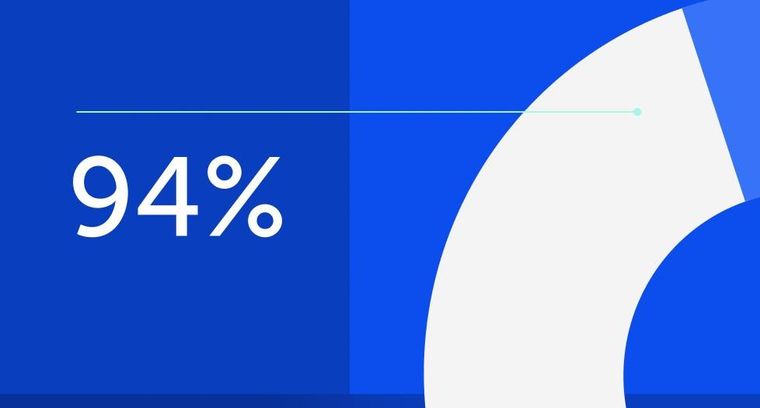
94% of researchers rate our articles as excellent or good
Learn more about the work of our research integrity team to safeguard the quality of each article we publish.
Find out more
ORIGINAL RESEARCH article
Front. Mar. Sci., 29 January 2025
Sec. Marine Ecosystem Ecology
Volume 12 - 2025 | https://doi.org/10.3389/fmars.2025.1503019
This article is part of the Research TopicTurning with the Tide and Time in the Salish Sea: Change in Estuary and Nearshore Habitats and Species Dependent on ThemView all 9 articles
Introduction: In late June 2021, the Pacific Northwest region of the United States and Canada experienced an unprecedented atmospheric heatwave that co-occurred with one of the lowest day-time tide series of the year. Several consecutive days of air temperatures 10-20°C above normal, coupled with mid-afternoon low tides proved deadly for many rocky intertidal organisms, which live at the margin of land and sea.
Methods: To assess short (weeks) and longer-term (1 year) impacts of the heatwave on rocky intertidal communities, we used long-term monitoring data collected annually at 16 sites throughout Washington State.
Results and discussion: Our findings indicate that impacts were most severe at sites within the Salish Sea region of WA, where peak low tides occurred during the hottest, mid-afternoon hours. Focal species assemblages at Olympic coast sites, where low tides occurred in the morning, were largely spared. In addition, while the heatwave was associated with substantial short-term changes in acorn barnacle, rockweed, and California mussel assemblages, lasting impacts (1 year) were only observed in the mussel assemblage at the one Salish Sea site where this species is common. These findings will aid in forecasting both short-term and longer lasting impacts of future heatwave events and help direct potential mitigation efforts to regions and species assemblages where impacts will likely be greatest.
The atmospheric heatwave that impacted the Pacific Northwest in late June 2021 was the most extreme on record for the region (Philip et al., 2021) and coincided perfectly with one of the lowest day-time tide series of the year (Raymond et al., 2022; White et al., 2023). This unlikely combination of extreme air temperatures and low tides proved to be deadly for intertidal communities in many areas of Washington, U.S.A and British Columbia, Canada, particularly those in the Salish Sea and Puget Sound regions, where peak low tides occurred during the hottest, mid-day hours for an entire week (Raymond et al., 2022, 2024). While intertidal organisms are adapted to thrive in an environment of wave shock, desiccation, and wide temperature fluctuations (e.g., Burrows et al., 2008; Lindegarth and Gamfeldt, 2005; Tomanek and Helmuth, 2002), this heatwave/low tide combination pushed organisms beyond their physical limits in many areas. Dead mussels, clams, barnacles, sea stars, anemones, and desiccated seaweeds were reported along seashores stretching from British Columbia to south Puget Sound, WA (Raymond et al., 2022; White et al., 2023).
Several studies have described the unusual and lasting intensity of the atmospheric heatwave event (Raymond et al., 2022; White et al., 2023), and others have documented initial impacts on individual species within soft sediment and rocky shore intertidal communities (Raymond et al., 2022; White et al., 2023; Raymond et al., 2024). To our knowledge, however, no study has examined the impacts of the event on communities as a whole, using a long-term (pre/post heatwave), spatially broad (including areas outside of the Salish Sea) approach. Ecological communities are not static; documenting the “normal” level of community change over a long time period allows impacts of a disturbance event, such as the 2021 heatwave, to be assessed against the “expected” bounds of community dynamics. Long-term patterns of community change can also be used to assess: (1) whether there is an expected community state and, (2) how long it will take an impacted community to recover, based on the trajectory toward that expected state.
The Multi-Agency Rocky Intertidal Network (MARINe) is a consortium of organizations collecting long-term monitoring data at sites along the entire west coast of North America, including 16 sites in Washington State, USA. MARINe data bring a geographically and temporally broad perspective to assessment of impacts and subsequent recovery following the 2021 heatwave, and the wealth of data and knowledge within the network allow for evaluation of: 1) short-term (weeks to months) and longer-term (1 year) impacts, 2) how location and timing of low tides influenced level of impact, and 3) how species’ life history traits affected recovery trends. This holistic approach is key to understanding the magnitude and lasting impact of disturbance events, such as the recent extreme atmospheric heatwave, on seashore communities.
Long-term monitoring data from the Multi-Agency Rocky Intertidal Network (MARINe; see pacificrockyintertidal.org) were used to quantify the impacts of the 2021 heatwave event on rocky intertidal communities. Using standardized protocols and a shared database, MARINe coordinates the collection of biological community-level survey data along the entire west coast of North America. Organizations using MARINe methods to monitor rocky intertidal communities at 16 sites throughout Washington State include Olympic National Park, Olympic Coast National Marine Sanctuary, Padilla Bay National Estuarine Research Reserve, University of California Santa Cruz, University of Washington, and Washington Department of Natural Resources, with support from the Makah Tribe, the Quinault Indian Nation, Feiro Marine Life Center, and the Puget Sound Restoration Fund. Annual surveys at most MARINe sites in Washington were completed prior to the late June 2021 heatwave event (see Supplementary Table S1 for survey dates) and emergency funds were secured to resurvey a subset of sites (8) within the Salish Sea immediately following the heatwave. Funding for these additional surveys was limited and thus the focus was on sites where impacts would likely be greatest. All sites were resurveyed in summer 2022.
MARINe sites in WA were established between 2008-2014 in areas of interest to partner organizations and are thus not evenly distributed (Figure 1). For temporal consistency among sites, only data collected in 2014-2022 were included in the analyses presented here. Note that using this subset of survey years precluded us from assessing potential impacts from another anomalous event, the 2014-2016 North Pacific Marine Heatwave because no “pre” data were included (but see Miner et al., 2021 for assessment of the 2014-2016 marine heatwave at a broader scale). Thus, the focus of this study was to assess potential impacts of the 2021 atmospheric heatwave event. Most sites consisted of contiguous (at least 30 meters along-shore), gently sloping rocky intertidal benches, with macrophyte and macroinvertebrate communities representative of the region. For the purposes of this assessment, sites were divided into two groups based on location: Olympic Coast and Salish Sea. It is important to note though, that one Salish Sea site, Freshwater Bay, is located in the Strait of Juan de Fuca, a transition zone between the two regions.
