring, but the current then begins to decline at the end of May through October. These spring increases in current speed correspond in time with the greater connectivity observed in the particle tracking experiments between CMTS or stratum 40 and Stellwagen Bank in the spring. Likewise, they correspond to the decline in connectivity between Stellwagen Bank and stratum 36, perhaps indicating that the higher speeds are transporting the particles past the bank at a faster rate. Buoy E01 has the least apparent seasonality, with a relatively small decline in current speed during the fall. Nevertheless, the selected model predicting the number of particles on Stellwagen Bank released from CMTS included Buoy E01’s east-west current component along with Buoy B01’s east-west current component. The model coefficients and their significance tests are summarized in Table 2. The selected model had an AIC of 1,598, which is 2,139 less than the null, and 2.6 greater than the model with the lowest AIC, which was not chosen because it had two additional parameters. According to residual diagnostic tests, there were no issues with excessive residual deviance (K-S test p-value = 0.95), dispersion (p-value = 0.392), or outliers (p-value = 0.54).
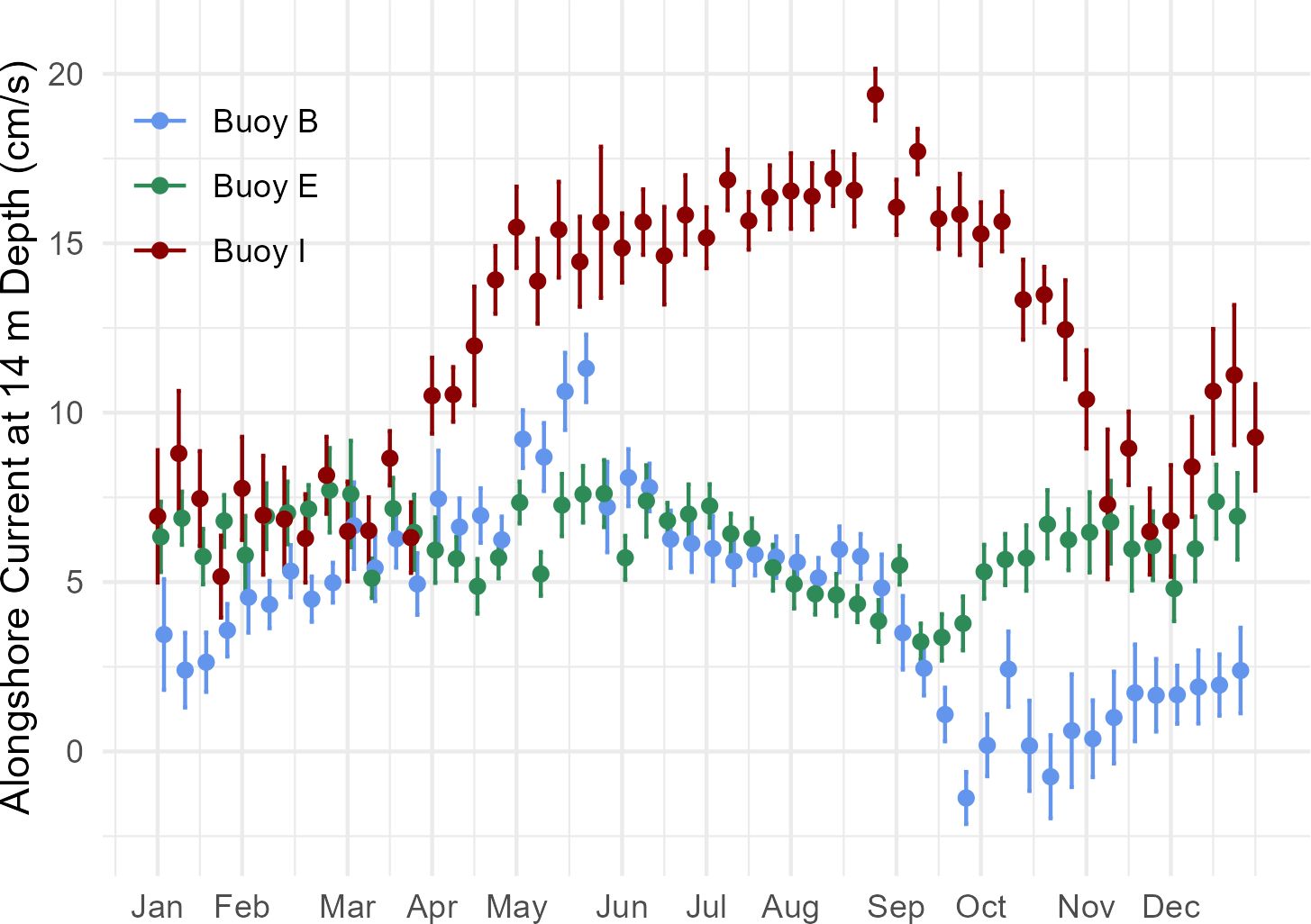
Figure 8. Climatology of alongshore current speed at 14 m depth from 2001–2023 for three buoys at their specified bearing: Buoy B, 217.12° (blue); Buoy E, 249.50°, (green); and Buoy I, 238.81° (red). The data points represent weekly means, with standard errors. A jitter was added to prevent overlap of points.
