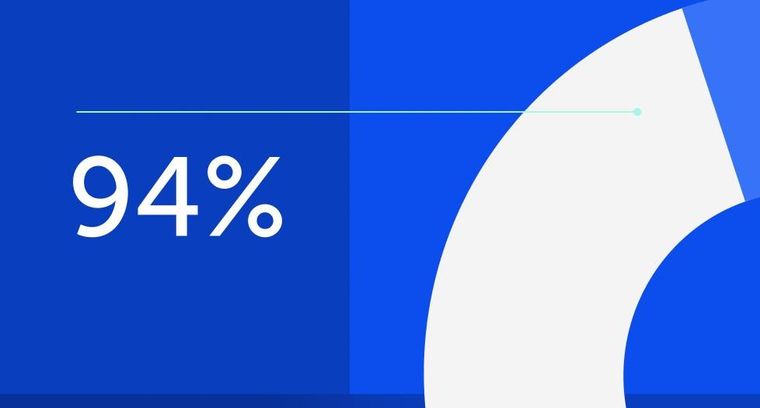
94% of researchers rate our articles as excellent or good
Learn more about the work of our research integrity team to safeguard the quality of each article we publish.
Find out more
ORIGINAL RESEARCH article
Front. Mar. Sci., 28 March 2025
Sec. Marine Fisheries, Aquaculture and Living Resources
Volume 12 - 2025 | https://doi.org/10.3389/fmars.2025.1513070
Otolith Sr/Ca profiles are widely used to investigate early life migration and habitat use in both diadromous and oceanodromous fishes. This study focuses on Larimichthys polyactis, an oceanodromous species of significant ecological and commercial importance in East Asian waters. This study investigates the influence of environmental factors on the otolith Sr/Ca ratios during its early life stages (ELS). In Experiment A, we analyzed both laboratory-reared specimens—maintained under stable temperature (19–21°C) and salinity (27–28 PSU) conditions—and wild-captured specimens. The results revealed a consistent decline in Sr/Ca ratios throughout the ELS in both groups, suggesting that temperature and salinity may not be the primary drivers of otolith Sr/Ca ratios during early development. In Experiment B, Sr/Ca ratios in the core (incubation stage) and edge (recently spawned stage) zones of otoliths from both wild and laboratory-reared adult fish were compared. Sr/Ca ratios were significantly higher in the core zone than in the edge zone, indicating that maternal influences are may not be the main cause of elevated Sr/Ca ratios in the otolith core. Collectively, these findings suggest that otolith Sr/Ca ratios during ELS in L. polyactis are more affected by the ontogenetic developmental stage than by environmental factors such as temperature, salinity, or maternal effects. This challenges previous assumptions about the dominance of environmental factors in shaping otolith chemistry and highlights the need for more nuanced interpretations of Sr/Ca data, especially in studies of oceanodromous fishes. When utilizing otolith microchemistry to reconstruct life history, it is essential to minimize physiological effects through controlled culture experiments to ensure the accuracy and reliability of the results.
The early life stages (ELS), encompassing embryonic, larval, and juvenile phases, are pivotal periods in the life cycle of fish, marked by notably high mortality rates (Houde, 1997). Connectivity among these ELS acts as a vital link, bridging spatially segregated life history stages and profoundly influencing the population structure, dynamics, and evolution of fish species (Cowen and Sponaugle, 2009; Kool et al., 2013; Ovenden, 2013). For numerous marine species, dispersal occurs during the ELS, which is crucial for population connectivity and recruitment success (Ospina-Alvarez et al., 2015; Cabral et al., 2021). For example, the Pacific salmon undergoes a remarkable journey from freshwater spawning grounds to the ocean, navigating through various habitats during its ELS, which greatly influences its population dynamics and survival (Arbeider et al., 2024). The challenges encountered during ELS, especially among marine fish, include their limited swimming capabilities and the complexities of their vast environment (Cowen and Sponaugle, 2009), underscoring the significant roles played by abiotic factors, such as temperature, currents, and dissolved oxygen (Bailey et al., 2008; Molina-Valdivia et al., 2021); biotic factors, including prey density, predators, and competition (Saito et al., 2009; Fennie et al., 2020); and anthropogenic pressures, such as fishing activity (Cartwright, 2009); all of which directly or indirectly influence migration, growth, and recruitment patterns. Based on the critical importance of early life history (ELH) information, especially for migratory and overexploited fish species, there is a growing consensus within the fisheries management community to prioritize robust research in this area (Tulp et al., 2013; Jiang et al., 2022). Understanding ELS not only contributes to effective fisheries management, but also informs broader conservation efforts and ecosystem management strategies, ensuring the sustainability of marine ecosystems and the livelihoods of coastal communities.
There are formidable challenges associated with identifying and delineating the ELH traits of migratory fishes, particularly for the vast and intricate environment of the open ocean (Cowen and Sponaugle, 2009). The microchemistry of otoliths has proven an unrivaled tool for exploring the ELH traits of numerous fishes (Elsdon and Gillanders, 2003; Ruttenberg et al., 2008; Starrs et al., 2014). Two key assumptions underlie the use of otolith microchemistry as environmental tracers and proxies to reconstruct habitat histories. First, otolith chemistry is believed to reflect the chemical composition of the surrounding water (Elsdon and Gillanders, 2004; Izzo et al., 2015). Second, variations in otolith chemistry are influenced by ambient environmental conditions, such as salinity and temperature (Dorval et al., 2011; Morrissey et al., 2020). Among the various elements found in otoliths, strontium (Sr) has attracted particular attention due to its unique properties and potential applications for studying environmental histories of fish (Macdonald and Crook, 2010). The concentration of Sr relative to calcium (Ca) within otoliths has shown relative stability across different aquatic habitats, with significant variations observed among habitats (Elsdon and Gillanders, 2006; Yang et al., 2011; Xiong et al., 2021). This stability, along with Sr’s passive transport across membrane barriers and its incorporation into otolith mineral precipitation, renders it a promising candidate for environmental tracing in fish (Campana, 1999; Hüssy et al., 2020). Moreover, previous studies have demonstrated correlations between water Sr/Ca ratios and salinity (Yang et al., 2011), providing a basis for reconstructing salinity histories experienced by fish with various migratory patterns (Santana et al., 2018). However, it is essential to recognize that otolith microchemistry is influenced not only by environmental factors, but also by physiological factors and feeding habits (Sturrock et al., 2015; Izzo et al., 2018; Tran et al., 2019; Hüssy et al., 2020). However, the effects of these factors are often minimal, especially for diadromous migration between freshwater and marine environments, where the substantial differences in habitats offset these minor influences (Hüssy et al., 2020). In contrast, for oceanodromy exclusively within marine environments, where the salinity of the habitats is relatively stable, the relative importance of physiological factors increases (Sturrock et al., 2012). Relying solely on otolith microchemistry to reconstruct fish life histories may not be entirely reliable, particularly during periods of rapid growth and development during the ELS of fish (Brown and Severin, 2009; Sturrock et al., 2012).
Larimichthys polyactis, a typically oceanodromous species that inhabits the East China, Yellow, and Bohai Seas, holds significant ecological and commercial importance in East Asian nations (Ying et al., 2011; Choi and Kim, 2020; Song et al., 2022a). Studies that focused on the otolith microchemistry of L. polyactis predominantly centered around otolith Sr/Ca analysis, constituting approximately 73% of the relevant literature (based on searches conducted on 19 March 2024 via the Web of Science and China National Knowledge Infrastructure databases, with search criteria including (L. polyactis) and (otolith)). However, a substantial disparity exists between the findings of these studies (Xiong et al., 2017; Song et al., 2022b) and field monitoring observations for the eggs and larvae of L. polyactis (Lin et al., 2018; Xu et al., 2023) regarding ELH traits. In numerous investigations, the otolith Sr/Ca profiles of L. polyactis exhibited two notable characteristics: (i) markedly elevated Sr/Ca values in the otolith core zone, and (ii) a gradual decline in otolith Sr/Ca during ELH (Xiong et al., 2017; Song et al., 2022b; Xiong et al., 2021). Consequently, it is hypothesized that the ELH involves migration from offshore with high-salinity waters (salinity: 25–34 PSU) to nearshore areas with relatively lower salinity (salinity: 5–25 PSU) (Xiong et al., 2017). Conversely, field monitoring surveys of L. polyactis have indicated that the eggs predominantly occur in offshore waters exceeding 30 m in depth (salinity of 28–34 PSU), while larvae are mainly distributed in nearshore waters (salinity of 25–32 PSU), and juveniles tend to migrate to open ocean waters (Lin et al., 2018; Xu et al., 2023). The most significant difference between the otolith Sr/Ca profile interpretation and the field monitoring surveys lies in the salinity of the nursery habitats. Otolith microchemistry suggests that L. polyactis prefers lower salinity estuarine waters (5–25 PSU) as nursery grounds, while field surveys indicate a scarcity of L. polyactis in these estuarine, low-salinity areas (Xu et al., 2023). According to the field surveys, it is theoretically anticipated that the otolith Sr/Ca during the ELH of L. polyactis should exhibit a trend of an initial decrease followed by an increase in later stages.