Figure 1. Location of MARINe long-term monitoring sites in the Salish Sea (red diamonds) and along the Olympic Coast (blue circles) of Washington. Weather station locations used for air temperatures are indicated by white triangles, and numbers correspond to station names as follows: 1) Bellingham Airport, 2) San Juan Island, English Camp, 3) Bremerton, 4) Cushman Power House #2, 5) Port Angeles Airport, 6) Ozette Ranger Station, 7) Quillayute Airport, 8) Kalaloch Ranger Station. Organizations managing weather stations include the National Weather Service Cooperative Observer Program (NWS-COOP), the National Park Service (NPS), the US Environmental Protection Agency Weather Bureau Army Navy (WBAN), and the U.S. Climate Reference Network (CRN).
The two regions differ greatly in many of the key attributes that shape rocky intertidal community structure. Olympic Coast sites are directly exposed to open ocean swell and wave shock, whereas sites within the Salish Sea experience much-reduced wave energy with wind chop and wake from boats constituting the primary source of waves (Dayton, 1971; Harley and Helmuth, 2003). While large waves on the outer coast and the debris they carry are a continuous source of disturbance, the resulting wave run-up and spray also extends the high “splash” zone, providing organisms protection from desiccation far above the tidal range (Lewis, 1964; Harley and Helmuth, 2003). Another key difference is the timing of the lowest tides during the warm summer months (Helmuth et al., 2002). Along the Olympic Coast, the lowest tides typically occur in the early morning hours, when air temperatures have not reached their peak and conditions are often overcast or foggy (Dayton, 1971). By contrast, the lowest summer tides within the Salish Sea tend to occur during the warmest mid-day hours. Air temperatures on the Olympic Coast are further moderated by an increased frequency of fog as compared to the Salish Sea region, where desiccation at low tide can be exacerbated by warm, offshore winds (Dayton, 1971; Harley and Helmuth, 2003). Specific site details, including photos, can be found on the MARINe website (https://marine.ucsc.edu/sites/index.html).
Long term monitoring plots were established for the following species assemblages at each site, where common, because of their important contributions to rocky intertidal community structure.
Acorn barnacles, comprised of a mixture of Chthamalus dalli and Balanus glandula, typically dominate the high zone of rocky intertidal shorelines, with those at the highest tidal heights spending more of their lives out of water than submerged. Acorn barnacles are relatively short-lived compared to many other intertidal species, with typical lifespans of 1-3 years (Morris et al., 1980). A single adult can release thousands of nauplius larvae, which spend 2-4 weeks in the water column and thus have the potential to settle far from their source population (Strathmann, 1987; Shanks et al., 2003; Shanks, 2009). Acorn barnacles are often among the first organisms to colonize newly available habitat (Paine and Levin, 1981). This group of barnacle species plays a key role in facilitating recruitment and survivorship of other species, particularly newly settled algae, by creating habitat that provides a refuge from desiccation and grazing (Farrell, 1991). These barnacles are also an important food source for several other species, including whelks and ochre stars (Paine, 1966; Dayton, 1971; Kozloff, 1993).
The northern rockweed, Fucus distichus, is an important foundation species (McKindsey and Bourget, 2001), particularly on sheltered shorelines where it tends to dominate the mid-intertidal zone. While some rockweed species can be extremely long-lived, F. distichus is thought to live on the order of 2-5 years (O’Clair and Lindstrom, 2000). The alga’s dense canopy serves as a food source for several species (Van Alstyne, 1988) and provides protection from desiccation for a suite of other algae and invertebrates during low tide. Northern rockweed is quite hardy, able to withstand high levels of thermal stress (Smolina et al., 2016), but dehydration can weaken thalli and increase mortality via stipe breakage (Haring et al., 2002). Exposure to air also results in reduced rates of photosynthesis (Dethier et al., 2005). Extreme dehydration, such as that experienced during the 2021 atmospheric heatwave event, can disrupt biochemical pathways, lyse cell membranes, and lead to death (O’Clair and Lindstrom, 2000). Because gametes drop from parent plants at low tide and resulting zygotes attach to the substrate soon after fertilization, dispersal is limited (Rice and Chapman, 1985). Thus, high mortality of northern rockweed could result in very slow recovery at the local scale.
The California mussel, Mytilus californianus, is a key foundation species on surf-exposed rocky shorelines, forming extensive beds in the mid-intertidal zone that provide shelter, habitat, and food for hundreds of other organisms (Paine, 1966; MacGinitie and MacGinitie, 1968; Kanter, 1980; Seed and Suchanek, 1992; Lohse, 1993; Smith et al., 2006). This species is long-lived, on the order of 10-20 years, thus creating a stable, three-dimensional structure for species living within the beds (Suchanek, 1992). Mussel clearing experiments indicate that recovery of beds can be slow (recovery times in Washington range from months to several years depending on patch size (Paine and Levin, 1981; Wootton, 2002; median recovery times in California > 3.5 years, Conway-Cranos, 2012) and larval supply (Vesco and Gillard, 1980; Paine and Levin, 1981; Conway-Cranos, 2012). As with acorn barnacles, California mussel larvae have a long planktonic period (9-45 days; Trevelyan and Chang, 1983; Strathmann, 1987) and likely travel long distances from source populations; thus, undisturbed shorelines can serve as source populations for disturbed areas if loss is patchy or regional. However, unlike barnacles that will settle on bare rock, California mussels settle preferentially on turf algae or amongst conspecific byssal threads (Trevelyan and Chang, 1983; Petersen, 1984).
At each site, five long-term fixed plots (50 × 75 cm) were located within each common, conspicuous, and ecologically important focal species assemblage, which generally formed distinct bands within the intertidal zone. Focal assemblages included acorn barnacles (Chthamalus dalli, Balanus glandula), California mussels (Mytilus californianus), and rockweeds (Fucus distichus). Each plot was initially placed in an area with maximal abundance of a given focal species. Due to differences outlined in “Site Descriptions” above, as well as funding availability limiting the number of plot types that could be consistently surveyed over the long-term, the number of focal assemblages varied among sites. In general, sites on the Olympic Coast contained acorn barnacle and California mussel plots, and sites within the Salish Sea contained acorn barnacle and rockweed plots. One exception was at Freshwater Bay, a Salish Sea site located within the Strait of Juan de Fuca, where acorn barnacle and California mussel but not rockweed plots were established.
During each survey, plots were photographed to create a visual archive of macroscopic species (Figure 2). Percent cover of all sessile species was quantified using a rectangular grid of 100 uniformly spaced points placed on top of each plot. Data collection was completed in the field for all surveys except at a handful of sites sampled immediately following the 2021 heatwave, where time and funding limitations meant that only photos of plots could be taken. At these five sites, percent cover was estimated in the lab by overlaying a grid of 100 points onto the plot photos. In all cases, the taxon or substrate occurring directly under each point was recorded and percent cover was calculated by dividing the total number of hits out of the total number of possible points. A comparison of data collected in the field vs the lab by Ambrose et al. (1995) found no significant difference in cover estimates as a function of these two methods. To ensure consistency between lab and field data, we lumped organisms that were difficult to identify to the species level from photos into higher taxonomic classifications and treated them as a single taxon in our analyses.
