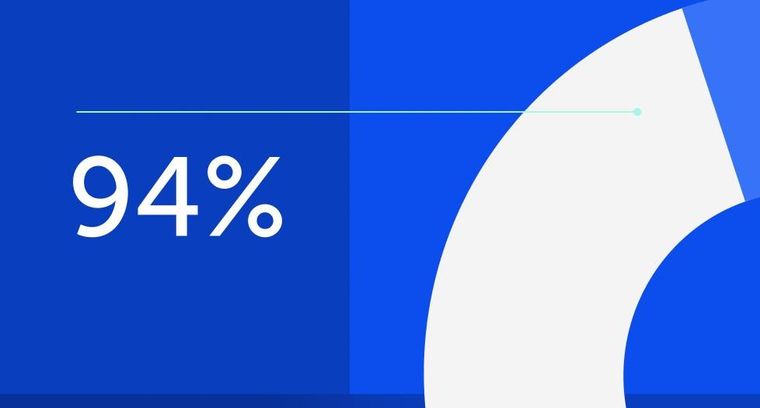
94% of researchers rate our articles as excellent or good
Learn more about the work of our research integrity team to safeguard the quality of each article we publish.
Find out more
ORIGINAL RESEARCH article
Front. Mar. Sci., 01 April 2025
Sec. Aquatic Microbiology
Volume 12 - 2025 | https://doi.org/10.3389/fmars.2025.1516784
This article is part of the Research TopicOccurrence of Harmful Algal Blooms and Marine BiotoxinsView all 5 articles
Introduction: Coastal regions around the world are influenced by numerous dynamical processes that supply nutrients for primary producers and trigger the food web. Rivers, submarine groundwater discharges (SGD), sediment suspension events, and upwelling, are amongst the more important. However, it is not just the concentration, but also the composition of nutrient supply that determines the type of phytoplankton community that develops, subsequently influencing the entire food web. It is therefore necessary to better understand the link between physical processes, nutrient composition and phytoplankton response in coastal oceans. This study investigates the effects of upwelling and submarine groundwater discharges on the phytoplankton community of a wide and shallow continental shelf.
Methods: Results are achieved by using numerical modeling of dispersion events, as well as field data obtained from three oceanographic cruises, each representing a different hydrographic scenario (“No upwelling”, “Minor upwelling and SGD” and “Major upwelling and minor SGD”).
Results: The upwelled water mass (SUW – Subtropical Underwater) was primarily found at the eastern end of the shelf (Cabo Catoche), where it rises and is transported westward by advection. The oceanographic stations influenced by the upwelled water showed an increase in diatom species population, known to thrive in environments with abundant inorganic nutrients. In contrast, submarine groundwater discharges were recorded nearshore on the western half of the shelf, mainly related to karst features of hydrogeological importance (a sinkhole ring associated with the Chicxulub crater). The stations with SGD influence had higher presence of nutrients such as NH4, suggesting recycling processes. This can modify either the phytoplankton community advected from Cabo Catoche, or promote local growth, leading to a dominance of dinoflagellates and unknown flagellates.
Discussion: This result implies a food web, similar to that of the mixoplankton-dominated microbial loop, which would be fed by organic matter of continental origin.
Various oceanographic processes occur on continental shelves which affect phytoplankton community assemblages. Among these, the most important is usually associated with upwelling: the vertical movement of deeper water masses (usually cold and nutrient-rich), which can occur as a result of wind stress (surface Ekman transport), current friction with the seabed (bottom Ekman layer) (Simpson and Sharples, 2012), interaction of shelf slope with mesoscale structures (eddies, meanders, internal waves) (Wolanski and Delesalle, 1995) and island effects (De Falco et al., 2022).
Another process that increases the nutrient content of the waters on continental shelves is the input from continental sources (Chen et al., 2003). Although rivers and lagoons are the most obvious source of these enrichment, fresh submarine groundwater discharges have been increasingly recognized as a very important source of nutrients in the coastal zone, and recent estimates show that nutrient fluxes from Submarine Groundwater Discharges (SGDs) can consistently exceed river inputs (Taniguchi et al., 2019; Santos et al., 2021). The effects of such discharges have been recorded several kilometers offshore on continental shelves around the world (Moore, 2010) and the type and quantity of nitrogen compounds reaching the coastal zone due to SGDs depends partly on the origin of the continental water. Groundwater discharges associated with conserved areas, or ecological reserves, tend to be less polluted than those associated with areas influenced by anthropic activities (Álvarez-Góngora et al., 2012), such as those destined to urban, industrial, livestock and agricultural uses. Nevertheless, it has been found that nitrogen input from SGDs is mostly in the form of ammonium and dissolved organic nitrogen (measured within the underground system), which often mitigates nitrogen limitation in coastal waters (Santos et al., 2021). Nutrients in SGDs are often recorded in a wide range of ratios (N:Si:P), most of the time these ratios exceed the Redfield Ratio (16:16:1) because Phosphorus can be absorbed in mineral surfaces (with Fe or Mn), or can be precipitated with calcium carbonate (Santos et al., 2021). In SGDs of China, N:P ratios can vary from 0.4 to 44,475, and in the case of Si:P from 3.65 to 14,300, showing a clear P limitation (Zhang et al., 2022).
The input of nutrients to continental shelves from any source is also modified by the inherent advection, diffusion and mixing produced by oceanographic conditions which modify water masses, transforming their properties (i.e. salinity, temperature, transparency and supply of nutrients) and inducing favorable or unfavorable conditions for the development of primary producers.
In general, increased nutrient availability produces a rise in primary producers, such as phytoplankton, but the composition of a phytoplankton community is determined by the nutrient types (its chemical composition) and the ratio between the different nutrients. For example, the dominance of diatom groups has been associated with typical Redfield ratios of 16:1 N:P, whereas the dominance of cyanobacteria occurs with ratios of 25:1 NP (Hillebrand et al., 2013). The presence of urea in the nutrient sources brings about a rise in dinoflagellates and other organisms that produce harmful algal blooms, just as ratios of DOC>>DON facilitates the dominance of mixotrophs (Glibert and Legrand, 2006; Glibert et al., 2006). In principle it is possible to determine the composition of a phytoplankton community using different factors and conditions, such as temperature, flow conditions, nutrient type and ecological preferences, as summarized in previous works in diagrammatic form, such as Ramon Margalef mandala and similar updated efforts (Glibert, 2016). In this way, the records of species observed in upwellings, red tides, estuaries, coastal zones, oligotrophic seas, deep zones, and so on can be used to identify phytoplankton communities typical of certain processes.
This paper examines the relative importance of upwelling vs continental input (in the form of diluted SGD) on nutrient concentrations and community composition of phytoplankton on a wide and shallow continental shelf, where oceanographic processes play important roles on the advection and mixing of water masses. The article is organized as follows: The study site section explains the physiographic, oceanographic and ecological characteristics of the location where this study focuses, and how these characteristics make this study important. The methodology section explains how information was obtained from the oceanographic cruises, how the dispersion model (Ichthyop) was implemented, and details the statistical methodologies. These include the definition of appropriate indexes for the identification of SGD or upwelling, and the establishment of statistical associations between phytoplankton, nutrients and physical processes. The section on physical processes details the upwelling, SGD, and Lagrangian drifter conditions of each cruise. The section of phytoplankton communities provides details of phytoplankton assemblages and how they are linked to the different processes that affect nutrient input. Finally, conclusions and a graphical summary are drawn in the last section.
In the shallow regions of the Yucatan Shelf, currents consistently flow westwards. Despite this apparent simplicity in the circulation patterns, thermohaline dynamics are highly influenced by several complex processes (Enriquez et al., 2013) that nourish primary productivity on the shallow Yucatan shelf (Herrera-Silveira and Morales-Ojeda, 2009). For example, on the eastern boundary (Holbox and Cabo Catoche), upwelling has long been recognized as the main process that governs primary productivity and triggers the development of a dynamic food chain (Cochrane, 1966; Ruiz Renteria, 1979; Belousov et al., 1966; Bulanienkov and Garcia, 1973; Merino, 1997; Morales-Ojeda et al., 2010; Reyes-Mendoza et al., 2019). Cabo Catoche on the eastern tip of the peninsula and a region called “the Notch” (see Figure 1) on the eastern continental slope, are identified as the main gateways of cool, nutrient rich water that enters onto the shelf. The most likely explanation of the drivers of this upwelling include bottom Ekman transport, linked to the friction exerted by the intense Yucatan current on the continental slope (Cochrane, 1966) and the associated veering upslope of the bottom boundary layer. Given its topographic nature, the intensity of the upwelling has been clearly linked to the variability in the position of the Yucatan current relative to the bottom slope (Enriquez and Marino-Tapia, 2014; Jouanno et al., 2018; Reyes-Jimenez et al., 2023). Furthermore, when the Yucatan current moves offshore (towards Cuba), it favors changes in sea level on the shelf and the development of cyclonic vorticity whose divergence helps maintain the upwelling for prolonged periods of time (Reyes-Jimenez et al., 2023). During autumn-winter this behavior is disrupted by the occurrence of northerly storms (cold fronts) which have been observed to inhibit upwelling (Reyes-Mendoza et al., 2015). The persistent occurrence of coastal trapped waves during such seasons might be involved in the explanation (Reyes-Jiménez et al., 2023), although this topic deserves further investigation.
Figure 1. Yucatan Shelf map including sampling stations (yellow dots), and location of sinkholes inland (blue dots). The sinkhole ring is noticeable on the western region of the peninsula. The Notch, a bathymetric feature, is indicated in a red ellipse (Jouanno et al., 2018).
Another important process that strongly influences the thermohaline dynamics of the Yucatan shelf is linked to continental discharge of water, which occurs predominantly as submarine groundwater discharge (SGD), due to the existence of a karstified limestone shelf in the northern Yucatan Peninsula. The limestone structural heterogeneities make it difficult to identify the groundwater discharge locations on the coast. Nevertheless, the continental groundwater has been observed to follow a preferential flow associated with the Chicxulub crater’s outer ring (Pope et al., 1991; Sharpton et al., 1993; Perez-Ceballos et al., 2021). Hence, visible submarine groundwater discharges exist in the Yucatan coastal sea where the crater rings intersect with the coast mainly at Dzilam Bravo and Celestun on the west (Enríquez et al., 2013, see Figure 1). A major port and several urban developments occur at Progreso, where higher concentrations of ammonium and phosphates could be associated with urban wastewaters (Alvarez-Gongora and Herrera-Silveira, 2006).