In the present study, we aimed to explore the drivers of otolith Sr/Ca during the ELS, focusing on L. polyactis, a species subject to overexploitation and migratory patterns. The schematic representation of the study’s fundamental framework is depicted in Figure 1, which consists of four parts (Figure 1a, b). Figure 1a illustrates the basic procedure for extracting otoliths from the brain of L. polyactis for Sr/Ca analysis, while the subsequent figures detail other key processes in the study. Previous investigations into the otolith Sr/Ca of L. polyactis have revealed two common characteristics (Figure 1b). Drawing from the correlations between water Sr/Ca ratios and salinity, it is inferred that their ELH involves incubation in offshore waters with high salinity (25–34 PSU), followed by feeding and nursery phases in nearshore waters with relatively lower salinity (5–25 PSU). However, this inference contradicts findings from field monitoring surveys of L. polyactis eggs and larvae (Lin et al., 2018; Xu et al., 2023). Therefore, experiments A and B were conducted to explore the potential factors that contributed to the observed common characteristics of otolith Sr/Ca. Experiment A was designed to investigate whether the Sr/Ca ratio patterns observed in wild L. polyactis could be attributed to specific environmental factors, such as temperature and salinity. This experiment did not aim to directly compare wild and cultured samples but rather to use the cultured samples to validate the Sr/Ca patterns observed in the wild. The cultured fish were reared under stable and controlled environmental conditions (i.e., constant temperature and salinity), where significant fluctuations in otolith Sr/Ca ratios would not be expected. The wild samples, in contrast, were exposed to natural environmental variations. This setup allows us to determine whether the Sr/Ca changes observed in the wild are primarily driven by these environmental factors. Thus, the purpose of Experiment A was not to compare the two groups directly but to use the cultured group to explore the potential role of environmental conditions in the observed Sr/Ca patterns in the wild (Figure 1b). Experiment B focused on comparing the Sr/Ca ratios in the core and edge regions of otoliths from adult L. polyactis (wild and cultured), specifically targeting individuals at gonadal development stages V (spawning period) and VI (spent period) (Lim et al., 2010). The otolith core reflects the environmental conditions experienced during the early life stage (i.e., hatching), whereas the edge corresponds to conditions experienced during the adult stage (i.e., after spawning). By comparing these two regions, we aimed to assess whether maternal influence affected the Sr/Ca deposition in the otolith. If the Sr/Ca ratios in the core and edge are similar, it would suggest a maternal effect on Sr/Ca deposition. Conversely, if significant differences exist between the two regions, it would imply that environmental or physiological factors played a more prominent role in shaping the otolith’s Sr/Ca profile (Hegg et al., 2018). This comparison allows us to explore the potential influences of maternal inheritance versus environmental conditions (Figure 1b).
Figure 1. Fundamental framework of this study. (a): Procedural framework entailing the extraction, grinding, observation, and subsequent analysis of otolith chemistry. (b): Two common characteristics of otolith Sr/Ca in ELS of L. polyactis (Xiong et al., 2017; Song et al., 2022b; Xiong et al., 2021). The first characteristic, represented by a black hollow arrow, indicates a gradual decline in otolith Sr/Ca concentrations throughout ELS. The second characteristic, depicted by a light-blue rectangle, highlights the notable elevation in Sr/Ca values at the otolith core zone. The letters E, L, M, and J correspond to the egg, larva, metamorphosis, and juvenile stages, respectively. Experiment A devised to probe the first characteristic of otolith Sr/Ca in L. polyactis. Experiment B tailored to scrutinize the second characteristic of otolith Sr/Ca in L. polyactis.
Eggs were acquired through induced spawning of captive broodstock housed at Rudong Station, Jiangsu Marine Fisheries Research Institute, located in Nantong, China, adjacent to the Lvsi fishing ground (Figure 2), utilizing the methodology outlined by Fang et al. (2023). A cohort of 30 robust and vigorous two-year-old L. polyactis (with a sex ratio of two females to one male) received intramuscular injections of gonadotropin-releasing hormone analog (GnRHa). Eggs were transferred and incubated in 2,500-L fiber-reinforced plastic tanks maintained at mean temperature, salinity, and pH levels of 18°C, 25 PSU, and 8.0, respectively.
Figure 2. Map depicting the breeding grounds of Larimichthys polyactis and the sampling sites for wild L. polyactis in the offshore Lvsi fishing ground, China. The letters “LS” in the figure represent the Lvsi fishing ground.
After approximately 60 hours, the eggs hatched, and the larvae were transferred to a 750-L fiber-reinforced plastic tank, where the water temperature was controlled between 19 °C and 21 °C, with salinity levels of 27–28 PSU and a pH range of 8.0 to 8.3, for up to 45 days. Previous research has shown that a temperature close to 21 °C promotes better growth and higher survival rates for L. polyactis compared to lower (16 °C) or higher (34 °C) temperatures (Sun et al., 2018). In this study, the salinity was maintained at 27-28 PSU throughout the entire rearing period, from the development of juvenile fish to adulthood, to ensure consistency with the broodstock’s environment. In China, coastal waters typically have lower salinity levels, ranging from 25 to 30 PSU, and over time, farmed L. polyactis have adapted to these conditions. By keeping the salinity stable at 27–28 PSU, we aimed to avoid stressing the larvae due to sudden changes in salinity, providing them with an environment similar to that of the broodstock.
Temperature control was facilitated by a digital temperature controller (ZKH-WK90, ZHONGKEHAI, Qingdao, China). The salinity levels were regulated by diluting filtered seawater from the Lvsi fishing ground (32 ± 0.25 PSU) with non-chlorinated well water from Rudong Station. Pre-mixed salinity treatments were stored in each cold room for 24 h prior to use in tanks to eliminate the need for acclimation during daily water exchanges. Partial water exchanges (50%) were conducted daily, coupled with the removal of excess food, waste, or deceased fish through siphoning. The temperature and salinity levels were monitored daily for consistency using a YSI-55 probe. To maintain water quality, ammonia levels were regularly assessed and maintained below 0.25 ppm using commercial test kits (API, Chalfont, PA, USA). Beginning four days post-hatching (DPH), the larvae were provided with a sequential diet consisting of rotifers, artemia nauplii, and pellet feed. As the fry continued to develop, timely segregation was performed to reduce fry density, thereby promoting higher survival rates.
On the first DPH and approximately every 10 d thereafter, duplicate water samples were collected from the aquaculture tank using polypropylene syringes to determine the water’s elemental concentrations. Throughout the entire experimental aquaculture period, a total of six water samples were collected. These were filtered through GF/F filters (0.22-μm pore size) into 100-mL flasks and acidified with concentrated ultrapure nitric acid (HNO3) in a 1:50 ratio. These samples were then stored at 4°C until analysis.
Prior to elemental quantification by inductively coupled plasma–mass spectrometry (ICP-MS), 3 mL of each water sample underwent further acidification at a 1:10 ratio by adding 3 mL of previously distilled 10% nitric acid solution. The water samples were then analyzed for 43Ca and 88Sr using a Thermo Fisher Scientific Model iCAP Q spectrophotometer (Bremen, Germany). The ICP-MS measurements were calibrated using a five-point calibration curve per element. Standard solutions were prepared by diluting a multi-element standard (92091, Periodic table mix 1 for ICP, Sigma-Aldrich) and a standard curve was generated for each element. For calcium (Ca), the calibration was performed within the range of 0 to 5 mg/L with an R² value of 0.99996. This calibration range was selected to accurately reflect the concentration of Ca, given that it is a macro element present in higher concentrations. For strontium (Sr), the calibration curve was within the range of 0 to 100 µg/L, as previously detailed in the Sr calibration curve. This range was selected to capture the trace levels of Sr in the samples. The calibration for Sr also demonstrated high linearity with an R² value of 0.9997. The blank controls comprised ultrapure water acidified with HNO3 at 10%. Standards and blanks were analyzed at the beginning and throughout each analysis session. After each group of samples is analyzed, rinse twice with 10% HNO3 and triple rinsed with Milli-Q water. The deviation of three parallel samples with known concentrations from the actual concentration was less than 10%. The quantification limits for 43Ca and 88Sr were determined to be 0.014 µg/L and 14.66 μg/L, respectively.
A total of 10 cultured young L. polyactis, aged between 45 and 60 DPH, were collected, encompassing the whole ELS. The wild young L. polyactis were captured using a custom-designed sampling net in the offshore Lvsi fishing ground (Figure 2; Table 1). The sampling nets were constructed using a framework of stow nets comprised of a square steel pipe (1.35 m × 1.35 m) affixed to the mouth. Fabricated from polyethylene, these nets had a length of 6 m and mesh size of 2 mm. Anchored by a single stake, with additional floats affixed to enhance buoyancy, the orientation of the net mouth was designed to dynamically adjust with the prevailing current, optimizing the sample capture efficiency. Based on otolith microstructure (Campana and Neilson, 1985; Li et al., 2013), 20 wild individuals with an approximate age of 60 DPH were selected for comparative analysis with the cultured young individuals.
