- 1College of Fisheries, Guangdong Ocean University, Zhanjiang, China
- 2Guangdong Provincial Key Laboratory of Aquatic Animal Disease Control and Healthy Culture, Guangdong Ocean University, Zhanjiang, China
- 3Guangdong Provincial Key Laboratory of Control for Disease of Aquatic Animals of Guangdong Higher Education Institutes, Southern Marine Science and Engineering Guangdong Laboratory, Zhanjiang, China
- 4Guangdong Provincial Engineering Research Center for Aquatic Animal Health Assessment, Shenzhen, China
- 5Shenzhen Institute of Guangdong Ocean University, Shenzhen, China
- 6School of Biological and Marine Sciences, University of Plymouth, Plymouth, United Kingdom
- 7Department of Fisheries and Water Resources, University of Energy & Natural Resources, Sunyani, Ghana
- 8Department of Marine Science, Faculty of Marine Science and Fisheries, Hasanuddin University, Makassar, South Sulawesi, Indonesia
As aquaculture takes on a major role in global seafood production, the industry has encountered several hurdles, notably in disease management and overharvesting in natural habitats challenges. Vaccination is a critical component of immunological preventive strategy essential for the health management of animals. Over the past two decades, vaccines have revolutionized the sector by addressing these issues while enhancing productivity and ecological balance. Advanced vaccine technologies, including DNA, recombinant, and inactivated vaccines, have demonstrated their potential to transform aquaculture and sea ranching. Innovations like the recombinant DNA vaccine for goldfish using the G protein expressed by baculovirus for spring viremia for carp and the ME-VAC Aqua Strept vaccine for tilapia highlight their ability to reduce antibiotic dependence and support greener practices. Multivalent vaccines in salmon farming further showcase their effectiveness in improving fish health and productivity. Emerging solutions such as plant-based and mucosal vaccines offer scalable, cost-effective options for immunizing large fish populations, reducing disease-related losses, and stabilizing seafood supply chains. Vaccines also improve the survival rates of hatchery-reared fish in natural habitats, supporting long-term sustainability. By integrating vaccination with selective breeding for disease resistance, aquaculture can achieve enhanced productivity and reduced environmental impact. The article highlights the impact vaccines can have on technology leap forward and research cooperation that will allow for collective mobilization to prevent aquatic disease. Not only that, this review also discusses the challenges and opportunities of using vaccines to increase fish resilience for surviving in open waters. Emphasis on the transformative role of vaccines in enabling technological advancements, fostering research collaborations, and addressing economic and environmental challenges to ensure a sustainable future for aquaculture and sea ranching have been highlighted as well. Future research directions and economic implications of widespread vaccine adoption in aquaculture are also discussed.
1 Introduction
Driven by modern advancements and escalating global demand for fisheries products, aquaculture is undergoing substantial growth. Annual increases in production are necessary to ensure that the rising requirements for food and nutrition are adequately met. Over 80 million tons of aquatic animals, worth US$ 232 billion, are generated by aquaculture activities globally each year, reflecting the scale and economic significance of the industry in addressing the ever-growing demand for seafood and other aquatic products (Angulo et al., 2021). However, the aquaculture sector is severely impacted by several challenges, including fish diseases, which have resulted in substantial mortality rates, posing a major threat to the industry’s sustainable growth (Van Muiswinkel, 2008).
Statistical data reveal that bacterial pathogens cause the majority of disease outbreaks, accounting for 54.9% of cases, followed by viruses (22.6%), parasites (19.4%), and mycotic agents (3.1%), with bacteria posing the greatest threat to fish health in aquaculture operations (Mondal and Thomas, 2022). The disease attacks several types of aquatic animals, such as shrimp, finfish, and mollusks, highlighting their high frequency of spread and significant socio-economic impacts, including reduced production, loss of income and employment, restricted market access, food shortages, and even the closure of businesses or industries. In many cases, economic losses from aquatic diseases have been estimated to reach millions of dollars. For instance, combined production value losses due to shrimp diseases from 1987 to 1994 across 11 countries [Taiwan (1987), the Philippines (1989), Indonesia (1991), China (1992), Ecuador (1992), the USA (1993), Bangladesh (1994), India (1994), Mexico (1994), Thailand (1994), and Vietnam (1994)] were estimated at approximately US$ 3019 million (Israngkura and Sae-Hae, 2002). Another notable example is the infectious salmon anemia (ISA) outbreak in 1998–1999, which caused losses of £20 million to the Scottish fishing industry. Additionally, it led to ongoing annual losses of US$ 11 million in Norway and US$ 14 million in Canada (Bondad-Reantaso et al., 2005). Research on fish diseases is advancing, leading to new prevention and treatment options. Antibiotics and chemotherapies are commonly used, but they face challenges like drug resistance and safety concerns. While effective in the short term, the overreliance on antibiotics has contributed to the global problem of antimicrobial resistance (AMR), posing significant risks to aquaculture and public health. Misuse and overuse of antibiotics in aquaculture not only reduce their efficacy but also promote the proliferation of resistant pathogens that can transfer resistance genes to other microorganisms, including those affecting humans (Preena et al., 2020). This overdependence on antibiotics underscores the urgent need for alternative strategies that are both sustainable and effective.
Since the 1940s, vaccines have been utilized to combat bacterial and viral diseases, contributing to enhanced economic sustainability in global aquaculture and helping mitigate disease outbreaks while reducing the dependency on antibiotics, thereby addressing the dual challenge of fish health management and AMR prevention (Angulo et al., 2021).
Currently, multiple vaccine types exist, such as DNA vaccines, recombinant vaccines, and those based on conventional methods, all aimed at protecting fish from serious diseases. Some of these vaccines have gained approval for use in various aquaculture species (Mondal and Thomas, 2022). Licensed fish vaccines are available for use in different fish species worldwide (Table 1).
Sustainable fish aquaculture focuses on cultivating fish in a manner that minimizes environmental impact, respects natural ecosystems, and prioritizes the health and welfare of the fish. In this context, vaccination has become an essential strategy for disease prevention, playing a vital role in promoting sustainable practices within the industry (Radhakrishnan et al., 2023). Various innovative strategies have been implemented since the mid-20th century to ensure the sustainability of wild fish populations (Bayliss et al., 2017). One prominent approach involves increasing stocks in marine, coastal, and freshwater areas by releasing aquatic animals bred in hatcheries, and such practices are prominent in countries such as the United States and Norway. The pioneers in adopting this practice have become crucial for managing fish population growth, enhancing production, and improving the profitability of fisheries, particularly as demand for seafood continues to rise with the growing human population. While raising fish in hatcheries offers many benefits, early challenges emerge, including a lack of biological understanding of the species and their adaptive properties to natural environments (Araki et al., 2008). These challenges make it difficult to ensure the health and survival of fish once released (López-Vázquez et al., 2023). However, technological advancements in breeding, nutrition, and health management have significantly improved hatchery practices, enabling better fish preparation for their eventual release. Despite these improvements, concerns remain regarding the vulnerability of hatchery-raised fish, particularly their interaction with pathogens, which pose significant risks. Research has indicated the presence of pathogenic bacteria and parasites in various regions, potentially affecting the health and survival of hatchery-reared fish after release (Buchmann et al., 1997). In this context, vaccination has emerged as a promising approach to increase the survival rates of hatchery-raised fish, both in captivity and after their release into natural habitats, thereby supporting sustainable aquaculture practices.
This review aims to examine the role of sustainable fish aquaculture and sea ranching, focusing on the application of vaccination as a vital tool for improving fish health and survival (Buchmann et al., 2001). The study seeks to analyze current practices and innovations in fish farming and sea ranching while addressing the challenges posed by disease outbreaks and environmental impacts. For instance, polyvalent and live-attenuated vaccines have demonstrated reduced mortality rates while enhancing post-release survival when applied via immersion methods (Buchmann et al., 1997; 2001). By bridging insights from immunoprophylactic approaches and sea ranching practices, this review underscores the potential of targeted vaccination strategies to mitigate disease risks, contributing to sustainable aquaculture and marine biodiversity conservation. Furthermore, the review assesses the effectiveness of various vaccination strategies in enhancing the sustainability of aquaculture operations and supporting stock replenishment in natural habitats. By synthesizing existing research and identifying knowledge gaps, this review intends to offer insights and recommendations that can inform future practices for the sustainable management of aquatic resources.
2 Vaccines in aquaculture
2.1 Importance of vaccines in aquaculture
Vaccines represent a pivotal advancement in aquaculture, offering effective solutions against the major threats posed by bacterial, viral, parasitic, and other pathogenic agents (Table 2). Their role in safeguarding fish health and sustaining productivity is becoming increasingly significant. Vaccines strengthen the immune systems and effectively reduce disease risks in fish and other farmed aquatic species. Countries such as China, Japan, and Norway have integrated vaccination programs into their aquaculture practices, underscoring the global recognition of vaccines as essential tools for improving health management (Gudding and Van Muiswinkel, 2013). The strategic use of vaccines supports the growth of aquaculture production as it aids in supporting aquaculture development, thus addressing the rising global demand for fishery resources.
2.1.1 Disease prevention
Disease remains a significant challenge in global aquaculture, resulting in annual losses exceeding 10 billion USD and impacting approximately 10% of cultured animals. Vaccination is vital for mitigating these losses and protecting fish and other aquatic species from infections caused by bacteria, viruses, parasites, and other pathogens (McKenzie et al., 2016). This approach mirrors the use of vaccines in terrestrial livestock and sea ranching, which help ensure healthier populations and promote sustainable practices (Irshath et al., 2023). The importance of vaccination in fish sea ranching cannot be overstated, as it plays a crucial role in disease prevention, much like its role in traditional livestock farming (Lu et al., 2021). The first aquaculture vaccination designed to prevent bacterial infections was implemented in 1970 (Mondal and Thomas, 2022). Common methods for administering fish vaccines have been injection, immersion (Table 3), and mixing with feed, each presenting distinct merits and demerits concerning protection levels, practicality, side effects, and cost-effectiveness (Table 4). Notably, injection and immersion techniques have been developed for industrial-scale applications, enhancing their effectiveness in aquaculture (Zhou et al., 2019).
2.1.2 Reduction in antibiotic use
The threat of antibiotic resistance has received increasing global attention in recent years (Ventola, 2015). Many countries with high levels of antibiotic consumption have a higher incidence of antibiotic resistance (Goossens, 2009). In developing countries, the increase in antibiotic resistance cases is also faster than as witnessed in developed countries; hence, there have reported high mortality cases as a result of the inadequate health facilities and resources (Buckley et al., 2019). Many countries continue to strive to improve the management of antibiotics for use in animals (Goossens, 2009). Fish and shellfish production have been essential for ensuring nutritional security in Asia’s low- and middle-income countries (ILMICs) and sub-Saharan regions such as Africa and South America. In these areas, the local consumption of freshwater fish varieties such as carp, tilapia, and catfish remains crucial in providing essential nutrients for healthy development (Stentiford et al., 2020). The significant disruptions in aquaculture production stemming from bacterial and viral infections lead to substantial losses in these nations. One effective strategy employed within the fish farming industry to mitigate disease outbreaks is the utilization of anti-microbiota (Subasinghe et al., 2019). Excessive and uncontrolled use of microbes causes antimicrobial resistance (AMR), exacerbating the AMR crisis in veterinary medicine and public health. Since the early 2000s, there has been an increasing trend toward merging traditionally distinct disciplinary approaches to research and policy concerning human, animal, and environmental health. This shift embraces a more comprehensive One Health approach, acknowledging the intricate interconnectedness of humans, animals, and the planet. AMR in bacteria within human populations is correlated with AMC (antimicrobial consumption) in both human and animal populations, demonstrating a connection between AMR in bacteria found in animals and humans (Stentiford et al., 2020). The challenges surrounding AMR usage to the One Health framework necessitates a comprehensive examination of AMR drivers, including those within food production. As aquaculture continues to expand and play a vital role in global food security, the scrutiny of AMR risks during aquatic food production is increasingly prominent within the One Health framework (Stentiford et al., 2020) (Figure 1).