The processes explained above, contribute with different nutrient combinations to the marine environment and the phytoplankton is bound to have different responses to these physical processes. There are several ecological and taxonomic studies on the different marine ecosystems of the Yucatan shelf. For example, works carried out on coastal lagoons (Herrera-Silveira, 1998; Herrera-Silveira et al., 1999; Nava-Ruiz and Valadez, 2012) show that the phytoplankton is dominated by cyanobacteria and chrysophyceae communities near SGD, and by marine diatoms near the tidal channels. Tychoplanktonic diatoms are strongly present in coastal regions of the Yucatan shelf (Merino-Virgilio et al., 2013). Whereas coastal regions influenced by SGD (Troccoli-Ghinaglia et al., 2010; Alvarez Gongora et al., 2012) show the predominance of highly silicified pennate diatoms, and a strong presence of benthic diatoms. Coastal regions that have clear anthropic impacts have also shown a change in the phytoplankton community consisting of the dominance of dinoflagellates (Álvarez-Góngora et al., 2012). Records of phytoplankton species found offshore, on the oligotrophic regions of the Yucatan shelf have been published by Merino-Virgilio et al. (2013). Most of the studies of phytoplankton in the Yucatan Shelf are focused on the Cabo Catoche upwelling region, the first studies available where performed in 1960’s decade, including several Cuban-Soviet reports and data (Okolodkov, 2003). Phytoplankton studies in Cabo Catoche since 1960 have found dominance of small diatoms like Pseudo-nitzschia pungens, Chaetoceros affinis, Ch. messanensis among others (Licea et al., 2017). But when phytoplankton is transported to the west of the Yucatan shelf, bigger diatom species tend to dominate the community, for example Skeletonema costatum, Chaetoceros compressus and Guinardia striata (Licea et al., 2017).
In chlorophyll concentration studies of the Yucatan shelf, the Cabo Catoche upwelling has been proposed as the main physical process affecting the phytoplankton and marine community in general (Reyes-Mendoza et al., 2019; Medina-Gómez et al., 2020). On the other hand, regions influenced by SGD and anthropogenic waste have the potential of modifying their trophic state, leading to eutrophication and changes in the phytoplankton community (Troccoli-Ghianglia et al., 2010; Morales-Ojeda et al., 2010). Until now, those aforementioned studies clearly show that the effects of upwelling (marine influence) and SGD (terrestrial influence) on phytoplankton are different, but there is still a need to understand and further clarify the community transformations that follow when advection and mixing occurs through regions of dissimilar nutrient sources. Furthermore, the intrinsic spatio-temporal variability of the processes involved hampers the identification of effects if the dynamics are not considered.
Despite there are large uncertainties on the effects of climate change on tropical rain (Chadwick et al., 2016); storms are very likely to produce more intense precipitation events due to the increased water holding capacity of air in a warmer atmosphere. Even where total precipitation is decreasing, the risk of flooding is increasing (Trenberth, 2011). This was observed during 2020 in the Yucatan peninsula when several storms (Cristobal, Gamma and Delta) caused extreme rain, generating flooding and much increased SGD. Given the accelerated growth in human activities on land, it is of very high relevance to understand the effects of anthropogenic nutrient sources on the phytoplankton dynamics.
Previous studies have laid the foundations of SGD influences on the phytoplankton communities of the Yucatan shelf (Troccoli-Ghianglia et al., 2010; Álvarez-Góngora and Herrera-Silveira, 2006; Alvarez Gongora et al., 2012). Their analyses were based on traditional ecological indicators (richness, diversity, etc.), linked to measurements of environmental variables (temperature, salinity, nutrients). They found strong relationships between local hydrological characteristics, and phytoplankton community structure. For instance, more alpha diversity was reported in regions of high continental water influence (Álvarez-Góngora and Herrera-Silveira, 2006) with SGDs areas dominated by dinoflagellates and unpolluted SGDs locations dominated by diatoms with high silicification (Troccoli-Ghinaglia et al., 2010; Alvarez Gongora et al., 2012). These studies focused their analysis on geographical regions very close to shore (0.2 – 4 km) where SGDs processes are thought to be more abundant. Also, in previous studies, the emphasis of the causative analysis was by using direct environmental variables to identify their link with phytoplankton, hydrochemistry, or trophic status (Morales-Ojeda et al., 2010).
In this work, we built upon these experiences, but rather than using direct environmental measurements (temperature, salinity, oxygen) we calculated indexes that compile the variables that assess the existence of the two processes conceived as the most important nutrient inputs (SGD and upwelling) on shelf-wide scale. We also assigned high importance to the dynamic characteristics of the water masses, which were bound to the hydrodynamic conditions that will advect, disperse and mix different water parcels that influenced the marine environment. Finally, we applied a powerful statistical tool (dbRDA) to establish the links between the physical forcing, nutrient contents and phytoplankton communities. Then, phytoplankton communities were examined with a functional perspective (trophic characteristics – autotrophs vs mixotroph).
The Yucatan Shelf is usually associated with the northern section of the Campeche Bank, a very shallow continental shelf that extends approximately 200 km offshore with nearly monotonic slope of approximately 1:1000, which is sporadically interrupted by atolls and cays. It is one of the largest shelves in the world. This physiography promotes a somehow simple circulation with a very persistent westward flow of 0.2 m/s in average over the shallow shelf (< 40 m), driven by the trade winds and modulated by sea breezes and tides, which are predominantly diurnal and of small range (max 0.7 m during springs) (Enriquez-Ortiz et al., 2010; Ruiz-Castillo et al., 2016; Torres-Freyermuth et al., 2017). During autumn and winter, cold front events (strong gusty winds from the northern quadrant) can disrupt this circulation for a few days, inverting it (Kurczyn et al., 2021). To the east, the shelf is bordered by the Yucatan current, a high velocity (~ 1.5 m/s) western boundary current, which flows northward, parallel to the coast of Quintana Roo and into the Gulf of Mexico (Figure 1).
Samples and data were obtained during three oceanographic campaigns carried out from 2 to 20 November 2015 (GOMEX 04), 24 August to 9 September 2016 (GOMEX 05), and 5 to 18 July 2018, (GOMEX 06). A grid of oceanographic stations was designed to cover the entire Yucatan shelf (from ~ 15 m depth to the shelf break). The core analysis of this study was focused on the coastal stations with depths between 15 and 20 m. Temperature, pressure, salinity, dissolved oxygen and fluorescence profiles were measured at each station using a CTD Seabird 9 plus. The CTD data were filtered, aligned and interpolated using standard procedures included in the Seabird processing software. These data are used to determine the influence of SGD and upwelling water parcels on the shelf.
Water samples were collected with Niskin bottles, from which fractions were taken to study the concentration of nutrients in the water and the phytoplankton community. For each sampling station, surface and bottom water samples were processed and analyzed with the spectrophotometric method (Strickland and Parsons, 1972) at the Primary Production Laboratory, of CINVESTAV-IPN campus Mérida, to obtain the concentrations of NO3, NH4, PO3 and SiO4. Another fraction of these water samples were fixed with lugol iodine solution to estimate the abundance and diversity of phytoplankton using the Utermöhl sedimentation method (Hasle, 1978). The samples were analyzed in sedimentation chambers using an inverted microscope, and the species were identified and counted in the phytoplankton section of the same laboratory. Several results of the three oceanographic campaigns (interpolated maps of temperature, salinity, chlorophyll, T-S diagrams, CTD profiles, etc.) and their weather conditions (rain, wind, etc.) have been published already (Medina-Gomez et al., 2020). Medina-Gómez et al. (2020) dataset was used to generate indexes linked to processes regarded as the most important for nutrient loading on the shelf (i.e. upwelling and SGD).
In order to understand the movement of SGD and upwelling influenced waters, surface current velocity data for the region were downloaded from HYCOM products (experiment GOMl0.04 with 1/25° resolution from https://www.hycom.org/data/goml0pt04) for each campaign, which included 31 days of data encompassing two weeks prior to the initial date of each cruise and during the time of the cruise. Two-dimensional simulations were performed seeding 1000 inert particles in each zone of interest (see the Results section). The velocity data from HYCOM was fed off-line into the Ichthyop model version 3.3. Ichthyop is a free Java tool designed to study the effects of physical and biological factors on drifter dynamics (Lett et al., 2008). In this study the model was used to obtain dispersion patterns of Lagrangian tracers without behavior, to approximate the possible pathways of water parcels and any properties contained within (nutrients, plankton, etc). These results provided a first approximation to guide the data interpretations, despite the fact that no behavior was included to alter any transport pathways. Ichthyop required that the ocean current data from HYCOM were rearranged in a matrix of a specific order and in NetCDF format file, which was made using Matlab. The model set up included a Runge–Kutta 4th order advection numerical scheme, a default value of horizontal diffusion along the advection path (1 x10-9) and a time step of 30 minutes.
In order to link the phytoplankton communities with the nutrient sources, and therefore the processes linked to their development, it was necessary to identify areas within the shelf under the influence of SGD and upwelling. Several previous investigations have shown that the upwelling at Cabo Catoche can be identified by tracing the location of the 22.5°C isotherm, and its intensity is linked to the depth of such isotherm, the shallower the more intense the upwelling (Cochrane, 1966; Ruiz-Renteria, 1979; Merino, 1997; Enriquez et al., 2013). This temperature value corresponds to the upper thermal limit of the Yucatan upwelled water, which originates from the subsurface underwater (SUW) also called subsurface Caribbean water (Merino, 1997; Enriquez et al., 2013). According to Merino (1997), who made several oceanographic cruises in the area, salinity variations are small in the SUW, therefore it plays a secondary role in characterizing the Yucatan upwelling and therefore temperature became a good proxy for upwelling intensity and location. Furthermore the salinity range of the upwelled water was similar to that of the existing salinity on the Yucatan shelf becoming irrelevant on the identification of the upwelled water mass. The depth of the 22.5°C isotherm was identified in each station and was interpolated to generate maps using the Diva interpolation (Troupin et al., 2012) available in the Ocean Data View software.
