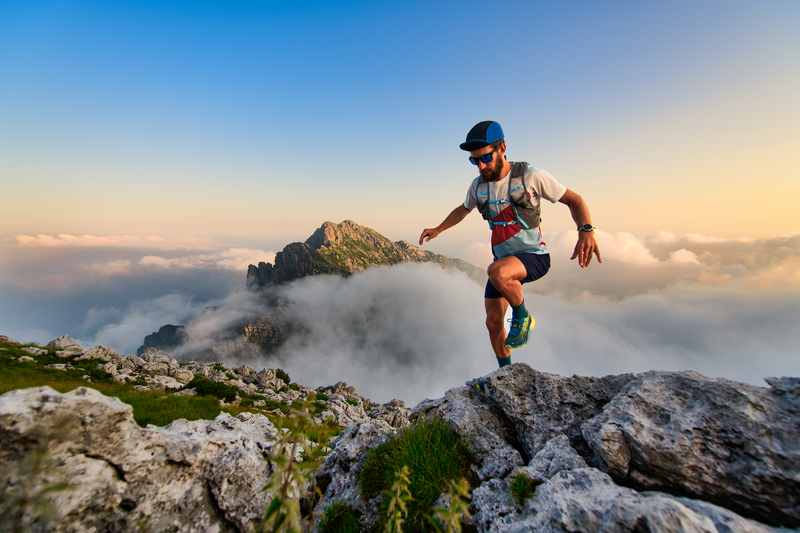
95% of researchers rate our articles as excellent or good
Learn more about the work of our research integrity team to safeguard the quality of each article we publish.
Find out more
ORIGINAL RESEARCH article
Front. Mar. Sci. , 11 March 2025
Sec. Marine Biogeochemistry
Volume 12 - 2025 | https://doi.org/10.3389/fmars.2025.1518631
As human activities intensify, ecosystems are constantly being polluted by microplastics, which may change the microbe-driven nitrogen cycling and associated nitrous oxide emissions therein. However, the exact impact of microplastics on specific nitrogen cycling processes remains to be clarified, limiting accurate assessments of nitrous oxide production. Additionally, a gap in our understanding of the isotopic dynamics of nitrogen cycling under the impact of microplastics restricts deeper insights into nitrogen cycling in microplastic-polluted environments. Accordingly, this study represents the first integration of natural abundance isotope techniques with microcosm experiments involving various microplastics, offering a novel approach for detailed investigation into the impacts of microplastics on the nitrogen cycle dynamics and their potential role in regulating nitrous oxide production. Our results suggest that microplastics of different sizes (0.02 mm, 0.1 mm, and 1 mm) and polymer types (polypropylene, polyvinyl chloride, polyamide, and polyethylene) impact both nitrite production and consumption, highlighting the important role of size in these processes. Particularly, nitrite dual isotopic signatures help identify specific nitrogen cycling processes impacted by microplastics. More importantly, isotopic evidence indicates that nitrite may be lost from the environment primarily by reduction to gaseous products nitrous oxide or dinitrogen in polyethylene and polyvinyl chloride, especially the largest-size polyamide treatments. Conversely, polypropylene treatment, especially at large sizes, may promote nitrite oxidation, thus retaining more nitrogen within the environment. Our findings offer a new paradigm for the comprehensive assessment of the impact of microplastics on the nitrogen cycle and highlight the importance of considering microplastics when assessing greenhouse gas emissions, especially in the context of increasing microplastic pollution.
Nitrite (NO2−) is a key intermediate in the nitrogen (N) cycle, which is involved in almost all N biogeochemical processes driven by microbes. Its main fate in the environment, being reduced via denitrification and anaerobic ammonium oxidation (anammox) or oxidized through NO2− oxidation, directly affects the reconciliation of the bioavailable N budget and the production of the greenhouse gas nitrous oxide (N2O) (Zhang et al., 2020). However, ecosystems have changed in recent decades due to human activities (e.g., environmental pollution), and the microbe-driven N cycle may also change accordingly (Fang et al., 2024; Greenfield et al., 2022; Hu et al., 2023; Li et al., 2020; Lyu et al., 2024; Seeley et al., 2020; Wang et al., 2024; Yu et al., 2022; Zhu et al., 2022), which necessitates a continuous updating of our knowledge of NO2− cycle and its associated production of N2O in a changing environment.
Microplastics (MPs, generally defined as tiny plastic fragments or particles with a diameter of <5 mm; Hartmann, 2019), an emerging environmental pollutant, are found in various ecosystems in different shapes, sizes, ages, and polymer types (Cluzard et al., 2015; de Souza MaChado et al., 2019; Song et al., 2023; Wang et al., 2023), and have an impact on the cycling of elements therein (Fang et al., 2024; Green et al., 2016; Greenfield et al., 2022; Hu et al., 2023; Hope et al., 2020; Li and Liu, 2022; Lyu et al., 2024; Rillig et al., 2021; Riveros et al., 2022; Seeley et al., 2020; Sun et al., 2023; Yin et al., 2023; Wang et al., 2024; Zhu et al., 2022). Sediment represents the ultimate sink for MPs in marine water (Lebreton et al., 2017) because MPs will continue to be deposited, albeit over a longer period of time. Since sediment is a hotspot for microbial activity, these settled MPs may have an impact on microbially mediated elemental cycling in the sediment, such as the N cycle (Chen et al., 2022a; Fang et al., 2024; Green et al., 2016; Hope et al., 2020; Lyu et al., 2024; Seeley et al., 2020) and carbon cycle (Ikenoue et al., 2024). This impact is now considered to stem from the formation of biofilms on the surface of MPs (Amaral-Zettler et al., 2020; Oberbeckmann et al., 2015; Pinnell and Turner, 2019; Su et al., 2022); the adsorption of other pollutants, nutrients, and organic matter by MPs (Ikenoue et al., 2022; Mato et al., 2001; Wang et al., 2020); the modification of the physicochemical properties of the sediment by MPs (Cluzard et al., 2015; de Souza MaChado et al., 2019; Fang et al., 2024; Wan et al., 2018; Zhang et al., 2019); and the release of MP additives (Azizi et al., 2021; Seeley et al., 2020; Vo and Pham, 2021). Based on these, MPs directly or indirectly impact the key microbes (e.g., nitrifiers, denitrifiers, and anammox bacteria), enzymes [e.g., ammonia monooxygenase, nitrate (NO3−) reductase, and NO2− reductase] and functional genes (e.g., amoA, nirS, and nirK) that regulate N transformation, and consequently the N cycling process (e.g., nitrification, denitrification, and anammox).