Figure 2. Example Fucus plot at Southeast Cove, Saddlebag Island, WA showing cover of rockweed and associated organisms pre-heatwave, 1 month post-heatwave, and 1 year post-heatwave.
While long-term monitoring plots were established within focal species “zones” that are in part, formed by differences in tidal height and associated emersion time, plot-specific tide height information was unfortunately not recorded at most sites. Because tide height was an important factor for interpreting results, we estimated tide heights for each focal species assemblage at each site using data from another MARINe survey type called Biodiversity Surveys. Biodiversity Surveys are done infrequently to complement annual fixed-plot surveys but occurred at nearly all Washington sites between 2022-2024. These surveys involve collecting point contact data at approximately 100 evenly spaced points along 11 sampling transects that run perpendicular to a 30 meter-long fixed baseline transect located in the very high intertidal zone. The 11 sampling transects stretch from the very high intertidal zone to the very low zone and are designed to capture the abundance and distribution of species throughout each site. Tide height data are also recorded along each sampling transect. Thus, Biodiversity data could be used to calculate the mean tide height values for C. dalli/B. glandula, F. distichus, and M. californianus assemblages at each site. While these values cannot be used to determine tidal heights for the fixed plots described above, they are representative of the tidal ranges at which each focal species was found at each site, which was important for estimating emersion time (see section 2.5 below).
To illustrate the anomalous nature of the heatwave event, daily maximum air temperature data were compiled for 2000-2022 from eight weather stations throughout WA (Figure 1). For each station, the daily maximum air temperature anomaly was calculated as the difference between the daily maximum for the year and the average daily maximum for the period 2000-2022, excluding 2021. The same was done for the daily maximum for 2021, creating two sets of anomalies: one for 2021 and the other for all other years. Both sets yielded an anomaly for all calendar days: the first was for 2021 relative to all other years, the second was the maximum anomaly for the period 2000-2022 excluding 2021 (Figure 3). For each station, the 2021 values are shown in red and the anomaly for the period 2000-2022, excluding 2021, is shown in blue.
Figure 3. Maximum daily air temperature anomalies for 2000-2022, excluding 2021 (blue) and 2021 (red) at eight weather station locations (see Figure 1). Arrows point to the highest values recorded at each location during the 2021 atmospheric heatwave event. See methods for more details.
Emersion time (aerial exposure) for rocky intertidal organisms is affected by three primary factors, the tide height at which an organism is located on the shore, the water level (tide cycle), and the local wave climate (wave run-up and splash). Abnormally hot air temperature events can be particularly stressful for intertidal organisms if periods of emersion (low tides) occur during midday hours (Harley, 2008). During the 2021 atmospheric heatwave event, the timing of daytime low tides varied by region; the earliest tides occurred each day at sites along the Olympic Coast and became progressively later moving eastward within the Strait of Juan de Fuca and still later further into the Salish Sea. As described in section 2.1 above, a similar gradient existed for wave climate, with wave energy generally highest along the exposed Olympic Coast and progressively dampening with distance into the more protected Salish Sea. To show how the timing of the low tides and wave climate affected emersion time during the 2021 heatwave for each species assemblage by region, we did the following: 1) Four MARINe sites located near four tide prediction stations were selected to represent variation in emersion time throughout the study area, 2) Water level (tide level) data were adjusted to incorporate effects of wave climate using wave height data from buoys located near the long-term monitoring sites, 3) Mean tide heights for each focal species assemblage were obtained from MARINe Biodiversity Survey data (see Section 2.3 above for methods), 4) Total emersion time between the hours of noon-8pm each day was calculated for each site/species assemblage combination (Figure 4). These hours were selected because temperatures climbed rapidly beginning around noon and stayed warm until around 8pm each day during the heatwave event. Tide height stations, wave height buoys, and MARINe sites were paired as listed in Table 1.
Figure 4. Estimates of total emersion times for each focal species assemblage between noon-8pm at 4 representative sites on the Olympic Coast, Strait of Juan de Fuca, and the Salish Sea. Technically part of the Salish Sea, the Strait of Juan de Fuca is a “transitional” area connecting the two regions. Calculations incorporate mean tide height values for each focal species assemblage at each site, water level (from tide prediction data), and mean wave height estimates. See Table 1 and methods for details. Note that the closest buoy to Post Point and Manchester with wave height data is located at the far east end of the Strait of Juan de Fuca and likely overestimates the wave climate at these two Salish Sea sites (thus, emersion time is likely underestimated).
Table 1. Information used to calculate emersion times shown in Figure 4 for focal species assemblages at four representative sites.
Patterns of change within acorn barnacle (Chthamalus/Balanus), rockweed (Fucus distichus), and California mussel (Mytilus californianus) zones were assessed using annual percent cover data from fixed plots in each of the three focal assemblages at Salish Sea and Olympic Coast sites. For each focal assemblage, mean community similarity values for plots at Salish Sea and Olympic Coast sites were displayed over time using non-metric Multi-Dimensional Scaling (nMDS). Using this approach allowed us to visually assess whether shifts in community similarity occurred immediately following the June 2021 heatwave (2021_H survey on nMDS plots) and whether communities had recovered by 2022. Species vector plots, displaying species groups that were the primary drivers of changes in community similarity calculations, were used to help interpret patterns over time. The contribution of each species to community composition is indicated by the direction and length of each ray. Species listed at the ends of rays correlate with a Pearson’s r value of at least 0.5 (range: 0.5–1) in the direction of the ray for acorn barnacle and California mussel plots, and 0.75 for rockweed plots. To help interpret whether post-heatwave shifts in community similarity fell outside of the “expected” range based on long-term patterns of change, pairwise community similarity values and associated 95% confidence intervals were calculated between successive years for the “pre-heatwave” period (e.g., 2017 vs 2018), the immediate pre/post period (2021 vs 2021_H), and the 1-year pre/post period (2021 vs 2022) for each species assemblage at Olympic Coast and Salish Sea sites.
The 2021 atmospheric heatwave was an impressively anomalous event, with daily maximum air temperatures far exceeding all others recorded across a 23-year period at most of the eight weather stations examined (Figure 3). These record-breaking temperatures occurred across multiple days, during one of the lowest tide series of the year, and initial observations suggested that rocky intertidal communities might suffer severe and lasting impacts. However, our long-term monitoring data show that while the heatwave event was correlated with shifts in community similarity and species composition within rocky intertidal communities in Washington, USA, the severity and duration of impact varied substantially depending on two key factors, site location and species’ life history, discussed in detail below.