Various approaches were used to define the SGD zones of influence. Firstly, a Dilution Index (DI, Equation 1) was defined and calculated for each oceanographic station as:
in which S is the salinity recorded at each depth, and Sm is a reference salinity, calculated as the mean for the first 10 meters of the water column in a region least affected by continental water or any other coastal influence. The integration along the first 10 m of the water column also helped to outweigh the effect of torrential rain that remains on the surface. The geographic position of such a reference area was approximately 23.8° N, 88° W, the farthest station on the north east corner of the Yucatan shelf. This value is calculated independently for each oceanographic cruise. The integration on the first 10 m of the water column was designed to better capture buoyant surface water since the deeper station on the coast is 20 m. Negative values of such an index indicated water that was diluted as compared to oceanic water, and positive values denoted hyperhaline conditions, both tended to occur at stations closer to the coast. Maps of this variable were also generated using the DIVA interpolation (Troupin et al., 2012). Although such an index adequately captured the regions of continental SGD influence, the analysis was complemented using additional parameters to aid this estimation using a robust statistical method for classification. A machine learning technique was used for this purpose. This technique, Random Forest (Ho, 1995), is an ensemble learning method for classification that operates by constructing a multitude of decision trees using a training data set, and whose output is the class selected by most trees. The random nature of this method corrects the tendency of decision trees of overfitting to their training data set. As our “training” dataset we chose stations where SGD was more likely to be found, and stations where SGD was unlikely. Variables that indicate the presence of SGD, such as the dilution index (DI), and salinity itself were used, but also variables such as silicate concentration (Herrera-Silveira, 1998), and proximity to SGD outflow zones were considered. Several studies have found that significant inputs of dissolved silica are associated with terrestrial SGD, both worldwide and in the region (Herrera-Silveira, 1998; Rahman et al., 2019). Therefore, stations with high concentrations of dissolved silica could be interpreted as SGD influenced. The proximity to SGD outflow zones was calculated as the distance between each station and the nearest recorded source of inland discharge outflows (Enríquez et al., 2013; Pérez-Ceballos et al., 2021), with consideration of the advection patterns based on the results obtained with the Ichthyop model simulation. For example, stations that were very close to the SGD sources, but upstream (to the east), were discarded as this would imply dispersion of the groundwater against the direction of the pathway suggested by the model. The approach of considering the effects of advection helped to avoid errors in deciding which discharge zone was influencing which station. The Random Forest model returned the best classification of SGD influenced stations and a set of error data (out of bag), where a percentage of misclassified data was recorded. A list of the relative importance of the variables used for classification was also returned.
Having determined the influence of the two processes of interest in the study area (upwelling and groundwater discharges), along with the implications of hydrodynamics on their behavior (advection), the next step was to establish the associations of these processes with nutrients and the phytoplankton community. For this, only data from stations close to the coast were included, since the SGD effect is stronger there, and the upwelling is commonly detected in the eastern coast of the Yucatan shelf (Cabo Catoche).
For the ecological analysis, a set of independent variables (temperature, upwelling, salinity, SGD and nutrient concentration) were used to explain a new set of dependent variables corresponding to the phytoplankton community. Since there were many absences (zeros) in the species count matrices, the multivariate analyses were unable to properly solve the observed variability, therefore the phytoplankton were analyzed by species groups. Dinoflagellate species were grouped according to Smayda and Reynolds (2001), based on their ecological behavior. The diatoms found were grouped by genus, since it was not always possible to identify the species. On the other hand, existing methods for grouping diatoms, such as Williams and Kociolek (2011) are based on taxonomic criteria and give a very limited definition (only by 3 groups).
The data were processed and analyzed using various numerical and ecological methods (Legendre and Legendre, 2012). For example, the independent variables were standardized with state of the art methodologies (Borcard et al., 2011; Oksanen et al., 2018), and the biological data selected were transformed using Hellinger’s method to later calculate the Bray-Curtis dissimilarity. Both the standardized environmental matrix (independent variables) and the transformed biological matrix (dependent variables) were analyzed with a Distance Based Redundancy Analysis (dbRDA; Legendre and Anderson, 1999). This method can establish the relationship between dependent and independent variables in cases where biological data are not strictly metric (absence-presence, abundance, etc.) and have various values of zero. The ecological analysis of all data was computed using R language, with the help of some numerical ecology packages (i.e. vegan; Borcard et al., 2011; Oksanen et al., 2018).
The information provided by the dbRDA was related to the beta diversity (biological variation among sites in a community, in this case). This analysis can indicate the species that are representative to sites and relate them to environmental conditions and processes, also information of the biological variation of sites can be provided. Similar results in terms of species can be obtained when Beta Diversity is separated in its two components: Local contribution to beta diversity (LCBD) and species contribution to beta diversity (SCBD). LCBD shows the sites where unique species exist, while SCBD provides the importance of each species in the whole dataset in terms of biological variation (Legendre and De Caceres, 2013). In other words, LCBD values are comparative indicators of sites contributions to β diversity, whereas SCBD is the relative importance of each species to β diversity (Xia et al., 2022). These species usually have limited distribution, or are even unique species, with high abundance.
In order to focus the analysis in an ordered seasonal approach, results have been organized by month rather than year, in which July corresponds to the end of the dry season and beginning of the rainy season, August and September corresponds to the rainy season, and November corresponds to the beginning of the dry season with northerly storms.
The upwelling events from the three oceanographic campaigns were very different. Two oceanographic campaigns were carried out during summer, the season in which the Cabo Catoche upwelling is usually at maximum, inundating the coastal zone of the Yucatan shelf with cooler waters (Zavala-Hidalgo et al., 2006; Varela et al., 2018). Figure 2 shows the depth of the 22.5°C isotherm as an index to identify regions influenced by the upwelling, as explained in the methodology. Upwelling was observed during July 2018 with particular intensity (GOMEX 06, Figure 2a). The presence of the 22.5°C isotherm was widespread on the Yucatan shelf, including coastal stations (Cabo Catoche, Dzilam, Progreso, Sisal) and at the NNW edge of the shelf. The most intense upwelled water presence (shallower 22.5°C isotherm depths) was observed in the coastal stations with cool temperatures close to the surface. The dispersion patterns observed during that time (Figure 3a) suggest that particles from the upwelling source at Cabo Catoche followed a NNW pathway spreading over most of the outer shelf, beyond the 40 m isobath. This explains the widespread distribution of the upwelled water mass offshore, but fails to explain its presence on the coastal stations. The 2018 cruise will be referred to as the “Major upwelling and minor SGD” condition. From this point in the paper, these names are used to describe the processes dominating during each cruise.
Figure 2. Upwelled water distribution and depth for each oceanographic campaign. Colors represent the depth of the 22.5°C isotherm as an upwelling index, (a) July 2018, (b) August-September 2016, (c) November 2015.
Figure 3. Particle dispersion patterns on the Yucatan shelf obtained with HYCOM circulation and the Ichthyop model. (a) Major upwelling and minor SGD scenario (July 2018), (b) Minor upwelling and SGD scenario (August-September 2016), and (c) No upwelling nor SGD scenario (November 2015).
In August-September 2016 (GOMEX 05), two parcels with upwelled water were recorded: at Cabo Catoche, and at the north-northwestern edge of the Yucatan shelf (Figure 2b). The Cabo Catoche water parcel had a very shallow (~ 0 m) 22.5°C isotherm, which was an indication of an intense, yet localized upwelling process taking place. The second water parcel with upwelling characteristics was deeper in the water column (> 75 m) and therefore disappearing. The dispersion patterns (Figure 3b) show that particles from the upwelling source at Cabo Catoche would follow a NW path arriving at the same offshore region shown in Figure 2b after 20 to 30 days. Therefore, the presence of upwelled water on the NW region of the shelf at this time could be explained mainly by advection of upwelled water from the Cabo Catoche region. The 2016 data will be referred to as the “Minor upwelling and SGD” condition, as will be further explained in the following section.
During November 2015 (GOMEX 04, Figure 2c) temperatures of 22.5°C or below were largely absent on the Yucatan shelf, except for three stations on the outer shelf, where such temperatures were found close to the sea bottom. The dispersion patterns are able to explain the presence of the upwelling water masses in those localities (see Figure 3c). Since no significant upwelling water masses were found, nor evidence of SGD, this campaign’s data will be regarded as the “No upwelling nor SGD” condition. The upwelled water is expected to be greatly reduced or absent during autumn and winter on the Yucatan shelf (Varela et al., 2018; Reyes-Jiménez et al., 2023).
The dilution of seawater index (DI) was calculated for the three scenarios analyzed. In stations where the dilution index (DI) showed large negative values, the vertically integrated salinity was fresher (diluted) as compared to the salinity of an offshore station on the shelf edge (~200 km from the coast) where freshwater sources are unlikely. The behavior of the three scenarios was so different that their DI values needed their own color bar in order to appreciate the differences (Figure 4). In the Major upwelling and minor SGD scenario (July 2018), the DI values show saltier than usual conditions on the shelf (predominantly positive values), which could be explained by the combination of widespread upwelling water masses on the shelf, which have high salinity values, and local evaporation processes which tend to increase salinity on the coastal zone, to be later transported NW (Enriquez et al., 2013; Figure 4a). Mild negative values were found at Dzilam, a known SGD outflow, and in the Yucatan Channel, which indicate that the Caribbean water had lower salinities (marine nonetheless) than the salinity over the Yucatan shelf. The greatest changes in DI values were recorded for the Minor upwelling scenario (August 2016), with the stronger dilution (negative values) found towards the west close to the shore, suggesting inputs of water from the mainland, either locally generated or advected from elsewhere (Figure 4b). The patterns of Lagrangian dispersion found for this scenario (Figure 3b) could explain a transport to the west of diluted water from the main source at Dzilam Bravo (~ 89°W), a region consistently found as a major source of diluted water in several previous studies (Enriquez et al., 2013; Murgulet et al., 2020). Nevertheless, the possibility of local outflow was not ruled out, since observations of diluted coastal waters towards the west have been reported (Murgulet et al., 2020). In the No upwelling nor SGD scenario (November 2015), the DI showed an increase in salinity from east to west, with the western coastal areas showing the greatest increase in salinity (Figure 4c). This suggests very little or no SGD influence during this time since no negative DI values were found. Here, the more diluted region was the area close to the Yucatan Channel, although salinities were always within marine values.
Figure 4. Dilution index (DI) for (a) the Major upwelling and minor SGD scenarios (July 2018), (b) the Minor upwelling and SGD (August-September 2016), and (c) the No upwelling nor SGD (November 2015). Due to the wide differences among the scenarios in its variation of DI, color scale was adjusted for each one.
In order to strengthen the identification of stations that were under the influence of diluted water from the mainland, a Random Forest classification model was applied using the DI and extra criteria such as the distance to the known sources of SGD, silicate as an indicative of terrestrial SGD (Herrera-Silveira, 1998; Rahman et al., 2019), and salinity itself. An “out of bag error” (OOB error) of 14.71% was obtained, which means that 85.29% of the stations had a good probability of being properly classified. The key most important variables for the model in descending order were: DI with a value of 4.52, silicate concentration with 3.92, distance to the nearest discharge source with 3.73, and finally salinity with 3.46. Figure 5 presents the results.
