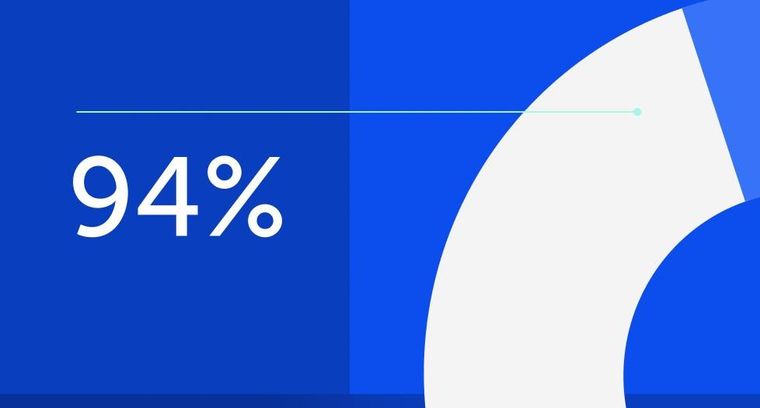
94% of researchers rate our articles as excellent or good
Learn more about the work of our research integrity team to safeguard the quality of each article we publish.
Find out more
ORIGINAL RESEARCH article
Front. Mar. Sci., 07 April 2025
Sec. Marine Biology
Volume 12 - 2025 | https://doi.org/10.3389/fmars.2025.1513138
Echinoids are an integral part of present-day and ancient marine trophic webs, and they host a variety of mutualistic, commensalistic, and parasitic epibionts on their spines and test. Cidaroid echinoid (slate pencil urchins) spines in particular are commonly colonized by epizoans. Eucidaris in the western Atlantic and eastern Pacific today are notable for the frequency and intensity of calcifying, non-calcifying, and galling colonization on their spines. While moderate levels of spine colonization may provide camouflage and other benefits to the host, a high density of encrusters may instead reduce host fitness, and galling is invariably parasitic. Significant environmental changes in the equatorial and sub-equatorial western Atlantic and eastern Pacific necessitate a paleobiological approach to constrain the timing of changes in epibiosis intensity on Eucidaris. Here, we compare rates of spine colonization in present-day Eucidaris populations with ancestral Pliocene Eucidaris assemblages. We find that Pliocene spines show no evidence of parasitic galling, and significantly less evidence of epibiosis than their present-day descendants in both the Atlantic and Pacific. This holds true even after accounting for taphonomic processes that would preferentially erase evidence of non-calcifying colonization. We propose that the high intensity of colonization on present-day Eucidaris spines is a relatively recent development and may reflect human-induced habitat degradation in the region, underscoring the need for further investigation into this biotic interaction.
Biotic interactions regulate whole ecosystem diversity, function, and stability, and are crucial components for understanding whole ecosystem responses to current and future environmental changes. Many biotic interactions are understudied because their dynamics are highly context-dependent (e.g. Sarkar and Das, 2021; Kumar et al., 2022), requiring research across various temporal scales to fully understand the impacts of environmental and biotic factors on these systems. Conservation paleobiology, which leverages diverse geohistorical archives (e.g., paleontological, archaeological, geochemical) to document pre-impact ecosystems and assess ecological consequences of human impacts, is increasingly used to gauge the magnitude and timing of human-driven shifts in populations and ecosystems (Kidwell, 2015; Dietl and Flessa, 2011; Rick and Lockwood, 2013; Barnosky et al., 2017; Tyler and Schneider, 2018; Dillon et al., 2022; Dietl et al., 2023), including changes in ecosystem composition (Barnosky, 1994; Burney et al., 2001; Kidwell, 2007; Kowalewski et al., 2015), productivity and biomass (Kowalewski et al., 2000; Jackson et al., 2001), and extinction risk (Finnegan et al., 2024; Kowalewski et al. 2023). These historical approaches have also been employed successfully to document changes in intensity and attributes of biotic interactions, including parasitism (e.g., Huntley et al., 2014; Scarponi et al., 2017) and predation (e.g., Cintra-Buenrostro et al., 2005; Smith and Dietl, 2016; Grun et al., 2017; Zuschin et al., 2024). Here, we employ geohistorical approaches to assess if interactions between epibionts and their echinoid hosts have changed in the recent past by comparing late Neogene and present-day records of spine epibiosis in the western Atlantic and eastern Pacific.
Although the study region has undergone several dramatic environmental, evolutionary, and climatic changes between the late Neogene and the present day (Jackson and O’Dea, 2013; Benitez et al., 2014), the persistence of numerous marine lineages and relatively minimal geographic changes in most areas make tracking the regional intensity and temporal changes in epibiont fouling more straightforward. The cidaroid urchin Eucidaris is a suitable candidate for comparative studies to constrain changes in intensity of biotic interactions (Figure 1) because it is common in both the Neogene and the present-day of the western Atlantic and eastern Pacific, having likely dispersed from the Indo-Pacific and across the Central American Seaway (CAS) before the formation of the Isthmus of Panamá (Mayr, 1954; Lessios et al., 1999). Echinoids in general are important participants in a number of biotic associations (e.g., Kowalewski and Nebelsick, 2003; Steneck, 2013), several of which produce evidence that can be reliably diagnosed and quantified in the fossil record (Farrar et al., 2020; Petsios et al., 2023 and references therein). Biotic interactions associated with the spines of cidaroid urchins such as Eucidaris can preserve fossil evidence of calcifying or bioerosive symbiotic associations. These associations can be identified and quantified from spines, even from disarticulated material, which is the common mode of Neogene cidaroid preservation (Cutress, 1980; Osborn et al., 2020). The three cidaroid species from the American tropics and subtropics examined in this study are particularly well-suited for this analysis due to their high abundance in both modern and fossil ecosystems within these regions, as well as the extensive history of systematic, biogeographic, and ecological research focused on these taxa: Eucidaris tribuloides (Lamarck, 1816, Figure 1), E. thouarsii (Valenciennes in Agassiz and Desor, 1846), and E. galapagensis (Döderlein, 1887). Eucidaris tribuloides has a present-day latitudinal range in the western Atlantic from North Carolina, U.S.A. to Brazil, and spans through the Gulf of Mexico, Caribbean, and Azores Islands (Mortensen, 1928). The fossil record of E. tribuloides extends back to at least the Pliocene (Cutress, 1980; Portell and Oyen, 1997) and potentially the Miocene (Lyell, 1845; Mayer-Emar, 1864; Rothpletz and Simonelli, 1890; Dartevelle, 1953; Ferreira, 1961; Maloney and Macsotay, 1968), and is widespread in the late Neogene and Quaternary of the Gulf of Mexico and Caribbean (Cutress, 1980; Donovan and Gordon, 1993; Donovan, 1993; Donovan et al., 1994; Donovan and Embden, 1996; Donovan and Lewis, 2009; Donovan and Portell, 2013; Gordon and Donovan, 1992) and in the Azores (Madeira et al., 2011). Eucidaris thouarsii and E. galapagensis are the two present-day species of Eucidaris in the eastern Pacific, and are both sister to E. tribuloides (Lessios et al., 1999). The distribution of E. thouarsii extends from Baja California, Mexico to Peru (Mortensen, 1928; González and Borrero-Pérez, 2020), while E. galapagensis is found on the isolated oceanic islands of the Galapágos Archipelago, Isla del Coco and Clipperton Atoll (Lessios et al., 1999). Although E. galapagensis had previously been considered a variant of E. thouarsii (Mortensen, 1928), Lessios et al. (1999) reestablished its status as a distinct species based on genetic differentiation from E. thouarsii. Though less extensively studied than the fossils in the Atlantic region, Eucidaris spines in the eastern Pacific are known from the Miocene and Pliocene of California, U.S.A. (Arnold, 1908; Mortensen, 1928; Hertlien and Grant, 1944; Vedder and Moore, 1976) and the Pleistocene of Isla Guadalupe and Baja California, Mexico (Lindberg et al., 1980). These fossil taxa are presumed to be ancestral to the present-day Eucidaris species in the region, as studies have shown that there has been minimal change in the ranges of extant taxa and their Pliocene ancestors (Finnegan et al., 2015).
Figure 1. Specimens of Eucidaris tribuloides, showing (A) present-day E. tribuloides from Key West, Florida (LACM E.1985-240.7), and (B) Pliocene E. tribuloides fossil from Tamiami Formation (UF 114517; FM locality CH046).
Epibionts (‘fouling’ organisms) are a common occurrence on present-day cidaroid echinoids (McPherson, 1968; Phelan, 1970; Salazar-Vallejo and López-Muraira, 1983; Hopkins et al., 2004) (Figure 2) but are largely absent in their crown-group sister-clade, the euechinoids. Complex and abundant spine epibiont associations are even documented in the ancestral archaeocidarids of the Paleozoic (Schneider, 2003; Schneider et al., 2010), signifying the deep evolutionary history of this biotic association. The presence of epibionts on living cidaroid spines is attributed to the lack of an epithelial layer in mature primary spines, resulting in an exposed cortex layer that allows for the settlement, attachment, and growth of fouling organisms (Mortensen, 1928; Märkel and Röser, 1983a; McKenzie and Grigolava, 1996). Several studies have systematically identified the diversity of epizoans and epiphytes found on present-day cidaroid spines, which include algae, foraminifera, sponges, hydrozoans, corals, polychaetes (including tubed serpulids and spirorbids), bryozoans, barnacles, isopods, brachiopods, molluscs, and other echinoderms (Steinbeck and Ricketts, 1941; Brusca, 1973, 1980; Salazar-Vallejo and López-Muraira, 1983; Gutt and Schickan, 1998; Hétérier et al., 2004, 2008; Linse et al., 2008; David et al., 2009; González and Borrero-Pérez, 2020). Of these associations, calcifying encrusting organisms have a robust fossil record (Rashwan et al., 2024), while evidence of soft-bodied or non-cemented epizoa is reliant on exceptional preservation quality (e.g., Schneider et al., 2010).