All collected specimens were promptly stored at –4°C until subsequent analysis. Prior to dissection, standard length (SL, mm) and body weight (BW, g) measurements were taken for each specimen. Sagittal otoliths were extracted from L. polyactis, and meticulously cleaned, desiccated, numbered, and stored in plastic tubes. Age information was obtained by observing otolith micro-increments (daily increments and annual increments) and by referring to the growth equation (Kang et al., 2022). Samples details are presented in Table 1.
Throughout their range, the ELS of L. polyactis tend to inhabit the upper strata of the water column, a behavior attributed to their constrained swimming abilities (Lin et al., 2018; Liu et al., 2022; Xu et al., 2023). Their diet predominantly comprises phytoplankton and zooplankton (including copepods, decapods, and mysids), positioning them strategically within the upper water layers (Wei et al., 2018; Oh et al., 2022). Thus, this study used daily surface salinity (SSS) and sea surface temperature (SST) datasets from the Hybrid Coordinate Ocean Model (HYCOM), to elucidate the thermal and salinity profiles experienced by wild L. polyactis. The HYCOM is a data-assimilative ocean model that integrates both observational data and numerical modeling to provide accurate estimates of oceanographic conditions, including temperature, salinity, and currents (Cummings and Smedstad, 2013). These datasets, featuring a spatial resolution of 1/12°, encompassed the geographic coordinates spanning from 121°E to 123°E longitude and 32°N to 34°N latitude, with temporal coverage spanning March to May 2022. The spatial extent of environmental data extraction aptly encapsulated the early activity range of the L. polyactis (Xu et al., 2023). The daily mean SST and SST values pertinent to the specified geographical coordinates were computed leveraging the capabilities Google Earth Engine (GEE).
Ten cultured adult L. polyactis (the first minimum age for sex maturity was age 1) at gonadal development stage VI (spawned stage) were collected, as well as ten wild adult L. polyactis at stage VI sampled from catches by commercial vessels utilizing gill nets with a mesh size of 50 mm. The methodologies for treating L. polyactis samples and preparing otoliths were as described for Experiment A.
The otolith Sr/Ca analysis regions for both wild and cultured adult L. polyactis were the core and edge zones. The otolith core zone corresponds to stage E described below. Additionally, the otolith edge zone corresponds to the last weeks before the fish were captured, representing the signature of the sampling location.
Research on larval rearing of L. polyactis has established a strong linear relationship between the fish’s age in days (t) and the number of otolith increments (y), expressed as y = –0.74 + 0.99t (n = 61, R² = 0.997, P < 0.001) (Li et al., 2013). The increments in L. polyactis otoliths were found to form daily, with characteristic marks indicating key stages of development such as incubation, first feeding, and metamorphosis (Li et al., 2013; Zhan et al., 2016). This daily growth pattern was validated through these experiments, providing a reliable method to track early life stages. Based on the morphological developmental characteristics and otolith microstructure features of L. polyactis, the ELS of L. polyactis can be subdivided into the yolk sac (E), larval (L), metamorphosis (M), and juvenile (J) stages (Table 2; Figure 3; Li et al., 2013; Zhan et al., 2016). (1) Stage E occurs before 4 DPH, during which the main source of nutrition is absorption from the yolk sac (Zhan et al., 2016). On the otolith, the prominent features are the primordium and the first feeding check. The first feeding check occurred on the fourth increment of a distance of 20.67 ± 2.28 μm from the central nucleus, displaying a larger width, deeper color, and high clarity (Li et al., 2013; Song et al., 2022b). (2) Stage L occurs between 6 and 15 DPH, during which feeding gradually shifts toward zooplanktonic organisms, such as Mysidacea, Copepoda, and Euphausiacea (Oh et al., 2022). (3) Stage M occurs between 16 and 25 DPH, marking the transition from larva to juvenile. During this period, the fish’s fin rays and skeletal structures gradually develop and mature, while the otolith exhibits sub-daily increments (Li et al., 2013; Zhan et al., 2016). The sub-daily increments in stage M exhibit irregularity and incompleteness, posing challenges in counting daily increments for investigating early life migration (Song et al., 2022b). (4) Stage J occurs between 26 and 45 DPH, during which L. polyactis exhibit significant changes in feeding habits, primarily consuming small planktonic shrimp (Wei et al., 2018). The developmental stages described correspond to the otolith regions of both wild and cultured L. polyactis that were analyzed for Sr/Ca.
Figure 3. Sagittal otolith of wild young Larimichthys polyactis showing detecting spots for otolith Sr/Ca analysis. The yellow, red, green, and blue dots represent the yolk sac, larva, metamorphosis, and juvenile stages, respectively. The letter “F” represents the first feeding check.
The methodology employed for the analysis of otolith Sr/Ca ratios was electron probe microanalysis (EPMA) that followed the protocols detailed by Jiang et al. (2017). Initially, prepared sagittal otoliths were embedded in transparent epoxy resin (EpoFix; Struers, Copenhagen, Denmark) and subjected to thin-sectioning along the sagittal plane using a diamond cup wheel (Discoplan-TS; Struers, Copenhagen, Denmark). Subsequently, these thin sections were affixed to glass microscope slides with AB glue, followed by meticulous sanding to expose the core encircled by distinct daily rings on both faces, utilizing 1200–2000-grit SiC paper. Further refinement was achieved through polishing with 0.3-μm alumina on an automated polishing wheel (Labopol-35, struers, Copenhagen, Denmark) to eliminate significant surface imperfections. Post-polishing, the otoliths underwent sonication in an ultrasonic cleaner for 5 min and rinsing with deionized water. Ultimately, all samples were dried and carbon-coated using a high-vacuum evaporator (JEE-420, JEOL Ltd., Tokyo, Japan) to facilitate subsequent analysis.
For EPMA, otolith sections were prepared following the methodology delineated by Jiang et al. (2017), with minor adaptations. Specifically, the Sr and Ca within the otoliths were quantitatively assessed along the longest linear path from the core to the periphery (i.e., otolith radius) utilizing an electron probe microanalyzer (JXA-8100; JEOL Ltd.). Quantitative determinations were conducted under the following beam parameters: 15 kV for accelerating voltage, 20 nA for the beam current, and a circular scanning beam with a diameter of 2 μm, with measurements spaced at 10 μm intervals. To ensure accuracy, commercial standards of calcite (CaCO3) and tausonite (SrTiO3) sourced from the Institute of Mineral Resources, Chinese Academy of Geological Sciences, Beijing, China, were employed to calibrate the Sr and Ca concentrations within the otoliths. The precision (error) of the Sr and Ca measurements was 0.36% for Sr and 0.22% for Ca, with limits of detection at 472 ppm for Sr and 92 ppm for Ca.
In conventional otolith research, the results for Sr and Ca are reported as ratios of Sr to Ca amplified by 1000 by a conversion based on the molecular mass of CaO and SrO. The Sr/Ca dataset in the ELS of L. polyactis was collected at 10 μm intervals, creating a time-series-like structure with strong correlations between data points. To reduce this correlation and increase data independence in Experiment A, we averaged the Sr/Ca values for each stage (E, L, M, and J), as well as for the core and edge zones in Experiment B.
In Experiment A, we first conducted Shapiro-Wilk normality tests and Levene’s test for homogeneity of variance on the data. The results confirmed that the data met the assumptions of normality and homogeneity. Consequently, a one-way ANOVA was performed to assess the differences in otolith Sr/Ca ratios among the E, L, M, and J stages for both cultured and wild samples. Given that there were only four groups, Tukey’s test was used for post-hoc multiple comparisons to identify specific group differences. Additionally, an independent samples t-test was used to determine the significance of differences between wild and cultured groups at the same developmental stages.
To better observe the Sr/Ca trends for wild and cultured samples during the early life stages (ELS), we applied the STARS analysis (Sequential t-test analysis of regime shifts), following the method of Rodionov (2004); Rodionov and Overland (2005); Xiong et al. (2017). This approach automatically detects significant changes in the mean levels of time-series data. For our analysis, we set the Huber weight to 1, confidence level to 0.5, and cut-off length to 5. The STARS analysis allowed us to effectively identify Sr/Ca trend shifts in the wild and cultured samples at different developmental stages, helping us better understand the significant transitions during the early life history of L. polyactis.
In Experiment B, we compared the Sr/Ca ratios between the core and edge zones within the same samples from both wild and cultured fish. Since this involved comparing paired data, a paired samples t-test was applied to determine the significance of the differences between the two regions.
All statistical analyses were performed using Origin 2024 (Originlab, Northampton, MA, USA).
In our aquaculture experiments, stringent control was exercised over the salinity and temperature of the rearing water, maintaining them within stable values of 27.44 ± 0.5 PSU and 20.17 ± 0.86°C, respectively. Throughout the 45-d rearing period, systematic monitoring of the water Sr/Ca ratio was conducted at 10-d intervals. The Sr/Ca ratio in the rearing water exhibited consistent values of 14.0, 14.8, 14.5, 14.6, and 14.4 μmol·mol−1 across successive assessments.