Figure 5. Maps showing the stations classified with presence of SGDs using the Random Forest model from the three oceanographic campaigns: (a) Major upwelling and minor SGD, (b) Minor upwelling and SGD (c) No Upwelling nor SGD scenarios.
For the Major upwelling and minor SGD scenario, only the area near Dzilam de Bravo was identified as SGD influenced (Figure 4a). In the Minor upwelling and SGD scenario, the stations classified as being influenced by SGDs were all in the western region of the shelf, close to shore, coinciding with the result of Figure 4b; while for the No upwelling nor SGD scenario, only two stations were classified with SGD influence, one near Celestún, and the other in the Holbox area (Figure 4c).
Particles for dispersion were initially placed at three zones (seeding sites) which represent the main processes that deliver nutrients to the shelf, as explained in the introduction. Cabo Catoche (east), represents the most common origin of the upwelling, Dzilam (middle) and Celestun (west) have been the locations of major SGD influence due to recurrent observations of diluted marine water (Enríquez et al., 2013), which coincide with the intersection of a ring of sinkholes with the coast.
In the “Major upwelling and minor SGD” scenario, the particles from the upwelling source (Cabo Catoche) showed a dispersion pathway towards the NNE and NW, broadening considerably its area of influence to encompass a vast region seaward of the 40 m isobath towards the NW. No evidence exists that the upwelled water followed a coastal pathway. Simulations for the SGD sites (middle and west) showed an initial western path, to turn north after 12 days of simulation, and finally follow again a westerly direction (Figure 3a). Differences among Lagrangian drifters and diluted substances in numerical modeling must be considered for the previous simulations, as diluted substances are usually transported slower than particles.
For the “Mild upwelling and SGD” scenario, the simulation from the three seeding sites showed a much wider advection, mainly towards the west, but spreading considerably towards the north and south of the shelf. The dispersion patterns from the middle SGD zone (Dzilam) showed a fairly fast transport close to the shore westward, to broaden considerably on the western part of the shelf (Figure 3b).
The dispersion patterns for the “No upwelling nor SGD” scenario showed rapid movement in a tight cluster close to shore from the SGD-influenced area of Dzilam de Bravo (middle) towards the west of the shelf. At Celestun, the particles followed a fast southwestward path at the beginning, to invert direction at the end of the simulation making its way back towards the shore. The particles seeded on the east (Cabo Catoche) were dispersed into two groups, one advected northwards, with the flow of the Yucatan Current, accumulating on the NW part of the shelf below the 50 m isobath after 25 to 30 days. The second group was transported 130 km westward along the coast at the beginning, but made their way back towards the Caribbean up to Cabo Catoche (Figure 3c).
Since the influence of SGD was restricted only to the coastal stations (see Figures 4 and 5), the phytoplankton community was only analyzed at those stations for each of the three scenarios. For the No upwelling nor SGD scenario, 37 species of centric diatoms were recorded, 38 species of pennate diatoms and 63 species of dinoflagellates. In the Minor upwelling and SGD scenario, 40 species of centric diatoms were recorded, 42 species of pennate diatoms and 68 species of dinoflagellates. In the Major upwelling and minor SGD scenario, 20 species of centric diatoms were recorded, 22 species of pennate diatoms and 44 species of dinoflagellates. For the Minor and Major upwelling scenarios a new classification was included, the unknown flagellates, which constituted various flagellated planktonic organisms that could not be identified with light microscopy. The oceanographic campaigns of the Minor and Major upwelling scenarios were performed later than the No upwelling nor SGD scenario (2 and 5 years respectively), several methods, such as the classification for unknown organisms changed. To identify the species that were most important for the variability of the community, we investigated which species were unique, in terms of their abundance and distribution (i.e. species with a high abundance at a given site). Thus, for each species its contributions to the beta diversity was analyzed and is reported in Table 1.
To gain some insight into the distribution of the main phytoplankton taxa analyzed on the coastal stations, the population density of each taxon was spatially plotted (Figure 6). In the No upwelling nor SGD scenario, diatoms were most abundant towards the west, but in the upwelling (Minor and Major) scenarios most diatoms were focused on stations with upwelled water influence, towards the east (Cabo Catoche). On the other hand, dinoflagellates were present mostly on the west in all three scenarios, and in the Minor upwelling and SGD scenario, their presence coincided with the SGD influenced stations. Unknown flagellates in general did not show a clear spatial pattern and seem to be present everywhere.
Figure 6. Maps showing abundance of phytoplankton population groups for the coastal stations. (a–c) Major upwelling and minor SGD scenario, (d–f) Minor upwelling and SGD scenario, and (g, h) No upwelling nor SGD scenario. Circle sizes represent concentrations in cells/L.
In the Major upwelling and minor SGD scenario, the dbRDA explains 61% of the variability. In this case, no significance could be obtained for the model, axis or variables, making this test less precise than the other two oceanographic cruises. This scenario showed the association of the DI and silicate concentration to the Dzilam area, which coincides with the fact that this area had intense SGDs. Here the associated species were naked dinoflagellates. The Río Lagartos and Holbox areas had the highest ammonium and phosphate concentrations, as well as the greatest number of species groups, such as diatoms Dactyliosolen fragilissimus, Climacodium spp. and the Ceratiaceae dynoflagellates. The highest nitrate concentrations were for the Progreso zone, where the diatom species associated were the Rhizosolenia spp, Guinardia spp., Cylindrotheca closterium and thecate dinoflagellates. This scenario showed a separation between Celestun and Progreso with associations to increased salinity in the first. The species associated with Celestun were the diatom Thalassiosira spp. and dinoflagellates Gymnodiniales (Figure 7). In this scenario, the effect of the upwelling was widespread from the region of Dzilam to Progreso as shown in the analysis, but was also mixed with effects of dilution from the SGD found in Dzilam, where there was a clear association with the dilution index and silicates.
Figure 7. dbRDA for the Major upwelling and minor SGD scenario. The environmental variables are shown in blue letters, the species in black letters. Localities: Cel, Celestun (Red polygon); Pro, Progreso (White polygon); Dzi, Dzilam de Bravo (Blue polygon); RL, Rio Lagartos (Yellow polygon); Hol, Holbox (Green polygon). Environmental variables: Temp, Temperature, Sal, Salinity; Dil, Dilution Index; Up, Upwelling presence. Diatoms: Cli, Climacodium spp.; Cyl, Cylindrotheca spp.; Dacfra, Dactyliosolen fragilissimus; Gui, Guinardia spp.; Rhi, Rhizosolenia spp.; Thlsr, Thalassiosira spp. Dinoflagellates: Gym, Gymnodiniales; NakD, Naked dinoflagellates; TecD, thecate dinoflagellates; Phl, Unknown flagellates.
For the Minor upwelling and SGD scenario, the dbRDA model explained 57% of the variance, and was significant at the 0.01 confidence level. The first axis was significant at 0.05. In this scenario, the most important variables in explaining the biological variation were the presence of the upwelling, and the silicate concentration which was linked to the dilution index (DI) and nitrates. At Cabo Catoche and Holbox (east of the domain) the presence of upwelling was most noticeable, with several diatom species associated (Navicula sp, Thalassionema spp, Pseudo-nitzschia delicatissima complex, Cylindrotheca closterium and Guinardia spp). On the other hand, the western side (Progreso and Celestun areas) was associated with increments in variables linked to SGD, such as silicate concentrations, dilution index, temperature, nitrates and phosphates. The species groups associated were naked dinoflagellates, thecate dinoflagellates and unknown flagellates. Dzilam was associated with ammonium and diatom species such as Dactyliosolen fragilissimus, and Leptocylindrus danicus, as well as some level of association with unknown flagellates (Figure 8). For this scenario, the effects of upwelling are clearly separated from those linked to SGD on the different phytoplankton groups and sites, as shown by the dbRDA analysis. This reflects the fact that this oceanographic cruise was made in conditions in which both processes could be clearly observed but were somehow independent.
Considering the dbRDA results (Table 2), for the No upwelling nor SGD scenario, the most important variables were temperature, density, and ammonium, with significance at 0.01, and DI with significance at 0.1 (Figure 10). Model explanation, and significance are shown in Table 2, column 1. Chrysophytes were associated with the highest temperatures, increases in salinity and phosphates near Progreso. Near Celestun (west corner) mainly diatom Thalassionema spp. and the Ceratiaceae dinoflagellates were recorded. Ammonium was associated with the area of Progreso-Dzilam, together with diatom species of the genus Navicula. Increased silicate concentrations and denser water were associated with eastern stations (Cabo Catoche, Holbox and Río Lagartos areas), and diatom species such as: Pseudo-nitzschia delicatissima complex, and Licmophora spp. Associations within this zone also include increases in DI and nitrate, associated with diatom species of the genus Pleurosigma (Figure 10). Despite these associations, this dbRDA showed no clear separation of regions or different variables, being all overlapped in the center.
In the following sections, we expand the analysis for each identified condition. Since the different oceanographic cruises correspond to distinct seasons (even though they were sampled across different years), the analysis is organized by month, reflecting varying seasonal conditions. However, it is important to note that interannual variability may influence these seasonal patterns.
In the Yucatan Peninsula, the month of July 2018 was characterized by low rainfall values (Medina-Gómez et al., 2020, Figure 2) which means lower aquifer levels and therefore limited SGD for this scenario. The Major upwelling and minor SGD scenario, was characterized by a widespread effect of the upwelled water masses over large parts of the Yucatan shelf, but mixed with effects of SGD close to shore and background marine conditions. The thermohaline conditions associated with the upwelled water mass were present very close to the surface in Cabo Catoche, indicating the intensity of the process there. Then its influence extended towards the NW, away from the coast, and returned close to shore from Dzilam towards the west (Progreso-Celestun), leaving a gap in the region between Holbox and Rio Lagartos, where this water mass was not detected (Figure 2a). The dispersion patterns for this scenario sustained these findings rather well, showing that the drifters traveled from Cabo Catoche towards the NW, away from the coast and with widespread dispersion, and some even reached the Loop current and returned to the shelf. The western presence of the upwelled water mass (T<22.5°C) was conceived as part of a previous event (older than the 15 days of the dispersion simulation previous to the cruise). Several investigations have shown that the upwelled water mass can be present along the northern Yucatan coast (Enriquez et al., 2013) up to northern Campeche (Ruiz-Castillo et al., 2016; Varela et al., 2018; Kurczyn et al., 2021). In such shallow continental shelf, subjected to high solar radiation, the existence of water masses with cool temperatures (T< 23.5°C) can be explained by either i) advection along the shelf from Cabo Catoche (Enriquez-Ortíz et al., 2010, Enriquez et al., 2013; Varela et al., 2018; Kurczyn et al., 2021), or ii) wind-driven Ekman transport that can carry such water from the outer shelf towards the coast (Ruiz-Castillo et al., 2016). In this oceanographic campaign upwelling favorable easterly winds blew for at least 6 consecutive days, which could have pumped the upwelled water towards the surface, as suggested by previous authors (Ruiz-Castillo et al., 2016; Varela et al., 2018). Under both scenarios the origin of the cool water was thought to be the Cabo Catoche upwelling on the eastern edge of the shelf.