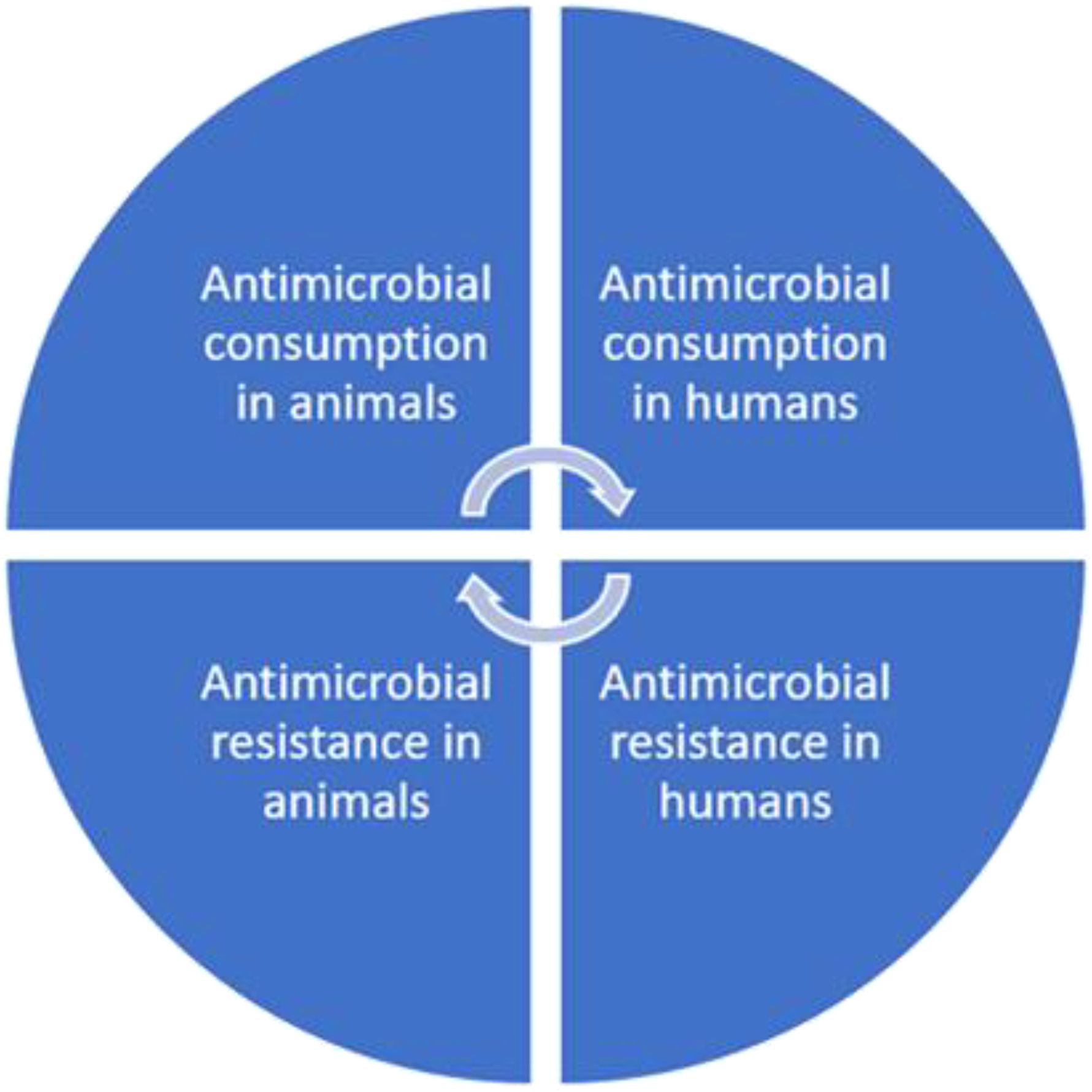
Figure 1. The cyclic relationship between antimicrobial consumption and resistance in animal and human populations illustrating the feedback loop between antimicrobial consumption and resistance in animal and human populations. Antimicrobial use in animals contributes to resistance to animal pathogens, which can transfer to humans through direct contact, food, or environmental exposure. Similarly, antimicrobial use in humans drives resistance to human pathogens, which can indirectly affect animals through environmental pathways, such as wastewater or contaminated animal feed.
By mitigating disease outbreaks, vaccines can reduce the need for antibiotics, lower the risk of antibiotic resistance, and reduce environmental pollution. Vaccination is an important discovery effective against various diseases, even though vaccines sometimes do not work in some species. Vaccines offer a viable solution for maintaining aquaculture health amidst the widespread use of antimicrobial agents that have caused widespread AMR. It is important to encourage discussion and advocate support for autogenous vaccination as a relatively easy technology that, when implemented locally, can reduce antibiotic use and disrupt the AMR chain in aquaculture (Barnes et al., 2022).
2.1.3 Increased productivity
Infections that occur in fish on commercial farms worldwide are serious, and there are many ways to develop therapy for controlling infections caused by fish pathogens. From time immemorial, antibiotics and chemotherapies have been used to treat diseases in several types of fish, but their weaknesses, such as causing the emergence of antibiotic-resistant genes, antibiotic-resistant bacteria, and consumer and environmental safety problems, have been known to outweigh their strengths. Some vaccines greatly reduce the impact of losses and increase aquaculture production. At present, millions of fish are vaccinated annually and these healthy fish are reported to grow faster, improving productivity and economic gains. Vaccination helps maintain biodiversity, restores depleted populations, and supports the overall stability and resilience of aquatic ecosystems by ensuring the health and survival of stocked fish. When cultured fish are stocked in oceans, rivers, or other natural habitats, they face various environmental challenges and disease risks that may compromise their survival and integration into wild populations. Vaccination provides a proactive approach to disease prevention, helping to protect stocked fish from common pathogens and infectious diseases that could otherwise threaten their health and the surrounding ecosystem (Mutoloki et al., 2015). Incorporating vaccination into stocking programs enhances the survival and well-being of stocked fish and contributes to the conservation and sustainable management of wild fish populations. By reducing the risk of disease transmission from stocked to wild fish, vaccination helps maintain the genetic integrity and ecological balance of native species, thereby preserving the natural biodiversity and functioning of aquatic ecosystems (Arechavala-Lopez et al., 2013). Healthy stocked fish contribute to fisheries overall productivity and resilience, supporting sustainable harvests and economic opportunities for fishermen and aquaculture producers. By bolstering fishery resources through stocking initiatives, vaccination plays a vital role in ensuring the long-term viability and profitability of the fishery industry. In keeping stocked fish healthy and maximizing their contribution to ecosystem health and fishery sustainability, it is essential to implement comprehensive vaccination programs as part of stocking strategies. This includes identifying disease risks, selecting appropriate vaccines, and implementing vaccination protocols tailored to the specific needs and conditions of the stocked fish and their environment (Mondal and Thomas, 2022).
2.1.4 Vaccination reduces the transfer of disease
Stock enhancement within marine and coastal areas through the release of aquatic animals raised in hatcheries is a potential management tool for increasing the production and profitability of marine fisheries and meeting the demand for seafood from an ever-increasing human population (Zhou et al., 2019). The technology to produce a large number of early-life stage aquatic organisms in hatcheries is well-developed in several countries. However, these practices require technical interventions in the rearing process that may substantially change how an organism interacts with the natural environment, the culture environment, and other species. These changes also influence how organisms interact with pathogens (Gregory et al., 2013). The use of alien species to create new fisheries brings special concerns such as having the high potential to transfer new pathogens or for the introduced animals to be susceptible to pathogens that do not affect native species. Bacteria, fungi, parasites, and other organisms that may not be pathogenic under normal environmental conditions for native species can become problematic in stock enhancement programs.
Risk assessment evaluates the probability of a specific pathogen infiltrating, establishing, and disseminating within an importing country or its receiving environment and the ensuing repercussions. This process comprises four distinct components, as depicted in Figure 2. A release assessment involves delineating the pathways for introducing a pathogen into a specific environment and estimating the likelihood of such an event, considering factors like transmission means, infectivity, and geographical characteristics (López-Vázquez et al., 2023). For instance, the White Spot Syndrome Virus (WSSV) displays a wide geographic spread across multiple host species, suggesting a high probability of survival upon release into the wild. Similarly, exposure assessment entails describing pathogen exposure pathways to the hatchery or wild animals and estimating the likelihood of exposure, with considerations mirroring those in release assessments (Bartley et al., 2006). Factors such as transmission mechanisms, biosecurity measures, and consequences on productivity and economy are evaluated. Consequence assessments are conducted when exposure assessments reveal more than a negligible risk, assessing potential impacts on productivity, environment, and economy, exemplified by cases like the Pilchard disease outbreak causing a significant economic loss. Risk estimation involves creating matrices to quantify pathogen risk, aiding in decision-making and mitigation strategies through comprehensive record-keeping and health profiling. Vaccination plays a crucial role in reducing disease transmission within aquaculture and even livestock farming systems. By administering vaccines to aquatic species and livestock, farmers can bolster their immune systems, making them more resistant to pathogens commonly found in their environments. This proactive approach helps mitigate the spread of diseases among individual animals and entire populations, ultimately leading to improved health and productivity. Vaccination strategies tailored to specific diseases and species can significantly contribute to the sustainability and profitability of aquaculture and sea ranching operations (Bartley et al., 2006).