Although the Buoy B01 east-west component was included in the selected model, indicating that it helps improve predictive performance, the parameter itself was not significant in determining the number of particles reaching Stellwagen Bank or the number of zero counts. The mean value of the east-west component at Buoy E01 is negative indicating an overall westward flow, with a weekly mean range of 2.8 to -9.9 cm/s. The model shows that as the westward current at Buoy E01 increases, becoming more negative, there is an increased likelihood that particles will reach Stellwagen Bank and a decreased likelihood that no particles will be found. Specifically, when focusing only on Buoy E01 with no current at B01, a 1 cm/s increase in the western component from 5 cm/s to 6 cm/s increases the expected number of particles reaching Stellwagen Bank by 0.9, while reducing the probability of a zero count by 2.5 percentage points. This is a substantial connectivity increase when considering that the mean number of particles reaching Stellwagen bank for each week is 19.8, if you ignore the zero counts which comprise 49% of the observations.
Discussion
The particle tracking numerical experiments align with our conceptual understanding of the mean circulation pattern in the GoM and highlight its seasonality and interannual variability in a novel manner. Particle tracking models are frequently used to demonstrate retention and connectivity, but previous studies focused on limited years (e.g. Huret et al., 2007; Ji et al., 2012), specific days of the year (e.g. Boucher et al., 2013), mean values (e.g. Suca et al., 2022), or a specific process of interest (e.g., Churchill et al., 2011). By quantifying the spring-to-summer connectivity over 39 years, our results illustrate long-term patterns of connectivity in the GoM, further support the CAST hypothesis (Ji et al., 2017), and extend its application to the supply of C. finmarchicus to Stellwagen Bank and beyond. Although several upstream strata, including those that host the MBON time series stations, are connected to Stellwagen Bank, we identified stratum 36 to the immediate north as the primary source. Thus, C. finmarchicus entrained in the MCC or aggregating in Wilkinson Basin are first advected to stratum 36 and may then be transported over the bank. Notably, we observe that the greatest connectivity between Stellwagen Bank and CMTS occurs in late spring when lipid-rich stages of C. finmarchicus at the time series station are abundant and sand lance on the bank are actively feeding. We further find the greatest connectivity between WBTS and strata 36 in late winter when females are emerging from diapause and seeding the next generation of C. finmarchicus (Runge et al., in review). Although C. finmarchicus are observed to be seasonally present at the time series stations in the area immediately surrounding Stellwagen Bank, relating the abundance there to the rest of the GoM is challenging due to differing sampling protocols (Costa et al., 2023). Still, these coastal zones encompassed by stratum 35 may be an important and more consistent source of C. finmarchicus supply to the sandy bank.
Population spatial structure and habitat connectivity are well-recognized considerations for managing fisheries stocks and MPAs, but usually in the context of fish reproduction and larval dispersal (Churchill et al., 2011, 2017; Kritzer and Liu, 2014; Suca et al., 2022). Here, we considered the role of C. finmarchicus and connectivity in the productivity of the SBNMS. Although we focus on the shallow sandy bank as foraging habitat for sand lance, C. finmarchicus play a vital ecological role for the whole Sanctuary as a grazer and prey for many species, including larval fish like cod and the NARW (Office of National Marine Sanctuaries (ONMS), 2020). Secondary production does occur over the bank, but the limited area and rapid exchange of water mean that advected supply will dominate the foraging habitat for sand lance and other planktivores (this study; Suca et al., 2022). Shoaling over the bank may also act as a mechanism for aggregating C. finmarchicus populations as they are transported from deeper waters to the shallow bank and become concentrated (see Sorochan et al., 2021). However, C. finmarchicus are pelagic species that migrate to depth to escape predation; the shallow bank prevents both diel vertical migration and diapause, exposing them to higher mortality (Baumgartner et al., 2011). When Calanus are unable to escape to depth, visual predators like sand lance can readily feed on the relatively large copepods, particularly the late stages, leading to a pattern of lower abundance over shallow shelf habitats (Baumgartner et al., 2011; Melle et al., 2014). Meanwhile, in the relatively shallow waters of the GoM, Wilkinson Basin provides an important refuge for Calanus populations despite predation at depth by non-visual predators, including chaetognaths, ctenophores, and siphonophores (Wiebe et al., 2022).
The pattern of lower C. finmarchicus abundance over shallow areas is most apparent during the overwintering period. It is well-documented that abundances of C. finmarchicus on Georges Bank reach an annual high during the spring when the population is growing, but as temperatures increase and the copepods develop to the diapausing stage, the population declines throughout the summer and fall (Meise and O’Reilly, 1996; Kane, 2007). Likewise, on Jeffreys Ledge, a narrow bank with a depth <50 m to the north of Stellwagen Bank, C. finmarchicus population abundances follow a similar pattern, with the lipid-rich CV stages observed at concentrations as high as 10 individuals per liter in late spring, then declining to non-existent by late fall, presumably due to visual predation (Runge and Jones, 2012). Limited observations have been made of C. finmarchicus populations on Stellwagen Bank, but data provided by Suca et al. (2021) indicate that, like other shallow areas, abundances are highest in the spring and decline toward fall. MWRA observations averaged over Massachusetts Bay also follow this seasonal pattern (Turner et al., 2006). Perhaps because the area of Stellwagen Bank is small and the water flux is high, the standing stock of C. finmarchicus is quickly replenished by advected supply from upstream sources. However, the relative importance of this connectivity to abundance is not clear when considering all the factors that control the population variability of C. finmarchicus and continues to be a point of discussion (Wiebe et al., 2022).