On the other hand, SGD showed mild effects, with most influence near the coast in front of Dzilam Bravo (negative values of DI, Figures 4a and 5b), as expected, and towards Rio Lagartos. The dispersion processes carried SGD water (from Dzilam) westwards up to Celestun, promoting mixing of these conditions close to shore, and then offshore (to the north, Figure 3a).
The distribution of phytoplankton groups matched the combined effects of upwelling and SGD mixing. For example, the upwelling index and high NO3 were strongly associated with the region between Dzilam Bravo and Progreso (Figure 7), where the upwelled water mass was present close to the surface and in association with diatoms such as Guinardia and Cylindrotheca closterium, species typical of the upwelling region (as observed on the Minor upwelling and SGD scenario). At the same time, towards Dzilam Bravo all this co-existed with associations to the dilution index (DI), silicates (SiO4), dinoflagellates (thecate and naked) and a brackish water-resistant diatom Rhizosolenia supporting the idea of a combined upwelling-SGD influence. The mixing of upwelling and SGD was in turn supported by the dispersion patterns, as mentioned before (see Figure 3a). It would be expected that the phyto- and mixoplankton dynamics followed the microbial loop described in Glibert and Mitra (2022), where species substitution could be in place as diatoms are succeeded by mixotrophic dinoflagellates which feeded on organic compounds from SGD, and would be succeeded by the more stress-resistant phytoplankton, similar to species as Ceratium spp. and Dinophysis spp (Smayda and Reynolds, 2001).
Two other regions detached from this behavior: Celestun, which showed associations with high salinity and species such as the diatom Thalassiosira and the Gymnodiniales dinoflagellates of marine influence; and the region from Rio Lagartos to Holbox, associated with NH4, PO4 and increase in temperature. Here the phytoplankton associations were with Dactyliosolen fragilissimum, a species found associated with mild SGD and NH4 on the other scenarios, and dinoflagellates of genus Ceratium. The greatest populations of unknown flagellates in this scenario were near Rio Lagartos (Figure 6c), supporting an association with both SGD and the microbial loop. In this scenario the unknown flagellates population were much smaller than in the Minor upwelling scenario (concentrations of 104 vs 106 cels/liter), which might be due to milder effects of SGD.
Of the two scenarios with upwelling, the Minor upwelling and SGD scenario gave the best insights on the biological consequences of these two processes, which seem to control most of the nutrient input and therefore the phytoplankton community of the inner Yucatan shelf. This was supported by a clear separation of the variables (and associated species) relevant to upwelling (upwelling index) from those relevant to SGD (DI, silicates, other nutrients) in the dbRDA (Figure 8). The upwelling index for this scenario (Figure 2b) clearly indicated the existence of upwelled water masses on the East, and the DI and random forest analysis showed that SGD was very active on the west (Figures 4b, 5b).
Figure 8. dbRDA for the Minor upwelling and SGD scenario. The environmental variables are shown in blue letters, the species in black letters. Localities: Cel, Celestun (Blue polygon); Pro, Progreso (Yellow polygon); Dzi, Dzilam de Bravo (Green polygon); RL, Rio Lagartos (Blue-green polygon); Hol, Holbox (White polygon); Cab, Cabo Catoche (Red polygon). Environmental variables: Temp, Temperature; Sal, Salinity; Dil, Dilution Index; Up, Upwelling presence. Diatoms: Cli, Climacodium spp.; Cyl, Cylindrotheca spp.; Dacfra, Dactyliosolen fragilissimus; Gui, Guinardia spp.; Lep, Leptocylindrus spp.; Nav, Navicula sp; Nit, Nitzschia spp.; PseNit, Pseudo-nitzschia delicatissima complex.; Rhi, Rhizosolenia spp.; Thlsn, Thalassionema spp. Dinoflagellates: Gym, Gymnodiniales; NakD, Naked dinoflagellates; TecD, Thecate dinoflagellates; Phl, Unknown flagellates.
The upwelling is identified as a provider of new nutrients to the system, and its first biological consequence was a high concentration of diatoms off Cabo Catoche, as seen in the maps of the species groups at the sampling stations (Figure 6d). Species such as Pseudo-nitzschia delicatissima complex, Navicula, Guinardia striata, and Cylindroteca closterium were abundant in Cabo Catoche and statistically associated with the upwelling index (Figure 8). P. pungens has been reported in studies from the 1980s (Licea et al., 2017). P. delicatissima and P. pungens are capable of producing neurotoxins in the eastern side of the Yucatan shelf. Diatoms are typical of upwelling regions due to their ability to consume NO3 more efficiently than other phytoplankton groups (Lomas and Glibert, 2000). This is because of the range of nitrogen transporters they have in their membranes (Lampe et al., 2018), including various membrane transporters for ammonium (Kang and Chang, 2014). This preference for NO3 consumption is often seen in upwelling systems (e.g. Wilkerson et al., 2006; Edwards et al., 2005; Hauss et al., 2012), in nitrate-added phytoplankton cultures (Ferreira et al., 2020), and in algal blooms (Heisler et al., 2008). The diatom population increases rapidly (with logistical growth) as a result of the additional nitrogen, and declines due to herbivory pressure, competition with other algal groups (mainly mixoplankton) and damage caused by heterotrophic bacteria and virioplankton (Glibert and Mitra, 2022). This high efficiency of NO3 uptake by diatoms can also be the reason behind no NO3 association with the upwelling (Figure 8).
The dispersion patterns shown for this scenario (Figure 3b) suggest that the advection of upwelled water masses from Cabo Catoche towards the west, occurred along the inner shelf but without reaching the coast (contrary to the No upwelling nor SGD scenario, Figure 3a). There was no evidence of connection between the upwelling and the SGD in this scenario, which suggests that the biological processes linked to the upwelling were not likely to occur in the inner western shelf. Furthermore, there was clear evidence that from Dzilam westwards, SGD had a profound influence on the water characteristics. In this case, the dispersion patterns from Dzilam showed a wide pathway along the inner shelf, but the particles were clearly reaching the coast and mixing more or less homogeneously towards the west (Progreso and Celestun) dispersing the effects of SGDs (Figure 3b). In this region of the northwestern coast, there was an increase in dinoflagellate populations (thecate and naked, Figure 6d), and a clear association between them and the environmental variables associated with groundwater discharges (Dilution, SiO4 and other nutrients, Figure 8). Another indication of SGD influence was the presence of unknown flagellates, whose concentrations were considerably high in the order of 106 cells/liter near Dzilam de Bravo and Progreso (Figure 6). Many of the unknown flagellates were considered mixotrophs, with different types of mixotrophy or osmotrophy (Glibert and Mitra, 2022; Glibert and Legrand, 2006), and their populations behaved similarly to those of the microbial loop (Glibert and Mitra, 2022). Therefore, the phytoplankton community of the western side was very different from the eastern communities, and linked to SGD and its nutrient composition.
In this study, Dissolved Organic Carbon (DOC) and Dissolved Organic Nitrogen (DON) were not part of the sampling plan, so our analysis does not have any data of DOC or DON available to associate with the phytoplankton data. Despite this limitation, DOC and DON are inferred to be in higher concentration in SGDs. It is already accepted that higher concentration of DOC and DON stimulates the presence of mixoplankton over pure photosynthetic phytoplankton (diatoms), and also it is possible that DOC is preferred over DON in algal bloom events dominated by mixoplankton (Glibert and Legrand, 2006). In the present contribution, the shift in the phytoplankton community to dinoflagellate-dominated towards the west (Figure 6) on the Minor upwelling and SGD scenario, was statistically linked to SGD presence. This implied a decay in diatoms, possible alteration (increase) of the N:P ratios, and a likely increase in dissolved and particulate organic carbon, as is often the case at river mouths or close to SGDs. The large amount of organic matter found in such places is thought to be consumed by some mixotrophic dinoflagellates, which can absorb this organic matter into their cells via alternative metabolic means (i.e. osmotrophy, Glibert and Legrand, 2006). High DOC concentrations have been recorded in similar karstic systems (Quintana Roo groundwater), and various processes may occur around nearshore discharge sites that control nutrient dynamics. It had been suggested that in anchialine systems and subterranean estuaries, water that was high in DOC concentration infiltrated the karst. This then enters the microbial loop, with heterotrophic and methanogenic bacteria decomposing the DOC, that was then consumed by detritivorous organisms (Brankovits et al., 2017). Remineralization of organic matter occurs in Yucatan SGD systems, with net CO2 export, high concentrations of NH4 that can be rapidly oxidized to NO3 once in the water column, and sequestration of remineralized phosphorus (Pain et al., 2020). Although northern Yucatan underground systems have some differences from the Quintana Roo coast, very similar processes are bound to occur, such as the export of allochthonous or autochthonous DOC and the associated nutrients. This will mainly occur in the zones of preferential discharge off the northern Yucatan coast (Celestun and Dzilam de Bravo), in areas of urban wastewater discharge (Progreso), and will be dispersed by the existing hydrodynamics. The association of NH4 to the SGD site (Dzilam), suggests the occurrence of regenerative production processes from SGD.
In our study, unknown flagellates populations increased where there was already a reduction in diatom populations. Due to the probable mixotrophy of the unknown flagellates (microphotographs of some organisms considered as unknown flagellates are provided in Figure 9), these populations may continue to increase where there is some source of DOC (Glibert and Legrand, 2006), or high DIN: DIP ratios (linked to inland water discharges). The increased presence of unknown flagellates in the Progreso area was very much in line with the work of Glibert (2016) who described the conditions where this type of organism is predicted to occur: warm water, regenerated production, higher amounts of dissolved organic matter from SGD. In addition, the presence of unknown flagellates in conjunction with naked dinoflagellates around Progreso agreed with earlier work on zone delimitation according to water quality and phytoplankton (Herrera-Silveira and Morales-Ojeda, 2009).
Figure 9. (a-c) Correspond to microphotographs of some of the organisms considered as unknown flagellates, these microphotographs were provided by Daniela Medina-Euan.