It is unclear whether spine-fouling epizoans and epiphytes are beneficial, neutral, or detrimental to the cidaroid basibiont, and few studies of spine fouling have attempted to constrain this since Mortensen (1928) first postulated on the nature of this association. Previous workers have proposed that biofilm and epibiont colonization plays a beneficial role for the cidaroid hosts, with the epibionts providing camouflage (Kier and Grant, 1965) and protection from spine dissolution under acidifying conditions (Dery et al., 2014; 2017). Others have postulated that fouling burden reduces fitness of the host echinoid and is collectively a parasitic association, likely through increased drag, encumbrance, and reduced motility of the spines and host (Mortensen, 1928; David et al., 2009), and by breaching and eroding the dense outer cortex of the spines (David et al., 2009; Dery et al., 2017). While echinoids have been documented to shed broken spines (Märkel and Röser, 1983b), field tagging has demonstrated that they do not preferentially shed fouled spines (McPherson, 1968), suggesting that, at least in isolation, colonized spines are not treated as “damaged” by the animal. It is likely that fouling associations can span the spectrum of beneficial to detrimental effects for the host, depending on the degree and mode of fouling, but to date no studies have determined if and where this tipping point lies.
In contrast, spine galling, (Figures 2N-U) a specialized symbiosis commonly reported in present-day Eucidaris populations (Thiele, 1925; Pilsbry, 1956; McPherson, 1968; Queiroz et al., 2017) as well as other cidaroid taxa (Warén, 1983), is recognized as wholly parasitic. The galling epibionts are thought to induce a skeletal hypertrophic response from the host (Jangoux, 1987; Ebert, 1988) where the irritant likely settles on immature cidaroid spines, stunting the growth of the infected spine and making it more susceptible to breakage (Figures 2O-R). Early stages of this association may appear as minimal swelling with an indentation at the attachment site (Figure 2U), while more well-developed galls appear as large swellings surrounding a cavity (Figure 2Q), which the eulimid often uses as a domicile (Queiroz et al., 2017). Certain species of the echinoderm-parasitizing gastropod family Eulimidae are known to form domicile galls on cidaroid primary spines (Warén, 1983; Queiroz et al., 2017). Specifically, the genus Sabinella specializes in galling Eucidaris spines, with S. shaskyi (Warén, 1992) galling eastern Pacific eucidarids and S. troglodytes (Thiele, 1925) specifically targeting the western Atlantic species Eucidaris tribuloides (Queiroz et al., 2017). Populations of Eucidaris in the American tropics and subtropics are common in shallow marine environments, have received more scientific attention than other cidaroids (e.g., Hopkins et al., 2004), are known to exhibit extensive epibiont colonization, and have a relatively well-sampled fossil record. Despite the ubiquity of eulimid-galled spines in these present-day Eucidaris populations, there is no known instance of eulimid galling preserved in the eucidarid fossil record (Petsios et al., 2023).
Figure 2. Examples of calcifying and non-calcifying epibionts found on Florida Shelf Eucidaris tribuloides individuals from the FWC collections. Spines with: (A) serpulid encrustation, (B) Chama bivalve, (C) Spirorbis polychaete worm tubes and bryozoa, (D) algae and polychaete worm tubes, (E) Spirorbis polychaete worm tubes, (F) bryozoa and algae, (G) bryozoa, (H) poriferan. (I) unknown soft-bodied epibiont, (J) filamentous algae, (K) pedunculate barnacle, (L) branching bryozoa, and (M) bryozoa. Spines with: (N) gall with biofilm covering spine, (O) spine with apex missing above gall, (P) active eulimid gall causing stunted and malformed growth of spine, with serpulid encrustation, (Q) non-active eulimid gall with empty cavity, (R) active eulimid gall on stunted or broken spine. (S) parasitized spine (arrow indicating eulimid parasite) with no obvious gall cavity, (T) spine with multiple eulimid parasites attached, and (U) spine with a minute cavity, likely in early stages of gall formation. Scale bar 1 cm.
The apparent lack of Pliocene galling in the extant genus Eucidaris hints at a relatively recent shift in the nature of spine epibiosis in general. Since parasitic galling and high densities of spine epibionts may be associated with diminished host fitness in present-day populations, spine colonization rates could reflect temporal changes in eucidarid host fitness specifically and the dynamics of these biotic interactions more generally. To detect trends and drivers of this biotic association, we must first establish historical levels of spine colonization in Eucidaris populations. Here, we quantify the frequency of spine colonization and degree of epibiont burden on the three living cidaroid species from the American tropics and subtropics, including the western Atlantic and eastern Pacific, and compare infestation frequencies between their modern and Pliocene Eucidaris populations from California and Florida (Figure 3). Human-induced disruption of habitats (e.g., overfishing, contamination) can complexly impact levels of epibiosis in echinoids (Sonnenholzner et al., 2011), suggesting human activity may mediate these biotic associations and the larger trophic webs they belong to. As ocean warming is projected to increase intensity of fouling throughout marine ecosystems (Dobretsov et al., 2019; Khosravi et al., 2019), this study elucidates the response of epibiosis in eucidarids specifically, in terms of frequency in populations and intensity of epibiosis on individuals, and in marine ecosystems experiencing changing climate more broadly. The quantitative assessment of epibiont fouling investigated here serves two goals. First, the study aims to quantify any notable changes in epibiont fouling that may be temporally linked to human impacts. Second, the data and analyses presented below aim to improve our understanding of ecological and taphonomic aspects of host-epibiont interactions to improve our ability to interpret fossil occurrences of epibiont fouling.
Modern echinoid datasets – The frequency of spine colonization and degree of epibiont burden on present-day American Eucidaris populations was quantified using two datasets of different resolutions (Supplementary Table S1): a high-resolution but limited geographic scope dataset of individual spines compiled from surveyed populations of E. tribuloides on the Florida Shelf (Gulf of Mexico; referred to as Florida dataset), and a dataset of field photographed Eucidaris individuals reported from the equatorial and sub-equatorial western Atlantic and eastern Pacific on the Global Biodiversity Information Facility (GBIF) database (gbif.org; referred to as the GBIF dataset). The spine-level Florida dataset was constructed using individuals of E. tribuloides that were collected between 1964 and 2012, preserved in ethanol, and cataloged at the Florida Biodiversity Collection (FWRI, FWC) as part of the Southeast Area Monitoring and Assessment Program (SEAMAP) surveys. The Florida dataset includes 54 localities across the Florida coast and shelf (Figure 3B), 115 individual echinoids and 4,361 spines.
Figure 3. Map of the (A) American tropics and subtropics showing the occurrences of Eucidaris specimens used for this study, including fossil collections (black diamonds, fossil dataset), and living individuals from field photographs of E. tribuloides (filled orange diamonds, GBIF dataset) and E. thouarsii and E. galapagensis, combined (filled blue diamonds. GBIF dataset). Inset (B) shows a close-up of Florida shelf individuals of E. tribuloides (orange open diamonds, Florida dataset) from the FWC collections.
To assess the epibiont burden on a larger swath of present-day Eucidaris populations, an additional dataset (the GBIF dataset) was constructed by querying GBIF (accessed August, 2024, see Supplementary Table S3 for full list of citations) for occurrences of the three Eucidaris species entered with expert identification, geospatial information, and associated specimen multimedia in the form of field photographs. Photographs were manually filtered based on whether the individuals reported were a) in their natural habitat (not removed from life position), and b) of sufficient quality such that the spines of the individual were clearly visible. Occurrences of E. thouarsii and E. galapagensis were pooled due to the absence of rigorous non-molecular (i.e., morphological) criteria to differentiate the two (Lessios, 2005), making field-based and photograph-based identifications dubious. Additionally, disarticulated Eucidaris fossil spines from the eastern Pacific cannot be confidently distinguished at the level of species (Mortensen, 1928; Ruiz-Nava et al., 2021), so grouping present-day E. thouarsii and E. galapagensis makes the two datasets more directly comparable.
Fossil echinoid dataset – Evidence of epibionts in fossil Eucidaris populations was assessed using disarticulated spine material from Petsios et al. (2023). The dataset (referred to here as the fossil dataset) includes 474 disarticulated spines identified as belonging to Eucidaris, likely E. thouarsii or E. galapagensis, from the Pliocene San Diego Formation (California, U.S.A.) from the Natural History Museum of Los Angeles (LACMNH), and 404 disarticulated spines identified as belonging to E. tribuloides from the Pliocene Tamiami Formation (Florida, U.S.A.) from the Florida Museum of Natural History (FLMNH), University of Florida (UF). These cataloged collections consisted of aggregated disarticulated spine material gathered by multiple collectors over several years and from various localities. To avoid overestimating spine counts or underestimating epizoan presence, fragmentary spines and those significantly abraded were excluded from the analysis.