Quantitative data are not available for most Olympic Coast sites immediately following the heatwave event, but qualitative observations revealed little to no evidence of die-offs similar to those documented at Salish Sea sites (Miner and Fradkin, pers. obs.). This regional difference in assemblage response is likely due to the timing of the low tides during the several-day heatwave event. Within the Salish Sea, peak low tides occurred during mid-day and focal species assemblages were emersed when air temperatures were at their hottest (Figure 4). By contrast, peak low tides at sites on the Olympic Coast were in the early morning hours, when temperatures were cooler; afternoon emersion times were comparatively much lower (Figure 4). Timing of low tides in the Strait of Juan de Fuca, which connects the Salish Sea to the Pacific Ocean, and where Freshwater Bay is located, were in between the Olympic Coast region and areas further within the Salish Sea. This difference in the timing of low tides meant that intertidal organisms within the Salish Sea and Strait of Juan de Fuca regions experienced both more extreme temperatures and cumulatively longer aerial exposure over the multi-day heatwave event, as compared to those on the Olympic Coast (Figure 4; Helmuth, 1998). It is important to note that because wave height data were not available from most buoys within the Salish Sea, emersion time estimates for the two easternmost sites, Post Point and Manchester use data from the New Dungeness buoy, located at the eastern end of the Strait of Juan de Fuca. Wave heights at these two Salish Sea sites were almost certainly lower than those recorded at the New Dungeness buoy, and thus emersion times shown in Figure 4 are likely underestimates.
To understand the patterns and severity of change in rocky intertidal communities at Olympic Coast and Salish Sea sites resulting from the 2021 heatwave, it is important to look at both the direction in which communities shifted and the species associated with those shifts (Figures 5–7). Long-term patterns of community similarity displayed using nMDS plots suggest that the 2021 heatwave caused substantial, but largely short-term shifts in community structure at MARINe sites within the Salish Sea. Similar shifts were not observed within rocky intertidal communities at Olympic Coast sites but note that surveys immediately following the extreme weather event (labeled 2021-H in Figures 5-7) were rare in this region. Comparisons of community similarity between successive years in the pre-heatwave period, the short-term pre/post period (2021 vs. 2021_H) and year-long pre/post period (2021 vs. 2022) indicate that post-heatwave shifts in acorn barnacle and rockweed assemblages were not larger than “expected”, based on confidence intervals of one period overlapping mean values for comparison periods (Figure 8), likely because there is a fair amount of natural variability in these communities. Community similarity patterns for most plot types in both regions suggest that communities had largely recovered by summer 2022. The one exception is the California mussel assemblage at Freshwater Bay, the only Salish Sea site where this species is common. Here, mussel cover had not returned to pre-heatwave levels as of summer 2022, and indeed, loss of mussels continued into 2023 (see Supplementary Figure S1, California mussel plots).
Figure 5. (A) nMDS plot showing shifts in community similarity (Bray-Curtis) over time within acorn barnacle (Chthamalus dalli/Balanus glandula) plots at all Salish Sea sites (red) and Olympic Coast sites (blue). Survey years are labeled and 2021_H (Salish Sea sites only) represents the post-June 2021 heatwave survey. (B) Primary species driving the patterns of change over time (≥0.5 correlation). Species contributing the most (longest vectors in 5B) to observed patterns in 5A are in bold font. Note that positive vectors indicating increase in cover for a given species are shown, but loss of a species can be inferred by assemblage shifts in the direction of corresponding, but not shown, negative vectors. A star symbol in (A) indicates where the centroid for species vectors in (B) would be located if the two plots were merged.
Figure 6. (A) nMDS plot showing shifts in community similarity (Bray-Curtis) over time within rockweed (Fucus distichus) plots at all Salish Sea sites. Survey years are labeled and 2021_H represents the post-June 2021 heatwave survey. (B) Primary species driving the patterns of change over time (>0.75 correlation). Species contributing the most [longest vectors in (B)] to observed patterns in (A) are in bold font. Note that positive vectors indicating increase in cover for a given species are shown, but loss of a species can be inferred by assemblage shifts in the direction of corresponding, but not shown, negative vectors. A star symbol in (A) indicates where the centroid for species vectors in (B) would be located if the two plots were merged.
Figure 7. (A) nMDS plot showing shifts in community similarity (Bray-Curtis) over time within California mussel (Mytilus californianus) plots at Freshwater Bay (red), the only Salish Sea site where M. californianus is common and Olympic Coast sites (blue). (B) Primary species driving the patterns of change over time (≥0.5 correlation). Survey years are labeled and 2021_H represents the post-June 2021 heatwave survey. Note that the 2021_H survey at Freshwater Bay is drastically dissimilar from all other region/year combinations and plots off of the page (red lines to right). A star symbol in (A) indicates where the centroid for species vectors in (B) would be located if the two plots were merged.
Figure 8. Pairwise comparisons of community similarity between successive surveys in the pre-heatwave period of 2014-2020 (e.g., 2018 vs. 2019, “Pre”), 2021 vs. 2021 immediately post-heatwave or “short-term” (“Post-ST”), and 2021 vs. 2022 or “long-term” (“Post-LT”). 95% confidence intervals are included where data from multiple surveys were available.
Within acorn barnacle assemblages, Salish Sea sites experienced a die-off of several species and a corresponding increase in bare space between pre- (2021 survey, Figure 5A) and post-heatwave (2021-H) surveys, although the magnitude of change was not outside the “expected” range based on long-term survey data (Figure 8). Note though, that the magnitude of barnacle assemblage change considered “normal” is based on year-to-year differences because sites are sampled annually each summer. The 2021 to 2021_H shift occurred over just weeks, suggesting that this was a large shift for such a short time period. The 2021_H surveys were done in July and early August (Supplementary Table S1), later in the summer than annual monitoring is typically done. Thus, it is possible that assemblage shifts could be explained, in part, by changes that are “normal” for this later summer period. However, the types of changes documented suggest that heat stress and desiccation were the likely causes of change in the barnacle assemblage. Primary drivers of change as evidenced by vector length and direction in Figure 5B include loss in cover of acorn barnacles (Chthamalus/Balanus) and rockweed (Fucus), and gain in cover of bare rock, dead barnacles, and non-coralline crustose algae, as would be expected with die-back of the foliose phase of several algal species. Communities appear to have completely recovered by the following summer (2022). Barnacle assemblages at Olympic Coast sites were not surveyed quantitatively immediately following the heatwave, but qualitative observations suggest that die-offs were minimal compared to Salish Sea sites (Miner and Fradkin, pers. obs.), and surveys in summer 2022 indicate no evidence of long-term impact.
Rockweed assemblages at Salish Sea sites changed between pre- and post-heatwave surveys, largely driven by a loss in cover of rockweed and a gain in cover of sessile invertebrates, including acorn barnacles (Figures 6A, B), but as with the acorn barnacle assemblage, the magnitude of change was not outside of the “expected” range based on pre- and immediate post- 2021 heatwave survey comparisons (see red bar Figure 8). Because this analysis only included top-layer points (no understory layers) it is likely that the “gain” in cover of sessile invertebrates is due to a loss of rockweed canopy cover, which exposed the underlying species (see Figure 2 for example photos). Somewhat surprisingly, percent cover of rockweed had returned to pre-heatwave levels after just 1 year in summer 2022. Rockweed communities are only surveyed at Salish Sea sites, so we cannot make comparisons of impacts between the two regions.