Recent studies have highlighted the predominant role of internal growth processes in shaping C. finmarchicus abundance in the GoM’s deep basins. Specifically, Ji et al. (2022) used scaling and sensitivity analyses to show that internal growth, rather than external supply, primarily drives seasonal fluctuations in population levels, with dynamics being most sensitive to mortality during the winter-spring period. Honda et al. (2023) corroborated these findings through synchrony analysis, demonstrating that the spring abundances of C. finmarchicus in the deep basins are largely influenced by heterogeneous internal dynamics. When these internal dynamics are similar across spatially separated strata, the Moran effect (1953) may produce synchrony between their abundances. Similarly, the abundance of C. finmarchicus in strata supplying the Stellwagen Bank foraging habitat may reflect distant areas due to their internal similarities, as observed across the entire western GoM on an interannual basis (Honda et al., 2023, unpublished data). While C. finmarchicus population abundances in any given region are influenced by both heterogeneous internal dynamics and advective supply, each subpopulation depends on these factors to different degrees. At one extreme, a seed supply of adult C. finmarchicus is advected into a region, but subsequent generation abundances are primarily determined by local reproduction, growth and mortality rates. At the other extreme, there is minimal local production, and the observed abundance is largely due to advection from upstream.
Based on the results here and our understanding of C. finmarchicus ecology in the GoM, we reason that the population over Stellwagen Bank is generally more influenced by external exchange than local population growth, with supply from the adjacent strata 35 and 36 supporting abundance over Stellwagen Bank. The populations of the supplying strata are then driven to a greater extent by internal dynamics, following Honda et al. (2023), but advective supply following the CAST hypothesis (Ji et al., 2017) is nonetheless important. This describes the general balance of processes as we understand it, but for any given time period, a “powershift” among these processes could occur (Ji et al., 2022), as suggested by the interannual variability in connectivity. In most years, the absolute abundance of C. finmarchicus in the western GoM may be primarily influenced by internal dynamics, but the external supply of a seed stock may still pose a potential bottleneck. In the winter months, the abundance of C. finmarchicus in the western MCC can be below detectable levels at the relatively shallow CMTS station (see Runge et al., in review) and likewise at the MWRA stations (MWRA, unpublished data). For a new generation to establish in the absence of a resident population, some individuals must be transported in from upstream, and that number will influence abundance after a given time. Potentially, the local abundance of C. finmarchicus on Stellwagen Bank could be stifled when population growth is low and its connectivity to seeding areas –such as the upstream MCC or the deep overwintering basins— is reduced, limiting the absolute supply of the copepod prey.
Given the substantial variability observed in our experiments and the potential climate impact on the foraging habitat of Stellwagen Bank, monitoring upstream C. finmarchicus abundance and modeling its connectivity is warranted. Suca et al. (2021) found that over the whole Northeast Shelf, the abundance of age 2–3 year-old sand lance was correlated to the population of C. finmarchicus 3–4 years earlier, when the parental generation of those observed sand lance would have been feeding prior to fall spawning. Thus, FVCOM hindcast ocean simulations with particle tracking, combined with time series observations and other environmental variables, could be used to build a forecast of habitat suitability and climate vulnerability. Alternatively, or in addition to the Lagrangian measure of connectivity, we may rely on current speeds at buoys to provide a Eulerian measure. Since these long-term observations of currents have been in place, there has been a significant decline in current speed, particularly in spring, attributed to an increase in southwesterly winds (Burkholder et al., 2024). As demonstrated here, reduced current speed diminishes the connectivity between the MCC and Stellwagen Bank, which, if the trend continues, may manifest in decreased abundances of C. finmarchicus on Stellwagen Bank. The model suggested here would provide both a useful forecast of C. finmarchicus abundance over Stellwagen Bank or other areas and would elucidate the variable influences of advected supply and internal production. Notably, long-term data sets of abundance and connectivity are vital for building indices used in the model and emphasizes the importance of continued monitoring. Fortunately, the MWRA and MBON time series stations have been monitored for 17-25 years and continue to be active. These stations may also inform secondary production in other regions downstream. Specifically, the experiments showed high connectivity between WBTS and southern New England, where NARW have been observed to be feeding in increasing numbers in recent years (National Academies of Sciences, Engineering, and Medicine, 2024).