The main characteristic of this scenario was that the influence of upwelling and SGD on the phytoplankton community was not clearly separated nor evident, since the behavior of the dbRDA was rather congested with no clear separations (Figure 10). This could be interpreted as a period of low importance of the two processes, and absence of environmental factors that could generate such behavior on the phytoplankton community.
Figure 10. dbRDA for the No upwelling nor SGD scenario. The environmental variables are shown in blue letters, the species in black letters. Localities: Cel, Celestun (Blue polygon); Pro, Progreso (Yellow polygon); Dzi, Dzilam (Green polygon); RL, Rio Lagartos (Red polygon); Hol, Holbox (White polygon); Cab, Cabo Catoche. Environmental variables: Temp, Temperature; Sal, Salinity; Dil, Dilution Index; Up, Upwelling presence. Diatoms: Cyl, Cylindrotheca spp.; Licm, Licmophora spp.; Nav, Navicula sp.; PseNit, Pseudo-nitzschia delicatissima complex.; Thlsn, Thalassionema spp. Dinoflagellates: Cer, Ceratians; Cris, Unknown Chrysophyceae.
During this oceanographic cruise, even when the upwelling index showed no clear effects of the upwelling process on the shelf, some remnants were found close to Cabo Catoche and on the northernmost part of the shelf. These seem enough to show some diatom species typical of the upwelling (Pseudo-nitzschia delicatissima complex, Cylindrotheca closterium, Guinardia spp.) associated with Cabo Catoche and Holbox, areas that were most separated from the rest of the polygons on the dbRDA analysis (see Figure 10). On the other hand, the DI had positive values (weak or null SGD) over the shelf with the lowest values close to the coast on the eastern side (Rio Lagartos) linked to high nitrate and silicate concentrations, an indication of mild SGD influence. The dispersion experiments demonstrated a connectivity between Rio Lagartos and Cabo Catoche which suggests that the above mentioned phytoplankton species could continue their presence thanks to the input of nutrients from mild SGD close to Rio Lagartos, as seen in the dbRDA (Figure 10).
The dispersion patterns for this scenario also showed that the tracers followed a track which transported all of them very close to the shore, enhancing the mixing of water masses from different sources. On the other hand, this time of the year (November) is characterized by the presence of northerly storms which were fairly active during this oceanographic campaign and absent in the others (see Figure 2 in Medina-Gomez et al., 2020). The main effect of these weather systems was the mixing that wind and waves induce in the water column, which will combine the effects of the different nutrient sources making them less evident. During this campaign, the genus Navicula and Licmophora, which are phytobenthos species, were found in the water column in stations near Dzilam-Progreso and Holbox. Navicula sp, was associated with high ammonium concentrations. This could be interpreted as a consequence of northerly storms suspending Navicula and recycled nitrogen from the bottom, making them available on the water column. This genus has high tolerance to ammonium (Underwood and Provot, 2000), and some Navicula species are even considered resistant to human pollution stress (Sullivan, 1999). Licmophora spp. was related to higher silicate concentrations, and is commonly attached to surfaces with flexible mucilage, forming an apical pad (Kooistra et al, 2007), strong bottom stress due to storms must be necessary to detach Licmophora spp. from their substrate.
The presence of an unknown Chrysophyceae was possibly related to groundwater discharge, most of the reported Chrysophyceans in the region were registered in cenotes (Díaz-Hernández et al., 2023) and coastal hyperhaline lagoons (most of them have SGD presence).
In general, the species dominance at each site can be explained by the fertilization source (upwelling vs SGD), the dispersion patterns, and the degree of mixotrophy of the species. Constitutive mixotrophic species (species that could form their own chloroplasts, as well as stole them from their prey) dominated by using alternative nutrients available in the environment, e.g. carbon and dissolved and particulate organic nitrogen (Glibert and Mitra, 2022). In areas such as Progreso and to the west (towards Celestun), where dissolved inorganic nutrients (NO3) concentrations were high, and particulate and dissolved organic matter from dispersed SGD were likely, mixoplankton tended to dominate (e.g. dinoflagellates and unknown flagellates, see Figure 6) in response to the wide variety of nutrients.
In this work, dissolved or particulate organic nutrients were not quantified, but given the results found here, we recommend they should be examined in the future, since they can trace inland sources of water (Chen et al., 2003), and better explain mixoplantkon dynamics (Glibert et al., 2006).
This paper examined the relative importance of upwelling and submarine groundwater discharges on the nutrient concentration and community composition of phytoplankton on a wide and shallow continental shelf, where oceanographic processes played a very important role on the advection and mixing of water masses. Although other processes might be in place (i.e. sediment resuspension and nutrient recycling), the ones considered here had been recognized to be the most important (Morales-Ojeda et al., 2010).
A novel approach of data integration contributed to a better understanding of the link between physical, and biogeochemical dynamics. This consisted on the quantification of important processes using analytical indexes to identify the presence of upwelling and SGD on data from three oceanographic cruises that spanned different hydrographic and hydrodynamic conditions (No upwelling nor SGD, Minor upwelling and SGD, Major upwelling and minor SGD). The dynamics of the identified processes was characterized through numerical modeling of dispersion, resulting from the oceanic currents encountered during each campaign. This helped to establish the connections between physical processes. Finally, statistical associations were established with the hydrographic variables, nutrient concentration and phytoplankton species found in the coastal stations, where SGD is most likely to have influence.
When the Cabo Catoche upwelled water caused fertilization, the phytoplankton community became dominated by diatoms, mainly Pseudo-nitzschia delicatissima complex, Thalassionema, Navicula, Guinardia striata, and Cylindrotheca closterium, as seen in the Minor upwelling scenario. Populations of dinoflagellates were scarce in the region where the upwelled water influence dominates. The phytoplankton community that emerged from the upwelling was advected westwards, and depending on the dispersion patterns, it could be mixed with SGD-influenced water parcels that dominated the western coast. In this case, the trophic dynamics were modified and photoautotrophic phytoplankton coexisted with mixotrophic populations (dinoflagellates and unknown flagellates), as observed in the Major upwelling and minor SGD scenario. If the upwelled water and SGD did not mix, the phytoplankton population had scarce diatom presence towards the SGD influenced regions (which changed with dispersion patterns) and the dinoflagellates and unknown flagellates dominated. They obtained newly available nutrients from the SGDs (e.g. DIN, DON, DOC and PON) which made them thrive as their metabolism was more mixotrophic than photoautotrophic, improving their survival rates and leading to their dominance.
These results revealed how relevant are the local processes of continental shelves in controlling the phytoplankton community in terms of its diversity and species dominance. Previous studies and microbial loop recent paradigms show that mixotrophy is a key factor in the trophic chain (Glibert and Mitra, 2022) and depending on the available substrate, mixotrophy may affect in different ways the population dynamics of autotrophic phytoplankton, constitutive and non constitutive mixotrophs. Our results showed and explained how the species replacement depends on the interaction and connectivity of the dynamical processes responsible for nutrient loading in the marine environment such as SGD and upwelling.
Strict autotrophic phytoplankton (diatoms) is related to the upwelling process in Cabo Catoche. Upwelled water is transported westwards through the Yucatan Shelf, and the phytoplankton community tends to change. As reported in previous works (Licea et al., 2017) small chain pennate diatoms were replaced with bigger centric diatoms (Pseudo-nitzschia delicatissima complex was replaced for Guinardia striata, in this case). Phytoplankton populations decreased from their upwelled origin at Cabo Catoche, until they reached SGD influenced regions. SGDs can provide inorganic and organic nutrients, which increased mixotrophic phytoplankton populations. The diluted and particulate organic matter derived from human activities produced ecological conditions where mixotrophs could consume and dominate over autotrophs, increasing the mixotroph population in the western coast of Yucatan (Figure 11). It is possible that in a strong upwelling event, the upwelling ecological conditions had dominated over SGDs ecological conditions, with both autotrophs and mixotrophs coexisting in very high abundances in several parts of the shelf near the coast.
Figure 11. Autotrophic and Mixotrophic phytoplankton relation to processes. Blue polygons indicate the upwelling area and the upwelling affected zone in strong upwelling events. Green polygons indicate the SGDs affected area. Plotted species include the most important autotrophs as Pseudo-nitzschia delicatissima complex and Guinardia Striata, and mixotrophs as thecate and naked dinoflagellates.
Northerly winds and storms produced strong water mixing near the coast, hiding the effects of SGDs. The salinity trace of the SGD could be unnoticeable due to fast diffusion, and difficult to be identified with our Dilution Index. Also northerly winds occurred in months when the Yucatan Upwelling was less active. Phytoplankton communities also were different in Northerly winds. Autotrophs tended to be more persistent, and phytobenthos species from the genus Navicula could be detached from the bottom and be registered in very high abundances suspended in the water column (Figure 12).
Figure 12. Mixed water scenario due to northerly wind events. Gray polygon indicate the water mixing zone in this scenario. Plotted species include Navicula sp. and Pseudo-nitzschia delicatissima complex.
The raw data supporting the conclusions of this article will be made available by the authors, without undue reservation.
RB-R: Conceptualization, Data curation, Formal analysis, Methodology, Software, Validation, Visualization, Writing – original draft, Writing – review & editing. IM-T: Conceptualization, Funding acquisition, Resources, Software, Validation, Writing – review & editing, Supervision. JH-S: Funding acquisition, Methodology, Writing – review & editing, Data curation. CE: Data curation, Methodology, Software, Validation, Writing – review & editing.
The author(s) declare that financial support was received for the research and/or publication of this article. The first author ‘s doctoral scholarship was granted by the Consejo Nacional de Humanidades, Ciencias y Tecnologías (CONAHCyT, CVU: 855210). Funding for data was possible thanks to the project Consorcio de Investigación del Golfo de México (CIGOM) from the CONAHCYT and Secretaría de Energía (SENER).
I thank the National Autonomous University of Mexico (UNAM), and the Posgrado en Ciencias del mar y Limnología from the UNAM which provided me with a doctoral degree. I also thank the technicians who obtained and processed the samples: Emanuel Uc Sanchez, Giuliana Cruz Trejo, Ana Aguilar-Trujillo, Fanny Merino-Virgilio, Daniela Medina-Euan, and Ileana Osorio-Moreno. Also special thanks for the recommendations and comments to Yury B. Okolodkov. We wish to thank Mrs Jill Taylor for the initial review of the English language.
The authors declare that the research was conducted in the absence of any commercial or financial relationships that could be construed as a potential conflict of interest.
The author(s) declare that no Generative AI was used in the creation of this manuscript.