However, the exact impact of MPs on specific N cycle processes remains uncertain, limiting the accurate estimation of N2O production linked to these processes (Wang et al., 2024). In addition, there is still a gap in the understanding of the isotopic dynamics of the N cycle under MP pollution, limiting the in-depth understanding of the N cycle in modern MP-polluted environments. The natural abundance stable isotope techniques may be able to address these issues, as the N cycle is composed of various N transformations driven by diverse microbes that operate to give unique isotopic signatures to N species (Buchwald and Casciotti, 2013; Casciotti, 2016a, 2016b; Chen et al., 2021, Chen et al., 2022a; Chen and Chen, 2022). These isotopic signatures can trace the sources, transformations, internal cycles, and losses of N (Buchwald and Casciotti, 2013; Casciotti, 2016a, 2016b; Chen et al., 2021, 2022; Chen and Chen, 2022). However, this approach is not yet in the field, leaving an unanswered question regarding the potential impact of MPs on the N and oxygen (O) isotope dynamics in the N cycle. Furthermore, the impact of MP size on N cycling has not yet been well discussed. This is obviously an issue that needs to be addressed (Lee et al., 2022; Li and Liu, 2022; Wang et al., 2024) because changes in MP size can impact their own physical disturbance, adsorption, and biofilm formation, which may have an impact on N cycling processes (Amaral-Zettler et al., 2020; Cluzard et al., 2015; de Souza MaChado et al., 2019; Oberbeckmann et al., 2015; Pinnell and Turner, 2019; Su et al., 2022; Wan et al., 2018; Wang et al., 2020; Zhang et al., 2019). Taken together, we have gradually recognized the impact of MPs on the N cycle (Chen et al., 2022b; Fang et al., 2024; Greenfield et al., 2022; Hu et al., 2023; Li et al., 2020; Lyu et al., 2024; Seeley et al., 2020; Wang et al., 2024; Yu et al., 2022; Zhu et al., 2022), but an accurate understanding of the N cycle under MP pollution remains elusive due to the above-mentioned knowledge gaps.
The natural abundance of N [δ15NNO2 = (15N/14NSample/15N/14NAir − 1) × 1000‰] and O [δ18ONO2 = (18O/16OSample/18O/16OVSMOW − 1) × 1000 ‰] isotope ratios of NO2− are ideal tools for probing the NO2− cycle (Buchwald and Casciotti, 2013; Casciotti, 2016a, 2016b; Chen et al., 2021; Chen et al., 2022a; Chen and Chen, 2022). Accordingly, with the aid of this tool, MPs of different sizes (0.02 mm, 0.1 mm, and 1 mm) and polymer types [polypropylene (PP), polyvinyl chloride (PVC), polyamide (PA), and polyethylene (PE)] were deployed to provide detailed information on NO2− cycling in the context of MP pollution (Supplementary Figure S1).
Sediment samples were collected in July 2023 from the coastal intertidal zone of Haizhou Bay, Western Yellow Sea, China, following low tide, and simultaneously, ambient seawater was also collected at the same site. The sediments were sieved on-site to remove larger impurities and macroorganisms, and subsequently dried in air and sieved through a 2mm stainless steel mesh to achieve complete homogenization and removal of small impurities and interstitial water (Seeley et al., 2020). Concurrently, ambient seawater was filtered on-site using a 0.45 µm Millipore membrane prior to use.
The microcosm experimental design includes four commonly used MP treatments (PP, PVC, PA, and PE, reviewed in Wang et al., 2024) and a no MP control treatment, with replicates per treatment (Supplementary Figure S1). These MPs are commercially available (Shenzhen Guangyuan Plasticisation Co., Ltd., China; high purity, greater than 99%) and are made in specific sizes as per the requirements of our experiments. MPs of different polymer types were further treated at different sizes (0.02 mm, 0.1 mm, and 1 mm; n = 26; Supplementary Figure S1). It needs to be clarified that the specific size of the MPs selected may not be fully representative of the size characteristics of MPs in diverse sediments, but rather reflect the range of sizes in which MPs may be present in environmental sediments (reviewed in Wang et al., 2024). The choice of these MPs was based on the main types of MPs commonly observed in the water column at the location where the sediment samples were collected (Song et al., 2023), in order to ensure a realistic representation of actual conditions. Furthermore, these are also the types of MPs that are frequently selected in other studies (reviewed in Wang et al., 2024), thus enabling a meaningful comparison. A relatively practical concentration of MPs (0.5% w/w) was set for the experiment based on available studies (Baysal et al., 2020; Fang et al., 2024; Seeley et al., 2020; Wang et al., 2024). We weighed 300 grams of the above fully homogenized sediment into a 1L beaker (sediment sample depth of 3 cm) and added the different polymer types and sizes of MPs mentioned above to achieve a consistent concentration (weight ratio to wet sediment), followed by seawater addition without disturbing the sediment as much as possible (Seeley et al., 2020). Prior to the start of the incubation experiment, the microcosm was gently aerated to maintain oxygen in the overlying water and to allow an oxic/anoxic gradient to develop in the sediment over a 24-hour period (Seeley et al., 2020). The control treatment followed the same steps as the MP treatment except that no MPs were added. Subsequently, the experiments were operated at room temperature (20°C), and each beaker was covered with a piece of tin foil for the duration of the experiments to prevent water evaporation or other disturbances caused by other factors.
The overlying water samples were collected at 30 mL on days 1, 3, 7, 18, and 25 of the experimental system. For NO2− concentration analysis, the samples were measured immediately or frozen for later measurement. For NO2− dual isotope analysis, 6 M sodium hydroxide was added to preserve the samples (prevent O isotope exchange between NO2− and H2O) for isotopic measurement (Casciotti et al., 2007; Chen et al., 2021, 2022; Chen and Chen, 2022).
NO2− concentration was determined on a QuAAtro flow analyzer (Seal, Germany) by the flow injection technique using a Greiss-Ilosvay colorimetric method (Strickland and Parsons, 1972), with steps including reagent preparation (sulfanilamide and N-(1-naphthyl) ethylenediamine), and correction of blanks and refractive index.
The δ15NNO2 and δ18ONO2 were determined using the well-established azide reduction method (McIlvin and Altabet, 2005). Depending on the NO2− concentration, an appropriate sample volume (calculated based on an optimal injection size of 20 nmol for N2O formed by the sample) was pipetted into a pre-acid washed and scorched 20 mL headspace vial. The vial was then sealed with an aluminum cap and silica pad, and purged with helium to remove any N2O present (Chen et al., 2021; Chen et al., 2022a; Chen and Chen, 2022). The reaction reagents in the azide reduction method include sodium azide and acetic acid solutions, which were prepared and used on the same day. These solutions were added to a 50 mL headspace vial in a 1:1 ratio (the volume added is determined by the number of samples) and purged with helium for half an hour (Chen et al., 2021; Chen et al., 2022a; Chen and Chen, 2022). Since the sample had a high pH with the addition of sodium hydroxide, the concentration of the acetic acid solution needed to be increased to 7.84 M in order to achieve the conditions for optimal reaction rate (pH = 4.5, Chen et al., 2021; Chen et al., 2022a; Chen and Chen, 2022; Hu et al., 2016). After purging, 0.9 mL of sodium azide-acetic acid buffer solution was transferred to the sample vial with a syringe for reaction, and the reaction was ended by adding 0.5 mL of 10 M sodium hydroxide at 1 hour (Chen et al., 2021; Chen et al., 2022a; Chen and Chen, 2022). The N2O produced by the reaction was automatically extracted, purified, concentrated, and analyzed online using a Thermo Finnigan Gasbench II purge-trap system interfaced with a DELTAplus XP isotope ratio mass spectrometer. To calibrate the isotope values of the samples, the NO2− isotopic standards (RSIL-N23, RSIL-N7373, and RSIL-N10219, Casciotti et al., 2007) were preserved and processed in the same procedure as the samples. A set of reference standards was inserted every 6–10 samples for analysis. The volume of the reaction solution was kept the same for each sample and standard to minimize the effect of pH in the azide reduction reaction (Granger et al., 2020; Kobayashi et al., 2021). The N content of the standards was matched to the samples to minimize the effect of sample size on the isotopic analysis (Granger et al., 2020; Kobayashi et al., 2021). The mean standard deviations for all replicate measurements of samples and standards in this study were 0.6‰ for δ15N and 0.4‰ for δ18O, respectively.