In practice, it may be challenging to accurately forecast the abundance of C. finmarchicus in downstream areas due to the numerous factors involved, including the connectivity between a time series station and Stellwagen Bank—or any other area. However, monitoring and modeling would provide valuable insight into the confidence with which time series data can be used as an indicator for the habitat suitability and climate vulnerability of the downstream area. In a highly connected year, we would increase our confidence that the indicator would likely reflect similar conditions in both locales, versus a low-connectivity year where abundance may be higher at the time series location but not at Stellwagen Bank. This Bayesian approach can also be applied when examining data within the framework of synchrony, where the Moran effect is responsible for similarity between distinct spatial areas experiencing the same environmental forcing. When environmental indicators from two locations diverge, we can lower our confidence that a measure of C. finmarchicus abundance in one area will be representative of the other. Building on the findings presented here, the next steps toward developing a decision support tool for the SBNMS involve applying such an approach through statistical analysis. The statistical model could relate sand lance habitat to connectivity, C. finmarchicus abundance at various time series stations, and environmental factors like the influx of Warm Slope Water into the GoM. Demonstrated significant relationships would then support the use of those variables in forecasts of foraging habitat or as standalone indicators. Such information will be useful for stakeholders making data-driven decisions and will ultimately contribute to the resilience of the SBNMS in the face of ongoing climatic changes. For example, forecasts of favorable sand lance foraging habitat could signal a likely increase in humpback whale presence, aiding whale-watching operations in planning their season while simultaneously alerting other stakeholders to exercise caution during vessel transit to mitigate ship strikes. More generally, tracking the upstream abundance of C. finmarchicus and its connectivity to a downstream location could provide a valuable indicator of climate vulnerability because of the foundational role of C. finmarchicus in North Atlantic food webs. Here we focused specifically on Stellwagen Bank with broader implications for the whole Sanctuary, but this approach could readily be applied to any area or species of interest.
Conclusion
This study underscores the critical role of habitat connectivity in the Gulf of Maine for the ecological health of the Stellwagen Bank National Marine Sanctuary. Through Lagrangian particle tracking experiments, we demonstrated that the supply of Calanus finmarchicus to Stellwagen Bank is connected to upstream sources, particularly the Maine Coastal Current. Notably, we revealed previously undescribed seasonal patterns and large interannual variability in this connectivity—a finding that enhances our understanding of the region’s ecological dynamics. Understanding these dynamic connectivity patterns enhances our ability to predict ecological conditions at Stellwagen Bank based on upstream indicators. This insight is crucial for enabling stakeholders to make informed, data-driven decisions. Ultimately, accounting for the dynamics of habitat connectivity—including its seasonal and interannual variability—will contribute to the resilience of the Stellwagen Bank National Marine Sanctuary in the face of ongoing climatic impacts.
Data availability statement
The processed data supporting the findings of this study are provided as Supplementary Material. All other datasets utilized in the analysis, including buoy data and FVCOM hindcasts, are publicly available from their respective sources. The raw data generated from particle tracking experiments are extensive and are currently stored on the Woods Hole Oceanographic Institute servers but are not guaranteed to be stored indefinitely. These data can be made available upon reasonable request to the corresponding author.
Author contributions
CT: Conceptualization, Data curation, Formal analysis, Investigation, Methodology, Project administration, Resources, Software, Validation, Visualization, Writing – original draft, Writing – review & editing. IH: Data curation, Formal analysis, Investigation, Methodology, Software, Writing – original draft, Writing – review & editing. JK: Conceptualization, Funding acquisition, Project administration, Supervision, Writing – original draft, Writing – review & editing. JM: Conceptualization, Funding acquisition, Project administration, Supervision, Writing – original draft, Writing – review & editing. JR: Conceptualization, Funding acquisition, Investigation, Project administration, Supervision, Validation, Writing – original draft, Writing – review & editing. RJ: Conceptualization, Formal analysis, Funding acquisition, Investigation, Methodology, Project administration, Resources, Software, Supervision, Validation, Writing – original draft, Writing – review & editing.
Funding
The author(s) declare financial support was received for the research, authorship, and/or publication of this article. This work was primarily supported by the NOAA Climate Program Office (NA22OAR4310557), with additional support from the NOAA Marine Biodiversity Observation Network (NA24NOSX012C0020, NA19NOS0120197), NOAA Cooperative Institute for the North Atlantic Region (NA24OARX4320008) and the Bureau of Ocean Energy Management Environmental Studies program (M23AC00010).
Acknowledgments
We express our gratitude to the staff of the Stellwagen Bank National Marine Sanctuary, particularly Tammy Silva, Dave Wiley, and Ben Haskell, for their invaluable guidance and support throughout this project. We also thank Zhengchen Zang for his assistance with the FVCOM and IBM models, and Bob Fleming for providing the University of Maine buoy data. Our appreciation extends to the Massachusetts Water Resources Authority and their staff for supplying zooplankton data and for explaining the details of their monitoring program. We also acknowledge the advice and work of Justin Suca, whose contributions laid important groundwork for this study. Finally, we are deeply grateful to Joel Llopiz without whom this project would not have been possible.
Conflict of interest
The authors declare that the research was conducted in the absence of any commercial or financial relationships that could be construed as a potential conflict of interest.
Generative AI statement
The author(s) declare that no Generative AI was used in the creation of this manuscript.
Publisher’s note
All claims expressed in this article are solely those of the authors and do not necessarily represent those of their affiliated organizations, or those of the publisher, the editors and the reviewers. Any product that may be evaluated in this article, or claim that may be made by its manufacturer, is not guaranteed or endorsed by the publisher.