All claims expressed in this article are solely those of the authors and do not necessarily represent those of their affiliated organizations, or those of the publisher, the editors and the reviewers. Any product that may be evaluated in this article, or claim that may be made by its manufacturer, is not guaranteed or endorsed by the publisher.
Álvarez-Góngora C., Herrera-Silveira J. A. (2006). Variations of phytoplankton community structure related to water quality trends in a tropical karstic coastal zone. Mar. pollut. Bull. 52, 48–60. doi: 10.1016/j.marpolbul.2005.08.006
Álvarez-Góngora C. C., Liceaga-Correa M. A., Herrera-Silveira J. A. (2012). Variaciones estacionales de la estructura comunitaria del fitoplancton en zonas de descarga de agua subterránea en la costa norte de la Península de Yucatán. Rev. Biología Trop. 60, 157–172. 10.15517/rbt.v60i1.2749
Belousov I. M., Ivanov Y. A., Pasternak S. A., Rass T. S., Rossov V. V. (1996). Oceanographic research by the soviet-cuban marine expedition. Oceanology 6 (2), 312–320.
Borcard D., Gillet F., Legendre P. (2011). Numerical ecology with R Vol. 2 (New York: Springer), 688.
Brankovits D., Pohlman J. W., Niemann H., Leigh M. B., Leewis M. C., Becker K. W., et al. (2017). Methane- and dissolved organic carbon-fueled microbial loop supports a tropical subterranean estuary ecosystem. Nat. Commun. 8, 1–12. doi: 10.1038/s41467-017-01776-x
Bulanienkov S. K., Garcia C. (1973). Influencia de los procesos atmosféricos en el afloramiento del Banco de Campeche Vol. 2 (Cuarta Reunión de Balance de Trabajo, Centro de Investigaciones Pesqueras/Instituto Nacional de la Pesca, Cuba, Informe de Investigación), 28.
Chadwick R., Good P., Martin G., Rowell D. P. (2016). Large rainfall changes consistently projected over substantial areas of tropical land. Nat. Climate Change 6, 177–181. doi: 10.1038/nclimate2805
Chen K., Liu K., Macdonald R. (2003). “Continental margin exchanges,” in Ocean biogeochemistry: The role of the ocean carbon cycle in global change. Ed. Fasham M. J. R. (Berlin, Germany: Springer-Verlag), 82.
Cochrane J. D. (1966). “The Yucatan Current, upwelling off Northeastern Yucatan, and currents and waters of Western Equatorial Atlantic,” in Oceanography of the Gulf of Mexico (Progress Rep, TAMU, Ref No. 66-23T), 14–32.
De Falco C., Desbiolles F., Bracco A., Pasquero C. (2022). Island mass effect: A review of oceanic physical processes. Front. Mar. Sci. 9, 894860. doi: 10.3389/fmars.2022.894860
Díaz-Hernández J. A., Ugalde-Silva P., Berriozabal-Islas C., Novelo A., Hernández-Uc J., Arana-May A., et al. (2023). Aquatic microdiversity from urban cenotes in Cancun, Quintana Roo, Mexico. Subterranean Biol. 46, 129–145. doi: 10.3897/subtbiol.46.108082
Edwards V., Icely J., Newton A., Webster R. (2005). The yield of chlorophyll from nitrogen: a comparison between the shallow Ria Formosa lagoon and the deep oceanic conditions at Sagres along the southern coast of Portugal. Estuar. Coast. Shelf Sci. 62, 391–403. doi: 10.1016/j.ecss.2004.09.004
Enriquez C., Mariño-Tapia I. (2014). “Mechanisms driving a coastal dynamic upwelling,” in Proceedings of the 17th Physics of Estuaries and Coastal Seas (PECS) Conference. 17–20 (Brazil: Porto de Galinhas Pernambuco).
Enríquez C., Mariño-Tapia I., Jeronimo G., Capurro-Filograsso L. (2013). Thermohaline processes in a tropical coastal zone. Continental Shelf Res. 69, 101–109. doi: 10.1016/j.csr.2013.08.018
Enriquez-Ortíz C., Mariño-Tapia I., Herrera-Silveira J. A. (2010). Dispersion in the Yucatan coastal zone: Implications for red tide events. Continental Shelf Res. 30, 127–137. doi: 10.1016/j.csr.2009.10.005
Ferreira A., Sá C., Silva N., Beltrán C., Dias A. M., Brito A. C. (2020). Phytoplankton response to nutrient pulses in an upwelling system assessed through a microcosm experiment (Algarrobo Bay, Chile). Ocean Coast. Manage. 190, 105167. doi: 10.1016/j.ocecoaman.2020.105167
Glibert P. M. (2016). Margalef revisited: A new phytoplankton mandala incorporating twelve dimensions, including nutritional physiology. Harmful Algae 55, 25–30. doi: 10.1016/j.hal.2016.01.008
Glibert P. M., Harrison J., Heil C. A., Seitzinger S. (2006). Escalating worldwide use of urea—a global change contributing to coastal eutrophication. Biogeochemistry 77, 441–463. doi: 10.1007/s10533-005-3070-5
Glibert P. M., Legrand C. (2006). “The Diverse Nutrient Strategies of Harmful Algae: Focus on Osmotrophy,” in Ecology of Harmful Algae. Ecological Studies, vol. 189 . Eds. Granéli E., Turner J. T. (Springer, Berlin, Heidelberg).
Glibert P. M., Mitra A. (2022). From webs, loops, shunts, and pumps to microbial multitasking: Evolving concepts of marine microbial ecology, the mixoplankton paradigm, and implications for a future ocean. Limnol. Oceanogr. 67, 585–597. doi: 10.1002/lno.12018
Hasle G. R. (1978). “Using the inverted microscope,” in Phytoplankton Manual. Ed. Sournia A. (Organización de las Naciones Unidas para la educación, la ciencia y la cultura, Paris, Francia), 191–196.
Hauss H., Franz J. M. S., Sommer U. (2012). Changes in N:P stoichiometry influence taxonomic composition and nutritional quality of phytoplankton in the Peruvian upwelling. J. Sea Res. 73, 74–85. doi: 10.1016/j.seares.2012.06.010
Heisler J., Glibert P., Burkholder J., Anderson D., Cochlan W., Dennison W., et al. (2008). Eutrophication and harmful algal blooms: A scientific consensus. Harmful Algae 8, 3–13. doi: 10.1016/j.hal.2008.08.006
Herrera-Silveira J. A. (1998). Nutrient-phytoplankton production relationships in a groundwater-influenced tropical lagoon. Aquat. Ecosystem Health Manage. 1, 373–385. doi: 10.1080/14634989808656931
Herrera-Silveira J. A., Martín M., Díaz-Arce V. (1999). Variaciones del fitoplancton en cuatro lagunas costeras del Estado de Yucatán, México. Rev. Biología Trop. 47, 47–56.
Herrera-Silveira J. A., Morales-Ojeda S. M. (2009). Evaluation of the health status of a coastal ecosystem in southeast Mexico: Assessment of water quality, phytoplankton and submerged aquatic vegetation. Mar. pollut. Bull. 59, 72–86. doi: 10.1016/j.marpolbul.2008.11.017
Hillebrand H., Steinert G., Boersma M., Malzahn A., Meunier C. L., Plum C., et al. (2013). Goldman revisited: faster-growing phytoplankton has lower N:P and lower stoichiometric flexibility. Limnol. Oceanogr. 58, 2076–2088. doi: 10.4319/lo.2013.58.6.2076
Ho T. K. (1995). “Random decision forests,” in Proceedings of 3rd International Conference on Document Analysis and Recognition, Montreal, QC, Canada, Vol. 1. 278–282.
Jouanno J., Pallas-Sanz E., Sheinbaum J. (2018). Variability and dynamics of the Yucatan upwelling: High-resolution simulations. J. Geophysical Research: Oceans 123, 1251–1262. doi: 10.1002/2017JC013535
Kang L., Chang J. (2014). Sequence diversity of ammonium transporter genes in cultured and natural species of marine phytoplankton. J. Mar. Sci. Technol. 22, 89–96. doi: 10.6119/JMST-013-0412-1
Kooistra W. H. C. F., Gersonde R., Medlin L. K., Mann D. G. (2007). “Chapter 11- The Origin and Evolution of Diatoms: Their adaptation to a Planktonic Existence,” in Evolution of Primary Producers in the Sea. Eds. Falkowski P. G., Knoll A. H. (Burlington, Massachusetts, USA: Academic Press).
Kurczyn J. A., Appendini C. M., Beier E., Sosa-López A., López-González J., Posada-Vanegas G. (2021). Oceanic and atmospheric impact of central American cold surges (Nortes) in the Gulf of Mexico. Int. J. Climatol. 41, E1450–E1468. doi: 10.1002/joc.v41.S1
Lampe R. H., Cohen N. R., Ellis K. A., Bruland K. W., Maldonado M. T., Peterson T. D., et al. (2018). Divergent gene expression among phytoplankton taxa in response to upwelling. Environ. Microbiol. 20, 3069–3082. doi: 10.1111/emi.2018.20.issue-8
Legendre P., Anderson M. J. (1999). Distance based redundancy analysis: Testing multispecies responses in multifactorial ecological experiments. Ecol. Monograps 69, 1–24. doi: 10.1890/0012-9615(1999)069[0001:DBRATM]2.0.CO;2
Legendre P., De Cáceres M. (2013). Beta diversity as the variance of community data: dissimilarity coefficients and partitioning. Ecol. Lett. 16, 951–963. doi: 10.1111/ele.2013.16.issue-8
Lett C., Verley P., Mullon C., Parada C., Brochier T., Penven P., et al. (2008). A Lagrangian tool for modelling ichthyoplankton dynamics. Environ. Model. Software 23, 1210–1214. doi: 10.1016/j.envsoft.2008.02.005
Licea S., Luna R., Okolodkov Y., Cortés-Altamirano R. (2017). Phytoplankton abundance and distribution on the Yucatan shelf (June 1979 and April 1983). Novosti Sist. Nizsh. Rast. 51, 121–144. doi: 10.31111/nsnr/
Lomas M. W., Glibert P. M. (2000). Comparisons of nitrate uptake, storage and reduction in marine diatoms and flagellates. J. Phycol. 369, 03–913. doi: 10.1046/j.1529-8817.2000.99029.x
Medina-Gómez I., Cahuich-López M., Aguilar-Trujillo A., Cruz-Trejo G., Juárez M., Mariño-Tapia I., et al. (2020). Spatio-temporal patterns of chlorophyll-a in a wide and low-relief shelf sea of the Gulf of Mexico: Insights of interannual climatic patterns on the phytoplankton biomass varying behavior. Continental Shelf Res. 205, 104174. doi: 10.1016/j.csr.2020.104174
Merino M. (1997). Upwelling on the Yucatan Shelf: hydrographic evidence. J. Mar. Syst. 13, 101–121. doi: 10.1016/S0924-7963(96)00123-6
Merino-Virgilio F. C., Okolodkov Y. B., Aguilar-Trujillo A. C., Herrera-Silveira J. A. (2013). Phytoplankton of the northern coastal and shelf waters of the Yucatan Peninsula, southeastern Gulf of Mexico, Mexico. Check List 9, 771–779. doi: 10.15560/9.4.771
Moore W. S. (2010). The effect of submarine groundwater discharge on the ocean. Annu. Rev. Mar. Sci. 2, 59–88. doi: 10.1146/annurev-marine-120308-081019
Morales-Ojeda S. M., Herrera-Silveira J. A., Montero J. (2010). Terrestrial and oceanic influence on spatial hydrochemistry and trophic status in subtropical marine near-shore waters. Water Res. 44, 5949–5964. doi: 10.1016/j.watres.2010.07.046
Murgulet D., Douglas A. R., Silveira J. A. H., Mariño-Tapia I., Valle-Levinson A. (2020). “Submarine groundwater discharge along the northern coast of the Yucatán peninsula,” in 16th Sinkhole Conference, 12-16 Abril, Puerto Rico.