We found distinct changes in NO2− concentration at different incubation times (Figure 1). On day 18 of the incubation experiment, there was a significant increase in the NO2− concentration in both control and MP treatments compared to the initial and day 7 (Figure 1), with the highest NO2− concentration of 51.24 ± 5.09 μmol L-1 recorded in the PP-1mm treatment (Figure 1A). Therefore, this period belongs to the NO2− accumulation stage, which is similar to the previous results (Seeley et al., 2020; Zhu et al., 2022). Although the NO2− concentration increased in each treatment compared to the initial and day 7, differences were observed between the control and MP sediments, and between the MP treatments with different polymer types and sizes (Figure 1). The difference in NO2− accumulation concentration between the control and the MP treatments depended on the polymer type of the MPs, which either negatively or positively impacted NO2− accumulation (Figure 1), supporting the previously proposed role of MP polymer type in regulating N transformation (reviewed in Wang et al., 2024). Notably, our results clearly identify the role of MP size in NO2− production (Figure 2). Interestingly, the degree of NO2− accumulation increased with increasing size in the PA treatment (Figure 2), with the concentration being progressively higher than in the control. In particular, the greatest increase in NO2− concentration was found when the size changed from 0.02 to 0.1 mm (Figures 1B, C and 2). Similar scenarios were also observed in the PE, PVC, and PP treatments (Figures 1, 2).
Figure 1. Changes in nitrite concentration during the incubation experiment. (A) represents the case of MP treatments of different polymer type at the 1 mm setting and the control (CON, no MPs in the sediment), while (B, C) are the scenarios at the 0.02 mm and 0.1 mm settings, respectively. The black lines are error bars (n = 2 per treatment).
Figure 2. The impact of MP size on nitrite production during the nitrite accumulation stage. The red shading shows the 95% confidence intervals and grey lines are error bars.
The accumulation of NO2− may involve processes such as ammonia (NH3) oxidation, assimilatory NO3− reduction, and dissimilatory NO3− reduction (Buchwald and Casciotti, 2013; Casciotti, 2016a, 2016b; Chen et al., 2021; Chen et al., 2022a; Chen and Chen, 2022). Although assimilatory NO3− reduction is also responsible for NO2− production, this process was not considered in our microcosm due to the absence of phytoplankton. Thus, the different polymer types and sizes of MPs in this study have different impacts on the degree of NO2− accumulation, implying that MP treatments with higher (or lower) NO2− concentrations may promote (or impede) NO2− production, NH3 oxidation, or dissimilatory NO3− reduction, as compared to the control (See further isotopic analyses below).
Upon reaching day 25, the NO2− concentration in each treatment showed a declining trend in comparison to day 18 (Figure 1), with the PP-1mm treatment showing the greatest decline, suggesting that this stage was characterized by the consumption of NO2−. Similar to the previous stage, the size and polymer type of MPs also played different roles in NO2− consumption (Figure 1). As the size increased, the degree of decrease in NO2− concentration in the PA, PE, PVC, and PP treatments all showed an increasing trend (Supplementary Figure S2) and were greater than that of the control. Furthermore, the greatest decrease in NO2− concentration was also recorded when the size varied between 0.02 mm and 0.1 mm (Supplementary Figure S2), which aligns with the characteristics of the previous stage (Figure 2). At a fixed size, we also observed a variation in NO2− consumption with the different polymer types of the MPs.
The decrease in NO2− concentration during this stage was due to the operation of NO2−-consuming processes including the oxidation of NO2− to NO3− through the process of NO2− oxidation and the reduction of NO2− to gaseous products N2O or dinitrogen (N2) through the process of denitrification (dissimilatory NO2− reduction) or anammox (Buchwald and Casciotti, 2013; Casciotti, 2016a, 2016b; Chen et al., 2021; Chen et al., 2022a; Chen and Chen, 2022). Although the assimilation of NO2− by phytoplankton is also a NO2−-consuming process (Buchwald and Casciotti, 2013; Casciotti, 2016a, 2016b; Chen et al., 2021; Chen et al., 2022a; Chen and Chen, 2022), it was excluded from consideration in the same way that assimilatory NO3− reduction was not considered. Thus, the difference in NO2− consumption under different polymer types and sizes of MP treatments as compared to the control may be due to the regulatory impact of these MP treatments on NO2− oxidation, dissimilatory NO2− reduction, or anammox.
Our results clearly show that NO2− production and consumption vary with MP polymer type and size, among which the impact of MP size deserves special attention (Figure 2 and Supplementary Figure S2). The variation in sediment response to different MPs may be attributed to the diverse physicochemical properties of polymers and their associated additives, and the biofilm formation on MP surfaces (Amaral-Zettler et al., 2020; Azizi et al., 2021; Oberbeckmann et al., 2015; Pinnell and Turner, 2019; Seeley et al., 2020; Su et al., 2022; Vo and Pham, 2021). These factors can differentially influence microbial activity, enzyme functions, and functional genes related to the N cycle, thereby altering biogeochemical processes within sediment ecosystems (Wang et al., 2024; Seeley et al., 2020; Shen et al., 2022; Su et al., 2022). In particular, the formation of biofilms on MPs in aquatic environments facilitates microbial adhesion and enhances local nutrient availability, leading to shifts in microbial community composition and activity, which in turn modulate N transformation processes (Wang et al., 2024; Seeley et al., 2020; Shen et al., 2022; Su et al., 2022). Moreover, the interactions between biofilms and MPs play a pivotal role in N cycling, as biofilm structures create microhabitats that influence microbial metabolic pathways and stress responses, particularly under environmental perturbations (Shen et al., 2022). These microhabitats can serve as hotspots for N transformations, creating conditions that either promote or impede key microbial processes such as nitrification and denitrification (Wang et al., 2024; Seeley et al., 2020; Shen et al., 2022; Su et al., 2022). Thus, the interplay between MP characteristics, biofilm development, and microbial dynamics is crucial in shaping N cycling within sedimentary environments.