California mussel assemblage dynamics at Olympic Coast sites pre-/post-heatwave were well within the range of expected levels of change, suggesting little to no impact (Figure 7A). By contrast, at Freshwater Bay, the only Salish Sea site where the California mussel assemblage is surveyed, significant loss of mussels occurred during the heatwave (Figures 7A, B, 8). The post-heatwave (2021-H) survey was so drastically dissimilar from all other survey periods that its symbol on the nMDS plot is off the page (Figure 7A). This change was due almost entirely to high cover of dead mussels that had been killed during the heatwave but were still attached to the substrate by their byssal threads (Figures 7B, 9). Similarity values for the mussel assemblage at Freshwater Bay in 2022 suggest substantial recovery (Figure 7A), but this pattern is misleading for two reasons: 1) the dead mussels that were primarily responsible for high dissimilarity between 2021_H and all other surveys had disappeared by 2022, and 2) the apparent increase in live mussel cover between the 2021 post-heatwave survey and the 2022 survey was due to reorientation of surviving mussels—no new mussels recruited to the plots, as evidenced by an absence of small individuals in 2022. The overall structure of the mussel bed shifted from expansive, tightly packed beds to sparse clusters or single individuals (see example plot photos, Figure 9). In order to more securely attach to the substrate and reduce chances of dislodgement, surviving mussels shifted from a vertical to a horizontal orientation relative to the substrate, thus increasing the surface area to which byssal threads could attach to the rock. This reorientation means that the potential area sampled for an individual mussel using our point contact method increased between pre- and post-heatwave surveys, thus inflating our estimate of “recovery”. Because of this, our percent cover data does not accurately capture the “true” and lasting impacts of the 2021 heatwave.
Figure 9. Example Mytilus californianus plot from Freshwater Bay, WA showing cover of live and dead mussels and associated organisms pre-heatwave, 1 month post-heatwave, and 1 year post-heatwave.
The post-heatwave recovery of acorn barnacle communities after just one year can likely be attributed to the ability of this species complex to quickly colonize newly available substrate. Acorn barnacles that survived the heatwave (e.g., in protected microhabitats or at lower tidal elevations) had the potential to produce an abundance of larvae capable of traveling long distances and settling on substrate available post-heatwave. This ability to disperse widely and grow rapidly makes acorn barnacles quite resilient to disturbance (Conway-Cranos, 2012).
Loss of rockweed cover in both acorn barnacle plots and rockweed plots was also short-term, with community similarity trajectories and associated species vectors suggesting recovery after just one year (Figures 5, 6). It is important to note though, that canopy cover can be highly variable under “normal” conditions, as evidenced by mean community similarity values in 2015 and 2016, both low rockweed cover years. In an experimental study of rockweed recovery rates in Kachemak Bay, AK, percent cover varied +/- 30% around the long-term mean within plots over a 12-year period (Klinger and Fukuyama, 2011). Unlike acorn barnacle larvae, rockweed zygotes do not travel far from parent thalli. Thus, it is likely that enough rockweed survived the heatwave at Salish Sea sites to allow for regrowth and repopulation at the local scale. Rockweed has been described as “legendary” in its ability to withstand desiccation during low tide, but extreme dehydration can result in mortality via cell death and dry, brittle fronds are susceptible to breakage (O’Clair and Lindstrom, 2000; Haring et al., 2002). If loss of rockweeds had been more extensive, recovery trajectories would almost certainly have been much slower.
The most extreme pre/post community similarity shifts occurred in plots targeting California mussels at Freshwater Bay, the only Salish Sea site where this species is common. This was also the only assemblage with impacts lasting beyond 1 year (Figures 7 , 9). Like acorn barnacles, California mussels have planktonic larvae that can travel long distances, and there is the potential for new recruits to arrive from unimpacted populations on the Olympic coast. However, whereas acorn barnacles will settle on newly exposed bare rock, California mussels have a strong preference for settling on turf algae or among other mussels (Petersen, 1984). Thus, assemblage changes associated with extreme heatwave events could reduce preferred settlement habitat for mussels. In addition, California mussels are much longer-lived and slower-growing than acorn barnacles, so even if successful recruitment occurs, it will be several years before mature, dense mussel beds return.
The shift in the structure of the mussel assemblage at Freshwater Bay, from a densely-packed bed to isolated individuals or small clusters, has repercussions for both the mussels themselves, and the numerous species that depend on them for habitat. The post-heatwave, prostrate orientation of most surviving mussels means that they have more surface area exposed to solar radiation and are no longer afforded the protection from desiccation that being in a tightly packed bed provides. Helmuth (1998) estimated that living in an aggregation decreases the solar radiation a mussel is exposed to by up to 40%, and showed that mussels living in beds had, on average, markedly lower body temperatures compared to solitary individuals. Thus, mussels that survived the heatwave event could now experience greater stress from future warm weather events that previously might have posed little to no threat. Loss of mussels also creates patches of open space, which can expose remaining individuals to increased hydrodynamic wave force (Denny, 1987; Bell and Gosline, 1997). Once a patch is formed, it is not uncommon for subsequent waves to enlarge it (e.g., Dayton, 1971; Denny, 1987; Guichard et al., 2003), thereby resulting in additional mussel mortality. Reduced mussel density also means less interstitial space and reduced three-dimensional surface for organisms that depend on this foundation species for habitat and protection from desiccation (Seed and Suchanek, 1992; Suchanek, 1992). These multi-faceted effects of California mussel loss resulting from the 2021 heatwave event could have long-lasting ramifications for assemblage recovery.
Heat-related mass mortality of Mytilus californianus has been documented in past studies (see Harley, 2008 and references therein). Harley (2008) identified excessive heat as the cause of mussel mortality at one site in California. Specifically, mussels on substrate directly facing the sun during mid-day hours at low tide experienced much higher levels of mortality compared to those angled away from the sun. Somewhat surprisingly, intertidal height (and associated differences in emersion times) was not correlated with mussel death, suggesting that desiccation and cumulative exposure time to high temperatures might be less important in explaining mortality patterns than maximum body temperature (Harley, 2008). Quantifying substrate orientation within MARINe fixed plots was outside the scope of this study but could add substantial explanatory power to future heatwave impact forecasting and assessment efforts.
Other studies have attempted to quantify the loss of mussels at Salish Sea sites that can be attributed to the 2021 heatwave event (Raymond et al., 2022; White et al., 2023). It is important to note though, that these estimates were for bay mussels, a species complex largely composed of Mytilus trossulus in the Salish Sea, but also including M. edulis and M. galloprovincialis. Bay mussels are quite different from California mussels in terms of their life history and the role they play in rocky intertidal communities in Washington (Suchanek, 1981). Bay mussels can recruit in huge numbers, appearing as black specks carpeting rocky intertidal shores and other hard substrates. However, their thinner shells (relative to California mussels; Suchanek, 1981) make them a favorite food for many predators, including whelks and sea stars (Dayton, 1971; Wootton, 2002), and they often disappear within months of settlement (Miner, pers. obs.). Even when they escape predation, bay mussels are relatively short-lived (on the order of a few years, Suchanek, 1981), and thus do not form the stable and complex habitat that makes California mussels a key foundation species.