Supplementary material
The Supplementary Material for this article can be found online at: https://www.frontiersin.org/articles/10.3389/fmars.2025.1527073/full#supplementary-material
References
Battista T., Clark R., Pittman S. (2006). An ecological characterization of the Stellwagen Bank National Marine Sanctuary region: oceanographic, biogeographic, and contaminants assessment Vol. 45 (National Oceanic and Atmospheric Administration (NOAA)). Available at: https://repository.library.noaa.gov/view/noaa/2491 (Accessed December 12, 2024).
Baumgartner M., Lysiak N., Schuman C., Urban-Rich J., Wenzel F. (2011). Diel vertical migration behavior of Calanus finmarchicus and its influence on right and sei whale occurrence. Mar. Ecol. Prog. Ser. 423, 167–184. doi: 10.3354/meps08931
Beaugrand G., Kirby R. R. (2010). Climate, plankton and cod. Glob. Change Biol. 16, 1268–1280. doi: 10.1111/j.1365-2486.2009.02063.x
Boucher J. M., Chen C., Sun Y., Beardsley R. C. (2013). Effects of interannual environmental variability on the transport-retention dynamics in haddock Melanogrammus aeglefinus larvae on Georges Bank. Mar. Ecol. Prog. Ser. 487, 201–215. doi: 10.3354/meps10462
Bowman R. E. (2000). Food of northwest Atlantic fishes and two common species of squid (National Oceanic and Atmospheric Administration (NOAA)). Available at: https://repository.library.noaa.gov/view/noaa/3140/ (Accessed October 12, 2024).
Brooks M. E., Kristensen K., Van Benthem K. J., Magnusson A., Berg C. W., Nielsen A., et al. (2017). glmmTMB balances speed and flexibility among packages for zero-inflated generalized linear mixed modeling. R. J. 9, pp.378–pp.400. doi: 10.32614/RJ-2017-066
Burkholder K. C., Lee J. H., Kime M., Calabro C., Manning J. P. (2024). Decadal-scale variability in the surface flow of the gulf of maine coastal current: the impact of changing climate conditions on coastal circulation. J. Geophys. Res.: Oceans. 129, e2023JC020512. doi: 10.1029/2023JC020512
Campbell R., Wagner M., Teegarden G., Boudreau C., Durbin E. (2001). Growth and development rates of the copepod Calanus finmarchicus reared in the laboratory. Mar. Ecol. Prog. Ser. 221, 161–183. doi: 10.3354/meps221161
Carloni J. T., Wahle R. A., Fields D. M., Geoghegan P., Shank B. (2024). Diverging phenology of American lobster (Homarus americanus) larvae and their zooplankton prey in a warming ocean. ICES. J. Mar. Sci. 81, 918–928. doi: 10.1093/icesjms/fsae051
Carloni J. T., Wahle R., Geoghegan P., Bjorkstedt E. (2018). Bridging the spawner-recruit disconnect: trends in American lobster recruitment linked to the pelagic food web. Bull. Mar. Sci. 94, 719–735. doi: 10.5343/bms.2017.1150
Chen C., Huang H., Beardsley R. C., Liu H., Xu Q., Cowles G. (2007). A finite volume numerical approach for coastal ocean circulation studies: Comparisons with finite difference models. J. Geophys. Res. 112, 2006JC003485. doi: 10.1029/2006JC003485
Chen C., Huang H., Beardsley R. C., Xu Q., Limeburner R., Cowles G. W., et al. (2011). Tidal dynamics in the Gulf of Maine and New England Shelf: An application of FVCOM. J. Geophys. Res.: Oceans. 116. doi: 10.1029/2011JC007054
Churchill J. H., Kritzer J. P., Dean M. J., Grabowski J. H., Sherwood G. D. (2017). Patterns of larval-stage connectivity of Atlantic cod (Gadus morhua) within the Gulf of Maine in relation to current structure and a proposed fisheries closure. ICES. J. Mar. Sci. 74, 20–30. doi: 10.1093/icesjms/fsw139
Churchill J. H., Pettigrew N. R., Signell R. P. (2005). Structure and variability of the western maine coastal current. Deep. Sea. Res. Part II. Top. Stud. Oceanogr. 52, 2392–2410. doi: 10.1016/j.dsr2.2005.06.019
Churchill J. H., Runge J., Chen C. (2011). Processes controlling retention of spring-spawned Atlantic cod (Gadus morhua) in the western Gulf of Maine and their relationship to an index of recruitment success. Fish. Oceanogr. 20, 32–46. doi: 10.1111/j.1365-2419.2010.00563.x
Costa A., Goodwin C., Hudak C., McKenna B. (2023). Water Column Monitoring Results for Cape Cod Bay and Stellwagen Bank National Marine Sanctuary 2020-2022 (Boston: Massachusetts Water Resources Authority). Available online at: https://www.mwra.com/harbor/enquad/pdf/2023-05.pdf (Accessed August 9, 2024).