Spine colonization frequency – Mature primary spines, including ambital and aboral spines, from specimens of the Florida dataset were counted per individual specimen and tallied based on a) the presence of calcifying epibiont colonization, b) the presence of non-calcifying (soft-bodied) epibionts, or c) no evidence of epibionts. Epibionts were identified to the lowest taxonomic level possible with the use of a binocular microscope. In these counts, newly regenerated immature spines (as determined by their size and absence of a fully developed outer cortex) were not counted. Additionally, oral spines (spines positioned in the two most adoral rows of spines) were excluded from the count, as these highly specialized spines are morphologically, functionally, and developmentally non-analogous to primary spines (Cutress, 1965) and are rarely colonized, likely due to their active role in movement and feeding (Salazar-Vallejo and López-Muraira, 1983). Galled spines were counted separately. Ambital test size was recorded for all individuals using digital calipers (± 0.001 inches), and ranged between 1.18 cm and 6.07 cm test size.
Fossil Pliocene spines (Figure 4) were individually tallied and calcifying colonizers (including polychaetous, bryozoan, poriferan, molluscan, cirripedian, or foraminiferan epibionts) were noted as present or absent. Additionally, evidence of bioerosion (e.g., polychaete burrows [figured in Salazar-Vallejo and López-Muraira, 1983] and corrosion by foraminifera [figured in David et al., 2009]) was tallied when present. The present-day spine dataset of Florida Eucidaris populations was most comparable to the disarticulated spine level data collected from the fossil record (but see discussion section for known caveats), so these two datasets were compared to constrain the effects of sample size on observed counts. A 1000-replicate resample of the present-day spine data using the Tamiami Formation fossil spine sample number (n = 404) was used to simulate comparable sampling between fossil and present-day spine populations. The proportion of different spine types recovered from bootstrap resampling efforts was reported as the 95% interquartile range.
Epibiont burden score – In addition to scoring each spine, each individual from the Florida dataset was also scored using a semi-quantitative ranking metric for macroscopic epizoan burden based on the expected impact on individual fitness. Individuals were ranked from 0 – 3 with 0 representing no/minimal evidence of epizoa, having no impact on the host, and 3 representing greater than half of spines with epizoa, likely having a significant negative impact through reduced spine movement (see Table 1 for full scoring criteria and Supplementary Figure S1 for example specimens, and Supplementary Table S2 for identified epibionts). These scores were also applied to the GBIF dataset. Each individual from the GBIF dataset with associated multimedia data was then scored using the same semi-quantitative epizoan burden score used for the first dataset and described above (Table 1). This was done to also gauge the agreement between the two present-day datasets. The primary purpose of the GBIF dataset was to assess whole-individual epibiont burden across a broader geographic region than was available from the Florida spine-based dataset. While whole-individual epibiont burden can be reliably quantified using these field photographs, the detection of galled spines, particularly on the often-obscured oral surface, cannot be consistently relied upon. Although some instances of galled spines were observed in the GBIF dataset and are reported here for transparency, we do not consider these frequencies to accurately represent actual population galling rates.
Table 1. Rubric table of epibiont burden score criteria for scoring Florida and GBIF field photographed specimens and the associated impacts on host fitness of each burden score category.
Statistical analyses – A Wilcoxon rank-sum test (with Bonferroni adjustment applied where appropriate) was used to assess the significance of differences in epibiont burden scores between groups (α = 0.05). Linear regression analysis was performed to evaluate relationships within the spine-level Florida dataset, with ANOVA used to test for significance (α = 0.05). To generate 95% confidence intervals for observed spine colonization rates given the sample size, we used 1000 replicate bootstrap resampling and calculated the 95% confidence intervals based on these simulated distributions using the statistical software R.
Taphonomic Experiments – To assess the influence of postmortem transport and abrasion on the preservation of evidence of epibionts in disarticulated spines, tumbling experiments were conducted with colonized, non-colonized, and galled E. tribuloides spines. Ten non-colonized spines, ten colonized spines (including calcifying and non-calcifying epibionts), and six galled spines were removed from ethanol-preserved individuals collected from the Florida Shelf, air dried, and weighed. Each group was then tumbled in a cylindrical drum separately at 120 RPM in a mixture of 500 mL of Instant Ocean (Spectrum Brands, Blacksburg, VA; diluted to manufacturer specifications) and 50 g of aquarium sand at room temperature in 24-to-32-hour sessions. Spines were then retrieved via sieving and allowed to air dry completely before weighing to measure the loss of material. Fresh mixture of Instant Ocean and sand was used for each tumbling session. Each group was tumbled for a total of 152 hours, until the preservation state of the spines matched those observed in the fossil spines (rounded spine tips, eroded base, and loss of beaded rib texture).
Spine Colonization – Individuals from the Florida dataset exhibited a range between 0 to 100% of spines colonized per individual, with an average of 70.5% of spines of an individual colonized. Considering the aggregate of all spines examined from the Florida dataset regardless of the individual, which is a more comparable metric to the disarticulated fossil spine dataset, we find 48.0% calcifying colonization rate, 24.2% soft-bodied epizoan attachment, 26.6% non-colonized spines, 1.2% galled spines, and no observed bioerosion (Table 2; Figure 2). If we assume that spines colonized by soft-bodied epizoa in life would appear ‘non-altered’ when compared to the fossil record, the comparison then becomes 51.0% ‘non-altered’ spines in present-day Florida populations.
Table 2. Summary table of spine colonization and galling frequencies and epibiont burden scores compared across the studied living and fossil populations of the Florida, GBIF, and fossil datasets.
Disarticulated fossil Eucidaris spines (fossil dataset Figure 4) from the Pliocene Tamiami Formation of Florida exhibited a calcifying colonization rate of 7.9% and a bioerosion rate of 22.7%, while the remaining 69.3% exhibited no alteration or some abiotic abrasion or breakage. Disarticulated fossil spines from the Pliocene San Diego Formation of California exhibited a calcifying colonization rate of only 2.9% and a bioerosion rate of 11.6%, while the remaining 85.4% exhibited no alteration or only abiotic breakage (Table 2). All noted bioerosion was identified as likely polychaete traces (Figure 4B).
Figure 4. Fossil dataset Eucidaris spines from (A) the Pliocene Tamiami Formation of Florida from the FLMNH. First two spines on left from lot UF 314398 and remainder from lot UF 40867 and (B) The Pliocene San Diego Formation of California from the LACMNH (LACMIP 305.2192; 305.2194; 305.2197). Scale bar 1 cm.
Figure 5. Relationship between Florida dataset specimen size at ambitus and (A) proportion of spines that are colonized (including calcifying and non-calcifying epizoans) R2 = 0.154, p << 0.01, (B) proportion of spines that are colonized (calcifying epizoans only) R2 = 0.124, p << 0.01 (C) epibiont burden category with significant pairwise Wilcoxon rank-sum p-value p with Bonferroni adjustment (a = 0.05): (D) total primary spines per individual R2 = 0.563, p << 0.01.
Figure 6. Comparing galled and non-galled individuals from the Florida dataset in terms of (A) overall epibiont burden scores W = 955.5, p = 0.325, (B) proportion of colonized spines (both calcifying and non-calcifying) W = 1178.5, p = 0.495, (C) proportion of colonized spines (only calcifying) W = 1403.5, p = 0.025, and (D) ambital width of individual, W = 1397.5, p = 0.036.
The resampling efforts yield a spine calcifying colonization rate 95% confidence interval in Florida dataset present-day spines of 44.3-53.0%, a ‘non-altered’ spine (combining soft-bodied epizoan and non-colonized spines) rate of 46.0-55.4%, a galled spine rate of 0.2-2.5%, and a bioeroded spine rate of 0% (no bioeroded spines were found in the present-day population) (Table 2). Fossil spine calcifying colonization rate is significantly less than the resampled rate (95% confidence interval) in present-day Florida populations, and the proportion of non-altered fossil spines is significantly more.
Spine Galling – In present-day populations, galled spines were found in 20.9% of individuals in the Florida dataset (Table 2), in which each individual spine of each specimen was examined in detail. In contrast, less than 0.6% of individuals in the Atlantic and 0.3% of individuals in the Pacific exhibited any evidence of galling from the GBIF dataset. This is less likely a reflection of true galling frequency in these populations compared to the Florida shelf populations and more likely a consequence of incomplete observation of photographed specimens, since the oral side of individuals in life position was often obstructed from view. In the Florida dataset, 52 total galled spines were observed, and several individuals were observed to have more than one galled spine, with two individuals having the maximum observed seven galled spines. On multi-galled individuals, not all galls appeared to be active domiciles, as judged from the absence of at least one eulimid preserved attached near the gall. Inactive galls either appeared as simple swelling of the spine (Figure 2S), or as a partially broken swollen cavity which was sometimes secondarily covered by other spine epizoans (Figure 2P). Notably, not all active galls exhibited clear swelling (Figure 2U), and so could only be identified as galled due to the presence of the attached eulimid or obvious cavity at the attachment site. In these situations, a minute indent into the shaft of the spine is present at the attachment point of the eulimid proboscis, which has the potential to be identified in the fossil record, despite the lack of clear swelling. When binned by collection year (1964-1971 and 2008-2012) there was a notable but not statistically significant increase in frequency of galled individuals in the Florida dataset (15% to 21.1%, Supplementary Table S4).
Individuals that had at least one galled spine were larger than individuals with no galling (p = 0.036). These same larger individuals tended to have more spines relative to smaller specimens, and had proportionally more colonized spines relative to smaller individuals (p < 0.01, Figure 5). Present-day individuals on the Florida Shelf (Florida dataset) displayed no significant relationship between overall proportion of colonized spines and likelihood of having eulimid galling (Figure 6). However, when only calcifying colonization is considered, galled individuals had significantly higher levels of calcifying colonization than non-galled individuals (p = 0.025 Figure 6C). There was no significant relationship found between epibiont burden score and presence of galling (Figure 6A). Individuals collected between 2008 and 2012 displayed a statistically significantly higher proportion of colonized spines (74.2%) compared to those collected between 1964 and 1971 (64.7%). Supplementary Table S4 provides a summary of the spine-level data from the Florida dataset, grouped by collection years, with statistical significance determined by non-overlapping 95% confidence intervals. Notably, no galled spines were found in either fossil Pliocene population, an absence that had been noted previously by Petsios et al., 2023.