Furthermore, the MP-size-dependent variation may be related to changes in their surface area, which influence biofilm formation and microbial colonization (Amaral-Zettler et al., 2020; Lebreton et al., 2017; Oberbeckmann et al., 2015; Su et al., 2022). Additionally, MPs significantly alter sediment properties, including porosity, pH, and oxygen availability (Cluzard et al., 2015; de Souza MaChado et al., 2019, 2019; Fang et al., 2024), thereby impacting microbial communities involved in N cycling. A notable effect of MPs in sediment ecosystems is their ability to increase porosity, which may enhance oxygen diffusion (Cluzard et al., 2015) and subsequently promote aerobic microbial processes such as nitrification. This mechanistic pathway aligns with our observations that the accumulation of NO2− exhibited a positive correlation with increasing MP size (Figure 2). However, it is important to recognize that once oxygen flux reaches a threshold sufficient to sustain nitrification, any further increase in MP size may have a diminishing impact on NO2− accumulation (Figure 2). This suggests a potential saturation effect, where oxygen availability ceases to be a limiting factor beyond a certain MP size. In our experimental conditions, the medium MP size likely provided adequate oxygen flux to support nitrification, which could explain why the increase in NO2− accumulation at the largest MP size was not significantly higher than at the medium MP size (Figure 2). This suggests that while MP size variation can initially enhance NO2− production, the incremental benefits diminish as size increases beyond a certain threshold. The identified role of MP size on NO2− production and consumption may help to explain the inconsistent findings regarding the impact of MPs on the N transformation process proposed in different studies (3). For example, the impact of PE on the N transformation process may be either positive or negative in different studies (Dai et al., 2020; Fang et al., 2024; Li et al., 2020; Seeley et al., 2020; Yin et al., 2023). Such disparate roles are also identified in our study, where PE, for instance, showed a positive or negative impact on NO2− production at varying sizes (Figure 1). Despite the varying media across studies (e.g. sediment, activated sludge, and soil; Dai et al., 2020; Fang et al., 2024; Li et al., 2020; Seeley et al., 2020; Yin et al., 2023), the size of the MPs could be responsible for the inconsistencies in these studies.
Taken together, the treatment of specific MPs regulates the strength of NO2− production and consumption. However, there is no clear information regarding which specific process in the NO2− cycling is impacted by MPs. For instance, the observed NO2− concentrations in the MP treatments were higher than that in the control, but we do not know exactly which source process was promoted by the MPs (NH3 oxidation or dissimilatory NO3− reduction). Similarly, the consumption of NO2− depends on which NO2−-consuming process is either promoted or impeded by the MPs. In light of these aspects, we offer an in-depth discussion using NO2− dual isotopes.
This study first reports the impact of MPs on the isotopic signatures of NO2− (Figure 3) and analyzes the NO2− transformation pathways accordingly (Figures 3, 4). The impact of MPs on the source of NO2− was mainly analyzed on day 18 in our experiments, as NO2− was continuously produced, although there may also have been a simultaneous partial consumption of NO2−. As for the subsequent NO2− consumption stage, the regulation of the NO2− pool by the NO2− consumption process was mainly considered, and therefore the analysis of the source process in this stage was not made here. Before an analysis of the specific source process of NO2−, one basic framework that needs to be stated is that compared with δ15NNO2, δ18ONO2 has the advantage of distinguishing signals from two potential sources (NH3 oxidation and dissimilatory NO3− reduction) of NO2−, since the δ18ONO2 values produced by these two processes are on opposite sides of the expected equilibrium value (δ18ONO2, eq, when O isotope exchange equilibrium is reached between NO2− and ambient H2O) (Buchwald and Casciotti, 2013; Casciotti, 2016a, 2016b; Chen et al., 2021; Chen et al., 2022a; Chen and Chen, 2022; Figure 4). Specifically, NO2− from dissimilatory NO3− reduction has a higher δ18ONO2 than that from NH3 oxidation, with the latter being lower than the equilibrium value (Buchwald and Casciotti, 2013; Casciotti, 2016a, 2016b; Chen et al., 2021; Chen et al., 2022a; Chen and Chen, 2022; Figure 4). Thus, although we did not measure the isotopes of NO3− and NH4+, the major source of NO2− could be inferred from the measured δ18ONO2 compared to the δ18ONO2, eq. Using the equations from Buchwald and Casciotti, 2013, along with the measured temperature in the overlying water for each treatment and the assumed δ18O value of the H2O (0‰, Buchwald and Casciotti, 2013), we then estimated the δ18ONO2, eq in our experimental system to be 13.2 ± 0.1‰. During the NO2− accumulation stage, both the MPs and the control treatments were relatively close to each other in terms of δ18ONO2 and all were slightly lower than the δ18ONO2, eq (Figure 3A and Figure 4), implying that the NO2− accumulation in all treatments was mainly contributed by NH3 oxidation (Buchwald and Casciotti, 2013; Casciotti, 2016a, 2016b; Chen et al., 2021, 2022; Chen and Chen, 2022; Figure 4).
Figure 3. The impact of MPs on nitrite isotope dynamics. (A, B) represent changes in δ15NNO2 and δ18ONO2 during the nitrite accumulation stage in the control and MP treatments, respectively, while (C, D) are changes during the nitrite consumption stage. The blue square represents the control. The grey lines in (A, C) represent the δ18ONO2, eq and the black lines are the error bars.
Figure 4. Schematic description of biogeochemical processes affecting nitrite isotopes. Possible sources of NO2− include dissimilatory NO3− reduction (grey square) and NH3 oxidation (grey diamond). The isotopic values for these two sources are not specific and are shown a range based on previous reports (41, 43, 45, 46). Possible NO2− consumption processes in our experiment include NO2− oxidation (orange dashed line), dissimilatory NO2− reduction (green dashed line), and anammox (not shown). Given that the O isotope effect for the reduction of NO2− to N2 in the anammox process is not yet known, its effect on NO2− dual isotopes is not shown in the figure. The horizontal yellow dashed line represents δ18ONO2, eq, while the vertical yellow dashed and grey lines represent abiotic equilibrium processes and source mixing processes (41-46). The circle and triangle colored in red represent the CON and the black lines are error bars.
Thus, based on isotopic evidence, the higher or lower accumulation of NO2− in the MP treatment compared to the control may indicate that the polymer type and size of the MPs either promoted or impeded NH3 oxidation, a specific NO2− source process. For instance, in the case of PE-0.02 mm, the accumulated NO2− was lower than that of the control (Figure 1C). Furthermore, given that its δ18ONO2 was lower than the equilibrium value (Figures 3A, 4), this may imply that this treatment may have impeded NH3 oxidation, leading to a lower accumulated concentration of NO2−. However, the assessment regarding this impediment impact should be viewed with caution. This is because, although we focus on the role of NO2− source processes in regulating the NO2− pool at this stage, NO2− consumption processes may also be operating simultaneously. These consumption processes may alter δ18ONO2, which, in turn, may affect the assessment of the impact of MPs on NO2− production. Therefore, in conjunction with the analysis of δ15NNO2, we provide a comprehensive analysis of this aspect below.