Through meticulous examination of otolith microstructures, we selectively sampled wild young L. polyactis specimens that hatched around April 1st from the Lvsi fishing ground. April 1st marks the designated birth date for L. polyactis, with subsequent periods delineated as follows: stages E (April 1st to 4th), L (April 5th to 15th), M (April 16th to 25th), and J (April 26th to May 15th). The daily average SST and SSS data spanning April 1st to May 31st, 2022, were extracted for the nearshore waters of the Lvsi fishing ground (Figure 4). SST exhibited a progressive rise from 11.54°C to approximately 20°C. Notably, wild young L. polyactis experienced average SSTs of 11.66, 13.35, 14.95, and 17.77°C during stages E, L, M, and J, respectively. Conversely, SSS demonstrated a marginal decline from an initial value of approximately 32 to 30, maintaining an approximate 2 PSU differential relative to the aquaculture water.
Figure 4. Daily average SST and SSS of Lvsi fishing ground from April to May 2022. The letters E, L, M, and J in the figure represent the four stages of L. polyactis early life history, separated by vertical dashed lines.
The Lvsi Fishing Ground, located near the northern part of the Yangtze River Estuary, is heavily influenced by the freshwater outflow from the Yangtze River, which significantly affects both SST and SSS in the surrounding marine environment (Bao et al., 2015; Yu et al., 2020). The observed environmental changes, including the 8°C increase in SST and the 2 PSU decrease in SSS over the 45-day study period, align with the region’s seasonal dynamics. The period from April to May marks the transition from spring to early summer in the Northern Hemisphere, during which surface waters warm rapidly (Xu et al., 2023). Additionally, this period coincides with the increased freshwater discharge from the Yangtze River, diluting the seawater causing a decrease in salinity. These factors collectively reflect the natural seasonal changes in the region, driven by both climatic conditions and the hydrological cycle of the Yangtze River.
The Sr/Ca ratio trends in wild and cultured L. polyactis during their ELS were analyzed using the STARS method. In the wild L. polyactis group, the overall Sr/Ca ratios exhibited a gradual decline during the ELS (Figure 5). Within each of the four developmental stages, the Sr/Ca ratios showed distinct patterns. During stage E, the ratios remained relatively high but began to decrease steadily by the onset of stage L. In stages L and M, the decline became more pronounced, with noticeable shifts in mean Sr/Ca levels. By stage J, the Sr/Ca ratios stabilized at lower levels, indicating a gradual reduction over time as the fish progressed through the early life stages. For the cultured group, a similar downward trend in Sr/Ca ratios was observed (Figure 5). However, the decline was less steep compared to the wild samples. In stages E and L, the ratios started higher but decreased more gradually. The transitions between stages were smoother in the cultured group, with fewer abrupt shifts in Sr/Ca levels compared to the wild group. Stage J showed lower Sr/Ca ratios, but the overall decline was less significant than in the wild samples.
Figure 5. Otolith Sr/Ca profiles of the wild (n = 20) and cultured (n = 10) young L. polyactis during their early life stages. The solid lines represent the overall fluctuation of Sr/Ca ratios for wild and cultured groups, based on the Sequential t-test analysis of regime shifts (Rodionov, 2004). The letters E, L, M, and J in the figure represent the four stages of L. polyactis early life history, separated by vertical dashed lines.
The Sr/Ca ratios (mean ± SD) observed at stages E, L, M, and J among wild and cultured L. polyactis were as follows: 8.30 ± 1.98 and 7.64 ± 1.79, 7.13 ± 1.35 and 6.16 ± 1.14, 6.72 ± 1.22 and 6.19 ± 0.96, and 5.42 ± 1.12 and 3.96 ± 1.07, respectively. Throughout the ELS of both wild and cultured L. polyactis, a gradual decline in the Sr/Ca ratios of their otoliths was evident (Figure 5), punctuated by two significant decline nodes: from stages E to L and from stages M to J (p < 0.05; Figure 6). Notably, the otolith Sr/Ca ratios at stages E, L, M, and J of the wild samples consistently exceeded those of cultured samples (Figure 6). Furthermore, within these stages, stages L and J of the wild samples exhibited significantly higher Sr/Ca values compared with cultured samples (Figure 6).
Figure 6. Boxplot illustrating the otolith Sr/Ca variation of the wild and cultured young L. polyactis. Lowercase and uppercase letters positioned above the boxes indicate significant differences among the early life stages (E, L, M, and J) of wild and cultured L. polyactis, respectively, as determined by one-way ANOVA (p < 0.001). Asterisks indicate significant differences between the same stages based on independent samples t-test (*** = p < 0.001).
The Sr/Ca ratio (mean ± SD) observed at the core and edge zones of wild adult L. polyactis were 6.53 ± 1.12 and 4.70 ± 1.13, respectively, whereas those from cultured adult L. polyactis were 7.37 ± 1.18 and 5.60 ± 0.91. Notably, the otolith Sr/Ca ratios at the core zone for both wild and cultured samples were significantly higher than those observed at their respective edge zones (p < 0.05; Figure 7).
Figure 7. Otolith Sr/Ca variation between core and edge zones of the wild and cultured adult L. polyactis. Asterisks indicate significant differences based on paired samples t-test (*** = p < 0.001).
During the ELS, the otolith Sr/Ca ratios in both cultured and wild L. polyactis exhibited a descending trend (Figure 5), consistent with previous findings (Xiong et al., 2017; Song et al., 2022b). Notably, there was no significant difference between the Sr/Ca ratios at stages L and M (Figure 6). If not for the comparison with cultured samples, based solely on the variations in SST and SSS of the habitat of wild samples (Figure 4), it would be highly plausible to attribute the pattern of otolith Sr/Ca in the wild samples to the changes in SST. Indeed, some experimental studies have shown a negative correlation between otolith Sr/Ca and temperature, such as for Gadus macrocephalus (DiMaria et al., 2010) and Perca flavescens (Collingsworth et al., 2010). Generally, in regions with cold water, the solubility of strontium (Sr2+) and barium (Ba2+) ions tends to be higher, leading to elevated concentrations (Corrège, 2006; Saenger et al., 2008). Consequently, fish inhabiting such environments incorporate more of these ions into their otoliths during formation, resulting in higher Sr/Ca or Ba/Ca ratios (Mondal et al., 2022). Conversely, in warmer regions, the solubilities of Sr2+ and Ba2+ ions are lower, resulting in lower concentrations in water (Corrège, 2006; Saenger et al., 2008). As a result, fish forming otoliths in warmer water absorb fewer Sr2+ and Ba2+ ions, leading to lower Sr/Ca and Ba/Ca ratios (DiMaria et al., 2010; Mondal et al., 2022). In aquaculture environments with relatively stable water temperatures, the otolith Sr/Ca ratios of cultured L. polyactis also gradually declined, suggesting that water temperature may not be the primary factor influencing the deposition of Sr/Ca in L. polyactis otoliths. Previous studies have conducted meta-analyses focusing on Sr and Ba in otolith chemistry literature, that considered various species, life histories, and environmental conditions, to explore the relationship between ambient salinity and otolith Sr/Ca ratios (Campana, 1999; Izzo et al., 2018). The synthesis of data from these studies indicates a significant positive relationship between ambient salinity and otolith Sr/Ca (Arai, 2010; Hicks et al., 2010; Panfili et al., 2015). Moreover, extensive monitoring data revealed that river and lake environments exhibit a wider range of Sr/Ca levels than marine environments (Brown and Severin, 2009). The median river (2.39) and lake (1.92) Sr/Ca levels are substantially lower than those in the sea (8.61), with Sr/Ca levels ranging from 0.27 to 19.18 in rivers, 0.20 to 5.02 in lakes, and 8.17 to 8.87 in marine water (Yang et al., 2011). The otolith Sr and Sr/Ca ratios are significantly related to salinity for all species, but their predictive resolution is only sufficient to discriminate among fresh water, brackish water, and saltwater residency (Zimmerman, 2005). The salinity of both wild and cultured L. polyactis’s habitat waters remained relatively stable without significant fluctuations, indicating salinity was unlikely to have been the primary determinant of the observed decrease in otolith Sr/Ca ratios. The black line in the Figure 5 represents the Sr/Ca trend in the wild L. polyactis samples. As observed, the Sr/Ca ratios in the wild samples exhibit more pronounced fluctuations and a steeper decline during the ELS. After ruling out temperature and salinity as the primary influencing factors, it is hypothesized that this trend may relate to the physiological growth and development of the fish (Pontual et al., 2003; Peek and Clementz, 2012). During ELS, rapid protein synthesis is essential for growth, particularly in the formation of skeletal structures. This increased demand for calcium in protein synthesis may result in lower calcium availability for otolith formation. As a consequence, the calcium content in the otolith crystal structure decreases, potentially causing other elements related to calcium, such as strontium (Sr), to increase in concentration. Mohan et al. (2022) suggested that the combination of heightened protein concentrations and lower calcium levels could lead to elevated concentrations of other elements, including Sr. In this scenario, Sr may replace Ca in the otolith’s calcium carbonate matrix, contributing to the observed higher Sr/Ca ratios in the core zone of the otolith during early life stages (Courtemanche et al., 2006; Doubleday et al., 2014). This substitution mechanism could explain the significant differences in Sr/Ca ratios among ELS, particularly during the initial stages of development, when the demand for calcium in biological processes is higher. Considering the temperature and salinity environments of their respective habitats, it is plausible this phenomenon related to an additive effect of temperature, salinity, and internal physiological factors (Mazloumi et al., 2017; Tian et al., 2021).