Epibiont Burden – Florida Shelf Eucidaris individuals (Florida dataset) that exhibited high and moderate levels of epibiont burden were generally larger, as measured by test width at the ambitus, than individuals with low to no epibiont burden (None to Low p < 0.01; None to Moderate p < 0.01; None to High p < 0.01; Low to Moderate p < 0.01; Low to High p = 0.049; Figure 5C), with a media difference of approximately 1.6 cm between burden category endmembers.
In Atlantic populations of the GBIF dataset, 39% of E. tribuloides individuals exhibited high epizoan burden, 37.3% of individuals moderate burden, 20.9% of individuals low burden, and 2.7% of individuals exhibited no visible epibionts in field photographs (Table 2). These rates are similar to the subset of E. tribuloides individuals scored from the Florida dataset, with 28.7% of individuals with high burden, 36.5% with moderate, 27.8% with low burden, and 7% with no evidence of epibionts. In the Pacific populations of the GBIF dataset, E. thouarsii and E. galapagensis individuals cumulatively exhibited 61.7% high epizoan burden, 28.5% moderate burden, 6.8% low burden, and 3.1% no burden (Table 2). Notably, Pacific populations overall exhibited a higher epibiont burden relative to their Atlantic counterparts.
Tumbling Experiments – Mass loss rate as a result of tumbling was calculated for each group over 152 hours (Figure 7). Initially, non-colonized and colonized spines experienced mass loss at the same rate (11.4 - 11.6 mg/hr), and galled spines at a much slower rate (3.3 - 4.0 mg/hr). After the first 80 hours of tumbling, non-calcifying epibionts on colonized spines began to degrade significantly, resulting in a large decrease in dry weight. Afterwards, colonized and non-colonized spines continued to exhibit the same rate of mass loss. When non-calcifying colonization was removed from spines, underlying bioerosion was observed in some cases. Overall, calcifying colonizers on spines and galls appear to be robust to abrasion from transport.
Figure 7. Exemplar spine specimens from the tumbling experiments, showing degree of abrasion and material loss over several hours of tumbling. (A) An example of a single spine colonized with calcifying serpulid worms over 152 hours of tumbling. (B) An example of a single spine colonized with non-calcifying sponge over 152 hours of tumbling. (C) The rate of mass loss over 152 hours of tumbling. Tumbling experiments were halted when spines resembled the typical taphonomic grade observed in the Pliocene fossil spines. Scale bar 1 cm.
Present-day populations of Eucidaris in the American tropics and subtropics exhibit higher levels of spine colonization and likely higher overall epizoan burden than their Pliocene ancestors on either side of Central America. This pattern persists after accounting for preferential erasure of evidence of non-calcifying epizoans from the fossil record by comparing only the proportion of calcifying colonization on spines between living and fossil populations. Differences in sample size between modern and fossil spine data was also considered, but do not wholly explain the observed differences either. Simulated transport using tumbling experiments have been previously used to elucidate the process of disarticulation and abiotic damage of echinoid skeletal material before it had the potential to enter the fossil record (Kidwell and Baumiller, 1990; Gorzelak and Salamon, 2013). However, the effects of transport on the preservation of evidence of epizoan colonization on echinoid spines is reported for the first time here. Not surprisingly, non-calcifying colonization does not survive long during the transportation process, leaving only evidence from calcifying and cementing epibionts to be preserved more reliably in the fossil record. We find no difference in mass loss due to transportation-related abrasion in non-colonized spines and those that have been colonized by calcifying organisms, suggesting also that colonizers do not protect disarticulated spines from damage. Surprisingly, galled spines had an even slower rate of mass loss due to transport than either the non-colonized or colonized spines. Galls, while skeletonized, represent malformed spine material that is potentially less stable than healthy spine cortex. Despite this, galled spines appeared to be more resilient to physical damage, potentially due to their smaller and rounder shapes decreasing relative surface area that could be damaged in transport.
Information that can be extracted from disarticulated fossil elements is, unsurprisingly, not directly comparable to how epibiont burden is observed and quantified in living populations. For one, the degree of epibiosis on whole individuals can never be known without fossils of whole, articulated spine-bearing populations (as in Schneider, 2003). As the skeletal material of regular echinoids typically disarticulates rapidly after death, whole fossils are rare in the fossil record (Greenstein, 1992, 1993). While whole E. tribuloides fossils are known from the Tamiami Formation (Osborn et al., 2020), these specimens are not plentiful enough for quantitative comparisons. Conversely, disarticulated spine material is common in the fossil record, but it is unclear how representative these fossil accumulations are of living populations. As echinoids shed spines during the normal growth process (Prouho, 1888), new spines form at the apical disc boundary as older spines migrate down and are shed at the oral plate boundary by dissolution of spine material at Prouho’s membrane (Cutress, 1965; Märkel and Röser, 1983b). Thus, a single individual may contribute multiple spines to the fossil record via shedding, and shed spines can be identified by the lack of the base below Prouho’s membrane (below the milled ring). Present day field tagging experiments have demonstrated that cidaroids do not preferentially shed fouled spines outside of the normal growth process (McPherson, 1968; Ebert, 1988). Spines may be shed if they are diseased or damaged (Cutress, 1965; Hendler, 1995), but this rate is unknown and not examined for galled spines. Several diseased and damaged spines were still attached to the test in the surveyed Florida populations. Some galls even exhibited secondary calcifying colonization, suggesting that galled spines are retained for some time by the living animal. Additionally, the majority of fossil spines from the fossil dataset still retained their complete base, suggesting that the disarticulated fossil spines were likely shed postmortem, rather than during the animals lifetime. We therefore expect the proportional representation of colonized, non-colonized, and galled spines to be comparable between living and fossil assemblages. Given this and the results of the experimental tumbling, it is likely that the fossil record of disarticulated spines is reliably recording the relative proportion of spines that have been colonized by calcifying organisms. This is further supported by the extensive known fossil record of encrustation of echinoid spines from other time periods (Schneider, 2003; Rashwan et al., 2024). This also suggests that the complete lack of fossilized spine galls is not a consequence of diminished preservation potential of the galled spine material but is a real ecological signal.
Assessments of the relationship between the number of colonized and galled spines per living individual and the individual’s overall epizoan burden allows for a comparative link to be made between modern populations and the fossil record. Spine-level data could not be directly compared between modern and fossil populations of the Pacific due to the rarity of available modern specimens. However, given the similarity between the epizoan burden scores of Florida shelf individuals and those that were scored using field photographs, we expect broader patterns in epizoan burden score to reflect the proportion of colonized spines in these present-day populations. We additionally demonstrate here the utility of georeferenced field digital images compiled in large online databases (such as GBIF.org) in exploring biotic associations in populations over a much larger region than what is feasible by individual field surveys alone. Large proportions of highly and moderately burdened individuals (~90%) in present-day Pacific populations is in stark contrast to low levels of evidence of spine bio-alteration in Pliocene San Diego Formation spines (less than 15% combined). This may reflect a relatively higher proportion of non-calcifying colonizers in Pacific populations compared to Atlantic populations, where non-calcifying epizoa are the sole colonizers on approximately 25% of spines. However, there is no reason to expect that there would be a significant difference in calcifying and non-calcifying colonization between the two oceans.
Galling parasitism in present-day Eucidaris populations has been reported extensively and was present on approximately a fifth of individuals (20.9%) from the Florida Shelf. In terms of individual spines, we observe a galling rate of only 1.2%. Despite this low rate, a galling rate of zero in fossil spines is still outside the 95% confidence intervals established when accounting sample size difference, if we assume galling rate has not changed. While more sampling of fossil Eucidaris spines may reveal fossil galled spines, it is worth considering the implications of a potentially true absence of fossil galls. Either a) the galling rate was much lower in the past, or b) galling is a newly evolved association between Eucidaris and the galling eulimid Sabinella. The genus Eucidaris originated in the Late Paleocene (Campbell, 1993; Maxwell, 2000) and is reliably present in the tropics of the Americas since at least the Pliocene if not the Miocene. Present-day eulimid gastropods are known to target specific echinoids. The genus Sabinella is known to parasitize other cidaroids such as Stylocidaris, Goniocidaris, and Ogmocidaris, but does not induce galling outside of its specialized symbiosis with Eucidaris (Warén, 1983). There is no known fossil record of Sabinella (Petsios et al., 2023), though Sabinella is listed as originating in the Pleistocene in Sepkoski’s Compendium (Sepkoski, 2002), which is the default first appearance assignment for extant genera. The lack of a fossil record for Sabinella is not all that surprising, as eulimids in general are small, thin-shelled, and difficult to differentiate using shell characteristics alone (Warén, 1983, but see González-Vallejo and León-González, 2018). Further systematic work is likely needed to establish if Sabinella truly lacks a fossil record, especially in the Pliocene of the American tropics. Warén (1992) proposed that the Pacific species S. shaskyi and the Atlantic species S. troglodytes likely underwent allopatric speciation similarly to their host eucidarid species associated with the closing of the CAS. This suggests that Sabinella was present and in association with these Eucidaris populations since at least before the Pliocene, and there is no reason to suspect that it was not engaging in galling parasitism at this time. Considering this, it is likely that galling of Eucidaris spines by Sabinella did likely occur in the Pliocene, but at a much lower rate, leading to a paucity of fossil evidence.