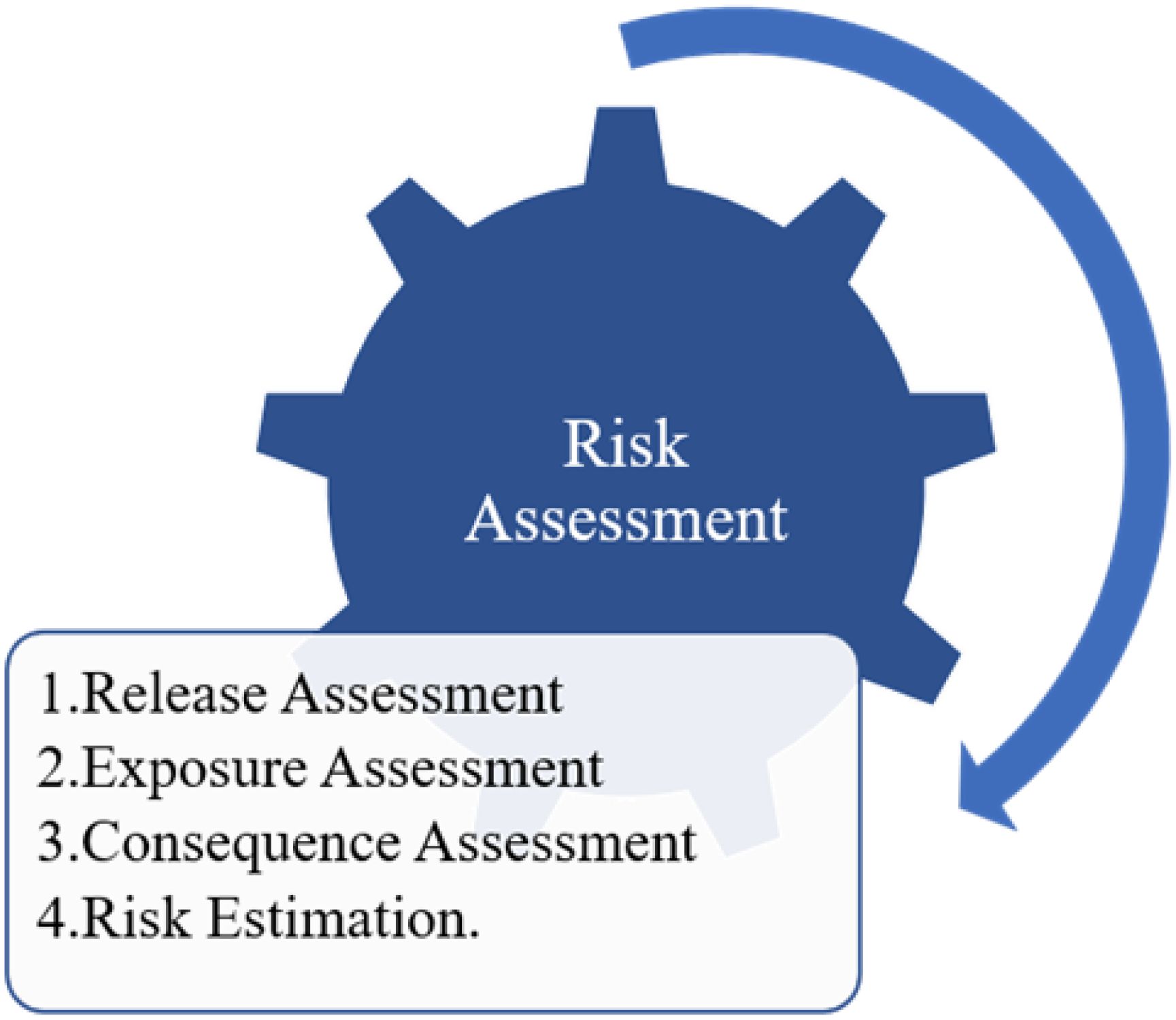
Figure 2. Four components of risk assessment of the possibility of pathogens entering and developing in the importing country or recipient environment.
2.2 Types of vaccines used in aquaculture
Vaccination is one of the most effective methods for disease prevention in aquaculture. A vaccine is an antigenic preparation designed to stimulate the production of antibodies and memory cells, thereby generating immunity against disease. An “ideal fish vaccine” should elicit a specific immune response and provide robust protection (Muñoz-Atienza, 2021). Vaccines used in aquaculture are classified based on their preparation method, including live attenuated vaccines, vectored vaccines, inactivated vaccines, and subunit vaccines. Conventional methods, such as live attenuated and killed vaccines, are widely used due to their ability to induce specific immune responses in the host, while advancements in molecular vaccines offer targeted and safer approaches (Muheem et al., 2016). The administration methods for aquatic vaccines include immersion, injection, feeding, or gene-based techniques, with each type having its advantages and disadvantages depending on the target pathogen, immune response, safety, and application feasibility (Tables 3, 4). A summary of commonly used vaccines in aquaculture is shown in Figure 3.
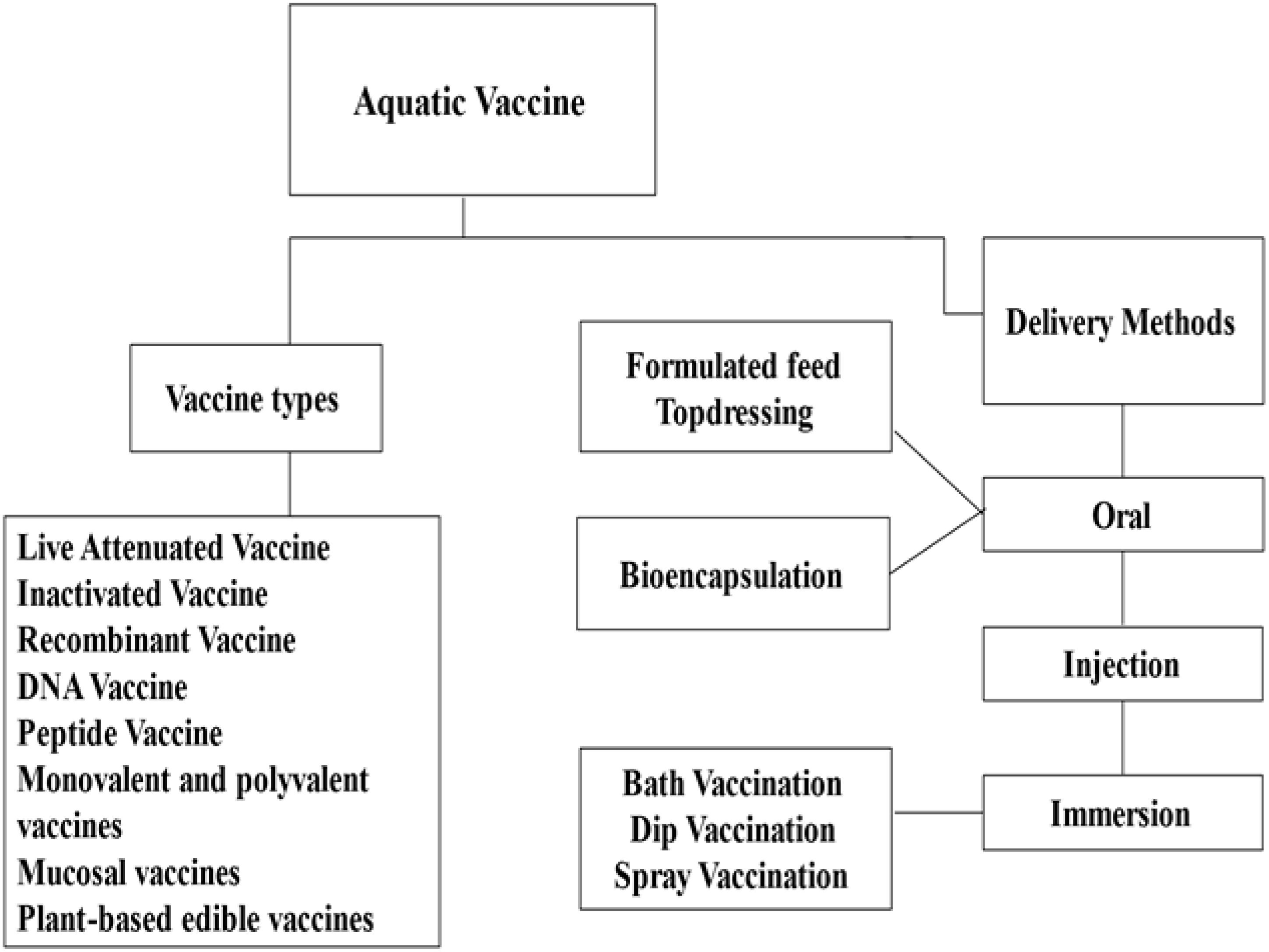
Figure 3. Presentation of the aquatic vaccine types and their delivery methods used in aquaculture. Vaccine types used in aquaculture include live attenuated, inactivated, recombinant, DNA, peptide, monovalent and polyvalent, mucosal, and plant-based edible vaccines. Delivery methods are categorized into oral (via feed or bioencapsulation), injection, and immersion (including bath, dip, and spray). These approaches aim to improve fish health, enhance immunity, and support sustainable aquaculture practices by effectively combating aquatic diseases.
2.2.1 Inactivated vaccines
Inactivated vaccines are reported to be the widely used vaccines in aquaculture. This vaccine is derived from bacteria obtained from cultivating certain microorganisms that are then exposed to formalin inactivation. These vaccines work through inoculation to provide protective immunity, but in some cases, viral diseases are an impractical approach because the emergence of the disease occurs in the early stages of life (Dadar et al., 2017). Many researchers conduct studies on the effects of formalin on retinopathy and viral encephalopathy to determine its immunological and protective effects. What can be seen is how formalin can kill bacteria when injected intraperitoneally. A study was conducted to assess the efficiency of inactivated vaccines using formalin to provide in vivo cross-protection against RGNNV made from Striped Jack strain Nervous Necrosis virus (SJNNV) strain 484.2.2009 and two different betanoda virus serotypes, RGNNV strain 283.2009 (Mondal and Thomas, 2022). The study found that the two serotypes did not protect each other in vivo. Based on their findings, they reported that the production of multivalent formulations, or different types of vaccines based on the needs of fish species and viruses, should be recommended for effective protection. Several studies report that the ALV405 antigen of SAV-based inactivated virus vaccines can effectively protect salmonids against pancreatic disease (PD) infection by using single or polyvalent vaccines as candidates (Dadar et al., 2017).
2.2.2 Recombinant vaccines
Recombinant vaccines use recombinant DNA technology to improve vaccines. This vaccine only works on immunogenic regions of the pathogen expressed in heterologous hosts for use as vaccines. Other studies have also suggested that to determine the survival rate of vaccinated fish, antigen-stimulated protective checks are needed when it comes to vaccinating fish with antigens and then infecting fish experimentally with live pathogens (Lu et al., 2021). Several studies have also used recombinant technology to induce protective immune responses against pathogens such as Aeromonas hydrophila, and infectious salmon anemia virus (ISAV). They report that recombinant DNA technology via oral vaccination can protect salmon from ISAV infection (Lu et al., 2011; Barnes et al., 2022; Dar et al., 2022). Despite the effectiveness of recombinant vaccines in increasing immunity and preventing disease infection in fish in some cases, the widespread application of recombinant vaccines in aquaculture still faces significant challenges, namely regulatory obstacles, including the need for comprehensive safety and efficacy evaluations, often complicating the approval process; thus, takes a long time before hitting the market. The high production costs of recombinant DNA technology, which requires constructing special facilities and scale-up of production for commercial use, is still a major obstacle (Barnes et al., 2023). Overcoming these challenges requires concerted efforts to simplify regulatory pathways and optimize production technologies, such as using cost-effective expression systems or increasing antigen yield through biotechnological advances.
2.2.3 Attenuated vaccines
Attenuated vaccines can genetically or chemically induce an immune response quickly. Microorganisms such as bacteria and viruses can no longer cause certain infections; hence, this vaccine can be used sustainably. From the 1990s till date, there are four live attenuated vaccines used or applied to several pathogens caused by bacteria such as carp disease, koi herpesvirus (KHV) (Harborne, 2013), bacterial kidney disease, columnaris disease (Bayliss et al., 2017) and enteric septicemia in catfish. This particular vaccine can be used via injection or soaking method. A previously conducted study reported that a live vaccine candidate deleted attenuated gene, ΔORF022L, on mandarin-1 fish seed cells (MFF-1) against infectious spleen and renal necrosis virus (ISKNV) (Du et al., 2022) resulted in a 100% survival of fish infected. In addition, the vaccine-induced an anti-ISKNV-specific antibody response that can be beneficial for controlling fish disease.