For the adult otolith, If the Sr/Ca ratios in the core and on the edges differ significantly, it could indicate that environmental factors (such as temperature and salinity) and internal physiological factors (such as individual growth and development), rather than maternal effects, are the dominant influences on otolith composition. Notably, the otolith Sr/Ca ratios within the core zone, relating to the incubation period, for both wild and cultured specimens markedly exceeded those manifesting within their respective edge zones, relating to the spawning period, as depicted in Figure 7. Given the myriad and efficacious applications of otolith Sr/Ca in diadromous fishes, it is widely acknowledged that otolith Sr/Ca exhibits a robust correlation with ambient water salinity (Lin et al., 2007; Walsh and Gillanders, 2018; Islam et al., 2019). Consequently, previous investigations utilizing otolith Sr/Ca consistently postulated that L. polyactis eggs are incubated in offshore waters with elevated ambient water salinity, thereafter migrating to nearshore waters with lower salinity for feeding and growth (Xiong et al., 2017; Song et al., 2022b). However, this assertion appears to falter under scrutiny when considering the relatively stable ambient water salinity experienced by the cultured L. polyactis, which exhibited a similar otolith Sr/Ca trend. Other oceanodromous fish species, such as Collichthys lucidus (Liu et al., 2015) and Miichthys miiuy (Xiong et al., 2015a), also exhibit declining otolith Sr/Ca during their ELH. Furthermore, Xiong et al. (2021) identified a striking resemblance in the otolith Sr/Ca trends of L. polyactis collected a decade apart in 2003 and 2013. Additionally, Song et al. (2022b) compared the otolith Sr/Ca trends of L. polyactis that originated from Lvsi fishing ground, Haizhou Bay fishing ground, and waters off the western coast of the Korean Peninsula, uncovering notable similarities. Consistent decreasing otolith Sr/Ca trends in L. polyactis during ELS, irrespective of spatial or temporal variations in samples, suggests that it is unlikely to be solely driven by external environmental factors, such as salinity and temperature (Tomás et al., 2006; Albuquerque et al., 2012). Therefore, the results of experiment a suggesting that temperature and salinity may not be the primary drivers of otolith Sr/Ca ratios during early development.
The descending Sr/Ca ratios observed during ELH likely originated from three principal factors. First, during ELH, individuals undergo substantial changes in their morphology, physiology, and feeding behavior (Li et al., 2013; Xiong et al., 2015b; Zhan et al., 2016; Wei et al., 2018). In the initial stages, individuals rely on internal yolk sacs for nourishment (Zhan et al., 2016). As development progresses, individuals transition to external feeding, shifting from initially consuming phytoplankton to more complex food sources, such as small zooplankton (Wei et al., 2018). Concurrently, there are significant morphological changes, with the skeletal structure, fin rays, and scales of the fish gradually developing (Li et al., 2013; Zhan et al., 2016). Yatsu et al. (1998) reported differential impacts of Sr and Ca concentrations in the otolith cores of Ommastrephes bartramii during embryonic stages compared with the subsequent ontogenetic phases. In the case of L. polyactis, the complete absorption of the yolk sac within six days post-hatching, followed by reliance on exogenous nutrition with constrained feeding capacities, was a likely contributor (Zhan et al., 2016). Second, significant protein levels are likely necessary in L. polyactis during otolith core zone calcification, as suggested by Murayama et al. (2004) and Svedäng et al. (2020). Moreover, studies propose lower Ca concentrations in the otolith core zone compared with the other zones (Dove et al., 1996). The combination of heightened protein concentrations and reduced Ca levels could lead to elevated concentrations of other Ca-related elements (Mohan et al., 2022). Elements such as Sr replacing Ca as the primary inclusion mechanism in otolith calcium carbonate matrices, may contribute to the higher Sr/Ca ratios in the core zone (Courtemanche et al., 2006; Doubleday et al., 2014). The third factor is centered around the appearance of L. polyactis’ first feeding ring on the fourth increment, approximately 20.67 ± 2.28 μm away from the core zone, indicating prior reliance on yolk sac nutrients for sustenance (Zhan et al., 2016; Song et al., 2022b). Sagittal otoliths in teleost fish form during the embryonic period (Marannu et al., 2017); hence, maternal contributions to progeny otoliths are inevitable (Hegg et al., 2018; Janak et al., 2021). This is supported by evidence of maternal influences in various fishes, such as Morone Saxatilis (Elking, 2014), Mallotus villosus (Loeppky et al., 2018), and Oncorhynchus nerka (Janak et al., 2021). It is suggested that embryonic otolith chemistry may not reflect environmental conditions, such as water chemistry and temperature during egg incubation; rather, it may reflect maternally derived trace elements in the yolk (Volk et al., 2000; Zimmerman and Reeves, 2002) or be influenced by genetically controlled uptake dynamics (Chittaro et al., 2006). Although the results of experiment b suggest that maternal influences may not be the primary cause of elevated Sr/Ca ratios in the otolith core, they remain an essential factor in studying the early life history of fish. Collectively, otolith Sr/Ca ratios during ELS in L. polyactis are more affected by the ontogenetic developmental stage than by environmental factors such as temperature, salinity, or maternal effects.
Given the limitations of current experimental conditions, the Sr/Ca ratios analysis of otoliths has been confined to their growth stages for L. polyactis. Our research will benefit from combining daily environmental data with daily incremental analysis, which may lead to more accurate results. Furthermore, this underscores the necessity for a more detailed interpretation of Sr/Ca ratios. When investigating the life history of oceanodromous fish using Sr/Ca ratios or other elemental analyses, controlled culture experiments should be conducted to mitigate the influence of physiological factors and other variables.
This study explored the dynamics of otolith Sr/Ca ratios in Larimichthys polyactis during early life stages (ELS), examining both wild and cultured populations. Through Experiment A, we identified a consistent decline in Sr/Ca ratios across developmental stages (E, L, M, and J), suggesting that factors beyond simple environmental conditions, such as temperature and salinity, influence otolith chemistry. Experiment B further demonstrated significant differences in Sr/Ca ratios between the otolith core and edge zones, indicating the potential role of early developmental processes in shaping otolith composition. These findings challenge the prevailing notion that Sr/Ca ratios are solely driven by external environmental factors. Instead, our results suggest that internal biological processes, e.g. ontogenetic changes, play a more critical role in determining Sr/Ca deposition patterns. The similar trends observed in both wild and cultured fish reinforce this conclusion, as environmental stability in aquaculture did not prevent the progressive decline in Sr/Ca ratios. While this study provides valuable insights, it also raises new questions. Future research should investigate the precise biological mechanisms behind these Sr/Ca dynamics, including the potential interaction of other environmental factors such as food availability and water chemistry. Additionally, employing advanced statistical models like generalized linear models (GLM) may offer further clarity on the interplay between various influences. The study also offers valuable insights into novel culture experiments that investigate early life history through the analysis of chemical elements at the edge of otoliths, utilizing continuous sampling in conjunction with daily increments. Overall, this study enhances our understanding of otolith chemistry in larval fish and underscores the importance of considering multiple factors when interpreting Sr/Ca data, particularly in tracking early life histories.
The raw data supporting the conclusions of this article will be made available by the authors, without undue reservation.
The animal study was approved by Jiangsu Marine Fisheries Research Institute. The study was conducted in accordance with the local legislation and institutional requirements.
ZK: Conceptualization, Formal Analysis, Investigation, Methodology, Software, Validation, Visualization, Writing – original draft, Writing – review & editing. DS: Conceptualization, Formal Analysis, Investigation, Methodology, Software, Validation, Writing – original draft, Writing – review & editing. HZ: Formal Analysis, Investigation, Methodology, Software, Validation, Writing – original draft. LL: Data curation, Formal Analysis, Investigation, Methodology, Software, Validation, Writing – original draft. CZ: Investigation, Methodology, Software, Writing – original draft. TJ: Investigation, Writing – original draft, Writing – review & editing. FZ: Investigation, Resources, Writing – original draft. YX: Conceptualization, Investigation, Methodology, Project administration, Resources, Supervision, Validation, Writing – review & editing.
The author(s) declare that financial support was received for the research and/or publication of this article. This study was funded by the National Natural Science Foundation of China (Grant No. 31802297).
The authors declare that the research was conducted in the absence of any commercial or financial relationships that could be construed as a potential conflict of interest.
The author(s) declare that no Generative AI was used in the creation of this manuscript.
All claims expressed in this article are solely those of the authors and do not necessarily represent those of their affiliated organizations, or those of the publisher, the editors and the reviewers. Any product that may be evaluated in this article, or claim that may be made by its manufacturer, is not guaranteed or endorsed by the publisher.