Establishing the timing of changes in pre-Modern levels of epibiosis on Eucidaris necessitates the use of the most recent fossil occurrences available in large enough numbers, i.e., the Pliocene of coastal North America. Though Eucidaris spines are known from the Pleistocene of both the western Pacific and eastern Atlantic, including the Waccamaw Formation of the Carolinas (Osborn et al., 2020), the Falmouth Formation of Jamaica (Donovan, 1993; Donovan and Portell, 2013; Gordon and Donovan, 1994), the Jaimanitas Formation of Cuba (FLMNH Collections), and the Abisinia Formation of Venezuela (FLMNH Collections), no large enough collections of Eucidaris spines have yet been recovered from these deposits to our knowledge. With this limitation, we are only able to establish that the increase of spine colonization in American Eucidaris populations likely occurred sometime between the Pliocene and the present-day. One event of note in this timeframe, the closing of the Central American Seaway (CAS), has induced significant oceanographic changes in the region and climatic changes globally since the Pliocene (O’Dea et al., 2016). Redirection of ocean currents and nutrient supplies between the once-connected Pacific Ocean and Caribbean Sea induced oligotrophic conditions on the Caribbean side of the Isthmus of Panamá (Jain and Collins, 2007), while the Pacific side developed nutrient-rich upwelling (Schmidt, 2007). This would have significantly and directly impacted the filter-feeding epizoans in these regions. The timing of the differentiation of water bodies associated with the gradual constriction, ultimate closure of the CAS, and subsequent formation of the landmass of the Isthmus is debated (O’Dea et al., 2016; Jaramillo et al., 2017; Molnar, 2017), with paleomagnetic evidence pointing to a Miocene or earlier initiation of tectonic uplift (Montes et al., 2012), paleontological and sedimentological data suggests a latest Pliocene closure sensu stricto (Keigwin, 1978; Duque-Caro, 1990; Kameo and Sato, 2000), and oceanographic geochemical interpretations suggesting a protracted process of water body differentiation spanning the time between (Steph et al., 2006; Kirillova et al., 2019; Öğretmen et al., 2020). Regardless, it is likely that the fossil eucidarid populations of the current study had already been impacted by nutrient supply changes brought about by the initial phases of constriction, and therefore the oceanographic changes associated with the closure of the CAS do not fully explain differences between epibiosis observed in these communities and those of the present-day. Additionally, given the development of nutrient-rich and nutrient-poor conditions, we would expect directionally opposite responses in populations on either side of the land bridge, which we do not observe. The more likely scenario is that the intensification of spine epibiosis observed in living populations is instead a result of some combination of the more recently introduced human-driven environmental changes that have occurred in the region since the Pliocene.
Nutrient influx combined with warming oceans are projected to increase the density of marine biofouling communities on biotic and abiotic substrates while diminishing their biodiversity (Dobretsov et al., 2019; Khosravi et al., 2019). Human-sourced runoff, toxic contamination, habitat degradation, and sea surface temperature warming in the studied regions (Benitez et al., 2014; Páez-Osuna et al., 2016; Gómez et al., 2022) have likely already impacted the epibiont communities. Additionally, parasite-host associations have been and are projected to continue to be complexly impacted by human activity (Kelly et al., 2010; Sonnenholzner et al., 2011; Khan, 1990; Budria and Candolin, 2014; Huntley and Scarponi, 2021). Sonnenholzner et al. (2011) report on a decrease of parasitic eulimids on E. thouarsii in the Galapágos induced by a proliferation of the commensal crab Mithrax nodosus (currently Mithraculus nodosus), in turn brought about by anthropogenic overfishing of its own predators. The present study, however, suggests that proliferation of eulimid parasitism can also be expected, though potentially via a different mechanism. McPherson (1968) reported a 2% parasitic galling rate in E. tribuloides populations of the Florida shelf between 1965 and 1966, while the present study found 21% of total individuals galled in the same populations collected between 1964 and 2011 (with a notable but non-significant increase in galling rates across this period). Earlier studies on spine fouling on living Eucidaris do not report on the per spine rate or overall intensity of fouling as we do here, but given the significant relationship between presence of galling and calcifying epibiosis in individuals, we can assume that the two are closely associated. Further work is likely needed to elucidate whether the proliferation of galling parasitism and spine fouling in present-day populations is related to trophic web disturbances similar to those reported by Sonnenholzner et al. (2011), or if these trends are the result of some other anthropogenic perturbation.
The detailed life histories of epizoans on cidaroid echinoid spines remain largely unknown. It is not fully understood how dependent and host-specific many of these epibionts are on the substrate provided by their echinoid hosts, how they are impacted after the host dies, how they interact with both the host and each other, or the specific magnitude and direction of changes in these relationships caused by human-induced environmental shifts. In this study, we provide new insights into these questions across a broad region and over geological timescales by demonstrating that living populations of the cidaroid Eucidaris in the tropics and subtropics of the Americas exhibited higher frequencies of colonization and parasitic galling than did their late Neogene direct ancestors. Whereas taphonomic processes inevitably result in loss of non-calcifying epizoa, experimental work and taphonomic patterns in the fossil record both suggest that galled spines and calcifying epibionts are unlikely to have been lost preferentially relative to spines unaffected by epibiosis. A high proportion of individuals in present-day populations across a wide region are heavily colonized. This degree of epizoan burden and galling parasitism may be detrimental to fitness, but further studies, specifically detailed aquaria and field surveys, are needed to demonstrate whether this is indeed the case. Increasing fouling density has been linked to some anthropogenic impacts, namely warming ocean temperatures, habitat pollution, and tropic web disturbances that may explain the present-day increase of epizoan burden and parasitism. With historical and current rates of eucidarid spine colonization established, further research is needed to determine what drove this recent increase in epibiosis and how future populations may respond.
The original contributions presented in the study are included in the article/Supplementary Material. Further inquiries can be directed to the corresponding author/s.
The manuscript presents research on animals that do not require ethical approval for their study.
EP: Conceptualization, Investigation, Methodology, Writing – original draft, Visualization. CF: Validation, Writing – review & editing. MK: Funding acquisition, Validation, Writing – review & editing, Conceptualization. PL: Resources, Validation, Writing – review & editing. RP: Resources, Validation, Writing – review & editing, Conceptualization. CT: Validation, Writing – review & editing, Conceptualization, Investigation, Resources.
The author(s) declare that financial support was received for the research and/or publication of this article. This work was partially funded by a National Science Foundation grant to C.L.T. and M.K. (EAR SGP-1630475 and EAR SGP-1630276).
The authors thank Gordon Hendler and Austin Hendy for facilitating access to the zoological collections and invertebrate paleontology collections of the Los Angeles County Natural History Museum, respectively. Janessa Fletcher kindly allowed access to the SEAMAP data logs and collection information.
The authors declare that the research was conducted in the absence of any commercial or financial relationships that could be construed as a potential conflict of interest.
The author(s) declare that no Generative AI was used in the creation of this manuscript.
All claims expressed in this article are solely those of the authors and do not necessarily represent those of their affiliated organizations, or those of the publisher, the editors and the reviewers. Any product that may be evaluated in this article, or claim that may be made by its manufacturer, is not guaranteed or endorsed by the publisher.
The Supplementary Material for this article can be found online at: https://www.frontiersin.org/articles/10.3389/fmars.2025.1513138/full#supplementary-material
Supplementary Figure 1 | Example specimens of Eucidaris occurrences reported on GBIF, showing epizoan burden categories of Table 1. (A) no epizoans (E. tribuloides, photo no. 2429255555, photo credit Logan Crees), (B) low burden (E. tribuloides, photo no. 4102891523, photo credit jesisly), (C) moderate burden (E. thouarsii, photo no. 2557811853, photo credit sandor_in), and (D) high burden (E. thouarsii, photo no. 1453362600, photo credit Robin Gwen Agarwal).
Supplementary Table 1 | Combined dataset of fossil, field-photographed specimens (GBIF), and Florida shelf specimens (FWC).
Supplementary Table 2 | List of non-motile attached epibionts identified from the Florida dataset (FWC collections), and catalog numbers of exemplar specimens.
Supplementary Table 3 | Citation list for occurrences and mediafiles downloaded from GBIF.org, accessed August, 2024.
Supplementary Table 4 | Spine colonization and individual galling frequencies as calculated from the Florida dataset when individuals were binned into time intervals based on the year of collection, 1964 to 1971 and 2008 to 2012.
Agassiz L., Desor P. J. E. (1846). Catalogue raisonné des familles, des genres, et des espèces de la classe des échinodermes. Annales des Sciences Naturelles, Troisième Série. Zoologie 6, 305–374.
Arnold R. (1908). Descriptions of new Cretaceous and Tertiary fossils from the Santa Cruz Mountains, California. Proc. United States Natl. Museum 34, 345–389. doi: 10.5479/si.00963801.34-1617.345
Barnosky A. D., Hadly E. A., Gonzalez P., Head J., Polly P. D., Lawing A. M., et al. (2017). Merging paleobiology with conservation biology to guide the future of terrestrial ecosystems. Science 355 (6325), eaah4787.
Barnosky E. H. (1994). Ecosystem dynamics through the past 2000 years as revealed by fossil mammals from Lamar Cave in Yellowstone National Park, USA. Historic. Biol. 8, 71–90. doi: 10.1080/10292389409380472
Benitez J., Cerón-Bretón R., Cerón-Bretón J., Rendón-Von-Osten J. (2014). The environmental impact of human activities on the Mexican coast of the Gulf of Mexico: review of status and trends. WIT Trans. Ecol. Environ. 181, 37–50 doi: 10.2495/EID140041.