The fate of NO2− determines whether N is lost or retained in the environment, influencing greenhouse gas N2O production and the environmental N budget. However, the impact of MP pollution on NO2− consumption pathways remains unclear (Fang et al., 2024; Green et al., 2016; Hope et al., 2020; Seeley et al., 2020). To investigate this, we used NO2− dual isotope data (Figures 3A, B), similar to analyzing the effect of MPs on NO2− source processes. Before proceeding, we must also clarify the effects of NO2− oxidation, denitrification (dissimilatory NO2− reduction), and anammox on NO2− dual isotopes to better assess the specific processes impacted by MPs. NO2− oxidation, either driven by NO2−-oxidizing bacteria (Buchwald and Casciotti, 2010; Casciotti, 2009) or anammox bacteria (Brunner et al., 2013; Kobayashi et al., 2019), is expected to decrease both δ15NNO2 (Figure 3C) and δ18ONO2 (Figure 3D) (Brunner et al., 2013; Buchwald and Casciotti, 2010; Casciotti, 2009; Kobayashi et al., 2019). Denitrification is discussed here as dissimilatory NO2− reduction, since dissimilatory NO3− reduction, although also part of the denitrification, is a process that acts as the source of NO2−. Dissimilatory NO2− reduction will result in an increase in δ18ONO2 (Figure 3C) and δ15NNO2 (Figure 3D) (Martin and Casciotti, 2016). Similarly, the operation of anammox will also result in an increase in δ15NNO2 during NO2− reduction to N2 (Brunner et al., 2013; Kobayashi et al., 2019), but its effect on δ18ONO2 remains unclear (Casciotti, 2016b; Kobayashi et al., 2019).
In light of the effects of these consumption processes on NO2− concentration and isotopes, we herein first reevaluate the reliability of the above discussion of the impacts of MPs on NO2− production, focusing on treatments with lower NO2− accumulation compared to the control. Some treatments, such as PA-0.02 mm, PE-0.02 mm, and PE-0.1 mm, had lower NO2− concentrations compared to the control (Figure 1C), implying that NO2− production may have been impeded. However, the relationship between NO2− accumulation and δ15NNO2 revealed a deviation in the PA-0.02 mm and PE-0.02 mm treatments (Figure 5), indicating enhanced NO2− oxidation, which can simultaneously decrease both NO2− concentration and δ15NNO2 (Brunner et al., 2013; Buchwald and Casciotti, 2010; Casciotti, 2009; Kobayashi et al., 2019). A similar effect was observed in the PE-0.1 mm treatment (Supplementary Figure S3). However, as the MP size increased, the impact of NO2− oxidation on the NO2− pool became limited (Supplementary Figure S4), likely due to the maximal NO2−yield (Figure 2), making the NO2− oxidation insufficient to significantly reduce NO2−accumulation. Consequently, the lower NO2− concentrations in small-sized MP treatments may not be solely due to reduced NO2− production, but rather the promotion of NO2−oxidation.
Figure 5. Relationship between nitrite concentration and δ15NNO2 during the nitrite accumulation stage. The various colored circles represent the MPs of different polymer types with the smallest size, while the black lines represent error bars.
It is important to note that stronger NO2− oxidation in these treatments may confound δ18O -based evaluations of NO2− source processes, as this process would lower δ18ONO2 (Brunner et al., 2013; Buchwald and Casciotti, 2010; Casciotti, 2009; Kobayashi et al., 2019), potentially masking the contribution of dissimilatory NO3− reduction to NO2− production (Figure 4). But here, we focus mainly on whether the lower NO2− concentrations in these MP treatments relative to the control were due to source processes being impeded, regardless of the specific source process. Obviously, the above analysis is a good example to show the ability of natural abundance stable isotopes in addressing the impact of MPs on the N cycle.
Furthermore, during the NO2− consumption stage, the δ15NNO2 was higher in the MP and the control treatments than in the previous stage (Figure 3D). This shows that the NO2− in some MP treatments, including the control, was consumed primarily through dissimilatory NO2− reduction or anammox (Figure 4). However, some MP treatments showed outliers in δ15NNO2 relative to the control (Figure 3D), meaning that there may have been variations in the pathway or strength of NO2− consumption. The presence of these outliers further supports size as an important factor in considering the impact of MPs on the N cycle. By analyzing these outliers, we can capture valuable information about the impact of MPs on the biogeochemical cycling of NO2−. Accordingly, we performed a discussion of the relationship between NO2− concentration (residual), degree of NO2− consumption, δ15NNO2 of residual NO2−, and δ15NNO2 variation in the MPs and control treatments during the NO2− consumption stage (Figure 6, Supplementary Figures S5-S7). Among these, the degree of NO2− consumption and δ15NNO2 variation represent the quantity of NO2− consumed and the impact of the NO2−-consuming process on δ15NNO2 over the period of 18–25 days, respectively.
Figure 6. Relationship between the δ15NNO2 and degree of NO2− consumption. Differences in NO2− concentrations on day 18 and day 25 in the experiment were used to create this figure. The numbers 1, 2, and 3 in the figure represent the three pathways of nitrite consumption, respectively. The different numerical labels on the arrows indicate the impact of MPs on NO2− fate and the black lines are error bars. The triangles, circles, and diamonds represent the smallest, medium and largest sizes of MPs, respectively.
The first case is enhanced NO2− oxidation (arrow 1 in Figure 6). The δ15NNO2 decreased with increasing NO2− consumption in the PP treatment of different sizes (Figure 6, Supplementary Figures S5-S7). In particular, for the PP treatment at 1 mm, the NO2− consumption was higher than that of the control, yet δ15NNO2 was lower (Figure 6). The low δ15NNO2 signal during NO2− consumption could either be due to a low δ15NNO2 during the previous NO2− accumulation stage compared to the control or a smaller increase in δ15NNO2 during NO2− consumption. The first possibility can be excluded, as δ15NNO2 values were consistent across treatments and the control during NO2− accumulation (Figure 3B). Therefore, the second possibility must be considered. As shown by the relationship between NO2− consumption and δ15NNO2variation, the δ15NNO2 increase in the PP treatment was smaller than in the control as NO2− consumption increased (Supplementary Figures S5-S7). This suggests a contribution of dissimilatory NO2− reduction or anammox (Brunner et al., 2013; Martin and Casciotti, 2016; Kobayashi et al., 2019), but the limited increase implies these processes were weaker than in the control. If these processes were weaker, NO2− consumption would be lower, which contradicts the higher NO2− consumption in the PP treatments (Figure 6, Supplementary Figures S5-S7). Thus, another process must be consuming NO2− and limiting δ15NNO2 increase. We propose that NO2− oxidation played a more significant role in NO2− consumption in the PP-0.1 mm and PP-1 mm treatments (Figure 6, Supplementary Figure S6, and Supplementary Figure S7), as it can both consume NO2−and reduce δ15NNO2 (Figure 4, Brunner et al., 2013; Buchwald and Casciotti, 2010; Casciotti, 2009; Kobayashi et al., 2019). This suggests that in environments polluted by these MPs, NO2− may be retained as bioavailable N, potentially reducing N2O greenhouse gas production.
The second scenario is enhanced dissimilatory NO2− reduction or anammox (arrow 2 in Figure 6). The higher δ15NNO2 values and NO2− consumption in some MP treatments, especially PA-1mm, imply a stronger operation of dissimilatory NO2− reduction or anammox (Figure 4, Brunner et al., 2013; Martin and Casciotti, 2016; Kobayashi et al., 2019). This is further supported by the observation that in the PA-1mm treatment, the increase in δ15NNO2 during NO2− consumption was greater than in the control (Supplementary Figure S6 and Supplementary Figure S7). Unlike the previous scenario, this indicates that more NO2− is being lost from the environment as gas (N2O or N2) in this scenario, suggesting that the production of the greenhouse gas N2O may be enhanced.