Perhaps the most surprising finding from this study was that rocky intertidal communities at all but one of the 16 MARINe long-term monitoring sites in Washington appeared to fully recover within just 1 year following the June 2021 extreme atmospheric heatwave event. Physical disturbance is a common process affecting rocky intertidal communities and plays a key role in shaping this system (e.g., Dayton, 1971; Paine and Levin, 1981). Forms of disturbance such as battering by drift logs or winter storm and wave damage tend to be patchy in nature, leaving substantial proportions of the affected communities intact. Surviving organisms can thus provide a local source of propagules that recruit to and recover disturbed areas. Heat-related disturbance events can be similarly patchy in nature. While the focus of this study was on the broad-scale processes affecting survivorship of organisms, local factors likely ameliorate the impacts of a large-scale heatwave event, resulting in a patchwork of survivors.
The ability of intertidal organisms to survive heat-stress events is highly influenced by heterogeneity in the physical environment (Harley and Helmuth, 2003; Harley, 2008). While substantial mortality was broadly associated with the 2021 heatwave event (this study; White et al., 2023; Raymond et al., 2022), at the individual organism scale, maximum body temperatures were likely highly variable due to the complex combination of localized contributing factors. Wave climate, including direction, force, run-up, and splash can vary substantially at very localized scales (e.g., Dayton, 1971; Denny et al., 2004) and greatly affect aerial exposure time. Other factors contributing to stress during periods of anomalously warm air temperatures include shading, angle relative to the sun, rock type, wind velocity and localized weather such as cloud cover and fog (e.g., Tomanek and Helmuth, 2002; Harley and Helmuth, 2003; Harley, 2008). These factors likely mitigated the effects of the 2021 heatwave event at a local scale, resulting in mortality that was patchy in nature (no local extirpation of focal species was observed). Surviving individuals could then contribute larvae and/or propagules, leading to rapid repopulation and recovery of disturbed areas.
The rapid recovery of most communities in the present study underscores the impressive resilience of the rocky intertidal system. However, atmospheric heatwave events differ from other common sources of disturbance to this system in one important way—they have the potential for much broader-scale impact. Extreme heatwave events could result in large “dead zones” that might overwhelm the capacity for recovery via propagules from surviving individuals. Under current climate conditions, the likelihood of occurrence for another atmospheric heatwave event in the Pacific Northwest region of the same intensity as the June 2021 event is estimated at once in 1,000-years (Philip et al., 2021). If true, rocky intertidal communities at all sites in Washington should recover fully to resemble those of the pre-heatwave period. However, under a scenario of 2°C of global warming, the predicted frequency of extreme atmospheric heatwave events increases to roughly every 5-10 years (Philip et al., 2021). The compounded effects of increasingly common heatwaves would almost certainly result in higher overall mortality, larger gaps between surviving populations (propagule sources), and less time between events for recovery. Severe, widespread, and increasingly frequent climate change-driven disturbance events have been linked to fundamental shifts in marine ecosystems, particularly in tropical regions (Babcock et al., 2019; Hughes et al., 2019; Donovan et al., 2021). The 2021 heatwave should serve as a warning that temperate regions are not immune from these extreme events, and species that are slow growing, such as California mussels, or those with limited dispersal, such as rockweeds, are at particular risk. Declines or loss of these key foundation species would have profound cascading effects, as numerous intertidal organisms depend on them for habitat, shelter, and food.
This study highlights the importance of broadscale, long-term monitoring as a key tool for assessing damage from disturbance events and estimating recovery rates within the context of long-term patterns of change. This is particularly true for highly dynamic rocky intertidal systems, which are subjected to numerous stressors on daily and seasonal cycles. Continued MARINe monitoring at established sites in WA will allow for assessment of the lingering impacts of the 2021 heatwave event and provide critical data for documenting impacts from future events. As of 2024, MARINe partners in WA have begun collecting drone survey data from shoreline habitat adjacent to long-term monitoring sites, to more broadly document the distribution and abundance of key foundation species. Geographically expansive drone surveys in combination with more focused and detailed biological survey data will inform forecasting tools and allow for better predictions of when and where impacts might be greatest. This information could, in turn, be used to develop and deploy potential mitigation efforts where impacts might be most severe.
The datasets presented in this study can be found in online repositories. The names of the repository/repositories and accession number(s) can be found below: https://data.piscoweb.org/metacatui/data. Data can also be accessed from the MARINe website via our data request form (marine.ucsc.edu/explore-the-data/contact/data-request-form.html).
The manuscript presents research on animals that do not require ethical approval for their study.
CMM: Conceptualization, Data curation, Funding acquisition, Investigation, Methodology, Project administration, Supervision, Visualization, Writing – original draft, Writing – review & editing. HDB: Conceptualization, Funding acquisition, Investigation, Project administration, Resources, Supervision, Writing – review & editing. HB: Conceptualization, Funding acquisition, Investigation, Project administration, Resources, Supervision, Visualization, Writing – review & editing. MD: Conceptualization, Funding acquisition, Investigation, Project administration, Resources, Supervision, Writing – review & editing. SF: Conceptualization, Data curation, Funding acquisition, Investigation, Project administration, Resources, Supervision, Writing – review & editing. RG: Data curation, Writing – review & editing. WR: Conceptualization, Writing – review & editing. PR: Conceptualization, Formal analysis, Funding acquisition, Investigation, Methodology, Project administration, Resources, Supervision, Visualization, Writing – review & editing.
The author(s) declare financial support was received for the research, authorship, and/or publication of this article. Annual, long-term monitoring surveys were supported by programmatic funding from Olympic Coast National Marine Sanctuary, Padilla Bay National Estuarine Research Reserve, and Washington Department of Natural Resources. Olympic National Park surveys were supported by funding from the National Park Service Inventory and Monitoring Division. Post-heatwave surveys of Salish Sea sites were supported by a program development grant to PTR from Washington Sea Grant, University of Washington, pursuant to NOAA Award NA18OAR4170095. The views expressed herein are those of the authors and do not necessarily reflect the views of NOAA or any of its sub-agencies. Management of the MARINe database has been primarily supported by the Bureau of Ocean Energy Management, National Parks Service, the David and Lucile Packard Foundation, and US Navy.