Albuquerque C. Q., Miekeley N., Muelbert J. H., Walther B. D., Jaureguizar A. J. (2012). Estuarine dependency in a marine fish evaluated with otolith chemistry. Mar. Biol. 159, 2229–2239. doi: 10.1007/s00227-012-2007-5
Arai T. (2010). Effect of salinity on strontium:calcium ratios in the otoliths of Sakhalin taimen, Hucho perryi. Fish Sci. 76, 451–455. doi: 10.1007/s12562-010-0235-5
Arbeider M., Pemberton-Renaud V., Hodgson E. E., Moore J. W. (2024). The estuarine growth and residency of juvenile Pacific salmon in North America: a compilation of empirical data. Can. J. Fish Aquat Sci. 81, 253–267. doi: 10.1139/cjfas-2023-0225253
Bailey K. M., Abookire A. A., Duffy-Anderson J. T. (2008). Ocean transport paths for the early life history stages of offshore-spawning flatfishes: a case study in the Gulf of Alaska. Fish Fish. 9, 44–66. doi: 10.1111/j.1467-2979.2007.00268.x
Bao M., Guan W., Wang Z., Wang D., Cao Z., Chen Q. (2015). Features of the physical environment associated with green tide in the southwestern Yellow Sea during spring. Acta Oceanol Sin. 34, 97–104. doi: 10.1007/s13131-015-0692-x
Brown R. J., Severin K. P. (2009). Otolith chemistry analyses indicate that water Sr: Ca is the primary factor influencing otolith Sr: Ca for freshwater and diadromous fish but not for marine fish. Can. J. Fish Aquat Sci. 66, 1790–1808. doi: 10.1139/F09-112
Cabral H., Drouineau H., Teles-MaChado A., Pierre M., Lepage M., Lobry J., et al. (2021). Contrasting impacts of climate change on connectivity and larval recruitment to estuarine nursery areas. Prog. Oceanogr. 196, 102608. doi: 10.1016/j.pocean.2021.102608
Campana S. E. (1999). Chemistry and composition of fish otoliths: pathways, mechanisms, and applications. Mar. Ecol. Prog. Ser. 188, 263–297. doi: 10.3354/meps188263
Campana S. E., Neilson J. D. (1985). Microstructure of fish otoliths. Can. J. Fish Aquat Sci. 42, 1014–1032. doi: 10.1139/f85-127
Cartwright R. L. (2009). Description of early life history stages of the northern sculpin (Icelinus borealis Gilbert) (Teleostei: Cottidae). Fish Bull. 107, 175–185. doi: 10.1016/j.dsr.2023.104020
Chittaro P. M., Hogan J. D., Gagnon J., Fryer B. J., Sale P. F. (2006). In situ experiment of ontogenetic variability in the otolith chemistry of Stegastes partitus. Mar. Biol. 149, 1227–1235. doi: 10.1007/s00227-006-0280-x
Choi M., Kim D. (2020). Assessment and management of small yellow croaker (Larimichthys polyactis) stocks in South Korea. Sustainability. 12, 8257. doi: 10.3390/su12198257
Collingsworth P. D., Van Tassell J. J., Olesk J. W., Marschall E. A. (2010). Effects of temperature and elemental concentration on the chemical composition of juvenile yellow perch (Perca flavescens) otoliths. Can. J. Fish Aquat Sci. 67, 1187–1196. doi: 10.1139/F10-050
Corrège T. (2006). Sea surface temperature and salinity reconstruction from coral geochemical tracers. Palaeogeogr Palaeoclimatol Palaeoecol. 232, 408–428. doi: 10.1016/j.palaeo.2005.10.014
Courtemanche D. A., Whoriskey F. G. Jr., Bujold V., Curry R. A. (2006). Assessing anadromy of brook char (Salvelinus fontinalis) using scale microchemistry. Can. J. Fish Aquat Sci. 63, 995–1006. doi: 10.1139/F06-009
Cowen R. K., Sponaugle S. (2009). Larval dispersal and marine population connectivity. Annu. Rev. Mar. Sci. 1, 443–466. doi: 10.1146/annurev.marine.010908.16
Cummings J. A., Smedstad O. M. (2013). “Variational data assimilation for the global ocean,” in Data assimilation for atmospheric, oceanic and hydrologic applications, vol. II . Eds. Park S., Xu L. (Springer, Berlin, Heidelberg). doi: 10.1007/978-3-642-35088-7_13
DiMaria R. A., Miller J. A., Hurst T. P. (2010). Temperature and growth effects on otolith elemental chemistry of larval Pacific cod, Gadus macrocephalus. Environ. Biol. Fish. 89, 453–462. doi: 10.1007/s10641-010-9665-2
Dorval E., Piner K., Robertson L., Reiss C. S., Javor B., Vetter R. (2011). Temperature record in the oxygen stable isotopes of Pacific sardine otoliths: Experimental vs. wild stocks from the Southern California Bight. J. Exp. Mar. Biol. Ecol. 397, 136–143. doi: 10.1016/j.jembe.2010.11.024
Doubleday Z. A., Harris H. H., Izzo C., Gillanders B. M. (2014). Strontium randomly substituting for calcium in fish otolith aragonite. Anal. Chem. 86, 865–869. doi: 10.1021/ac4034278
Dove S. G., Gillanders B. M., Kingsford M. J. (1996). An investigation of chronological differences in the deposition of trace metals in the otoliths of two temperate reef fishes. J. Exp. Mar. Biol. Ecol. 205, 15–33. doi: 10.1016/S0022-0981(96)02610-X
Elking B. A. (2014). Maternal input of striped bass (Morone saxatilis): Determining a mother’s life history from its progeny in coastal North Carolina rivers. MSc Thesis. (Greenville, NC: East Carolina University).
Elsdon T. S., Gillanders B. M. (2003). Reconstructing migratory patterns of fish based on environmental influences on otolith chemistry. Rev. Fish Biol. Fish. 13, 217–235. doi: 10.1023/B:RFBF.0000033071.73952.40
Elsdon T. S., Gillanders B. M. (2004). Fish otolith chemistry influenced by exposure to multiple environmental variables. J. Exp. Mar. Biol. Ecol. 313, 269–284. doi: 10.1016/j.jembe.2004.08.010
Elsdon T. S., Gillanders B. M. (2006). Identifying migratory contingents of fish by combining otolith Sr: Ca with temporal collections of ambient Sr: Ca concentrations. J. Fish Biol. 69, 643–657. doi: 10.1111/j.1095-8649.2006.01136.x
Fang X. Z., Hu W. H., Chen R. Y., Zeng Y. Q., Chai X. J., Xu D. D. (2023). Evaluation of the artificial oxytocin effect of GnRH injection and oral administration on small yellow croaker (Larimichthys polyactis). Acta Hydrobiologica Sin. 47, 1807–1815. doi: 10.7541/2023.2022.0497
Fennie H. W., Sponaugle S., Daly E. A., Brodeur R. D. (2020). Prey tell: what quillback rockfish early life history traits reveal about their survival in encounters with juvenile coho salmon. Mar. Ecol. Prog. Ser. 650, 7–18. doi: 10.3354/meps13300
Hegg J. C., Kennedy B. P., Chittaro P. M. (2018). What did you say about my mother? The complexities of maternally derived chemical signatures in otoliths. Can. J. Fish Aquat Sci. 76, 81–94. doi: 10.1139/cjfas-2017-0341
Hicks A. S., Closs G. P., Swearer S. E. (2010). Otolith microchemistry of two amphidromous galaxiids across an experimental salinity gradient: A multi-element approach for tracking diadromous migrations. J. Exp. Mar. Biol. Ecol. 394, 86–97. doi: 10.1016/j.jembe.2010.07.018
Houde E. D. (1997). Patterns and trends in larval-stage growth and mortality of teleost fish. J. Fish Biol. 51, 52–83. doi: 10.1111/j.1095-8649.1997.tb06093.x
Hüssy K., Limburg K. E., de Pontual H., Thomas O. R. B., Cook P. K., Heimbrand Y., et al. (2020). Trace element patterns in otoliths: the role of biomineralization. Rev. Fish Sci. Aquac. 29, 445–477. doi: 10.1080/23308249.2020.1760204
Islam M. R.-U., Schmidt D. J., Crook D. A., Hughes J. M. (2019). Non-diadromous life history and limited movement in northern lineages of Australian smelt: Evidence from otolith chemistry analysis. Ecol. Freshw. Fish. 28, 229–240. doi: 10.1111/eff.12446
Izzo C., Doubleday Z. A., Schultz A. G., Woodcock S. H., Gillanders B. M. (2015). Contribution of water chemistry and fish condition to otolith chemistry: comparisons across salinity environments. J. Fish Biol. 86, 1680–1698. doi: 10.1111/jfb.12672
Izzo C., Santos P. R., Gillanders B. (2018). Otolith chemistry does not just reflect environmental conditions: a meta-analytic evaluation. Fish Fish. 19, 441–454. doi: 10.1111/faf.12264
Janak J. M., Linley T. J., Harnish R. A., Shen S. D. (2021). Partitioning maternal and exogenous diet contributions to otolith 87Sr/86Sr in kokanee salmon (Oncorhynchus nerka). Can. J. Fish Aquat Sci. 78, 1146–1157. doi: 10.1139/cjfas-2020-0242