Nava-Ruiz V., Valadez E. (2012). Planktonic flora from lagartos lagoon, quintana roo. Rev. Mexicana Biodiversidad 83, 561–582. doi: 10.7550/rmb.24868
Okolodkov Y. B. (2003). A review of Russian plankton research in the Gulf of Mexico and the Caribbean Sea in the 1960-1980s. HIDROBIOLÓGICA 13, 207–221.
Oksanen J., Blanchet F. G., Kindt R., Legendre P., Minchin P. R., O’Hara R. B., et al. (2018). vegan: community ecology package (R package version 2.5 – 2). Available at: https://CRAN.R-project.org/package=vegan.
Pain A. J., Martin J. B., Young C. R., Valle-Levinson A., Mariño-Tapia I. (2020). Carbon and phosphorus processing in a carbonate karst aquifer and delivery to the coastal ocean. Geochimica Cosmochimica Acta 269, 484–495. doi: 10.1016/j.gca.2019.10.040
Pérez-Ceballos R., Canul-Macario C., Pacheco-Castro R., Pacheco-Ávila J., Euán-Ávila J., Merino-Ibarra M. (2021). Regional hydrogeochemical evolution of groundwater in the ring of cenotes, Yucatán (Mexico): An Inverse Modelling Approach. Water 13, 614. doi: 10.3390/w13050614
Pope K. O., Ocampo A. C., Duller C. E. (1991). Mexican site for K/T impact crater? Nature 351, 105. doi: 10.1038/351105a0
Rahman S., Tamborski J. J., Charette M. A., Cochran J. K. (2019). Dissolved silica in the subterranean estuary and the impact of submarine groundwater discharge on the global marine silica budget. Mar. Chem. 208, 29–42. doi: 10.1016/j.marchem.2018.11.006
Reyes-Jiménez T., Athié G., Enriquez C., Sheinbaum J., Mariño-Tapia I., Marín-Hernández M., et al. (2023). Triggering mechanisms of the Yucatan upwelling. Continental Shelf Res. 255, 104910. doi: 10.1016/j.csr.2022.104910
Reyes-Mendoza O., Herrera-Silveira J., Mariño-Tapia I., Enriquez C., Largier J. L. (2019). Phytoplankton blooms associated with upwelling at Cabo Catoche. Continental Shelf Res. 174, 118–131. doi: 10.1016/j.csr.2018.12.015
Reyes-Mendoza O., Mariño-Tapia I., Herrera Silveira J. A., Ruiz Martínez G., Enriquez-Ortíz C., Largier J. (2015). The effects of wind on upwelling off cabo catoche. J. Coast. Res. 32, 638–650. doi: 10.2112/JCOASTRES-D-15-00043.1
Ruiz-Castillo E., Gomez-Valdes J., Sheinbaum J., Rioja-Nieto R. (2016). Wind-driven coastal upwelling and westward circulation in the Yucatan shelf. Continental Shelf Res. 118, 63–76. doi: 10.1016/j.csr.2016.02.010
Santos I. R., Chen X., Lecher A. L., Sawyer A. H., Moosdorf N., Rodellas V., et al. (2021). Submarine groundwater discharge impacts on coastal nutrient biogeochemistry. Nat. Rev. Earth Environ. 2, 307–323. doi: 10.1038/s43017-021-00152-0
Sharpton V. L., Burke K., Camargo-Zanoguera A., Hall S. A., Lee D. S., Marin L. E., et al. (1993). Chicxulub multiring impact basin: Size and other characteristics derived from gravity analysis. Science 261, 1564–1567. doi: 10.1126/science.261.5128.1564
Simpson J. H., Sharples J. (2012). Introduction to the Physical and Biological Oceanography of the Shelf Seas (Cambridge, UK: Cambridge University Press), 463.
Smayda T. J., Reynolds C. S. (2001). Community assembly in marine phytoplankton: application of recent models to harmful dinoflagellate blooms. J. Plankton Res. 23, 447–461. doi: 10.1093/plankt/23.5.447
Strickland J. D. H., Parsons T. R. (1972). A practical handbook of seawater analysis. Fishery Res. Board Canada 167, 263–266.
Sullivan M. J. (1999). “Applied diatom studies in estuarine and shallow coastal environments,” in The Diatoms: Applications for the Environmental and Earth Sciences. Eds. Stoermer E. F., Smol J. P. (Cambridge University Press, Cambridge, UK), 334–351.
Taniguchi M., Dulai H., Burnett K. M., Santos I. R., Sugimoto R., Stieglitz T., et al. (2019). Submarine groundwater discharge: updates on its measurement techniques, geophysical drivers, magnitudes, and effects. Front. Environ. Sci. 7, 141. doi: 10.3389/fenvs.2019.00141
Torres-Freyermuth A., Puleo J. A., DiCosmo N., Allende-Arandía M. E., Chardón-Maldonado P., López J., et al. (2017). Nearshore circulation on a sea breeze dominated beach during intense wind events. Continental Shelf Res. 151, 40–52. doi: 10.1016/j.csr.2017.10.008
Trenberth K. E. (2011). Changes in precipitation with climate change. Climate Res. 47, 123–138. doi: 10.3354/cr00953
Troccoli-Ghianglia L., Herrera-Silveira J. A., Comín F. A., Díaz-Ramos J. A. (2010). Phytoplankton community variations in tropical coastal area affected where submarine groundwater occurs. Continental Shelf Res. 30, 2086–2091. doi: 10.1016/j.csr.2010.10.009
Troupin C., Barth A., Sirjacobs D., Ouberdous M., Brankart J.-M., Brasseur P., et al. (2012). Generation of analysis and consistent error fields using the Data Interpolating Variational Analysis (Diva). Ocean Model. 52-53, 90–101. doi: 10.1016/j.ocemod.2012.05.002
Underwood G. J. C., Provot L. (2000). Determining the environmental preferences of four estuarine epipelic diatom taxa: growth across a range of salinity, nitrate and ammonium conditions. Eur. J. Phycol. 35, 173–182. doi: 10.1080/09670260010001735761
Varela R., Costoya X., Enriquez C., Santos F., Gómez-Gesteira M. (2018). Differences in coastal and oceanic SST trends north of Yucatan Peninsula. J. Mar. Syst. 182, 46–55. doi: 10.1016/j.jmarsys.2018.03.006
Wilkerson F. P., Lassiter A. M., Dugdale R. C., Marchi A., Hogue V. E. (2006). The phytoplankton bloom response to wind events and upwelling nutrients during the CoOP WEST study. Deep-Sea Res. Part II Top. Stud. Oceanogr. 53, 3023. doi: 10.1016/j.dsr2.2006.07.007
Williams D., Kociolek P. (2011). “An Overview of Diatom Classification with Some Prospects for the Future,” in The Diatom World (Dordrecht, Netherlands: Springer), 49–91.
Wolanski E., Delesalle B. (1995). Upwelling by internal waves, Tahiti, French Polynesia. Continental Shelf Res. 15)2/3, 357–368. doi: 10.1016/0278-4343(93)E0004-R
Xia Z., Heino J., Yu F., He Y., Liu F., Wang J. (2022). Spatial patterns of site and species contributions to β diversity in riverine fish assemblages. Ecol. Indic. 145, 109728. doi: 10.1016/j.ecolind.2022.109728
Zavala-Hidalgo J., Gallegos-García A., Martínez-López B., Morey S. L., O’Brien J. J. (2006). Seasonal upwelling on the western and southern shelves of the gulf of Mexico. Ocean Dynamics 56, 333. doi: 10.1007/s10236-006-0072-3
Keywords: diatoms, mixoplankton, nutrient dispersion, nutrient supply, upwelling, continental shelf, submarine groundwater discharge
Citation: Becerra-Reynoso R, Mariño-Tapia I, Herrera-Silveira J and Enriquez C (2025) Effects of upwelling and submarine groundwater discharges on phytoplankton communities off the north coast of the Yucatan peninsula. Front. Mar. Sci. 12:1516784. doi: 10.3389/fmars.2025.1516784
Received: 15 November 2024; Accepted: 10 March 2025;
Published: 01 April 2025.
Edited by:
Pierina Visciano, University of Teramo, ItalyReviewed by:
Márcio Silva de Souza, Federal University of Rio Grande, BrazilCopyright © 2025 Becerra-Reynoso, Mariño-Tapia, Herrera-Silveira and Enriquez. This is an open-access article distributed under the terms of the Creative Commons Attribution License (CC BY). The use, distribution or reproduction in other forums is permitted, provided the original author(s) and the copyright owner(s) are credited and that the original publication in this journal is cited, in accordance with accepted academic practice. No use, distribution or reproduction is permitted which does not comply with these terms.
*Correspondence: Román Becerra-Reynoso, MzA5MDEzNTM2QGVuZXNtZXJpZGEudW5hbS5teA==
Disclaimer: All claims expressed in this article are solely those of the authors and do not necessarily represent those of their affiliated organizations, or those of the publisher, the editors and the reviewers. Any product that may be evaluated in this article or claim that may be made by its manufacturer is not guaranteed or endorsed by the publisher.
Research integrity at Frontiers
Learn more about the work of our research integrity team to safeguard the quality of each article we publish.