We thank Jenny Waddell, Katie Wrubel, and the Olympic Coast National Marine Sanctuary for supporting MARINe surveys at Kydikabbit and Point Grenville. We are grateful to the Makah Tribe and the Quinault Indian Nation for access to their lands. Padilla Bay National Estuarine Research Reserve provided field support and staff, including Washington Conservation Corps interns, to assist with surveys on Saddlebag and Hat Islands. We thank Hilary Hayford and Caitlin O’Brien from Puget Sound Restoration Fund for assistance with surveys at Manchester State Park. This work would not have been possible without the researchers and many volunteers who contributed to the collection of data at MARINe sites in Washington, including Helle Anderson, Bill Baccus, Barb Blackie, Betty Bookheim, Nicole Burnett, Dan Cannon, Tish Conway-Cranos, Tamara Galvan, Nicole Harris, Megan Juran, Hazel Levine, Ben Miner, Ann Raymond, Amelia Ritger, Suzanne Shull, Christi and Gavin Truckenmiller, Rachel Weston, Susan Wood, and Sylvia Yang. We are grateful for comments and thoughtful suggestions from Dr. Emily Grason and Dr. Eric Sanford, which greatly improved the manuscript. Data for this study were collected as part of the Multi-Agency Rocky Intertidal Network (MARINe): a long-term ecological consortium funded and supported by many groups. Please visit pacificrockyintertidal.org for a complete list of the MARINe partners responsible for monitoring and funding these data.
The authors declare that the research was conducted in the absence of any commercial or financial relationships that could be construed as a potential conflict of interest.
The author(s) declare that no Generative AI was used in the creation of this manuscript.
All claims expressed in this article are solely those of the authors and do not necessarily represent those of their affiliated organizations, or those of the publisher, the editors and the reviewers. Any product that may be evaluated in this article, or claim that may be made by its manufacturer, is not guaranteed or endorsed by the publisher.
The Supplementary Material for this article can be found online at: https://www.frontiersin.org/articles/10.3389/fmars.2025.1503019/full#supplementary-material
Ambrose R. F., Engle J. M., Raimondi P. T., Wilson M., Altstatt J. A. (1995). Rocky intertidal and subtidal resources: Santa Barbara County mainland [Final Report to the Minerals Management Service. (No. OCS Study MMS 95-0067)].
Babcock R. C., Bustamante R. H., Fulton E. A., Fulton D. J., Haywood M. D. E., Hobday A. J., et al. (2019). Severe continental-scale impacts of climate change are happening now: extreme climate events impact marine habitat forming communities along 45% of Australia’s coast. Front. Mar. Sci. 6. doi: 10.3389/fmars.2019.00411
Bell E. C., Gosline J. M. (1997). Strategies for life in flow: tenacity, morphometry, and probability of dislodgement of two Mytilus species. Mar. Ecol. Prog. Ser. 159, 197–208. doi: 10.3354/meps159197
Burrows M. T., Harvey R., Robb L. (2008). Wave exposure indices from digital coastlines and the prediction of rocky shore community structure. Mar. Ecol. Prog. Ser. 353, 1–12. doi: 10.3354/meps07284
Conway-Cranos L. L. (2012). Geographic variation in resilience: an experimental evaluation of four rocky intertidal assemblages. Mar. Ecol. Prog. Ser. 457, 67–83. doi: 10.3354/meps09715
Dayton P. K. (1971). Competition, disturbance, and community organization: the provision and subsequent utilization of space in a rocky intertidal community. Ecol. Monogr. 41, 351–389. doi: 10.2307/1948498
Denny M. W. (1987). Lift as a mechanism of patch initiation in mussel beds. J. Exp. Mar. Biol. Ecol. 113, 231–245. doi: 10.1016/0022-0981(87)90103-1
Denny M. W., Helmuth B., Leonard G. H., Harley C. D. G., Hunt L. J. H., Nelson E. K. (2004). Quantifying scale in ecology: lessons from a wave-swept shore. Ecol. Monogr. 74, 513–532. doi: 10.1890/03-4043
Dethier M. N., Williams S. L., Freeman A. (2005). Seaweeds under stress: manipulated stress and herbivory affect critical life-history functions. Ecol. Monogr. 75, 403–418. doi: 10.1890/03-4108
Donovan M. K., Burkepile D. E., Kratochwill C., Shlesinger T., Sully S., Oliver T. A., et al. (2021). Local conditions magnify coral loss after marine heatwaves. Sci. (American Assoc. Advancement Science) 372, 977–980. doi: 10.1126/science.abd9464
Farrell T. M. (1991). Models and mechanisms of succession: an example from a rocky intertidal community. Ecol. Monogr. 61, 95–113. doi: 10.2307/1943001
Guichard F., Halpin P. M., Allison G. W., Lubchenco J., Menge B. A. (2003). Mussel disturbance dynamics: signatures of oceanographic forcing from local interactions. Am. Nat. 161, 889–904. doi: 10.1086/375300
Haring R. N., Dethier M. N., Williams S. L. (2002). Desiccation facilitates wave-induced mortality of the intertidal alga. Fucus gardneri. Mar. Ecol. Prog. Ser. 232, 75–82. doi: 10.3354/meps232075
Harley C. D. G. (2008). Tidal dynamics, topographic orientation, and temperature-mediated mass mortalities on rocky shores. Mar. Ecol. Prog. Ser. 371, 37–46. doi: 10.3354/meps07711
Harley C. D. G., Helmuth B. S. T. (2003). Local- and regional-scale effects of wave exposure, thermal stress, and absolute versus effective shore level on patterns of intertidal zonation. Limnology Oceanography 4. doi: 10.4319/lo.2003.48.4.1498
Helmuth B. S. T. (1998). Intertidal mussel microclimates: predicting the body temperature of a sessile invertebrate. Ecol. Monogr. 68, 51–74.
Helmuth B., Harley C. D. G., Halpin P. M., O’Donnell M., Hofmann G. E., Blanchette C. A. (2002). Climate change and latitudinal patterns of intertidal thermal stress. Science 298, 1015–1017. doi: 10.1126/science.1076814
Hughes T. P., Kerry J. T., Connolly S. R., Baird A. H., Eakin C. M., Heron S. F., et al. (2019). Ecological memory modifies the cumulative impact of recurrent climate extremes. Nat. Climate Change 9, 40–43. doi: 10.1038/s41558-018-0351-2
Kanter R. G. (1980). “Biogeographic patterns in mussel community distribution from the southern California Bight,” in The California Islands: proceedings of a multidisciplinary symposium. Ed. Power D. M. (Santa Barbara Museum of Natural History, Santa Barbara, California), 341–355.
Klinger T., Fukuyama A. K. (2011). Decadal-scale dynamics and response to pulse disturbance in the intertidal rockweed Fucus distichus (Phaeophyceae). Mar. Ecol. 32, 313–319. doi: 10.1111/j.1439-0485.2011.00481.x
Kozloff E. N. (1993). Seashore life of the northern Pacific coast: an illustrated guide to northern California, Oregon, Washington, and British Columbia (Seattle: University of Washington Press).