Jiang T., Liu H., Hu Y., Chen X., Yang J. (2022). Revealing population connectivity of the estuarine tapertail anchovy coilia nasus in the changjiang river estuary and its adjacent waters using otolith microchemistry. Fishes. 7, 147. doi: 10.3390/fishes7040147
Jiang T., Yang J., Lu M., Liu H., Chen T., Gao Y. (2017). Discovery of a spawning area for anadromous Coilia nasus Temminck et Schlegel 1846 in Poyang Lake, China. J. Appl. Ichthyol. 33, 189–192. doi: 10.1111/jai.13293
Kang Z., Song D., Li G., Yan L., Zhong X., Tang J., et al. (2022). Age identification for Larimichthys polyactis based on different sections of sagittal otolith and comparison with result of age backward inferring from growth equation. Mar. Sci. 44, 543–554. doi: 10.13233/j.cnki.mar.fish.2022.05.003
Kool J. T., Moilanen A., Treml E. A. (2013). Population connectivity: recent advances and new perspectives. Landscape Ecol. 28, 165–185. doi: 10.1007/s10980-012-9819-z
Li Y., Tang J., Xu X., Xu J., Liu Z., Xu H., et al. (2013). Comparison of otolith microstructures in small yellow croaker larvae and juveniles from Sanmen Bay and Lvsi. Mar. Fish. 35, 423–431. doi: 10.13233/j.cnki.mar.fish.2013.04.008
Lim H. K., Le M. H., An C. M., Kim S. Y., Park M. S., Chang Y. J. (2010). Reproductive cycle of yellow croaker Larimichthys polyactis in southern waters off Korea. Fish Sci. 76, 971–980. doi: 10.1007/s12562-010-0288-5
Lin S. H., Chang C. W., Iizuka Y., Tzeng W. N. (2007). Salinities, not diets, affect strontium/calcium ratios in otoliths of Anguilla japonica. J. Exp. Mar. Biol. Ecol. 341, 254–263. doi: 10.1016/j.jembe.2006.10.025
Lin N., Chen Y., Jin Y., Yuan X., Ling J., Jiang Y. (2018). Distribution of the early life stages of small yellow croaker in the Yangtze River estuary and adjacent waters. Fish Sci. 84, 357–363. doi: 10.1007/s12562-0181177-6
Liu H., Jiang T., Huang H., Shen X., Zhu J., Yang J. (2015). Estuarine dependency in Collichthys lucidus of the Yangtze River Estuary as revealed by the environmental signature of otolith strontium and calcium. Environ. Biol. Fish. 98, 165–172. doi: 10.1007/s10641-014-0246-7
Liu Z., Jin Y., Yang L., Yan L., Zhang Y., Xu M., et al. (2022). Incorporating egg-transporting pathways into conservation plans of spawning areas: An example of small yellow croaker (Larimichthys polyactis) in the East China Sea zone. Front. Mar. Sci. 9. doi: 10.3389/fmars.2022.941411
Loeppky A. R., Purchase C. F., Davoren G. K. (2018). Chemical signatures in embryonic otoliths of capelin, Mallotus villosus: Influence of family and environmental conditions. J. Exp. Mar. Biol. Ecol. 498, 25–31. doi: 10.1016/j.jembe.2017.10.007
Macdonald J. I., Crook D. A. (2010). Variability in Sr: Ca and Ba: Ca ratios in water and fish otoliths across an estuarine salinity gradient. Mar. Ecol. Prog. Ser. 413, 147–161. doi: 10.3354/meps08703
Marannu S., Nakaya M., Takatsu T., Takabatake S., Joh M., Suzuki Y. (2017). Otolith microstructure of Arabesque greenling Pleurogrammus azonus: A species with a long embryonic period. Fish Res. 194, 129–134. doi: 10.1016/j.fishres.2017.06.002
Mazloumi N., Doubleday Z. A., Gillanders B. M. (2017). The effects of temperature and salinity on otolith chemistry of King George whiting. Fish Res. 196, 66–74. doi: 10.1016/j.fishres.2017.08.010
Mohan J. A., Dewar H., Snodgrass O. E., Miller N. R., Tanaka Y., Ohshimo S., et al. (2022). Otolith geochemistry reflects life histories of Pacific bluefin tuna. PloS One. 17, e0275899. doi: 10.1371/journal.pone.0275899
Molina-Valdivia V., Bustos C. A., Castillo M. I., Search F. V., Plaza G., Landaeta M. F. (2021). Oceanographic influences on the early life stages of a mesopelagic fish across the Chilean Patagonia. Prog. Oceanogr. 195, 102572. doi: 10.1016/j.pocean.2021.102572
Mondal S., Chakrabarti R., Ghosh P. (2022). A multi-proxy (δ44/40Ca, Sr/Ca, and Δ47) study of fish otoliths for determination of seawater temperature. Chem. Geol. 605, 120950. doi: 10.1016/j.chemgeo.2022.120950
Morrissey S. J., Schlaefer J. A., Kingsford M. J. (2020). Experimental validation of the relationships between cubozoan statolith elemental chemistry and salinity and temperature. J. Exp. Mar. Biol. Ecol. 527, 151375. doi: 10.1016/j.jembe.2020.151375
Murayama E., Takagi Y., Nagasawa H. (2004). Immunohistochemical localization of two otolith matrix proteins in the otolith and inner ear of the rainbow trout, Oncorhynchus mykiss: comparative aspects between the adult inner ear and embryonic otocysts. Histochem. Cell Biol. 121, 155–166. doi: 10.1007/s00418-003-0605-5
Oh G. H., Kim Y. J., Kim W.-S., Hong C., Ji C. W., Kwak I.-S. (2022). Analysis of stomach contents of marine orgnaisms in gwangyang bay and yeosu fish market using DNA metabarcodin. g. Korean J. Environ. Ecol. 55, 368–375. doi: 10.11614/KSL.2022.55.4.368
Ospina-Alvarez A., Catalán I. A., Bernal M., Roos D., Palomera I. (2015). From egg production to recruits: Connectivity and inter-annual variability in the recruitment patterns of European anchovy in the northwestern Mediterranean. Prog. Oceanogr. 138, 431–447. doi: 10.1016/j.pocean.2015.01.011
Ovenden J. R. (2013). Crinkles in connectivity: combining genetics and other types of biological data to estimate movement and interbreeding between populations. Mar. Freshw. Res. 64, 201–207. doi: 10.1071/MF12314
Panfili J., Darnaude A. M., Vigliola L., Jacquart A., Labonne M., Gilles S. (2015). Experimental evidence of complex relationships between the ambient salinity and the strontium signature of fish otoliths. J. Exp. Mar. Biol. Ecol. 467, 65–70. doi: 10.1016/j.jembe.2015.03.007
Peek S., Clementz M. T. (2012). Ontogenetic variations in Sr/Ca and Ba/Ca ratios of dental bioapatites from Bos taurus and Odocoileus virginianus. J. Trace Elem. Med. Biol. 26, 248–254. doi: 10.1016/j.jtemb.2012.04.001
Pontual H. D., Lagardère F., Amara R., Bohn M., Ogor A. (2003). Influence of ontogenetic and environmental changes in the otolith microchemistry of juvenile sole (Solea solea). J. Sea Res. 50, 199–211. doi: 10.1016/S1385-1101(03)00080-7
Rodionov S. N. (2004). A sequential algorithm for testing climate regime shifts. Geophys. Res. Lett. 31, L09204. doi: 10.1029/2004GL019448
Rodionov S. N., Overland J. E. (2005). Application of a sequential regime shift detection method to the Bering Sea ecosystem. ICES J. Mar. Sci. 62, 328–332. doi: 10.1016/J.ICESJMS.2005.01.013
Ruttenberg B. I., Hamilton S. L., Warner R. R. (2008). Spatial and temporal variation in the natal otolith chemistry of a Hawaiian reef fish: prospects for measuring population connectivity. Can. J. Fish Aquat Sci. 65, 1181–1192. doi: 10.1139/f08-052
Saenger C., Cohen A. L., Oppo D. W., Hubbard D. (2008). Interpreting sea surface temperature from strontium/calcium ratios in Montastrea corals: Link with growth rate and implications for proxy reconstructions. Paleoceanography. 23, PA3102. doi: 10.1029/2007PA001572
Saito T., Shimizu I., Seki J., Nagasawa K. (2009). Relationship between zooplankton abundance and the early marine life history of juvenile chum salmon Oncorhynchus ketain eastern Hokkaido, Japan. Fish Sci. 75, 303–316. doi: 10.1007/s12562-008-0040-6
Santana F. M., Morize E., Labonne M., Lessa R., Clavier J. (2018). Connectivity between the marine coast and estuary for white mullet (Mugil curema) in northeastern Brazil revealed by otolith Sr: Ca ratio. Estuar. Coast. Shelf. S. 215, 124–131. doi: 10.1016/j.ecss.2018.09.032