2.2.4 Monovalent and polyvalent vaccines
Most infectious diseases that are species-specific are reported to be prevented or controlled using polyvalent vaccines, which are an ideal vaccine form. Polyvalent vaccines provide superior protection against turbot and salmon, similar to monovalent vaccines (Radhakrishnan et al., 2023). Many studies have been developed in recent years on the advancement of polyvalent vaccines, one of which is ME-VAC (Marine Environment Vaccine) Aqua Strept, a vaccine product used in aquaculture to protect against infections caused by Streptococcus bacteria in fish or other aquatic animals. This product is probably formulated to prevent or control streptococcosis through injection and immersion methods in Nile tilapia, Oreochromis niloticus. This inactivated polyvalent vaccine showed combined protection against several bacterial infections, such as Streptococcosis, Enterococcosis, and Lactococcosis in tilapia (Mondal and Thomas, 2022).
2.2.5 DNA vaccines
Plasmid combinations carrying pathogen-specific antigens have gained widespread attention for promoting protective immunity against various diseases using DNA vaccines. This vaccine can produce a non-specific immune response, specific immunity, and strict and precise protection in fish. In using this vaccine via injection method, Ge virus encoding glycoprotein showed increased levels of protection against IHNV (infection hematopoietic necrosis virus) infection (Nishizawa et al., 2002). VHSV (viral hemorrhagic septicemia virus) glycoproteins were used to provide an effective immune response, followed by DNA vaccination for rainbow trout. Vibrio anguillarum has the extracellular zinc metalloprotease, a known virulence factor whose toxicity makes it a strong candidate antigen for vaccine development (Ringø et al., 2014). There are reports that the M99-conditioned LB20 stimulates protease activity in V. anguillarum NB10 strains while allowing V. anguillarum M93Sm strains to produce proteases in LB10 (Denkin and Nelson, 2004). A DNA vaccine encapsulated with chitosan was developed to protect European sea bass against nodavirus NNV (nervous necrosis virus) infection (Gámez-Valero et al., 2016). The report showed partial protection of European sea bass juveniles and the regulation of genes associated with cell-mediated cytotoxicity (CMC), TCRB (T-cell receptor beta), and IFN (interferon). A previously conducted study reported that DNA vaccination induces strong protective immunity against certain viral fish infections, particularly in rainbow trout and Atlantic salmon infected with Rhabdovirus and herpes virus infecting catfish (Nusbaum et al., 2002). Cross-protection disappears within 2-months after vaccination, while specific immunity to homologous viruses remains high.
2.2.6 Mucosal vaccines
The application of mucosal vaccines in some fish species are known to also aid in providing longer immunity periods in fish. This type of vaccine has received great attention in the aquaculture industry, and several studies have been carried out to observe a proactive response on mucosal surfaces by blocking pathogens at the initial site of replication (Muñoz-Atienza, 2021). Bath and oral immunizations of grouper (Epinephelus coioides) larvae with a binary ethylenimine (BEI)-inactivated adjuvants could help increase the immunogenicity of these antigens and bypass mucosal tolerance. It implies a greater knowledge of these regulatory systems and a search for adjuvants suited for mucosal delivery in fish. However, this particular vaccine development has been met with several challenges, including designing mucosal vaccines in ray-finned fish to determine the dose of protective antigens needed to provide immunity (Munang’andu et al., 2012). This is because it is known that injectable vaccines are considered more protective; thus, the conditions and doses required to develop an effective mucosal vaccine must be carefully designed.
2.2.7 Plant-based edible vaccines
Edible vaccines or vaccines derived from plants are a class of vaccines that are environmentally friendly compared to several other types of vaccines, such as attenuated and inactivated vaccines, which are expensive to use, and their method of administration, including injection are sometimes not easy to apply to a large number of fish (Shahid and Daniell, 2016). Thus, plant-based vaccine usage is economical for developing efficient vaccines (Bonilla-Aldana et al., 2020). Fish vaccines made from edible plants offer great potential for oral vaccination in aquaculture. A plant-generated recombinant subunit vaccine could simultaneously provide several antigen proteins (Buyel, 2019). Although plant-produced fish vaccine has been used frequently even on commercial bases, they are still under development. For example for salmonids, vaccines have been developed for diseases such as Infectious Pancreatic Necrosis Virus (IPNV) and IHNV. The IPNV vaccine uses an inactivated virus as the antigen, with an inactivated production platform administered intraperitoneally. This vaccine is commercially available and produced by Centrovet in Chile. The IHNV vaccine employs recombinant G protein as the antigen, utilizes a DNA production platform, and is delivered intramuscularly. It is also commercially available, with producers including Aqua Health Ltd. and Novartis, Canada. One type of vaccine that uses a plant expression system is VLP (viral capsid proteins) assembled from viral capsid proteins that mimic the tertiary structure of natural viruses. VLPs do not contain genetic material, thus avoiding the possibility of reversion mutations or pathogen infection (Noad and Roy, 2003). However, VLPs can potentiate the host immune response through recognizable repeat subunits that trigger cellular and humoral responses (Keller et al., 2010). VLP vaccines have been licensed and commercialized in humans, such as Cervarix human papillomavirus (HPV), Recombivax HB Hepatitis B virus (HBV) from Merck, and Gardasil (Yusibov et al., 2011). There have been increasing interest in VLP vaccination in fish. Injection of NNV-VLP vaccines produced by E. coli, yeast, baculovirus, and self-assembled expression from plants or cells have been tested to elicit immune responses in fish, stimulate specific antibody secretion, and trigger full-scale immune responses (Lai et al., 2014). VLP vaccines have become ideal advanced subunit vaccine candidates for fish vaccines. Oral VLP vaccines against grouper NNV can stimulate specific antibody production and provide more than 50% protection against NNV attack (Luu et al., 2017). A yeast-expressed IPNV VP2 capsid protein (SVP) subviral particle vaccine in rainbow trout has been developed to induce specific antibody secretion, proving its immunogenicity (Dhar et al., 2014). Therefore, it is extremely necessary to do further research on fish vaccine production using plant biotechnology.
2.2.8 Synthetic peptide vaccines
Peptide vaccines work almost the same as subunit vaccines or suitable antigenic sites. This vaccine is used to stimulate the production of antibodies to several pathogens such as noda virus, Rhabdovirus, birnavirus, IHNV, IPNV, and VHS, as reported by some researchers (Ma et al., 2019; Kumar et al., 2024). Synthetic peptides are potent antiviral agents and an alternative to control viral infections in Atlantic salmon. Peptides from both RNA viruses, ISAV (infectious salmon anemia virus) and IPNV, were designed based on in silico analysis and tested in vitro on fish cell lines. In addition, in vivo tests were carried out on Salmo salar fish with synthetic peptide GIM 182 from IPNV. The results proved that using peptides as antiviral agents in disease control may be a suitable alternative to explore in aquaculture (Cervera et al., 2024; Cárdenas et al., 2020). Other studies have shown that β-defensin peptides are effective against hemorrhagic septicemia virus (VHSV) in Oncorhynchus mykiss (Falco et al., 2008). Chinook salmon embryo (CHSE) 214 cells were protected from IPNV infection, a significant hazard to the salmon industry causing major economic damage, by administering Tilapia hepcidin (TH) 1–5. Epithelioma papulosumcyprini cells (EPC) were made resistant to hemorrhagic septicemia virus (VHSV) by transfection of a plasmid carrying recombinant rainbow trout β-defensin 1 (Defb1) (Naiel et al., 2023). Hepcidin showed antiviral effects against largemouth bass Micropterus salmoides reovirus (MsReV) and Sinipercachuatsi rhabdovirus (SCRV) in grass carp fin cells (GCF) and EPC (Gui et al., 2016). In addition, antiviral properties against fish NNV have been exhibited by TH1–5 from tilapia and epinecidin-1 from grouper (Chia et al., 2010). The potential of epinecidin-1 to rescue grouper from nerve necrosis disease by increasing survival has led to the proposal of further investigation into its possible therapeutic application for viral infections. Fish-derived antimicrobial peptides (AMPs) vaccination has immune-stimulating effects and antibacterial properties (Naiel et al., 2023). These peptides have been documented to exhibit a variety of pharmacological and nutraceutical properties, including antimicrobial, antioxidant, antihypertensive, anticoagulant, anticarcinogenic, immunomodulatory, and others (Välimaa et al., 2019).
2.3 Targeted vaccination
There is now a wide range of fish species genomes available, and the technological costs of whole genome sequencing are decreasing, but certain genomes are being reported regularly. The targeted vaccine designs for heterogeneous species have been characterized with even more than 300 Flavobacterium Sychrohilum species reported to be characterized, especially those originating from England to produce effective valent whole cells. This is a significant result from an epidemiological point of view (Dar et al., 2022). Sometimes, it is impossible to develop whole-cell vaccines such as some immunosuppressive epitopes; therefore, it is necessary to identify the specific antigen and produce a vaccine that eliminates the unwanted.
2.3.1 Discovering specific potential protective antigens
Reverse vaccinology is a genomic method that is widely used to determine potential vaccine elements for making protein subunits. The process is carried out by examining pathogens’ protein sequences using software to identify potential vaccine components. The selection of pathogen types depends on their status in vaccine candidates on different pathogens, high immunogenicity, and other specified standard criteria. The next step is the production of the recombinant subunit vaccines (produced by recombinant DNA technology) and an in vivo efficacy assessment test. This subunit vaccine (vaccine DNA) has received permission to be used in aquaculture in European countries, and in the future, it will be developed by researching the fertility of DNA vaccines against fish species (Ma et al., 2019).
2.3.2 The administration of vaccines facilitated by nanoparticles
Nanoparticles measuring less than 1000 nm, like virus-like particles (VLPs), liposomes, immunostimulating complexes (ISCOMs), polymeric, and non-degradable nanospheres, demonstrate promise as carriers for vaccine antigens. These entities stabilize vaccine antigens and serve as adjuvants, potentially steering immune responses in diverse directions to elicit protective reactions (Fredriksen et al., 2011). They prove especially suitable for delivering vaccines through mucosal routes, facilitating sustained antigen release, and reducing the necessity for booster shots. Polymeric systems, notably poly D, L-lactide-co-glycolic acid (PLGA) nanoparticles, have been extensively applied in controlled antigen delivery for peptides, synthetic proteins, and nucleic acids in humans, and they have also been evaluated for oral vaccine delivery in fish. Additionally, non-infectious VLPs have emerged as a novel vaccine platform, inducing neutralizing antibodies, as evidenced in studies on orange-spotted grouper NNV (OSGNNV) VLPs, demonstrating potential as oral vaccines. These VLPs, constructed from recombinant capsid proteins through self-assembly, were successfully produced in E. coli (Gregory et al., 2013) and may find applications in alternative expression systems like Pichia pastoris, serving as an effective vehicle for oral antigen delivery in fish (Adams, 2019).