Brusca R. C. (1973). A Handbook to the Common Intertidal Invertebrates of the Gulf of California (Tucson: The University of Arizona Press).
Brusca R. C. (1980). Common Intertidal Invertebrates of the Gulf of California. 2nd ed. (Tucson: The University of Arizona Press).
Budria A., Candolin U. (2014). How does human-induced environmental change influence host-parasite interactions? Parasitology 141, 462–474. doi: 10.1017/S0031182013001881
Burney D. A., James H. F., Burney L. P., Olson S. L., Kikuchi W., Wagner W. L., et al. (2001). Fossil evidence for a diverse biota from Kaua’i and its transformation since human arrival. Ecol. Monogr. 71, 615–641. doi: 10.1890/0012-9615(2001)071
Campbell L. D. (1993). Pliocene molluscs from the Yorktown and Chowan river formations in Virginia. Virginia Div. Min. Resour. 127, 1–259.
Cintra-Buenrostro C. E., Flessa K. W., Guillermo A. S. (2005). Who cares about a vanishing clam? Trophic importance of Mulinia coloradoensis inferred from predatory damage. Palaios 20, 296–302. doi: 10.2110/palo.2004.p04-21
Cutress B. M. (1965). Observations on growth in Eucidaris tribuloides (Lamarck), with special reference to the origin of the oral primary spines. Bull. Mar. Sci. 15, 797–834.
Cutress B. M. (1980). Cretaceous and tertiary cidaroida (Echinodermata: echinoidea) of the Caribbean area. Bullet. Am. Paleontol. 77, 221.
Dartevelle E. (1953). Les Échinides fossiles du Congo et d’Angola. Part 2: Description systématique des échinides fossiles du Congo et de l’Angola. Annales du Musée R. du Congo Belge Tervuren Belgium Sci. Géol. 13, 1–240.
David B., Stock S. R., De Carlo F., Hétérier V., De Ridder C. (2009). Microstructures of Antarctic cidaroid spines: diversity of shapes and ectosymbiont attachments. Mar. Biol. 156, 1559–1572. doi: 10.1007/s00227-009-1192-3
Dery A., Collard M., Dubois P. (2017). Ocean acidification reduces spine mechanical strength in euechinoid but not in cidaroid sea urchins. Environ. Sci. Technol. 51, 3640–3648. doi: 10.1021/acs.est.6b05138
Dery A., Guibourt V., Catarino A. I., Compère P., Dubois P. (2014). Properties, morphogenesis, and effect of acidification on spines of the cidaroid sea urchin Phyllacanthus imperialis. Invertebr. Biol. 133, 188–199. doi: 10.1111/ivb.2014.133.issue-2
Dietl G. P., Durham S. R., Clark C., Prado R. (2023). Better together: Building an engaged conservation paleobiology science for the future. Ecol. Sol. Evidence 4, e12246. doi: 10.1002/2688-8319.12246
Dietl G. P., Flessa K. W. (2011). Conservation paleobiology: putting the dead to work. Trends Ecol. Evol. 26, 30–37. doi: 10.1016/j.tree.2010.09.010
Dillon E. M., Pier J. Q., Smith J. A., Raja N. B., Dimitrijević D., Austin E. L., et al. (2022). What is conservation paleobiology? Tracking 20 years of research and development. Front. Ecol. Evol. 10, 1031483. doi: 10.3389/fevo.2022.1031483
Dobretsov S., Coutinho R., Rittschof D., Salta M., Ragazzola F., Hellio C. (2019). The oceans are changing: impact of ocean warming and acidification on biofouling communities. Biofouling 35, 585–595. doi: 10.1080/08927014.2019.1624727
Döderlein L. (1887). “I. Theil. Fammilie Cidaridae und Saleniidae. Stuttgart,” in Die Japanischen seeigel (Stuttgart, Germany: Schweizerbartsche Verlagsbuchhandlung).
Donovan S. K., Dixon H. L., Pickerill R. K., Doyle E. N. (1994). Pleistocene echinoid (Echinodermata) fauna from southeast Jamaica. J. Paleontol. 68, 351–358. doi: 10.1017/S0022336000022939
Donovan S. K., Embden B. J. (1996). Early pleistocene echinoids of the manchioneal formation, Jamaica. J. Paleontol. 70, 485–493. doi: 10.1017/S0022336000038415
Donovan S. K., Gordon C. M. (1993). Echinoid taphonomy and the fossil record: supporting evidence from the Plio-Pleistocene of the Caribbean. Palaios 8, 304–306. doi: 10.2307/3515152
Donovan S. K., Lewis ,. D. N. (2009). Paleontological implications of multiple genital pores in the apical system of Eucidaris tribuloides (Lamarck), Recent of Jamaica. Caribbean J. Sci. 45, 20–24. doi: 10.18475/cjos.v45i1.a5
Donovan S. K., Portell R. W. (2013). Fossil echinoids from the upper Pliocene Hopegate Formation of north central Jamaica. Caribbean J. Sci. 47, 125–139. doi: 10.18475/cjos.v47i3.a2
Duque-Caro H. (1990). Neogene stratigraphy, paleoceanography and paleobiogeography in northwest South America and the evolution of the Panama Seaway. Palaeogeogr. Palaeoclimatol. Palaeoecol. 77, 203–234. doi: 10.1016/0031-0182(90)90178-A
Ebert T. A. (1988). Growth, regeneration, and damage repair of spines of the slate-pencil sea urchin Heterocentrotus mammillatus (L.) (Echinodermata: Echinoidea). Pacific Sci. 42, 160–172.
Farrar L. E., Graves E., Petsios E., Portell R. W., Grun T. B., Kowalewski M., et al. (2020). Characterization of traces of predation and parasitism on fossil echinoids. Palaios 35, 215–227. doi: 10.2110/palo.2019.088
Ferreira O. (1961). Equinídeos do Miocénico de Portugal continental e Ilhas Adjacentes. Comunicações dos Serviços Geológicos Portugal 45, 529–564.
Finnegan S., Anderson S. C., Harnik P. G., Simpson C., Tittensor D. P., Byrnes J. E., et al. (2015). Paleontological baselines for evaluating extinction risk in the modern oceans. Science 348, 567–570. doi: 10.1126/science.aaa6635
Finnegan S., Harnik P. G., Lockwood R., Lotze H. K., McClenachan L., Kahanamoku S. S. (2024). Using the fossil record to understand extinction risk and inform marine conservation in a changing world. Annu. Rev. Mar. Sci. 16, 307–333. doi: 10.1146/annurev-marine-021723-095235
Gómez I., Silva R., Lithgow D., Rodríguez J., Banaszak A. T., van Tussenbroek B. (2022). A review of disturbances to the ecosystems of the Mexican Caribbean, their causes and consequences. J. Mar. Sci. Eng. 10, 644. doi: 10.3390/jmse10050644
González M. J. V., Borrero-Pérez G. H. (2020). First records and new information on the associations of echinoderms with other phyla in the rocky reefs of northern Chocó, Colombian Pacific. ZooKeys 921, 1.
González-Vallejo N. E., León-González J. Á. (2018). New ecological and taxonomic remarks on Sabinella troglodytes and Nanobalcis worsfoldi (Gastropoda: Eulimidae) living on the “slate-pencil sea urchin” from the Mexican Caribbean region. Rev. mexicana biodiversidad 89, 123–133. doi: 10.22201/ib.20078706e.2018.1.2185
Gordon C. M., Donovan S. K. (1992). Disarticulated echinoid ossicles in paleoecology and taphonomy: the last interglacial Falmouth Formation of Jamaica. Palaios 7, 157–166. doi: 10.2307/3514926
Gordon C. M., Donovan S. K. (1994). Some fossil echinoids (Echinodermata) from the Neogene of St. Croix, US Virgin Islands. Caribbean J. Sci. 30, 69–75.
Gorzelak P., Salamon M. A. (2013). Experimental tumbling of echinoderms—taphonomic patterns and implications. Palaeogeogr. Palaeoclimatol. Palaeoecol. 386, 569–574. doi: 10.1016/j.palaeo.2013.06.023
Greenstein B. J. (1992). Taphonomic bias and the evolutionary history of the family Cidaridae (Echinodermata: Echinoidea). Paleobiology 18, 50–79. doi: 10.1017/S0094837300012215
Greenstein B. J. (1993). Is the fossil record of regular echinoids really so poor? A comparison of living and subfossil assemblages. Palaios 8, 587–601. doi: 10.2307/3515034
Grun T. B., Kroh A., Nebelsick J. H. (2017). Comparative drilling predation on time-averaged phosphatized and nonphosphatized assemblages of the minute clypeasteroid echinoid Echinocyamus stellatus from Miocene offshore sediments (Globigerina Limestone Formation, Malta). J. Paleontol. 91, 633–642. doi: 10.1017/jpa.2016.123
Gutt J., Schickan T. (1998). Epibiotic relationships in the Antarctic benthos. Antarctic Sci. 10, 398–405. doi: 10.1017/S0954102098000480
Hendler G. (1995). Sea Stars, Sea Urchins, and Allies: Echinoderms of Florida and the Caribbean (Washington and London: Smithsonian Institution Press).
Hertlien L. G., Grant U. S. (1944). The geology and paleontology of the marine pliocene of San Diego, California. Memoirs San Diego Soc. Natural History 2, 1–72.