Song D., Xiong Y., Jiang T., Yang J., Zhong X., Tang J. (2022b). Early life migration and population discrimination of the small yellow croaker Larimichthys polyactis from the Yellow Sea: inferences from otolith Sr/Ca ratios. Chin. J. Ocean Limnol. 40, 818–829. doi: 10.1007/s00343-021-1041-x
Song D., Xiong Y., Jiang T., Yang J., Zhong X., Tang J., et al. (2022a). ). Evaluation of spawning-and natal-site fidelity of larimichthys polyactis in the southern yellow sea using otolith microchemistry. Front. Mar. Sci. 8. doi: 10.3389/fmars.2021.820492
Starrs D., Ebner B. C., Fulton C. J. (2014). All in the ears: unlocking the early life history biology and spatial ecology of fishes. Biol. Rev. 91(1), 86–105. doi: 10.1111/brv.12162
Sturrock A. M., Hunter E., Milton J. A., Johnson R. C., Waring C. P., Trueman C. N. (2015). Quantifying physiological influences on otolith microchemistry. Methods Ecol. Evol. 6, 806–816. doi: 10.1111/2041-210X.12381
Sturrock A. M., Trueman C. N., Darnaude A. M., Hunter E. (2012). Can otolith elemental chemistry retrospectively track migrations in fully marine fishes? J. Fish Biol. 81, 766–795. doi: 10.1111/j.1095-8649.2012.03372.x
Sun Y., Xie Q. P., Lou B., He X., Zhou F. Q., Zhan W., et al. (2018). Effect of temperature on early growth stage in little yellow croaker, larimichthys polyactis. J. Zhejiang Ocean Univ. (Natural Science). 37, 208–214. doi: 10.3969/j.issn.1008-830X.2018.03.004
Svedäng H., Thunell V., Pålsson A., Wikström S. A., Whitehouse M. J. (2020). Compensatory feeding in eastern baltic cod (Gadus morhua): recent shifts in otolith growth and nitrogen content suggest unprecedented metabolic changes. Front. Mar. Sci. 7. doi: 10.3389/fmars.2020.00565
Tian H., Liu J., Cao L., Dou S. (2021). Temperature and salinity effects on strontium and barium incorporation into otoliths of flounder Paralichthys olivaceus at early life stages. Fish Res. 239, 105942. doi: 10.1016/j.fishres.2021.105942
Tomás J., Geffen A. J., Millner R. S., Pineiro C. G., Tserpes G. (2006). Elemental composition of otolith growth marks in three geographically separated populations of European hake (Merluccius merluccius). Mar. Biol. 148, 1399–1413. doi: 10.1007/s00227-005-0171-6
Tran N. T., Labonne M., Hoang. H. D., Panfili J. (2019). Changes in environmental salinity during the life of Pangasius krempfi in the Mekong Delta (Vietnam) estimated from otolith Sr: Ca ratios. Mar. Freshw. Res. 70, 1734–1746. doi: 10.1071/MF18269
Tulp I., Keller M., Navez J., Winter H. V., de Graaf M., Baeyens W. (2013). Connectivity between migrating and landlocked populations of a diadromous fish species investigated using otolith microchemistry. PloS One. 8, e69796. doi: 10.1371/journal.pone.0069796
Volk E. C., Blakley A., Schroder S. L., Kuehner S. M. (2000). Otolith chemistry reflects migratory characteristics of Pacific salmonids: Using otolith core chemistry to distinguish maternal associations with sea and freshwaters. Fish Res. 46, 251–266. doi: 10.1016/S0165-7836(00)00150-8
Walsh C. T., Gillanders B. M. (2018). Extrinsic factors affecting otolith chemistry—Implications for interpreting migration patterns in a diadromous fish. Environ. Biol. Fishes. 101, 905–916. doi: 10.1007/s10641-018-0746-y
Wei X., Zhang B., Shan X., Jin X., Ren Y. (2018). Feeding habits of small yellow croaker Larimichthys polyactis in the Bohai Sea. J. Fishery Sci. China. 25, 12891298. doi: 10.3724/SP.J.1118.2018.18163
Xiong Y., Liu H., Jiang T., Liu P., Tang J., Zhong X. (2015a). Investigation on otolith microchemistry of wild Pampus argenteus and Miichthys miiuy in the Southern Yellow Sea, China. Haiyang Xuebao. 37, 36–43. doi: 10.3969/j.issn.0253-4193.2015.02.004
Xiong Y., Yang J., Jiang T., Liu H., Zhong X. (2021). Temporal stability in the otolith Sr: Ca ratio of the yellow croaker, Larimichthys polyactis (Actinopterygii, Perciformes, Sciaenidae), from the southern Yellow Sea. Acta Ichthyol Piscat. 51, 59–65. doi: 10.3897/aiep.51.63245
Xiong Y., Yang J., Jiang T., Liu H., Zhong X., Tang J. (2017). Early life history of the small yellow croaker (Larimichthys polyactis) in sandy ridges of the South Yellow Sea. Mar. Biol. Res. 13, 993–1002. doi: 10.1080/17451000.2017.1319067
Xiong Y., Yang J., Zhang Z., Liu H., Jiang T., Chen T. (2015b). Factors affecting morphological development of the sagittal otolith in juvenile and adult small yellow croaker (Larimichthys polyactis Bleeker 1887). J. Appl. Ichthyol. 31, 1023–1028. doi: 10.1111/jai.12914
Xu M., Wang Y., Liu Z., Liu Y., Zhang Y., Yang L., et al. (2023). Seasonal distribution of the early life stages of the small yellow croaker (Larimichthys polyactis) and its dynamic controls adjacent to the Changjiang River Estuary. Fish Oceanogr. 32, 390–404. doi: 10.1111/fog.12635
Yang J., Jiang T., Liu H. (2011). Are there habitat salinity markers of the Sr: Ca ratio in the otolith of wild diadromous fishes? A literature survey. Ichthyol Res. 58, 291–294. doi: 10.1007/s10228-011-0220-8
Yatsu A., Mochioka N., Morishita K., Toh H. (1998). ). Strontium/Calcium ratios in statoliths of the neon flying squid, Ommastrephes bartrami (Cephalopoda), in the North Pacific Ocean. Mar. Biol. 131, 275–282. doi: 10.1007/s002270050320
Ying Y., Chen Y., Lin L., Gao T. (2011). Risks of ignoring fish population spatial structure in fisheries management. Can. J. Fish Aquat Sci. 68, 2101–2120. doi: 10.1139/f2011-116
Yu Y., Chen S. H., Tseng Y. H., Guo X., Shi J. I., Liu G., et al. (2020). Importance of diurnal forcing on the summer salinity variability in the east China sea. J. Phys. Oceanogr. 50, 633–653. doi: 10.1175/jpo-d-19-0200.1
Zhan W., Lou B., Chen R., Mao G., Liu F., Xu D., et al. (2016). Observation of embryonic, larva and juvenile development of small yellow croaker, Larimichthys polyactis. Oceanol Limnol Sin. 47, 1033–1039. doi: 10.11693/hyhz20160500114
Zimmerman C. E. (2005). Relationship of otolith strontium-to-calcium ratios and salinity: experimental validation for juvenile salmonids. Can. J. Fish Aquat Sci. 62, 88–97. doi: 10.1139/f04-182
Keywords: Larimichthys polyactis, otolith Sr : Ca ratios, early life stages, cultured and wild populations, environmental and physiological factors
Citation: Kang Z, Song D, Zhang H, Liang L, Zhang C, Jiang T, Zhu F and Xiong Y (2025) Exploring the drivers of otolith Sr/Ca during the early life stages of Larimichthys polyactis: insights from cultured and wild populations. Front. Mar. Sci. 12:1513070. doi: 10.3389/fmars.2025.1513070
Received: 17 October 2024; Accepted: 11 March 2025;
Published: 28 March 2025.
Edited by:
Mohammad Afzal Khan, Aligarh Muslim University, IndiaReviewed by:
Aafaq Nazir, The University of Tokyo, JapanCopyright © 2025 Kang, Song, Zhang, Liang, Zhang, Jiang, Zhu and Xiong. This is an open-access article distributed under the terms of the Creative Commons Attribution License (CC BY). The use, distribution or reproduction in other forums is permitted, provided the original author(s) and the copyright owner(s) are credited and that the original publication in this journal is cited, in accordance with accepted academic practice. No use, distribution or reproduction is permitted which does not comply with these terms.
*Correspondence: Ying Xiong, eXhpb25nc2hmdUAxMjYuY29t
†These authors share first authorship
Disclaimer: All claims expressed in this article are solely those of the authors and do not necessarily represent those of their affiliated organizations, or those of the publisher, the editors and the reviewers. Any product that may be evaluated in this article or claim that may be made by its manufacturer is not guaranteed or endorsed by the publisher.
Research integrity at Frontiers
Learn more about the work of our research integrity team to safeguard the quality of each article we publish.