Nanoparticles in fish vaccine development are mostly limited to certain types, such as polymeric nanoparticles, nanoliposomes, carbon nanotubes, calcium phosphates, and ISCOMs. Other types of nanoparticles are still rarely explored. Although nano vaccines have clear advantages, such as increasing efficacy and immune response, there are major challenges, namely maintaining the stability and consistency of nanoparticle properties during production, as well as overcoming their potential toxicity. These challenges must be addressed carefully to ensure the safety and effectiveness of nanoparticle-based vaccines (Lai et al., 2013).
Nanoparticle-based vaccine delivery systems offer a variety of technical approaches, each of which has advantages and limitations. Polymeric nanoparticles provide controlled antigen delivery and biodegradability, but challenges include low antigen loading and premature antigen release (Fredriksen et al., 2011). Inorganic nanoparticles offer better antigen protection but face the problem of low biodegradability. Nanoliposomes, with intrinsic adjuvant properties, are stable in modified form but struggle with poor gastrointestinal stability. ISCOM simplifies antigen encapsulation, but hydrophilic antigens are difficult to incorporate. VLPs mimic viruses due to their potent but less reproducible immune response, while nanoemulsions effectively encapsulate antigens but suffer from gastrointestinal instability. These features determine their role in improving vaccine efficacy in aquaculture (Vinay et al., 2018).
2.3.3 Initial vaccination versus subsequent booster shots
The method of using vaccines by injection usually requires administering the vaccine one by one, triggering an immune response that can last in the long term, such as within one year. In contrast to using vaccines by immersion without adjuvants, the vaccine has no long-term effect. Still, it produces a short duration of immunity, so the next booster vaccination is often needed (Mutoloki et al., 2015). Much research is needed to continue developing vaccines to produce sterile vaccines. To achieve this protection, mucosal and systemic immunity stimulation is required. This can be done by combining the vaccine with a booster vaccine, namely carrying out immersion vaccination first, followed by administering an intraperitoneal (IP) booster vaccine, or with an oral booster vaccine (Nitimulyo et al., 2005).
2.3.4 Custom-made vaccines tailored for specific individual cases or outbreaks
Developing licensed vaccines for fish species susceptible to various diseases is impractical. It requires a lot of money and time and cannot be done for some types of pathogens due to multiple factors. So alternative ways are needed to overcome this, such as the use of emergency vaccines. Emergency vaccines or autogenous vaccines are valuable substitutes. For example, the use of other organisms to block pathogens can interact with fish, for example, the cleaner fish Ballen wrasse (Labrus bergilta) and lumpsucker fish, which are used to control sea lice in Norway, and the same case was also experienced in England (Rees et al., 2021). The research was carried out by isolating pathogens from Ballan fish, which showed variability across locations, while pathogenic bacteria targeted with autogenous vaccines have shown effectiveness in treating several diseases, such as artificial Aeromonas salmonicida. However, there is still a challenge for diseases with respect to fish fry because there is still a lack of understanding regarding the time of immunocompetence in this species for the successful use of vaccines using the immersion method. Significant increase in natural IgM levels in the intestinal tract of Ballans wrasse, suggesting a potential compensatory mechanism in gastric-deficient fish (Gregory et al., 2013).
2.4 Environmental impact
In America and Europe, regulations and marketing in the manufacture of animal products are getting stricter, including the manufacture of fish vaccines, and several rules are being passed. These rules span from acquiring permits or licenses for production facilities to specific production forecasts, raw materials, batch quality, and control of production processes (Sommerset et al., 2005a). Europe also has guidelines in place to apply at the time of producing vaccines. The vaccine to be produced must meet contemporary formation standards regarding vaccine quality, safety, and efficacy. Recombinant vaccines also follow the same strict criteria as other drug productions for humans and fish. The development of fish vaccines has also experienced obstacles, especially for the pharmaceutical industry, which faces high costs associated with meeting regulatory standards and the absence of recognition of evaluation reports among national authorities (Gudding and Van Muiswinkel, 2013). International veterinary medicine observed what happened in the Norwegian region, which showed that sales of fish medicine production experienced an important reduction in sales of fish vaccines, resulting in a decrease in the use of anti-microbial drugs in aquaculture, which is currently a trend often observed by researchers. At the end of the 1980s, the introduction of an effective vaccine against Vibrio infections and a furunculosis vaccine in the early 1990s in Norway also resulted in a decrease in medicated feed in aquaculture, which, of course, had a good impact on the surrounding environment, including for humans who consume fish. Although aquaculture in Norway reached an all-time high production of 300,000 tons per year, sales of antibacterial substances amounted to around 1 ton yearly, a drastic reduction from previous use levels (Angulo et al., 2021). Another case of the use of vaccines that can reduce the use of antibiotics in aquaculture activities can be compared to the level of antibiotic use in these two countries, namely Chile and Norway. Chile, a large fish farming country whose main production is salmon in 2020, produced 1,075,896 tons of salmon using 379,600 kg of antimicrobials. In 2019, Norway’s production, on the other hand, amounted to 1,375,307 tons; nevertheless, only 222 kg of antimicrobials were used, showing that Chile used 2,200 kg of antimicrobials more than as was witnessed in Norway’s production. This significant comparison occurs due to differences in vaccine application between the two countries. Norway has consistently applied vaccination in the field of aquaculture for the last 7 seasons and has shown that the vaccination strategy is effective in protecting salmon in the Norwegian seas (Barnes et al., 2022). One could posit that, in the short term, research into fish vaccines has made a more substantial contribution to safeguarding the aquatic environment than the collective efforts of the environmental and technological sciences.
2.5 Monitoring and research
The practice of sustainable aquaculture in monitoring fish health is carried out through research on various techniques to improve developments in aquaculture, one of which is the use of vaccines. Research programs on genetic improvement and development investment that focus on the recovery of commercial aquaculture species to increase disease-resistance can certainly increase production in aquaculture; thus must receive support (Ma et al., 2019). Research involving selective breeding could enhance the genetic resistance to common diseases, ultimately reducing the prevalence of diseases in the aquaculture systems. Again, fostering collaboration between the aquaculture industry stakeholders and research institutions to conduct ongoing research on disease dynamics, pathogen identification, and developing effective vaccines or treatments for prevalent aquaculture diseases is as well vital.
2.6 Models used specifically to test and assess challenges
Assessing vaccine effectiveness involves standardized disease challenge models in vivo, closely mimicking natural pathogen exposure (Mondal and Thomas, 2022). While bath and co-habitation challenges are more complex to control than injection methods, they best replicate natural exposure scenarios. For instance, when an experimental disease challenge method is not reproducible, pathogen load (measured via qPCR) and immunological markers may serve as proxies for vaccine efficacy evaluation. In cases like Rainbow Trout Fry Syndrome (RTFS) caused by Flavobacterium psychrophilum, inducing the disease through bath or co-habitation challenges is challenging without resorting to scarification or stress induction. Intramuscular injection, while inducing the disease, does not represent an appropriate challenge method for testing mucosal vaccines administered through dip immersion, necessitating pre-treatment with low levels of hydrogen peroxide. Despite this, infection levels might not be adequate to evaluate vaccine efficacy fully, prompting the planning of field trials (Arechavala-Lopez et al., 2013).
2.7 Administration methods
Determining the most effective methods for administering vaccines remains a crucial task. While certain emerging vaccines might confer protection, the existing administration techniques and vaccination strategies might not ensure optimal efficacy. For instance, employing prime/booster vaccination approaches might be necessary (Fredriksen et al., 2011). Fish possess extensive mucosal surfaces (such as skin, gills, gut, and nasal mucosae), making mucosal vaccine administration more feasible and cost-effective in some sectors than injection methods (Ringø et al., 2014). Despite this, only a limited array of mucosal (immersion and oral) vaccines are available commercially. Multiple challenges impede their development, including the absence of reliable indicators of protection, inadequate optimization of necessary protective doses, potential oral tolerance issues, the risk of denaturation in the stomach for oral vaccines, and the ability of antigens to traverse mucosal barriers for access to antigen-presenting cells (APCs) (Zhou et al., 2019) (Figure 3).
2.8 The reaction of the immune system and indicators that signify protection against pathogens
Measuring the responses triggered by vaccination in teleost fish is crucial for advancing new vaccines and assessing those already in existence (Papadopoulos et al., 2008). The adaptive immune response, orchestrated by T and B lymphocytes, primarily contributes to safeguarding following vaccination. Yet, there remain gaps in understanding, particularly concerning protective indicators for mucosal vaccines (Radhakrishnan et al., 2023), although strides are being made in this domain (Newman, 1993). Notably, methodologies have been established to isolate GALT cells from salmonids, revealing their expression of diverse T-cell, B-cell, and dendritic cell markers and their differential responsiveness to various PAMPS, cytokines, and PHA. Such insights are pivotal in guiding the development of oral vaccines (Mondal and Thomas, 2022).
2.9 Challenges and considerations
Fish vaccination functions to protect against disease and must be ecologically safe for the fish and the surrounding ecosystem, economically feasible for large-scale production, easy to carry out, and capable of eliciting a strong immune response in susceptible species, with few side effects (Gudding and Van Muiswinkel, 2013). Molecular research shows that immunoprophylaxis approaches in goldfish stimulate viral nucleic acid sensors, toll-like receptor (TLR), high mobility group box (HMGB), retinoic acid-inducible gene i-like receptor (RIG-I-Like Receptor) RLR, and pattern recognition receptor (PRR), thereby increasing viral resistance (Tamer et al., 2021). This approach can be significantly scaled up to provide the highest level of security. TLRs detect pathogen-associated molecular patterns (PAMPs), which are specific molecules present in pathogens (such as bacteria, viruses, or fungi) and not found in host cells that enhance innate immunity and adaptability to initiate signal cascade (Kumar et al., 2024).
It is required to identify antigens that have the potential to provide protection and validate protection against specific pathogens for the development of effective vaccines. Effective vaccination can be carried out based on several factors, such as the species of fish to be observed, the method of vaccine administration, the availability of reagents, the characteristics of the pathogen, and the challenge model to test the effectiveness of the vaccine (Terech-Majewska, 2016). Various approaches are used to identify protective antigens. Commercial fish vaccines usually consist of whole pathogen cells that have been killed and are given via intraperitoneal injection. Challenges also arise in using whole pathogens for vaccines due to the diverse species of pathogens, the cost of culturing, immunosuppressive epitopes, and intracellular pathogens (Adams, 2019). Some types of vaccines cannot be administered by injection, so the vaccine must be administered via the mucosal method to provide effective protection. Vaccine administration using the mucosal method still experiences several shortcomings, such as limited materials and basic knowledge, which can hamper its effectiveness. Vaccines that are administered by injection are also very expensive in some areas, so they are rarely used in developing countries. Factors like this greatly influence vaccine accessibility (Labh and Shakya, 2014).
3 Vaccines in sea ranching
In animal husbandry, vaccines help improve animal health and productivity. A notable example is the establishment of a salmon hatchery on the Danish island of Bomholm, which began operating in the 1990s. The facility aimed at hatching and rearing Baltic salmon juveniles, emphasizing imprinting them to return to a specific area after feeding in the central Baltic (Buchmann et al., 1997). Concerns have been raised regarding the susceptibility of hatchery-reared smolts to pathogens in the natural environment, given their controlled rearing under strict hygienic conditions. Several studies have assessed the health status of hatchery-reared smolts during the freshwater parr period and floating net cages. In addition, the efficacy of vaccination regimens has been explored as a potential prophylactic measure against pathogens. The goal is to successfully reintroduce hatchery-reared salmon to the natural environment while reducing the risks associated with exposure to pathogens (Bartley et al., 2006). Synthesizing existing research and insights from the Baltic region and beyond can provide valuable perspectives on the potential prophylactic impacts of vaccination systems and their implications for the health, resilience, and sustainability of hatchery-raised fish populations in marine and coastal environments.