Cowles G. W., Lentz S. J., Chen C., Xu Q., Beardsley R. C. (2008). Comparison of observed and model-computed low frequency circulation and hydrography on the New England Shelf. J. Geophys. Res.: Oceans. 113. doi: 10.1029/2007JC004394
DeLorenzo Costa A., Durbin E., Mayo C. (2006). Variability in the nutritional value of the major copepods in cape cod bay (Massachusetts, USA) with implications for right whales. Mar. Ecol. Prog. 27, 109–123. doi: 10.1111/j.1439-0485.2006.00087.x
Grieve B. D., Hare J. A., Saba V. S. (2017). Projecting the effects of climate change on Calanus finmarchicus distribution within the U.S. Northeast Continental Shelf. Sci. Rep. 7, 6264. doi: 10.1038/s41598-017-06524-1
Hartig F. (2023). DHARMa: Residual Diagnostics for Hierarchical (Multi-Level/Mixed) Regression Models, R package version 0.4.6. Available online at: https://CRAN.R-project.org/package=DHARMa.
Honda I. A., Ji R., Britten G. L., Thompson C., Solow A. R., Zang Z., et al. (2024). Shifting phenology as a key driver of shelf zooplankton population variability. Limnol. Oceanogr. doi: 10.1002/lno.12752
Honda I. A., Ji R., Solow A. R. (2023). Spatially varying plankton synchrony patterns at seasonal and interannual scales in a well-connected shelf sea. Limnol. Oceanogr. Lett. 8, 906–915. doi: 10.1002/lol2.10348
Hudak C., Stamieszkin K., Mayo C. (2023). North Atlantic right whale Eubalaena glacialis prey selection in Cape Cod Bay. Endang. Species. Res. 51, 15–29. doi: 10.3354/esr01240
Huret M., Runge J., Chen C. S., Cowles G., Xu Q. C., Pringle J. M. (2007). Dispersal modeling of fish early life stages: sensitivity with application to atlantic cod in the western gulf of maine. Mar. Ecol. Prog. Ser. 347, 261–274. doi: 10.3354/meps06983
Ji R., Ashjian C. J., Campbell R. G., Chen C., Gao G., Davis C. S., et al. (2012). Life history and biogeography of Calanus copepods in the Arctic Ocean: An individual-based modeling study. Prog. Oceanogr. 96, 40–56. doi: 10.1016/j.pocean.2011.10.001
Ji R., Feng Z., Jones B. T., Thompson C., Chen C., Record N. R., et al. (2017). Coastal amplification of supply and transport (CAST): a new hypothesis about the persistence of Calanus finmarchicus in the Gulf of Maine. CES. J. Mar. Sci. 74, 1865–1874. doi: 10.1093/icesjms/fsw253
Ji R., Runge J. A., Davis C. S., Wiebe P. H. (2022). Drivers of variability of Calanus finmarchicus in the Gulf of Maine: roles of internal production and external exchange. CES. J. Mar. Sci. 79, 775–784. doi: 10.1093/icesjms/fsab147
Jiang M., Brown M., Turner J., Kenney R., Mayo C., Zhang Z., et al. (2007). Springtime transport and retention of Calanus finmarchicus in Massachusetts and Cape Cod Bays, USA, and implications for right whale foraging. Mar. Ecol. Prog. Ser. 349, 183–197. doi: 10.3354/meps07088
Johnson C. L., Leising A. W., Runge J. A., Head E. J. H., Pepin P., Plourde S., et al. (2008). Characteristics of Calanus finmarchicus dormancy patterns in the Northwest Atlantic. CES. J. Mar. Sci. 65, 339–350. doi: 10.1093/icesjms/fsm171
Kane J. (2007). Zooplankton abundance trends on Georges Bank 1977-2004. ICES. J. Mar. Sci. 64, 909–919. doi: 10.1093/icesjms/fsm066
Kritzer J. P., Liu O. R. (2014). “Chapter three - fishery management strategies for addressing complex spatial structure in marine fish stocks,” in Stock Identification Methods (Second Edition). Eds. Cadrin S. X., Kerr L. A., Mariani S. (Academic Press, San Diego), 29–57. doi: 10.1016/B978-0-12-397003-9.00003-5
Liu C., Cowles G. W., Churchill J. H., Stokesbury K. D. E. (2015). Connectivity of the bay scallop (Argopecten irradians) in Buzzards Bay, Massachusetts, U.S.A. Fish. Oceanogr. 24, 364–382. doi: 10.1111/fog.12114
Maps F., Runge J. A., Leising A., Pershing A. J., Record N. R., Plourde S., et al. (2012). Modelling the timing and duration of dormancy in populations of Calanus finmarchicus from the Northwest Atlantic shelf. J. Plankton. Res. 34, 36–54. doi: 10.1093/plankt/fbr088
MATLAB. (2022). R2022a MATLAB and Simulink, MathWorks, Natick, Massachusetts version 9.12. Available online at: https://www.mathworks.com/.