The final scenario may involve an enhanced dissimilatory NO3− reduction and dissimilatory NO2− reduction (or anammox) (arrow 3 in Figure 6). This scenario is distinct because, although NO2− consumption was limited, there was an increase in δ15NNO2 compared to the control (PE-0.02 mm, PE-0.1 mm, PA-0.02 mm, Figure 6, Supplementary Figure S5, and Supplementary Figure S6). The increase in δ15NNO2 suggests that dissimilatory NO2− reduction or anammox are the primary processes responsible for NO2− consumption (Figure 4; Brunner et al., 2013; Martin and Casciotti, 2016; Kobayashi et al., 2019). However, since NO2− consumption was not significant, we propose that dissimilatory NO3− reduction, the first step of denitrification, may also be regulating the NO2− pool. This could offset NO2− consumption, and the δ18ONO2 analysis supports this hypothesis. The δ18ONO2 values of these MP treatments were all higher than the control and equilibrium values (Figure 3C), indicating a contribution from dissimilatory NO3− reduction (Figure 4). Consequently, the imbalance between the source and consumption of NO2− in this scenario likely led to insufficient NO2− consumption coupled with increased δ15NNO2. When considered in terms of NO2− consumption pathways, this also reveals a promotion of dissimilatory NO2− reduction or anammox in these MP treatments compared to the control. Without the isotope data, such low consumption of NO2− in these MP treatments might have been considered to be an inhibition of the dissimilatory NO2− reduction or anammox by these MPs relative to the control. Thus, this is once again a prime example of the value of natural abundance stable isotopes in identifying the real impact of MPs on the N cycle.
Overall, the isotopic evidence clearly indicates that the consumption pathways of NO2− vary depending on the polymer type and size of the MPs, which plays a crucial role in determining whether the production of the greenhouse gas N2O in the environment increases or decreases. This suggest that MPs may contribute to local variations in greenhouse gas emissions, potentially altering greenhouse gas inventories in affected regions (Li et al., 2022; Ren et al., 2020; Su et al., 2022; Wang et al., 2024). These findings underscore the importance of considering MPs as an emerging environmental factor in greenhouse gas management. Future studies should aim to explore the long-term effects of MPs on N transformations and their cumulative impact under various environmental stressors, thereby improving the accuracy of greenhouse gas emission inventories and informing more effective mitigation strategies.
Conclusively, our study presents the first picture of the impact of MPs with distinct polymer types and sizes on specific N cycle transformation processes based on the natural abundance of N and O isotope ratios of NO2−. This offers a new perspective for a more comprehensive and accurate evaluation of the impact of sediment exposure to MP pollution on the biogeochemical cycling of NO2− in aquatic ecosystems, which has profound implications for reconciling the balance of the environmental N budget and assessing greenhouse gas production in the context of increasing MP pollution. It is worth noting that while our study aims to provide a new paradigm for the study of the impact of MPs on the N cycle, the reasons behind the unique regulation of the N cycle by MPs with distinct polymer types and sizes remain to be further addressed.
The original contributions presented in the study are included in the article/Supplementary Material. Further inquiries can be directed to the corresponding author.
YC: Conceptualization, Data curation, Formal analysis, Funding acquisition, Investigation, Methodology, Writing – original draft, Writing – review & editing. XZ: Data curation, Formal analysis, Investigation, Methodology, Writing – original draft. YY: Investigation, Methodology, Validation, Writing – original draft. MC: Data curation, Formal analysis, Resources, Writing – review & editing.
The author(s) declare financial support was received for the research, authorship, and/or publication of this article. This work was supported by National Natural Science Foundation of China (42306055), Natural Science Foundation of Jiangsu Province (BK20230695), and Open-end Funds of Jiangsu Key Laboratory of Marine Biotechnology, Jiangsu Ocean University (HS2022001).
We thank Minfang Zheng and Mengya Chen for their help with the isotopic analysis. We also thank Xueting Chen for her assistance in sample processing.
The authors declare that the research was conducted in the absence of any commercial or financial relationships that could be construed as a potential conflict of interest.
The author(s) declare that no Generative AI was used in the creation of this manuscript.
All claims expressed in this article are solely those of the authors and do not necessarily represent those of their affiliated organizations, or those of the publisher, the editors and the reviewers. Any product that may be evaluated in this article, or claim that may be made by its manufacturer, is not guaranteed or endorsed by the publisher.
The Supplementary Material for this article can be found online at: https://www.frontiersin.org/articles/10.3389/fmars.2025.1518631/full#supplementary-material
Amaral-Zettler L. A., Zettler E. R., Mincer T. J. (2020). Ecology of the plastisphere. Nat. Rev. Microbiol. 18, 139–151. doi: 10.1038/s41579-019-0308-0
Azizi S. M. M., Hai F. I., Lu W., Al-Mamun A., Dhar B. R. (2021). A review of mechanisms underlying the impacts of (nano) MPs on anaerobicdigestion. Bioresource Technol. 329, 124894. doi: 10.1016/j.biortech.2021.124894
Baysal A., Saygin H., Ustabasi G. S. (2020). MPs occurrences in sediments collected from marmara sea-istanbul, Turkey. Bull. Environ. Contam. Toxicol. 105, 522–529. doi: 10.1007/s00128-020-02993-9
Brunner B., Contrerasa S., Lehmann M. F., Matantseva O., Rollog M., Kalvelage T., et al. (2013). Nitrogen isotope effects induced by anammox bacteria. Proc. Natl. Acad. Sci. U.S.A. 110, 18994–18999. doi: 10.1073/pnas.1310488110
Buchwald C., Casciotti K. L. (2010). Oxygen isotopic fractionation and exchange during bacterial nitrite oxidation. Limnol. Oceanogr. 55, 1064–1074. doi: 10.4319/lo.2010.55.3.1064
Buchwald C., Casciotti K. L. (2013). Isotopic ratios of nitrite as tracers of the sources and age of oceanic nitrite. Nat. Geosci. 6, 308–313. doi: 10.1038/ngeo1745