Several cases of vaccine use in marine farming to investigate whether immunoprophylaxis can improve the survival of fish that will be released into the wild to increase the population of several types of fish species have been conducted (Table 5).
3.1 Types of vaccines used in sea ranching
Vaccines are used in aquaculture and sea ranching to investigate whether immunoprophylactic measures can increase the survival of fish that will be released into the wild to increase their populations. Based on a previously conducted research (Buchmann et al., 1997, 2001), fish were divided into three groups, namely fish vaccinated using a polyvalent type of vaccine, a weakened vaccine, and the group without a vaccine to compare their effectiveness. In the end, the results showed that the group with vaccines had a low mortality rate, while those without vaccines had a higher death rate. The live-attenuated vaccine containing formalin-inactivated Aeromonas salmonicida, Vibrio anguillarum (serotypes 01 and 02), and Yersinia ruckeri, as well as non-mineral ingredients, after application via immersion method, showed an increase in the level of defense of fish when after being released into the wild.
3.2 Vaccination time
The timeliness of vaccine application is important for the success of the vaccination program. In the application of vaccines in sea ranching, one of the things that must be considered is the time of vaccination of the seeds that will be released. Usually, fish are vaccinated before being released 4-6 weeks into open waters so that there is enough time for the seeds to develop immunity (Loneragan et al., 2013). Fish size plays a significant determining factor, as most species require a minimum weight of 2-5 grams for effective vaccination (Sommerset et al., 2005a). Environmental factors, particularly water temperature, significantly impact the immune response, with temperatures above 10°C generally producing more rapid and robust immunity in species such as Atlantic salmon (Godoy et al., 2008). Some vaccination strategies use a prime-boost approach, where an initial dose is given a few weeks before release, followed by a booster closer to the release date, which has been shown to increase protection against pathogens such as hemorrhagic septicemia virus in rainbow trout (Schönherz et al., 2018). Vaccination is carried out at the enlargement stage in the hatchery or breeding place before the fish are released into the sea (Figure 4). Vaccination is carried out after the fish have reached a certain size and can withstand the stress of the vaccination process (Table 5). This is often done several weeks to months before release so that the fish’s immune system can respond well to the vaccine.
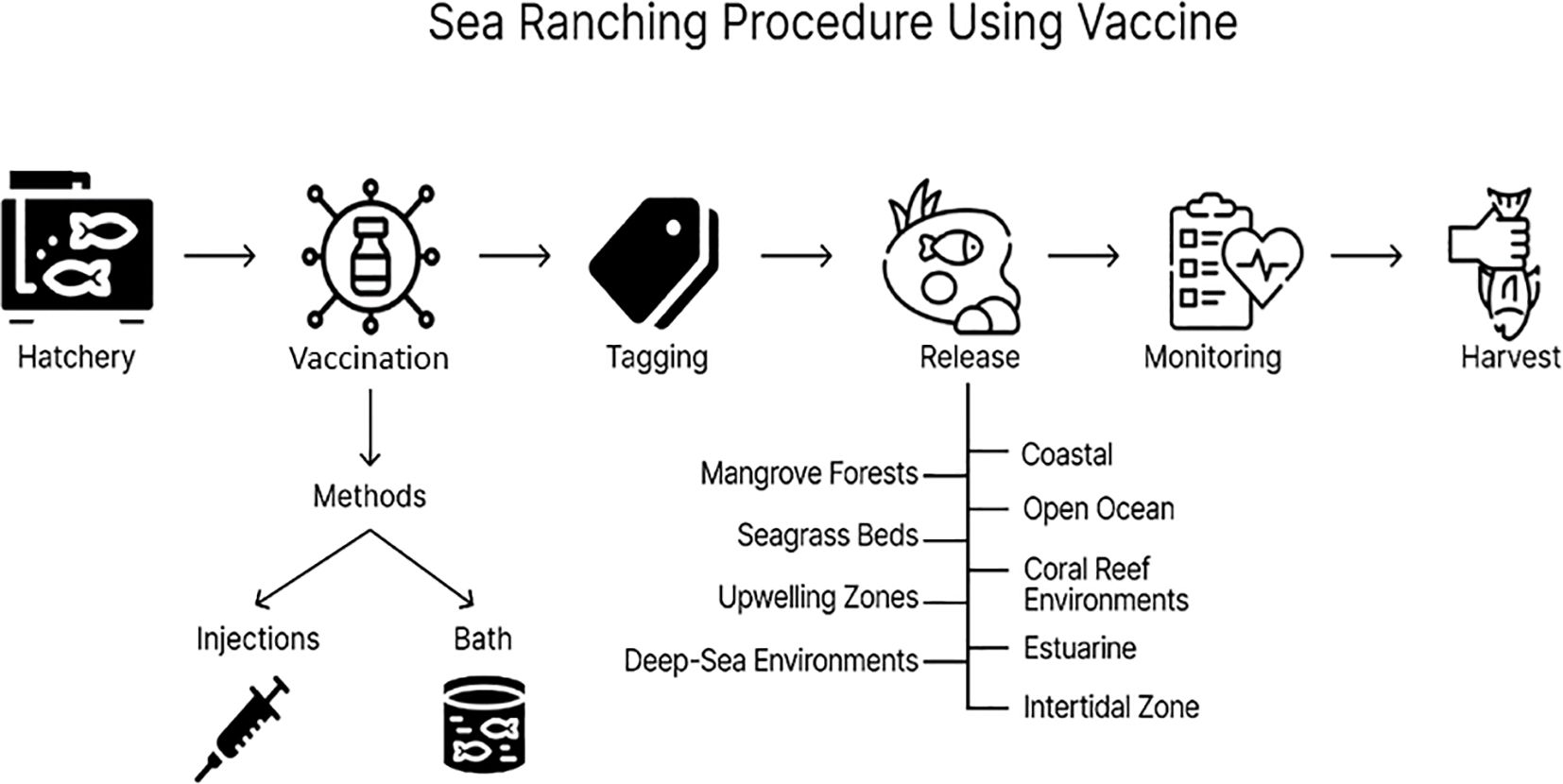
Figure 4. Sea ranching procedure using a vaccine. The procedure begins at the hatchery, where fish are bred and raised under controlled conditions. Vaccination is administered through methods such as injections or immersion (bath vaccination) to enhance immunity against diseases. Following vaccination, fish are tagged for identification and monitoring purposes. The tagged fish are then released into various marine and coastal environments, including mangrove forests, seagrass beds, upwelling zones, deep-sea environments, coral reef ecosystems, estuarine areas, and intertidal zones. Post-release, the fish are monitored to evaluate the wild’s health, growth, and survival rates. The process concludes with harvesting, ensuring sustainable fish production while maintaining ecological balance. This comprehensive approach integrates health management with ecosystem-based aquaculture practices.
3.3 Specific delivery methods used in sea ranching
3.3.1 Injection of vaccine
The method of using vaccines by injection usually takes a lot of time because vaccines have to be injected one by one into the parts of the fish body, which can trigger an immune response in the species to be released (Fredriksen et al., 2011). Still, this method is the most productive and efficient way to immunize fish and provides better protection than immersion (López-Vázquez et al., 2023). This method of vaccination has been used since time immemorial (Buchmann et al., 1997), and the steps involved initial anesthetization of the fish before intraperitoneal injection. Based on previous research (Buchmann et al., 1997), the method of vaccine injection in sea ranching activities is carried out when the fish are in the juvenile period with a body length of 27.9 cm and a weight of 187.4 g of salmon smelts after 4 months in the net-pens.
3.3.2 Bath vaccine
This bath vaccination method is a simple fish immunization method that is done by soaking the fish in a vaccine solution with the appropriate concentration for several hours, which is useful for fighting infections (Lai et al., 2014). Contrast to the use of vaccines that are administered through injection, the immersion method is much easier and quicker because the vaccination can encompass large fish numbers at once, although this vaccine types does not have long-term effects (Ma et al., 2019). Usually, the vaccines used in this method are known to come from live but weakened bacteria or a vector vaccine (Lim and Webster, 2001). Vaccines with this method are widely used on smaller fish or in the juvenile phase weighing between 0.5 to 5 g and this method is commonly used in sea ranching activities because it is effective, fast, comfortable, has a minimal level of stress on the fish and is economical (Buchmann et al., 2001).
3.4 Current status of fish vaccine and its application in sea ranching
Sea ranching is the activity of cultivating fish in the open sea by releasing young fish into their natural habitat and then harvesting them again (Kallio-Nyberg et al., 2013). However, sea ranching activities are still being discussed regarding their efficiency with one of its obstacles being the difference in hatchery conditions with natural habitats which can cause various risks to fish released into nature, such as diseases that can cause high mortality (Kavitha et al., 2022). Over time the vaccination strategy to improve health and survival of fish has been widely carried out in sea ranching activities as there are several reported cases of vaccine use in sea ranching (Table 5). With regards to the status of vaccine use in sea ranching, there are very limited information on the use of vaccines in sea ranching which is one of the reasons for the high cost of the available ones. Nevertheless, the increasing recognition of the importance of vaccines in increasing the efficiency and sustainability of this unique aquaculture practice provides enough evidence to engage in in-depth research in unearthing new vaccines (Purcell et al., 2012). In marine farming, the most commonly used vaccines are inactivated vaccines, which contain killed pathogens due to their stability and safety in the open water environment (Sommerset et al., 2005b). The right vaccine choice also depends on the pathogens present in the release area and the target species to be released. Several studies have shown that using vaccines in sea ranching can increase fish immunity when released into their natural habitat. For example, research conducted in Ireland on Atlantic salmon farms showed that vaccinated smolts had a 2-5% higher recapture rate than unvaccinated fish (López-Vázquez et al., 2023). Increasing the survival rate directly means increasing efficiency and productivity in sea ranching activities.
3.4.1 Bacterial vaccines
Bacterial vaccines are used to protect fish from dangerous infections, improve health, and reduce mortality. Aeromonas salmonicida, Yersinia ruckeri, Vibrio anguillarum, and Vibrio ordalii are common bacteria that often infect fish in both aquaculture and sea ranching activities (Gudding and Van Muiswinkel, 2013). A previous study (Buchmann et al., 1997) completed the immunization procedure on fish before being released into their natural habitat by grouping them into three groups with different treatments in each group. Briefly, as many as 22,000 pre-smolts (young fish before migrating to the sea) were vaccinated intraperitoneally (injected into the abdominal cavity) on March 27, 1996, using a polyvalent vaccine (a vaccine that can protect against several pathogens at once). The vaccine was made to fight Aeromonas salmonicida, Yersinia ruckeri, and Vibrio anguillarum (serotypes O1 and O2) without using mineral oil-based adjuvants at 0.1 mL per fish dose. This vaccine is known as Aquavac Multivac E, produced by Aquaculture Vaccines, Essex, England. In addition, another 22,000 fish were vaccinated on April 2, 1996, using a different method, namely by immersion in formalin solution containing inactivated (killed) pathogens, except for Vibrio ordalii bacteria that replaced the O2 serotype of Vibrio anguillarum in the Aquavac Triple vaccine. This immersion procedure was carried out for one hour. Meanwhile, a control group consisting of 22,000 fish was kept without vaccination. During the vaccination procedure, the water temperature was maintained at around 10°C to ensure an optimal environment for the fish. The results showed that fish that used the injection vaccine were the fish with the highest number of returns and fish that did not use the vaccine had a high mortality rate.