Meise C. J., O’Reilly J. E. (1996). Spatial and seasonal patterns in abundance and age-composition of Calanus finmarchicus in the Gulf of Maine and on Georges Bank: 1977–1987. Deep. Sea. Res. Part II. Top. Stud. Oceanogr. 43, 1473–1501. doi: 10.1016/S0967-0645(96)00048-3
Melle W., Runge J., Head E., Plourde S., Castellani C., Licandro P., et al. (2014). The North Atlantic Ocean as habitat for Calanus finmarchicus: Environmental factors and life history traits. Prog. Oceanogr. 129, 244–284. doi: 10.1016/j.pocean.2014.04.026
Meyer-Gutbrod E. L., Davies K. T. A., Johnson C. L., Plourde S., Sorochan K. A., Kenney R. D., et al. (2023). Redefining North Atlantic right whale habitat-use patterns under climate change. Limnol. Oceanogr. 68, S71–S86. doi: 10.1002/lno.12242
Meyer-Gutbrod E., Greene C., Davies K., Johns D. (2021). Ocean regime shift is driving collapse of the north atlantic right whale population. Oceanog 34, 22–31. doi: 10.5670/oceanog.2021.308
Mitchell M. R., Harrison G., Paule K. (2002). “Atlantic zonal monitoring program sampling protocol,” in Canadian Technical Report of Hydrography and Ocean Sciences (Dartmouth, Nova Scotia Canada: Bedford Institute of Oceanography), 223.
Murray C. S., Wiley D., Baumann H. (2019). High sensitivity of a keystone forage fish to elevated CO2 and temperature. Conserv. Physiol. 7, coz084. doi: 10.1093/conphys/coz084
National Academies of Sciences, Engineering, and Medicine. (2024). Potential Hydrodynamic Impacts of Offshore Wind Energy on Nantucket Shoals Regional Ecology: An Evaluation from Wind to Whales (Washington, D.C: National Academies Press). Available online at: https://www.nap.edu/catalog/27154 (Accessed October 16, 2023).
Northeast Fisheries Science Center (NEFSC) (2018). 65th Northeast Regional Stock Assessment Workshop (65th SAW) Assessment Summary (Woods Hole, MA). doi: 10.25923/vvpm-jy75
ONMS. (2020). 2020 Condition Report: Findings of Status and Trends for 2007-2018 (Silver Spring, MD: Department of Commerce, National Oceanic and Atmospheric Administration, Office of National Marine Sanctuaries. Silver Spring, MD), 263 pp. Available at: https://nmssanctuaries.blob.core.windows.net/sanctuaries-prod/media/docs/2020-stellwagen-condition-report.pdf.
Pershing A. J., Alexander M. A., Brady D. C., Brickman D., Curchitser E. N., Diamond A. W., et al. (2021). Climate impacts on the Gulf of Maine ecosystem: A review of observed and expected changes in 2050 from rising temperatures. Elementa.: Sci. Anthropocene. 9, 76. doi: 10.1525/elementa.2020.00076
Pershing A. J., Alexander M. A., Hernandez C. M., Kerr L. A., Le Bris A., Mills K. E., et al. (2015). Slow adaptation in the face of rapid warming leads to collapse of the Gulf of Maine cod fishery. Science 350, 809–812. doi: 10.1126/science.aac9819
Pershing A. J., Kemberling A. (2023). Decadal comparisons identify the drivers of persistent changes in the zooplankton community structure in the northwest Atlantic. ICES. J. Mar. Sci. 81, fsad198. doi: 10.1093/icesjms/fsad198
Pershing A. J., Stamieszkin K. (2020). The north atlantic ecosystem, from plankton to whales. Annu. Rev. Mar. Sci. 12, 339–359. doi: 10.1146/annurev-marine-010419-010752
Pettigrew N. R., Churchill J. H., Janzen C. D., Mangum L. J., Signell R. P., Thomas A. C., et al. (2005). The kinematic and hydrographic structure of the Gulf of Maine Coastal Current. Deep. Sea. Res. Part II. Top. Stud. Oceanogr. 52, 2369–2391. doi: 10.1016/j.dsr2.2005.06.033
Pettigrew N. R., Fikes C. P., Beard M. K. (2011). Advances in the ocean observing system in the gulf of maine: technical capabilities and scientific results. Mar. Technol. Soc J. 45, 85–97. doi: 10.4031/MTSJ.45.1.11
Powers K. D., Wiley D. N., Robuck A. R., Olson Z. H., Welch L. J., Thompson M. A., et al. (2020). Spatiotemporal characterization of non-breeding Great Shearwaters Ardenna gravis within their wintering range. Mar. Ornithol. 48, pp.215–pp.229. doi: 10.5038/2074-1235.48.2.1376
R Core Team (2023). R: A language and environment for statistical computing R version 4.3.1 (Vienna, Austria: R Foundation for Statistical Computing). Available at: https://www.R-project.org/.
Record N. R., Ji R., Maps F., Varpe Ø., Runge J. A., Petrik C. M., et al. (2018). Copepod diapause and the biogeography of the marine lipidscape. J. Biogeogr. 45, 2238–2251. doi: 10.1111/jbi.13414
Record N. R., Runge J. A., Pendleton D. E., Balch W. M., Davies K. T. A., Pershing A. J., et al. (2019). Rapid climate-driven circulation changes threaten conservation of endangered north atlantic right whales. Oceanography 32, 162–169. doi: 10.5670/oceanog.2019.201
Richardson D. E., Palmer M. C., Smith B. E. (2014). The influence of forage fish abundance on the aggregation of Gulf of Maine Atlantic cod (Gadus morhua) and their catchability in the fishery. Can. J. Fish. Aquat. Sci. 71, 1349–1362. doi: 10.1139/cjfas-2013-0489
Runge J. A., Ji R., Thompson C. R. S., Record N. R., Chen C., Vandemark D. C., et al. (2015). Persistence of Calanus finmarchicus in the western Gulf of Maine during recent extreme warming. J. Plankton. Res. 37, 221–232. doi: 10.1093/plankt/fbu098
Runge J. A., Jones R. J. (2012). Results of a collaborative project to observe coastal zooplankton and ichthyoplankton abundance and diversity in the western gulf of maine: 2003-2008. Am. Fish. Soc Symp. 79, 345–359.