Casciotti K. L. (2009). Inverse kinetic isotope fractionation during bacterial nitrite oxidation. Geochim. Cosmochim. Acta 73, 2061–2076. doi: 10.1016/j.gca.2008.12.022
Casciotti K. L. (2016a). Nitrogen and oxygen isotopic studies of the marine nitrogen cycle. Annu. Rev. Mar. Sci. 8, 379–407. doi: 10.1146/annurev-marine-010213-135052
Casciotti K. L. (2016b). Nitrite isotopes as tracers of marine N cycle processes. Philos. Trans. R. Soc A 374, 20150295. doi: 10.1098/rsta.2015.0295
Casciotti K. L., Böhlke J. K., McIlvin M. R., Mroczkowski S. J., Hannon J. E. (2007). Oxygen isotopes in nitrite: analysis, calibration, and equilibration. Anal. Chem. 79, 2427–2436. doi: 10.1021/ac061598h
Chen Y. J., Bardhan P., Zhao X. F., Zheng M. F., Qiu Y. S., Chen M. (2021). Nitrite cycle indicated by dual isotopes in the northern South China Sea. J. Geophys. Res. Biogeosciences 126, e2020JG006129. doi: 10.1029/2020JG006129
Chen Y. J., Chen M. (2022). Nitrite cycling in warming Arctic and Subarctic waters. Geophys. Res. Lett. 49, e2021GL096947. doi: 10.1029/2021GL096947
Chen Y. J., Chen M., Chen J. X., Fan L. F., Zheng M. F., Qiu Y. S. (2022a). Dual isotopes of nitrite in the Amundsen Sea in summer. Sci. Total Environ. 843, 157055. doi: 10.1016/j.scitotenv.2022.157055
Chen C., Pan J., Xiao S., Wang J., Gong X., Yin G., et al. (2022b). Microplastics alter nitrous oxide production and pathways through affecting microbiome in estuarine sediments. Water Res. 221, 118733. doi: 10.1016/j.watres.2022.118733
Cluzard M., Kazmiruk T. N., Kazmiruk V. D., Bendell L. I. (2015). Intertidal concentrations of MPs and their influence on ammonium cycling as related to the shellfish industry. Arch. Environ. Contam. Toxicol. 69, 310–319. doi: 10.1007/s00244-015-0156-5
Dai H. H., Gao J., Wang Z., Zhao Y., Zhang D. (2020). Behavior of nitrogen, phosphorus and antibiotic resistance genes under polyvinyl chloride MPs pressures in an aerobic granular sludge system. J. Clean. Prod. 256, 120402. doi: 10.1016/j.jclepro.2020.120402
de Souza MaChado A. A., Lau C. W., Kloas W., Bergmann J., Bachelier J. B., Faltin E., et al. (2019). MPs can change soil properties and affect plant performance. Environ. Sci. Technol. 53, 6044–6052. doi: 10.1021/acs.est.9b01339
Fang C., Yang Y., Zhang S., He Y., Pan S., Zhou L., et al. (2024). Unveiling the impact of MPs with distinct polymer types and concentrations on tidal sediment microbiome and nitrogen cycling. J. Hazard. Mater. 472, 134387. doi: 10.1016/j.jhazmat.2024.134387
Granger J., Boshers D. S., Böhlke J. K., Yu D., Chen N., Tobias C. R. (2020). The influence of sample matrix on the accuracy of nitrite N and O isotope ratio analyses with the azide method. Rapid Commun. Mass Spectrom. 34, e8569. doi: 10.1002/rcm.v34.1
Green D. S., Boots B., Sigwart J., Jiang S., Rocha C. (2016). Effects of conventional and biodegradable MPs on a marine ecosystem engineer (Arenicola marina) and sediment nutrient cycling. Environ. pollut. 208, 426–434. doi: 10.1016/j.envpol.2015.10.010
Greenfield L. M., Graf M., Rengaraj S., Bargiela R., Williams G., Golyshin P. N. (2022). Field response of N2O emissions, microbial communities, soil biochemical processes and winter barley growth to the addition of conventional and biodegradable MPs. Agric. Ecosyst. Environ. 336, 108023. doi: 10.1016/j.agee.2022.108023
Hartmann N. B. (2019). Are we speaking the same language? Recommendations for a definition and categorization framework for plastic debris. Environ. Sci. Technol. 53, 1039–1047. doi: 10.1021/acs.est.8b05297
Hope J. A., Coco G., Thrush S. F. (2020). Effects of polyester microfibers on microphytobenthos and sediment-dwelling infauna. Environ. Sci. Technol. 54, 7970–7982. doi: 10.1021/acs.est.0c00514
Hu H., Bourbonnais A., Larkum J., Bange H. W., Altabet M. A. (2016). Nitrogen cycling in shallow low-oxygen coastal waters off Peru from nitrite and nitrate nitrogen and oxygen isotopes. Biogeosciences 13, 1453–1468. doi: 10.5194/bg-13-1453-2016
Hu X. J., Gu H. D., Sun X. X., Wang Y. B., Liu J. J., Yu Z. H., et al. (2023). Distinct influence of conventional and biodegradable MPs on microbe-driving nitrogen cycling processes in soils and plastispheres as evaluated by metagenomic analysis. J. Hazard. Mater. 451, 131097. doi: 10.1016/j.jhazmat.2023.131097
Ikenoue T., Nakajima R., Osafune S., Siswanto E., Honda M. C. (2024). Vertical flux of microplastics in the deep subtropical Pacific Ocean: moored sediment-trap observations within the Kuroshio Extension recirculation gyre. Environ. Sci. Technol. 58, 16121–16130. doi: 10.1021/acs.est.4c02212
Ikenoue T., Yamada M., Ishii N., Kudo N., Shirotani Y., Ishida Y., et al. (2022). Cesium-137 and 137Cs/133Cs atom ratios in marine zooplankton off the east coast of Japan during 2012–2020 following the Fukushima Dai-ichi nuclear power plant accident. Environ. pollut. 311, 119962. doi: 10.1016/j.envpol.2022.119962
Kobayashi K., Fukushima K., Onishi Y., Nishina K., Makabe A., Yano M. (2021). Influence of δ18O of water on measurements of δ18O of nitrite and nitrate. Rapid Commun. Mass Spectrom. 35, e89799. doi: 10.1002/rcm.v35.2
Kobayashi K., Makabe A., Yano M., Oshiki M., Kindaichi T., Casciotti K. L., et al. (2019). Dual nitrogen and oxygen isotope fractionation during anaerobic ammonium oxidation by anammox bacteria. ISME J. 13, 2426–2436. doi: 10.1038/s41396-019-0440-x
Lebreton L. C. M., van der Zwet J., Damsteeg J.-W., Slat B., Andrady A., Reisser J. (2017). River plastic emissions to the world’s oceans. Nat. Commun. 8, 1–10. doi: 10.1038/ncomms15611
Lee J., Jeong S., Long C., Chandran K. (2022). Size dependent impacts of a model microplastic on nitrification induced by interaction with nitrifying bacteria. J. Hazard Mater. 424, 127363. doi: 10.1016/j.jhazmat.2021.127363
Li H., Liu L. (2022). Short-term effects of polyethene and polypropylene MPs on soil phosphorus and nitrogen availability. Chemosphere 291, 132984. doi: 10.1016/j.chemosphere.2021.132984
Li L., Song K., Yeerken S., Geng S. X., Liu D., Dai Z. L. (2020). Effect evaluation of MPs on activated sludge nitrification and denitrification. Sci. Total Environ. 707, 135953. doi: 10.1016/j.scitotenv.2019.135953
Li X., Yao S., Wang Z., Jiang X., Song Y., Chang S. X. (2022). Polyethylene MPs and biochar interactively affect the global warming potential of soil greenhouse gas emissions. Environ. pollut. 315, 120433. doi: 10.1016/j.envpol.2022.120433
Lyu L., Wu Y., Chen Y. J., Li J., Chen Y., Wang L. (2024). Synergetic effects of chlorinated paraffins and microplastics on microbial communities and nitrogen cycling in deep-sea cold seep sediments. J. Hazard. Mater. 480, 135760. doi: 10.1016/j.jhazmat.2024.135760