3.4.2 Viral vaccines
Viral vaccines play a crucial role in sea ranching, a form of aquaculture where juvenile fish are released into open marine environments and harvested at maturity. Currently, sea ranching operations utilize various types of viral vaccines, including inactivated, DNA, and recombinant vaccines, to combat major diseases such as viral pancreas disease (PD) and infectious salmon anemia (ISA) (Muñoz-Atienza, 2021). These vaccines have shown significant efficacy, with DNA vaccines demonstrating up to 85.9% relative percentage survival against VNN in European sea bass (Dhar et al., 2014). Species-specific applications include multivalent vaccines for Atlantic salmon targeting IPN, PD, and ISA, as well as DNA vaccines against VNN for European sea bass (Sommerset et al., 2005b). The integration of vaccination with selective breeding for disease resistance and other health management practices has further enhanced the effectiveness of these strategies. However, sea ranching presents unique challenges for vaccine delivery and maintaining efficacy in open-water environments. Ongoing research focuses on developing mucosal and oral vaccines for easier administration, exploring CRISPR-Cas9 technology for creating disease-resistant fish strains and improving delivery methods through nanoparticle-based systems. Future directions in this field aim to expand vaccine coverage for emerging viral threats, develop more multivalent vaccines, and improve delivery methods to address the specific needs of open-water aquaculture. As the aquaculture industry continues to grow, viral vaccines remain a critical component in ensuring the health and sustainability of sea ranching operations, protecting fish populations against major pathogens, and contributing to the overall viability of this important food production sector (Munang’andu and Evensen, 2019).
4 Conclusion
This study focuses on providing information about the importance of the role of vaccines in supporting aquaculture and sea ranching activities. The use of vaccines in aquaculture is an innovation in the field of aquaculture that continues to experience development over time. Increasing global demand for aquaculture products requires effective disease prevention measures, highlighting the importance of innovative fish vaccine technologies. Modern vaccines, such as plant-based vaccines, inactivated vaccines, subunits, recombinant protein, DNA, and live attenuated vaccines, play an important role in improving fish immunity and supporting aquaculture health. Despite challenges such as high costs and inefficiencies in traditional vaccine development methods, advances in biotechnology provide significant opportunities to address emerging diseases. In this era, vaccines are crucial in aquaculture, and they are used almost all over the world to prevent the slaughter of domesticated animals so that they can increase production yields. The integration of vaccines in marine farming has also been widely used in several cases when releasing fish seeds into natural habitats to improve fish health and reduce the risk of disease transmission. With the rapid development of sea ranching activities, it is proposed that the use of vaccines in sea ranching activities has the potential for sustainable practices, although there are scarce information. Improvements in research, rules, and public awareness are needed for the potential for sustainable vaccine use practices.
5 Future research perspectives
The application of vaccines in sea ranching operations is poised for significant progress despite limited information on vaccine use in its operations. Several researchers have emphasized the need for vaccines that support the long-term ecological balance of aquaculture operations (Adams, 2019). Sea ranching activities focus on keeping the balance of species availability in natural habitats or open waters, as a result sea ranching activities serve as a sustainable program for the future. Sea ranching activity is also closely related to the impact on fish health, which remains an investment for the future. Many researchers are increasingly focusing on the comprehensive effects of vaccines on farmed fish populations, immune responses, and overall fish health (Dadar et al., 2017). This understanding of fish health is essential to developing vaccination strategies that focus on disease prevention and improving the overall vitality of farmed fish. Vaccine development and optimization continue to be a key area for innovation.
The application of vaccines in marine farming operations is estimated to have made significant progress, although information regarding the use of vaccines in marine farming operations is still limited. Vaccine use practices in marine farms show that sustainability has become a major concern, and researchers emphasize the need for vaccines that support long-term ecological balance in aquaculture operations (Adams, 2019). Examining the large role of aquaculture in global food security and the importance of maintaining the ecological balance of the environment. Marine farming activities focus on maintaining a balance in the availability of species in natural habitats or open waters; thus showing that marine farming activities will become a sustainable program in the future with increased research and innovation that will continue to be carried out (Delphino et al., 2019).
Many researchers are increasingly focusing on the comprehensive impact of vaccines on farmed fish populations, immune responses, and overall fish health. Future research will likely explore new antigen delivery systems, better adjuvants, and vaccination strategies tailored to the unique challenges of marine farming environments (Ryan et al., 2022). Optimizing existing vaccines to increase efficacy and reduce side effects is equally important, with research aimed at refining delivery methods and dosing (Nusbaum et al., 2002).
The feasibility of implementing large-scale vaccination programs is still a significant concern, so the field of vaccine development is evolving with the development of artificial intelligence (AI), which is bringing revolutionary advances at every stage of vaccination from discovery to deployment. The study of AI technology will improve vaccine development procedures. AI can find target antigens, predict immune responses, accelerate the search for new vaccines, and improve vaccine design through advanced biological machines and computing. AI increases the efficacy of clinical trials by facilitating real-time data monitoring, optimizing trial design, improving participant recruitment, and predicting the appropriate timing of vaccine administration. However, despite these technological advances, data integrity and quality issues will face challenges, which will include model transparency, ethical and legal issues, and the need for computing resources. Further research is also needed to utilize the full potential of AI to advance research.
Future research will likely explore novel antigen delivery systems, improved adjuvants, and vaccination strategies tailored to the unique challenges of the sea ranching environment (Ryan et al., 2022). Optimizing existing vaccines to increase efficacy and reduce side effects is equally important, with studies aimed at refining delivery methods and dosing protocols (Nusbaum et al., 2002). However, economic considerations remain a significant concern, especially for small-scale operations. The feasibility of implementing large-scale vaccination programs hinges on cost-effectiveness. Future research must emphasize the development of low-cost vaccine solutions tailored to small-scale aquaculture systems, ensuring accessibility for farmers with limited resources. Affordable vaccines could improve fish health, boost production efficiency, and enhance small-scale operators’ economic resilience while contributing to sustainable aquaculture practices, as well as the potential economic benefits of disease prevention in sea ranching operations (Figure 5) (Delphino et al., 2019).
Author contributions
AA: Conceptualization, Data curation, Methodology, Visualization, Writing – original draft. KA: Conceptualization, Data curation, Methodology, Visualization, Writing – original draft, Writing – review & editing. JC: Methodology, Visualization, Writing – review & editing. YH: Project administration, Supervision, Writing – review & editing. MF: Formal analysis, Writing – review & editing. HNL: Formal analysis, Writing – review & editing. SL: Formal analysis, Writing – review & editing. XW: Conceptualization, Funding acquisition, Methodology, Resources, Supervision, Writing – review & editing. SM: Formal analysis, Writing – review & editing. BA: Formal analysis, Writing – review & editing. SS: Formal analysis, Writing – review & editing.
Funding
The author(s) declare financial support was received for the research, authorship, and/or publication of this article. The research leading to these results was financially supported by the National key research and development program of China (2024YFD2401803), the Program for Scientific Research Start-Up Funds of Guangdong Ocean University (060302022310, 060302022302, and 060302022301), Sino-Indonesian Technical Cooperation in Coastal Marine Ranching-Asian Cooperation Fund Program (12500101200021002), the National Natural Science Foundation of China (42306159), and the Guangdong Basic and Applied Basic Research Foundation (2022A1515110957).
Conflict of interest
The authors declare that the research was conducted in the absence of any commercial or financial relationships that could be construed as a potential conflict of interest.
The author(s) declared that they were an editorial board member of Frontiers, at the time of submission. This had no impact on the peer review process and the final decision.
Generative AI statement
The author(s) declare that no Generative AI was used in the creation of this manuscript.
Publisher’s note
All claims expressed in this article are solely those of the authors and do not necessarily represent those of their affiliated organizations, or those of the publisher, the editors and the reviewers. Any product that may be evaluated in this article, or claim that may be made by its manufacturer, is not guaranteed or endorsed by the publisher.