Sherman K., Smith W., Morse W., Berman M., Green J., Ejsymont L. (1984). Spawning strategies of fishes in relation to circulation, phytoplankton production, and pulses in zooplankton off the north-eastern United States. Mar. Ecol. Prog. Ser. 18, 1–19. doi: 10.3354/meps018001
Sorochan K. A., Plourde S., Baumgartner M. F., Johnson C. L. (2021). Availability, supply, and aggregation of prey (Calanus spp.) in foraging areas of the North Atlantic right whale (Eubalaena glacialis). ICES. J. Mar. Sci. 78, 3498–3520. doi: 10.1093/icesjms/fsab200
Sorochan K. A., Plourde S., Morse R., Pepin P., Runge J., Thompson C., et al. (2019). North Atlantic right whale (Eubalaena glacialis) and its food: (II) interannual variations in biomass of Calanus spp. on western North Atlantic shelves. J. Plankton. Res. 41, 687–708. doi: 10.1093/plankt/fbz044
Staudinger M. D., Goyert H., Suca J. J., Coleman K., Welch L., Llopiz J. K., et al. (2020). The role of sand lances (Ammodytes sp.) in the Northwest Atlantic Ecosystem: A synthesis of current knowledge with implications for conservation and management. Fish. Fish. 21, 522–556. doi: 10.1111/faf.12445
Suca J. J., Ji R., Baumann H., Pham K., Silva T. L., Wiley D. N., et al. (2022). Larval transport pathways from three prominent sand lance habitats in the Gulf of Maine. Fisheries. Oceanogr. 31, 333–352. doi: 10.1111/fog.12580
Suca J. J., Pringle J. W., Knorek Z. R., Hamilton S. L., Richardson D. E., Llopiz J. K. (2018). Feeding dynamics of Northwest Atlantic small pelagic fishes. Prog. Oceanogr. 165, 52–62. doi: 10.1016/j.pocean.2018.04.014
Suca J. J., Wiley D. N., Silva T. L., Robuck A. R., Richardson D. E., Glancy S. G., et al. (2021). Sensitivity of sand lance to shifting prey and hydrography indicates forthcoming change to the northeast US shelf forage fish complex. ICES. J. Mar. Sci. 78, 1023–1037. doi: 10.1093/icesjms/fsaa251
Townsend D. W., Pettigrew N. R., Thomas M. A., Moore S. (2023). Warming waters of the Gulf of Maine: The role of Shelf, Slope and Gulf Stream Water masses. Prog. Oceanogr. 215, 103030. doi: 10.1016/j.pocean.2023.103030
Townsend D. W., Pettigrew N. R., Thomas M. A., Neary M. G., McGillicuddy D. J., O’Donnell J. (2015). Water masses and nutrient sources to the gulf of maine. J. Mar. Res. 73, 93–122. doi: 10.1357/002224015815848811
Turner J., Borkman D., Hunt C. (2006). Zooplankton of Massachusetts Bay, USA 19922003: relationships between the copepod Calanus finmarchicus and the North Atlantic Oscillation. Mar. Ecol. Prog. Ser. 311, 115–124. doi: 10.3354/meps311115
Werme C., Hunt C. D. (2001). “Outfall monitoring overview,” in Report ENQUAD, vol. 10. (Massachusetts Water Resources Authority, Boston), 92.
Keywords: copepod, secondary production, Gulf of Maine, connectivity, phenology, population dynamics, marine protected area, particle tracking model
Citation: Thompson CRS, Honda IA, Kritzer JP, Motyka J, Runge JA and Ji R (2025) Modeling the advective supply of Calanus finmarchicus to Stellwagen Bank as an indicator of sand lance foraging habitat, and the climate vulnerability of a National Marine Sanctuary. Front. Mar. Sci. 12:1527073. doi: 10.3389/fmars.2025.1527073
Received: 12 November 2024; Accepted: 06 January 2025;
Published: 27 January 2025.
Edited by:
Randi D. Rotjan, Boston University, United StatesReviewed by:
Peter J. Auster, Mystic Aquarium, United StatesBoris Espinasse, UiT The Arctic University of Norway, Norway
Copyright © 2025 Thompson, Honda, Kritzer, Motyka, Runge and Ji. This is an open-access article distributed under the terms of the Creative Commons Attribution License (CC BY). The use, distribution or reproduction in other forums is permitted, provided the original author(s) and the copyright owner(s) are credited and that the original publication in this journal is cited, in accordance with accepted academic practice. No use, distribution or reproduction is permitted which does not comply with these terms.
*Correspondence: Cameron R. S. Thompson, Y2FtZXJvbkBuZXJhY29vcy5vcmc=