Lindegarth M., Gamfeldt L. (2005). Comparing categorical and continuous ecological analyses: effects of “wave exposure” on rocky shores. Ecology 86, 1346–1357. doi: 10.1890/04-1168
Lohse D. P. (1993). The importance of secondary substratum in a rocky intertidal community. J. Exp. Mar. Biol. Ecol. 166, 1–17. doi: 10.1016/0022-0981(93)90075-Y
McKindsey C. W., Bourget E. (2001). Diversity of a northern rocky intertidal community: the influence of body size and succession. Ecology 82, 3462–3478. doi: 10.1890/0012-9658(2001)082[3462:DOANRI]2.0.CO;2
Miner C. M., Burnaford J. L., Ammann K., Becker B. H., Fradkin S. C., Ostermann-Kelm S., et al. (2021). Latitudinal variation in long-term stability of North American rocky intertidal communities. J. Anim. Ecol. 90, 2077–2093. doi: 10.1111/1365-2656.13504
Morris R. H., Abbott D. L., Haderlie E. C. (1980). Intertidal invertebrates of California (Stanford: Stanford University Press).
Paine R. T. (1966). Food web complexity and species diversity. Amer Nat. 100, 65–75. doi: 10.1086/282400
Paine R. T., Levin S. A. (1981). Intertidal landscapes: disturbance and the dynamics of pattern. Ecol. Monogr. 51, 145–178. doi: 10.2307/2937261
Petersen J. H. (1984). Larval settlement behavior in competing species: Mytilus californianus Conrad and M. edulis L. J. Exp. Mar. Biol. Ecol. 82, 147–159. doi: 10.1016/0022-0981(84)90100-X
Philip S. Y., Kew S. F., van Oldenborgh G. J., Anslow F. S., Seneviratne S. I., Vautard R., et al. (2021). Rapid attribution analysis of the extraordinary heatwave on the Pacific Coast of the US and Canada June 2021. Earth system change: Climate scenarios. 13, 1689–1713. doi: 10.5194/esd-2021-90
Raymond W. W., Barber J. S., Dethier M. N., Hayford H. A., Harley C. D. G., King T. L., et al. (2022). Assessment of the impacts of an unprecedented heatwave on intertidal shellfish of the Salish Sea. Ecology 103, 1–7. doi: 10.1002/ecy.3798
Raymond W. W., Tobin E. D., Barber J. S., Hayford H. A., Raymond A. E. T., Speck C. A., et al. (2024). Short-term effects of an unprecedented heatwave on intertidal bivalve populations: fisheries management surveys provide an incomplete picture. Front. Mar. Sci. 11. doi: 10.3389/fmars.2024.1390763
Rice E. L., Chapman A. R. O. (1985). A numerical taxonomic study of Fucus distichus (Phaeophyta). Journal of the. Mar. Biol. Assoc. United Kingdom. 65, 433–459. doi: 10.1017/S0025315400050530
Seed R., Suchanek T. H. (1992). “Population & community ecology of Mytilus,” in The mussel Mytilus. Ed. Gosling E. M. (Amsterdam-London-New York-Tokyo: Elsevier: Elsevier Science Publishers), 87–169.
Shanks A. L. (2009). Pelagic larval duration and dispersal distance revisited. Biol. Bull. 216, 373–385. doi: 10.1086/BBLv216n3p373
Shanks A. L., Grantham B. A., Carr M. H. (2003). Propagule dispersal distance and the size and spacing of marine reserves. Ecol. Appl. 13, 159–169. doi: 10.1890/1051-0761(2003)013[0159:PDDATS]2.0.CO;2
Smith J. R., Fong P., Ambrose R. F. (2006). Dramatic declines in mussel bed community diversity: Response to climate change? Ecology 87, 1153–1161. doi: 10.1890/0012-9658(2006)87[1153:DDIMBC]2.0.CO;2
Smolina I., Kollias S., Jueterbock A., Coyer J. A., Hoarau G. (2016). Variation in thermal stress response in two populations of the brown seaweed, Fucus distichus, from the Arctic and subarctic intertidal. R. Soc Open Sci. 3, 150429. doi: 10.1098/rsos.150429
Strathmann M. F. (1987). “Reproduction and development of marine invertebrates of the northern Pacific Coast,” in Data and methods for the study of eggs, embryos, and larvae (Seattle and London: University of Washington Press).
Suchanek T. H. (1981). The role of disturbance in the evolution of life history strategies in the intertidal mussels Mytilus edulis and Mytilus californianus. Oecologia 50, 143–152. doi: 10.1007/BF00348028
Suchanek T. H. (1992). Extreme biodiversity in the marine environment: Mussel bed communities of Mytilus californianus. Northwest Environ. J. 8, 150–152.
Tomanek L., Helmuth B. (2002). Physical ecology of rocky intertidal organisms: a synergy of concepts. Integr. Comp. Biol. 42, 771–775. doi: 10.1093/icb/42.4.771
Trevelyan G., Chang E. (1983). Experiments on larval rearing of the California mussel (Mytilus californianus). J. World Maric Soc. 14, 137–148. doi: 10.1111/j.1749-7345.1983.tb00068.x
Van Alstyne K. L. (1988). Herbivore grazing increases polyphenolic defenses in the intertidal brown alga Fucus distichus. Ecology 69, 655–663. doi: 10.2307/1941014
Vesco L. L., Gillard R. (1980). Recovery of benthic marine populations along the Pacific Coast of the United States following man-made and natural disturbances including pertinent life history information (U.S. Department of the Interior, Bureau of Land Management Service, POCS Reference Paper No. 53–54).
White R. H., Anderson S., Booth J. F., Braich G., Draeger C., Fei C., et al. (2023). The unprecedented Pacific Northwest heatwave of June 2021. Nat. Commun. 14, 727. doi: 10.1038/s41467-023-36289-3
Keywords: atmospheric heatwave, community stability, foundation species, long-term monitoring, mussels, rockweed, acorn barnacles, temperate rocky intertidal
Citation: Miner CM, Berry HD, Bohlmann H, Dethier MN, Fradkin SC, Gaddam R, Raymond WW and Raimondi PT (2025) Location and natural history are key to determining impact of the 2021 atmospheric heatwave on Pacific Northwest rocky intertidal communities. Front. Mar. Sci. 12:1503019. doi: 10.3389/fmars.2025.1503019
Received: 27 September 2024; Accepted: 09 January 2025;
Published: 29 January 2025.
Edited by:
Julie S. Barber, Fisheries Department Swinomish Indian Tribal Community, United StatesReviewed by:
Emily W. Grason, University of Washington, United StatesCopyright © 2025 Miner, Berry, Bohlmann, Dethier, Fradkin, Gaddam, Raymond and Raimondi. This is an open-access article distributed under the terms of the Creative Commons Attribution License (CC BY). The use, distribution or reproduction in other forums is permitted, provided the original author(s) and the copyright owner(s) are credited and that the original publication in this journal is cited, in accordance with accepted academic practice. No use, distribution or reproduction is permitted which does not comply with these terms.
*Correspondence: C. Melissa Miner, Y21taW5lckB1Y3NjLmVkdQ==
Disclaimer: All claims expressed in this article are solely those of the authors and do not necessarily represent those of their affiliated organizations, or those of the publisher, the editors and the reviewers. Any product that may be evaluated in this article or claim that may be made by its manufacturer is not guaranteed or endorsed by the publisher.
Research integrity at Frontiers
Learn more about the work of our research integrity team to safeguard the quality of each article we publish.