Martin T. S., Casciotti K. L. (2016). Nitrogen and oxygen isotopic fractionation during microbial nitrite reduction. Limnol. Oceanogr. 61, 1134–1143. doi: 10.1002/lno.10278
Mato Y., Isobe T., Takada H., Kanehiro H., Ohtake C., Kaminuma T. (2001). Plastic resin pellets as a transport medium for toxic chemicals in the marine environment. Environ. Sci. Technol. 35, 318–324. doi: 10.1021/es0010498
McIlvin M. R., Altabet M. A. (2005). Chemical conversion of nitrate and nitrite to nitrous oxide for nitrogen and oxygen isotopic analysis in freshwater and seawater. Anal. Chem. 77, 5589–5595. doi: 10.1021/ac050528s
Oberbeckmann S., Löder M. G. J., Labrenz M. (2015). Marine MPs associated biofilms-a review. Environ. Chem. 12, 551–562. doi: 10.1071/EN15069
Pinnell L. J., Turner J. W. (2019). Shotgun metagenomics reveals the benthic microbial community response to plastic and bioplastic in a coastal marine environment. Front. Microbiol. 10, 1–13. doi: 10.3389/fmicb.2019.01252
Ren X., Tang J., Liu X., Liu Q. (2020). Effects of MPs on greenhouse gas emissions and the microbial community in fertilized soil. Environ. pollut. 256, 113347. doi: 10.1016/j.envpol.2019.113347
Rillig M. C., Hoffmann M., Lehmann A., Liang Y., Lück M., Augustin J. (2021). MPs fibers affect dynamics and intensity of CO2 and N2O fluxes from soil differently. Microplast. Nanoplast. 1, 3. doi: 10.1186/s43591-021-00004-0
Riveros G., Urrutia H., Araya J., Zagal E., Schoebitz M. (2022). MPs pollution on the soil and its consequences on the nitrogen cycle: a review. Environ. Sci. pollut. Res. 29, 7997–8011. doi: 10.1007/s11356-021-17681-2
Seeley M. E., Song B., Passie R., Hale R. C. (2020). MPs affect sedimentary microbial communities and nitrogen cycling. Nat. Commun. 11, 2372. doi: 10.1038/s41467-020-16235-3
Shen M., Song B., Zhou C., Almatrafi E., Hu T., Zeng G., et al. (2022). Recent advances in impacts of microplastics on nitrogen cycling in the environment: A review. Sci. Total Environ. 815, 152740. doi: 10.1016/j.scitotenv.2021.152740
Song K., Wang R., Yang G., Xie S., Chen Y., Yang F. (2023). Pollution concerns in mariculture water and cultured economical bivalves: Occurrence of MPs under different aquaculture modes. J. Clean. Prod. 406, 136913. doi: 10.1016/j.jclepro.2023.136913
Strickland J. D. H., Parsons T. R. (1972). A practical handbook of seawater analysis. Bull. Fish. Res. Board Canada 167, 1–310. doi: 10.1002/iroh.19700550118
Su X., Yang L., Yang K., Tang Y., Wen T., Wang Y. (2022). Estuarine plastisphere as an overlooked source of N2O production. Nat. Commun. 13, 3884. doi: 10.1038/s41467-022-31584-x
Sun X., Tao R., Xu D., Qu M., Zheng M., Zhang M., et al. (2023). Role of polyamide microplastic in altering microbial consortium and carbon and nitrogen cycles in a simulated agricultural soil microcosm. Chemosphere 312, 137155. doi: 10.1016/j.chemosphere.2022.137155
Vo H. C., Pham M. H. (2021). Ecotoxicological effects of MPs onaquatic organisms: a review. Environ. Sci. pollut. Res. 28, 44716–44725. doi: 10.1007/s11356-021-14982-4
Wan Y., Wu C., Xue Q., Hui X. (2018). Effects of plastic contamination on water evaporation and desiccation cracking in soil. Sci. Total Environ. 654, 576–582. doi: 10.1016/j.scitotenv.2018.11.123
Wang W., Ge J., Yu X. (2020). Bioavailability and toxicity of MPs to fish species: a review. Ecotoxicol. Environ. Saf. 189, 109913. doi: 10.1016/j.ecoenv.2019.109913
Wang W. F., Zhang Z. Y., Gao J., Wu H. T. (2024). The impacts of MPs on the cycling of carbon and nitrogen in terrestrial soil ecosystems: Progress and prospects. Sci. Total Environ. 915, 169977. doi: 10.1016/j.scitotenv.2024.169977
Wang P. Y., Zhao Z.-Y., Xiong X.-B., Wang N., Zhou R., Zhang Z.-M. (2023). MPs affect soil bacterial community assembly more by their shapes rather than the concentrations. Water Res. 24, 120581. doi: 10.1016/j.watres.2023.120581
Yin M. Y., Wang H., Wu Y., Wang X., Wang J. (2023). Effects of MPs on nitrogen and phosphorus cycles and microbial communities in sediments. Environ. pollut. 318, 120852. doi: 10.1016/j.envpol.2022.120852
Yu Y., Li X., Feng Z., Xiao M., Ge T., Li Y. (2022). Polyethylene MPs alter the microbial functional gene abundances and increase nitrous oxide emissions from paddy soils. J. Hazard. Mater. 432, 128721. doi: 10.1016/j.jhazmat.2022.128721
Zhang X., Ward B. B., Sigman D. M. (2020). Global nitrogen cycle: critical enzymes, organisms, and processes for nitrogen budgets and dynamics. Chem. Rev. 120, 5308–5351. doi: 10.1021/acs.chemrev.9b00613
Zhang G. S., Zhang F. X., Li X. T. (2019). Effects of polyester microfibers on soil physical properties: perception from a field and a pot experiment. Sci. Total Environ. 670, 1–7. doi: 10.1016/j.scitotenv.2019.03.149
Keywords: microplastics pollution, nitrite cycle, nitrous oxide, isotopic dynamics, climate change
Citation: Chen Y, Zhang X, Yang Y and Chen M (2025) Natural abundance isotope techniques offer a key to better deciphering the impact of microplastics on the nitrogen cycle. Front. Mar. Sci. 12:1518631. doi: 10.3389/fmars.2025.1518631
Received: 28 October 2024; Accepted: 24 February 2025;
Published: 11 March 2025.
Edited by:
Martin F. Soto-Jimenez, National Autonomous University of Mexico, MexicoReviewed by:
Peng Zhang, Guangdong Ocean University, ChinaCopyright © 2025 Chen, Zhang, Yang and Chen. This is an open-access article distributed under the terms of the Creative Commons Attribution License (CC BY). The use, distribution or reproduction in other forums is permitted, provided the original author(s) and the copyright owner(s) are credited and that the original publication in this journal is cited, in accordance with accepted academic practice. No use, distribution or reproduction is permitted which does not comply with these terms.
*Correspondence: Yangjun Chen, eWotY2hlbkBqb3UuZWR1LmNu
†ORCID: Yangjun Chen, orcid.org/0000-0002-7488-9338
Disclaimer: All claims expressed in this article are solely those of the authors and do not necessarily represent those of their affiliated organizations, or those of the publisher, the editors and the reviewers. Any product that may be evaluated in this article or claim that may be made by its manufacturer is not guaranteed or endorsed by the publisher.
Research integrity at Frontiers
Learn more about the work of our research integrity team to safeguard the quality of each article we publish.