Hétérier V., David B., De Ridder C., Rigaud T. (2008). Ectosymbiosis is a critical factor in the local benthic biodiversity of the Antarctic deep sea. Mar. Ecol. Prog. Ser. 364, 67–76. doi: 10.3354/meps07487
Hétérier V., De Ridder C., David B., Rigaud T. (2004). “Comparative biodiversity of ectosymbionts in two Antarctic cidarid echinoids, Ctenocidaris spinosa and Rhynchocidaris triplopora,” in Echinoderms (Balkema, Rotterdam).
Hopkins T., Thompson L., Walker J., Davis M. (2004). “A study of epibiont distribution on the spines of the cidaroid sea urchin, Eucidaris tribuloides (Lamarck 1816) from the shallow shelf of the eastern Gulf of Mexico,” in Echinoderms München (Taylor and Francis, London).
Huntley J. W., Fürsich F. T., Alberti M., Hethke M., Liu C. (2014). A complete Holocene record of trematode–bivalve infection and implications for the response of parasitism to climate change. Proc. Natl. Acad. Sci. 111, 18150–18155. doi: 10.1073/pnas.1416747111
Huntley J. W., Scarponi D. (2021). Parasitism and host behavior in the context of a changing environment: The Holocene record of the commercially important bivalve Chamelea gallina, northern Italy. PloS One 16, e0247790. doi: 10.1371/journal.pone.0247790
Jackson J. B., Kirby M. X., Berger W. H., Bjorndal K. A., Botsford L. W., Bourque B. J., et al. (2001). Historical overfishing and the recent collapse of coastal ecosystems. Science 293 (5530), 629–637. doi: 10.1126/science.1059199
Jackson J. B., O’Dea A. (2013). Timing of the oceanographic and biological isolation of the Caribbean Sea from the tropical eastern Pacific Ocean. Bull. Mar. Sci. 89, 779–800. doi: 10.5343/bms.2012.1096
Jain S., Collins L. S. (2007). Trends in Caribbean paleoproductivity related to the Neogene closure of the Central American Seaway. Mar. Micropaleontol. 63, 57–74. doi: 10.1016/j.marmicro.2006.11.003
Jangoux M. (1987). Diseases of echinodermata II. Agents metazoans (Mesozoa to bryozoa). Dis. Aquat. Org. 2, 205–234.
Jaramillo C., Montes C., Cardona A., Silvestro D., Antonelli A., Bacon C. D. (2017). Comment (1) on “Formation of the Isthmus of Panama” by O’Dea et al. Sci. Adv. 3, e1602321. doi: 10.1126/sciadv.1602321
Kameo K., Sato T. (2000). Biogeography of Neogene calcareous nannofossils in the Caribbean and the eastern equatorial Pacific—floral response to the emergence of the Isthmus of Panama. Mar. Micropaleontol. 39, 201–218. doi: 10.1016/S0377-8398(00)00021-9
Keigwin L. D. (1978). Pliocene closing of the Isthmus of Panama, based on biostratigraphic evidence from nearby Pacific Ocean and Caribbean Sea cores. Geology 6, 630–634. doi: 10.1130/0091-7613(1978)6<630:PCOTIO>2.0.CO;2
Kelly D. W., Poulin R., Tompkins D. M., Townsend C. R. (2010). Synergistic effects of glyphosate formulation and parasite infection on fish malformations and survival. J. Appl. Ecol. 47, 498–504. doi: 10.1111/j.1365-2664.2010.01791.x
Khan R. (1990). Parasitism in marine fish after chronic exposure to petroleum hydrocarbons in the laboratory and to the Exxon Valdez oil spill. Bulletin of Environmental Contamination and Toxicology 44, 759–763.
Khosravi M., Nasrolahi A., Shokri M. R., Dobretsov S., Pansch C. (2019). Impact of warming on biofouling communities in the northern Persian Gulf. J. Thermal Biol. 85, 102403. doi: 10.1016/j.jtherbio.2019.102403
Kidwell S. M. (2015). Biology in the Anthropocene: challenges and insights from young fossil records. Proc. Natl. Acad. Sci. 112, 4922–4929. doi: 10.1073/pnas.1403660112
Kidwell S. M. (2007). Discordance between living and death assemblages as evidence for anthropogenic ecological change. Proc. Natl. Acad. Sci. 104 (45), 17701–17706.
Kidwell S. M., Baumiller T. (1990). Experimental disintegration of regular echinoids: roles of temperature, oxygen, and decay thresholds. Paleobiology 16, 247–271. doi: 10.1017/S0094837300009982
Kier P. M., Grant R. E. (1965). Echinoid distribution and habits, key largo coral reef preserve, florida Vol. 149 (Smithsonian Miscellaneous Collections), 1–68.
Kirillova V., Osborne A. H., Störling T., Frank M. (2019). Miocene restriction of the Pacific-North Atlantic throughflow strengthened Atlantic overturning circulation. Nat. Commun. 10, 4025. doi: 10.1038/s41467-019-12034-7
Kowalewski M., Avila Serrano G. E., Flessa K. W., Goodfriend G. A. (2000). Dead delta’s former productivity: Two trillion shells at the mouth of the Colorado River. Geology 28, 1059–1062. doi: 10.1130/0091-7613(2000)28<1059:DDFPTT>2.0.CO;2
Kowalewski M., Nawrot R., Scarponi D., Tomašových A., Zuschin M. (2023). Marine conservation palaeobiology: What does the late Quaternary fossil record tell us about modern-day extinctions and biodiversity threats? Cambridge Prisms: Extinct. 1, 1–19, e24. doi: 10.1017/ext.2023.22
Kowalewski M., Nebelsick J. H. (2003). “Predation and parasitism on recent and fossil echinoids,” in Predator-Prey Interactions in the Fossil Record. Eds. Kelley P. H., Kowalewski M., Hansen T. A. (Kluwer Academic and Plenum Publishers, New York), 279–302.
Kowalewski M., Wittmer J. M., Dexter T. A., Amorosi A., Scarponi D. (2015). Differential responses of marine communities to natural and anthropogenic changes. Proc. R. Soc. B 282, 20142990. doi: 10.1098/rspb.2014.2990
Kumar R., Kumari S., Malika A., Sharma A. P., Dahms H. U. (2022). Protistan epibionts affect prey selectivity patterns and vulnerability to predation in a cyclopoid copepod. Sci. Rep. 12, 22631. doi: 10.1038/s41598-022-26004-5
Lessios H. A. (2005). Echinoids of the Pacific waters of Panama: status of knowledge and new records. Rev. Biol. Trop. 3, 147–170.
Lessios H. A., Kessing B., Robertson D. R., Paulay G. (1999). Phylogeography of the pantropical sea urchin Eucidaris in relation to land barriers and ocean currents. Evolution 53, 806–817. doi: 10.2307/2640720
Lindberg D., Roth B., Kellogg M., Hubbs C., Power D. (1980). “Invertebrate megafossils of Pleistocene (Sangamon interglacial) age from Isla de Guadalupe, Baja California, Mexico,” in The California Islands: Proceedings of a Multidisciplinary Symposium (Santa Barbara Museum of Natural History, Santa Barbara).
Linse K., Walker L. J., Barnes D. K. (2008). Biodiversity of echinoids and their epibionts around the Scotia Arc, Antarctica. Antarctic Sci. 20, 227–244. doi: 10.1017/S0954102008001181
Lyell C. (1845). On the miocene tertiary strata of Maryland, Virginia, and of North and South Carolina. Q. J. Geol. Soc. London 1, 427–429. doi: 10.1144/GSL.JGS.1845.001.01.90
Madeira P., Kroh A., Cordeiro R., Meireles R., Avila S. P. (2011). The fossil echinoids of Santa Maria Island, Azores (Northern Atlantic Ocean). Acta Geol. Polonica 61, 243–264.
Maloney N. J., Macsotay O. (1968). Geology of La Tortuga Island, Venezuela. Assoc. Venezuela Geol. Mineria Petréleo 10, 265–287.
Märkel K., Röser U. (1983a). The spine tissues in the echinoid Eucidaris tribuloides. Zoomorphology 103, 25–41. doi: 10.1007/BF00312056
Märkel K., Röser U. (1983b). Calcite-resorption in the spine of the echinoid Eucidaris tribuloides. Zoomorphology 103, 43–58. doi: 10.1007/BF00312057
Maxwell P. A. (2000). Early Paleogene climates in the southwest Pacific: evidence from marine Mollusca and other invertebrates. GFF 122, 104–105. doi: 10.1080/11035890001221104
Mayer-Emar K. (1864). “Systematisches Verzeichnis der fossilen Reste von Madeira, Porto-Santo und Santa Maria nebst Beschreibung der neuen Arten,” in Geologische Beschreibung der Inseln Madeira und Porto Santo. Ed. Hartung G. (Wilhelm Engelmann, Leipzig).
Mayr E. (1954). “Change of genetic environment and evolution,” in Evolution as a Process. Eds. Huxley J., Hardy A. C. (Allen and Unwin, London), 157–180.
McKenzie J., Grigolava I. (1996). The echinoderm surface and its role in preventing microfouling. Biofouling 10, 261–272. doi: 10.1080/08927019609386285
McPherson B. (1968). Contributions to the biology of the sea urchin Eucidaris tribuloides (Lamarck). Bull. Mar. Sci. 18, 400–443.
Molnar P. (2017). Comment (2) on “Formation of the Isthmus of Panama” by O’Dea et al. Sci. Adv. 3, e1602320. doi: 10.1126/sciadv.1602320
Montes C., Cardona A., McFadden R., Morón S., Silva C., Restrepo-Moreno S., et al. (2012). Evidence for middle Eocene and younger land emergence in central Panama: Implications for Isthmus closure. Geol. Soc. America Bull. 124, 780–799. doi: 10.1130/B30528.1
Mortensen T. (1928). A Monograph of the Echinoidea. 1 (Cidaroidea. Copenhagen and London: C. A. Reitzel and Oxford University Press).
O’Dea A., Lessios H. A., Coates A. G., Eytan R. I., Restrepo-Moreno S. A., Cione A. L., et al. (2016). Formation of the Isthmus of Panama. Sci. Adv. 2, e1600883. doi: 10.1126/sciadv.1600883
Öğretmen N., Schiebel R., Jochum K., Stoll B., Weis U., Repschläger J., et al. (2020). Deep thermohaline circulation across the closure of the Central American Seaway. Paleoceanogr. Paleoclimatol. 35, e2020PA004049. doi: 10.1029/2020PA004049
Osborn A. S., Portell R. W., Mooi R. (2020). Neogene echinoids of Florida. Bull. Florida Museum Natural History 57, 237–469. doi: 10.58782/flmnh.gbwl4736
Páez-Osuna F., Sanchez-Cabeza J., Ruiz-Fernández A., Alonso-Rodríguez R., Piñón-Gimate A., Cardoso-Mohedano J., et al. (2016). Environmental status of the Gulf of California: A review of responses to climate change and climate variability. Earth-Science Rev. 162, 253–268. doi: 10.1016/j.earscirev.2016.09.015
Petsios E., Farrar L., Tennakoon S., Jamal F., Portell R. W., Kowalewski M., et al. (2023). The Ecology of Biotic Interactions in Echinoids: Modern Insights into Ancient Interactions (Cambridge: Cambridge University Press).
Phelan T. (1970). A field guide to the cidaroid echinoids of the northwestern Atlantic Ocean, Gulf of Mexico, and the Caribbean Sea. Smithsonian Contrib. to Zool. 40, 1–67. doi: 10.5479/si.00810282.40
Portell R. W., Oyen C. W. (1997). Occurrence of the regular urchin Eucidaris tribuloides from the Tamiami Formation (Pliocene) of Florida. Tulane Stud. Geol. Paleontol. 30, 99–104.
Prouho H. (1888). Recherches sur le Dorocidaris papillata. Arch. zool. expérimentale générale 5, 213.
Queiroz V., Neves E., Sales L., Johnsson R. (2017). The gall-former Sabinella troglodytes (Caenogastropoda: Eulimidae) and its association with Eucidaris tribuloides (Echinodermata: Echinoidea). J. Conchol. 42, 371–377.
Rashwan M., Mandor M., El Hedeny M., El-Sabbagh A., Vinn O., Alkahtane A., et al. (2024). Taphonomy of the Middle Miocene regular echinoid spines from Cairo-Suez district, Egypt: palaeoecological and palaeoenvironmental interpretations. Palaeoworld. pp. 1697–1709. doi: 10.1016/j.palwor.2024.04.002
Rick T. C., Lockwood R. (2013). Integrating paleobiology, archeology, and history to inform biological conservation. Conserv. Biol. 27 (1), 45–54.
Rothpletz A., Simonelli V. (1890). Die marinen Ablagenmgen aug Gran Canaria: Zeitsch. Deutsche Geol. Gesellschaft 42, 677–736.
Ruiz-Nava M.-P., Conejeros-Vargas C.-A., Solís-Marín F.-A. (2021). Redescription of the sea urchin Eucidaris thouarsii (Cidaroida: Cidaridae) based on material from the Mexican Pacific. Rev. Biol. Trop. 69, 438–451. doi: 10.15517/rbt.v69iSuppl.1.46383
Salazar-Vallejo S. I., López-Muraira I. G. (1983). Estudio preliminar sobre la epifauna de Hesperocidaris asteriscus (Echinodermata: Echinoidea). Cienc. Marinas 9, 109–119. doi: 10.7773/cm.v9i2.421
Sarkar S. K. R. K. U., Das B. K. (2021). Record of epibiont ciliates (Ciliophora: Peritrichia) living on freshwater invertebrates in a floodplain wetland. J. Inland Fish. Soc India 53, 210–214. doi: 10.47780/jifsi.53.3-4.2021.122371
Scarponi D., Azzarone M., Kowalewski M., Huntley J. W. (2017). Surges in trematode prevalence linked to centennial-scale flooding events in the Adriatic. Sci. Rep. 7, 5732. doi: 10.1038/s41598-017-05979-6
Schmidt D. (2007). “The closure history of the Central American seaway: evidence from isotopes and fossils to models and molecules,” in Deep-Time Perspectives on Climate Change: Marrying the Signal from Computer Models and Biological Proxies. Eds. Williams M., Haywood A. M., Gregory F. J., Schmidt D. N. (London, U.K: Bath: The Geological Society Publishing House), 427–442.
Schneider C. L. (2003). Hitchhiking on Pennsylvanian echinoids: epibionts on Archaeocidaris. Palaios 18, 435–444. doi: 10.1669/0883-1351(2003)018<0435:HOPEEO>2.0.CO;2
Schneider C. L., Harris L., Boetger S., Walker C., Lesser M. (2010). “Epibionts on late Carboniferous through early Permian echinoid spines from Texas, USA,” in Echinoderms: Proceedings of the 12th International Echinoderm Conference, 7–11 August 2006. Eds. Harris L. G., Böttger S. A., Walker C. W., Lesser M. P. (USA. Durham, CRC Press, Durham, New Hampshire), 71–76.
Sepkoski J. J. (2002). A compendium of fossil marine animal genera. Bullet. Am. Paleontol. 363, 1–560.
Smith J. A., Dietl G. P. (2016). The value of geohistorical data in identifying a recent human-induced range expansion of a predatory gastropod in the Colorado River delta, Mexico. J. Biogeogr. 43, 791–800. doi: 10.1111/jbi.2016.43.issue-4
Sonnenholzner J. I., Lafferty K. D., Ladah L. B. (2011). Food webs and fishing affect parasitism of the sea urchin Eucidaris galapagensis in the Galápagos. Ecology 92, 2276–2284. doi: 10.1890/11-0559.1
Steinbeck J., Ricketts E. F. (1941). Sea of Cortez: A Leisurely Journal of Travel and Research (New York: Viking Press).
Steneck R. S. (2013). Sea urchins as drivers of shallow benthic marine community structure. Develop. Aquacult. Fish. Sci. 38, 195–212. doi: 10.1016/B978-0-12-396491-5.00014-9
Steph S., Tiedemann R., Prange M., Groeneveld J., Nürnberg D., Reuning L., et al. (2006). Changes in Caribbean surface hydrography during the Pliocene shoaling of the Central American Seaway. Paleoceanography 21, pp. 1–25. doi: 10.1029/2004PA001092
Thiele J. (1925). “Gastropoda der Deutschen Tiefsee-Expedition,” in Wissenschaftliche Ergebnisse der Deutschen Tiefsee-Expedition auf dem Dampfer “Valdivia”, vol. 2 . Ed. Fischer G. (Gustav Fischer, Jena, Berlin).
Tyler C. L., Schneider C. L. (2018). An overview of conservation paleobiology. Mar. Conserv. Paleobiol. 47, 1–10. doi: 10.1007/978-3-319-73795-9
Vedder J. G., Moore E. J. (1976). Paleoenvironmental implications of fossiliferous Miocene and Pliocene strata on San Clemente Island, California, in Howell D. G., ed., Aspects of the geologic history of the California Continental Borderland. Am. Assoc. Petrol. Geol. Pacific Sect. Miscellaneous Publ. 24, 107–135.
Warén A. (1983). A generic revision of the family Eulimidae (Gastropoda, Prosobranchia). J. Molluscan Stud. 49, 1–96. doi: 10.1093/mollus/49.Supplement_13.1
Warén A. (1992). Comments on and descriptions of eulimid gastropods from tropical west America. Veliger 35, 177–194.
Keywords: Echinoids, biotic interaction, conservation paleobiology, epibiosis, symbiosis, parasitism
Citation: Petsios E, Fuchs CE, Kowalewski M, Larson P, Portell RW and Tyler CL (2025) Quaternary intensification of spine epibiosis in the cidaroid echinoid Eucidaris: implications for anthropogenic impacts. Front. Mar. Sci. 12:1513138. doi: 10.3389/fmars.2025.1513138
Received: 17 October 2024; Accepted: 28 February 2025;
Published: 07 April 2025.
Edited by:
Sally Walker, University of Georgia, United StatesReviewed by:
Chiara Lombardi, Energy and Sustainable Economic Development (ENEA), ItalyCopyright © 2025 Petsios, Fuchs, Kowalewski, Larson, Portell and Tyler. This is an open-access article distributed under the terms of the Creative Commons Attribution License (CC BY). The use, distribution or reproduction in other forums is permitted, provided the original author(s) and the copyright owner(s) are credited and that the original publication in this journal is cited, in accordance with accepted academic practice. No use, distribution or reproduction is permitted which does not comply with these terms.
*Correspondence: Elizabeth Petsios, ZWxpemFiZXRoX3BldHNpb3NAYmF5bG9yLmVkdQ==
†Present address: Paul Larson, Washington State Department of Ecology, Lacey, WA, United States
Disclaimer: All claims expressed in this article are solely those of the authors and do not necessarily represent those of their affiliated organizations, or those of the publisher, the editors and the reviewers. Any product that may be evaluated in this article or claim that may be made by its manufacturer is not guaranteed or endorsed by the publisher.
Research integrity at Frontiers
Learn more about the work of our research integrity team to safeguard the quality of each article we publish.