References
Adams A. (2019). Progress, challenges and opportunities in fish vaccine development. Fish Shellfish Immunol. 90, 210–214. doi: 10.1016/j.fsi.2019.04.066
Angulo C., Tello-Olea M., Reyes-Becerril M., Monreal-Escalante E., Hernández-Adame L., Angulo M., et al. (2021). Developing oral nanovaccines for fish: a modern trend to fight infectious diseases. Rev. Aquac. 13, 1172–1192. doi: 10.1111/raq.12518
Araki H., Berejikian B. A., Ford M. J., Blouin M. S. (2008). Fitness of hatchery-reared salmonids in the wild. Evol. Appl. 1, 342–355. doi: 10.1111/j.1752-4571.2008.00026.x
Arechavala-Lopez P., Sanchez-Jerez P., Bayle-Sempere J. T., Uglem I., Mladineo I. (2013). Reared fish, farmed escapees and wild fish stocks - A triangle of pathogen transmission of concern to Mediterranean aquaculture management. Aquac. Environ. Interact. 3, 153–161. doi: 10.3354/aei00060
Ashraf U., Lu Y., Lin L., Yuan J., Wang M., Liu X. (2016). Spring viraemia of carp virus: Recent advances. J. Gen. Virol. 97, 1037–1051. doi: 10.1099/jgv.0.000436
Barnes E., Cooke G. S., Lauer G. M., Chung R. T. (2023). Implementation of a controlled human infection model for evaluation of HCV vaccine candidates. Hepatology 77, 1757–1772. doi: 10.1002/hep.32632
Barnes A. C., Silayeva O., Landos M., Dong H. T., Lusiastuti A., Phuoc L. H., et al. (2022). Autogenous vaccination in aquaculture: A locally enabled solution towards reduction of the global antimicrobial resistance problem. Rev. Aquac. 14, 907–918. doi: 10.1111/raq.12633
Bartley D. M., Bondad-Reantaso M. G., Subasinghe R. P. (2006). A risk analysis framework for aquatic animal health management in marine stock enhancement programmes. Fish. Res. 80, 28–36. doi: 10.1016/j.fishres.2006.03.011
Bayliss S. C., Verner-Jeffreys D. W., Bartie K. L., Aanensen D. M., Sheppard S. K., Adams A., et al. (2017). The promise of whole genome pathogen sequencing for the molecular epidemiology of emerging aquaculture pathogens. Front. Microbiol. 8. doi: 10.3389/fmicb.2017.00121
Bernardet J.-F., Bowman J. P. (2006). “The genus flavobacterium,” in The prokaryotes: Volume 7: Proteobacteria: Delta, epsilon subclass. Eds. Dworkin M., Falkow S., Rosenberg E., Schleifer K.-H., Stackebrandt E. (New York, NY: Springer New York), 481–531. doi: 10.1007/0-387-30747-8_17
Bondad-Reantaso M. G., Subasinghe R. P., Arthur J. R., Ogawa K., Chinabut S., Adlard R., et al. (2005). Disease and health management in Asian aquaculture. Vet. Parasitol. 132, 249–272. doi: 10.1016/j.vetpar.2005.07.005
Bonilla-Aldana D. K., Dhama K., Rodriguez-Morales A. J. (2020). Revisiting the one health approach in the context of COVID-19: A look into the ecology of this emerging disease. Adv. Anim. Vet. Sci. 8, 234–237. doi: 10.17582/journal.aavs/2020/8.3.234.237
Buchmann K., Dalsgaard I., Nielsen M. E., Pedersen K., Uldal A., Garcia J. A., et al. (1997). Vaccination improves survival of Baltic salmon (Salmo salar) smolts in delayed release sea ranching (net-pen period). Aquaculture 156, 335–348. doi: 10.1016/S0044-8486(97)00097-5
Buchmann K., Larsen J. L., Therkildsen B. (2001). Improved recapture rate of vaccinated sea-ranched Atlantic salmon, Salmo salar L. J. Fish Dis. 24, 245–248. doi: 10.1046/j.1365-2761.2001.00294.x
Buckley B. S., Henschke N., Bergman H., Skidmore B., Klemm E. J., Villanueva G., et al. (2019). Impact of vaccination on antibiotic usage: a systematic review and meta-analysis. Clin. Microbiol. Infect. 25, 1213–1225. doi: 10.1016/j.cmi.2019.06.030
Buyel J. F. (2019). Plant molecular farming – integration and exploitation of side streams to achieve sustainable biomanufacturing. Front. Plant Sci. 9, 1–17. doi: 10.3389/fpls.2018.01893
Cárdenas C., Guzmán F., Carmona M., Muñoz C., Nilo L., Labra A., et al. (2020). Synthetic peptides as a promising alternative to control viral infections in atlantic salmon. Pathogens 9, 600. doi: 10.3390/pathogens9080600
Cervera L., Arizcun M., Mercado L., Chaves-Pozo E., Cuesta A. (2024). Synthetic antimicrobial Nkl and Dic peptides are immunomodulatory but only Dic peptide can be therapeutic against nodavirus infection. Fish Shellfish Immunol. 152, 109772. doi: 10.1016/j.fsi.2024.109772
Chia T. J., Wu Y. C., Chen J. Y., Chi S. C. (2010). Antimicrobial peptides (AMP) with antiviral activity against fish nodavirus. Fish Shellfish Immunol. 28, 434–439. doi: 10.1016/j.fsi.2009.11.020
Costa J. Z., Thompson K. D. (2016). Understanding the interaction between Betanodavirus and its host for the development of prophylactic measures for viral encephalopathy and retinopathy. Fish Shellfish Immunol. 53, 35–49. doi: 10.1016/j.fsi.2016.03.033
Dadar M., Dhama K., Vakharia V. N., Hoseinifar S. H., Karthik K., Tiwari R., et al. (2017). Advances in aquaculture vaccines against fish pathogens: global status and current trends. Rev. Fish. Sci. Aquac. 25, 184–217. doi: 10.1080/23308249.2016.1261277
Dar G. H., Qadri H., Bhat R. A., Al-Ghamdy K. M., Hakeem K. R. (2022). Bacterial Fish Diseases (Elsevier). doi: 10.1016/C2020-0-02560-0
Delphino M. K. V. C., Barone R. S. C., Leal C. A. G., Figueiredo H. C. P., Gardner I. A., Gonçalves V. S. P. (2019). Economic appraisal of vaccination against Streptoccocus agalactiae in Nile tilapia farms in Brazil. Prev. Vet. Med. 162, 131–135. doi: 10.1016/j.prevetmed.2018.12.003
Denkin S. M., Nelson D. R. (2004). Regulation of Vibrio Anguillarum empA metalloprotease expression and its role in virulence. Appl. Environ. Microbiol. 70, 4193–4204. doi: 10.1128/AEM.70.7.4193-4204.2004
Dhar A. K., Manna S. K., Thomas Allnutt F. C. (2014). Viral vaccines for farmed finfish. Indian J. Virol. 25, 1–17. doi: 10.1007/s13337-013-0186-4
Du Y., Hu X., Miao L., Chen J. (2022). Current status and development prospects of aquatic vaccines. Front. Immunol. 13. doi: 10.3389/fimmu.2022.1040336
Falco A., Brocal I., Pérez L., Coll J. M., Estepa A., Tafalla C. (2008). In vivo modulation of the rainbow trout (Oncorhynchus mykiss) immune response by the human alpha defensin 1, HNP1. Fish Shellfish Immunol. 24, 102–112. doi: 10.1016/j.fsi.2007.09.007
Frans I., Michiels C. W., Bossier P., Willems K. A., Lievens B., Rediers H. (2011). Vibrio anguillarum as a fish pathogen: Virulence factors, diagnosis and prevention. J. Fish Dis. 34, 643–661. doi: 10.1111/j.1365-2761.2011.01279.x
Fredriksen B. N., Sævareid K., McAuley L., Lane M. E., Bøgwald J., Dalmo R. A. (2011). Early immune responses in Atlantic salmon (Salmo salar L.) after immunization with PLGA nanoparticles loaded with a model antigen and β-glucan. Vaccine 29, 8338–8349. doi: 10.1016/j.vaccine.2011.08.087
Gámez-Valero A., Monguió-Tortajada M., Carreras-Planella L., Franquesa M., Beyer K., Borràs F. E. (2016). Size-Exclusion Chromatography-based isolation minimally alters Extracellular Vesicles’ characteristics compared to precipitating agents. Sci. Rep. 6, 1–9. doi: 10.1038/srep33641
Gauthier D. T., Rhodes M. W. (2009). Mycobacteriosis in fishes: A review. Vet. J. 180, 33–47. doi: 10.1016/j.tvjl.2008.05.012
Gillund F., Dalmo R., Tonheim T. C., Seternes T., Myhr A. I. (2008). DNA vaccination in aquaculture - Expert judgments of impacts on environment and fish health. Aquaculture 284, 25–34. doi: 10.1016/j.aquaculture.2008.07.044
Godoy M. G., Aedo A., Kibenge M. J. T., Groman D. B., Yason C. V., Grothusen H., et al. (2008). First detection, isolation and molecular characterization of infectious salmon anaemia virus associated with clinical disease in farmed Atlantic salmon (Salmo salar) in Chile. BMC Vet. Res. 4, 1–13. doi: 10.1186/1746-6148-4-28
Goossens H. (2009). Antibiotic consumption and link to resistance. Clin. Microbiol. Infect. 15, 12–15. doi: 10.1111/j.1469-0691.2009.02725.x
Gregory A. E., Titball R., Williamson D. (2013). Vaccine delivery using nanoparticles. Front. Cell. Infect. Microbiol. 4. doi: 10.3389/fcimb.2013.00013
Gudding R., Van Muiswinkel W. B. (2013). A history of fish vaccination: Science-based disease prevention in aquaculture. Fish Shellfish Immunol. 35, 1683–1688. doi: 10.1016/j.fsi.2013.09.031
Gui D. Y., Sullivan L. B., Luengo A., Hosios A. M., Bush L. N., Gitego N., et al. (2016). Environment dictates dependence on mitochondrial complex i for NAD+ and aspartate production and determines cancer cell sensitivity to metformin. Cell Metab. 24, 716–727. doi: 10.1016/j.cmet.2016.09.006
Harborne A. R. (2013). The ecology, behaviour and physiology of fishes on coral reef flats, and the potential impacts of climate change. J. Fish Biol. 83, 417–447. doi: 10.1111/jfb.12203
Heppell J., Davis H. L. (2000). Application of DNA vaccine technology to aquaculture. Adv. Drug Deliv. Rev. 43, 29–43. doi: 10.1016/S0169-409X(00)00075-2
Hwang J. Y., Kwon M. G., Seo J. S., Hwang S. D., Min J., Lee J. H., et al. (2020). Current use and management of commercial fish vaccines in Korea. Fish Shellfish Immunol. 102, 1–24. doi: 10.1016/j.fsi.2020.04.004
Idakwo J. (2022). Proceedings of the 36th Annual National Conference of the Fisheries Society of Nigeria (FISON). Fisheries Society of Nigeria, Port Harcourt, Rivers State, Nigeria, 24th–29th October 2021. Available online at: www.fison.ng.org.
Irshath A. A., Rajan A. P., Vimal S., Prabhakaran V. S., Ganesan R. (2023). Bacterial pathogenesis in various fish diseases: recent advances and specific challenges in vaccine development. Vaccines 11, 2–14. doi: 10.3390/vaccines11020470
Israngkura A., Sae-Hae S. (2002). A review of the economic impacts of aquatic animal disease. FAO fisheries technical paper, 253–286.
Kallio-Nyberg I., Jutila E., Saloniemi I., Jokikokko E. (2013). Effects of hatchery rearing and sea ranching of parents on the life history traits of released salmon offspring. Aquaculture 402–403, 76–83. doi: 10.1016/j.aquaculture.2013.03.027
Kavitha M., Jagadis I., Linga Prabu D., Kalidas C., Ranjith L., Willington S. (2022). Hatchery production of juveniles of pharaoh cuttlefish, Sepia pharaonis (Ehrenberg 1831) from stranded eggs and sea ranching along the Thoothukudi coast. Indian J. Geo-Marine Sci. 51, 322–326. doi: 10.56042/IJMS.v51i04.36962
Keller S. A., Bauer M., Manolova V., Muntwiler S., Saudan P., Bachmann M. F. (2010). Cutting edge: limited specialization of dendritic cell subsets for MHC class II-associated presentation of viral particles. J. Immunol. 184, 26–29. doi: 10.4049/jimmunol.0901540
Kumar A., Middha S. K., Menon S. V., Paital B., Gokarn S., Nelli M., et al. (2024). Current challenges of vaccination in fish health management. Animals 14, 1–28. doi: 10.3390/ani14182692
Labh S. N., Shakya S. R. (2014). Application of immunostimulants as an alternative to vaccines for health management in aquaculture. Int. J. Fish. Aquat. Stud. 2 (1), 153–156.
Lai S. F., Chien C. C., Chen W. C., Chen H. H., Chen Y. Y., Wang C. L., et al. (2013). Very small photoluminescent gold nanoparticles for multimodality biomedical imaging. Biotechnol. Adv. 31, 362–368. doi: 10.1016/j.bioteChadv.2012.05.005
Lai Y. X., Jin B. L., Xu Y., Huang L., Huang R. Q., Zhang Y., et al. (2014). Immune responses of orange-spotted grouper, Epinephelus coioides, against virus-like particles of betanodavirus produced in Escherichia coli. Vet. Immunol. Immunopathol. 157, 87–96. doi: 10.1016/j.vetimm.2013.10.003
Laith A. A., Abdullah M. A., Nurhafizah W. W. I., Hussein H. A., Aya J., Effendy A. W. M., et al. (2019). Efficacy of live tenuated vaccine derived from the Streptococcus agalactiae on the immune responses of Oreochromis niloticus. Fish Shellfish Immunol. 90, 235–243. doi: 10.1016/j.fsi.2019.04.052
Lim C., Webster C. D. (2001). Nutrition and Fish Health (392). Boca Raton: CRC Press. doi: 10.1201/9781439800041
Loneragan N. R., Jenkins G. I., Taylor M. D. (2013). Marine stock enhancement, restocking, and sea ranching in Australia: future directions and a synthesis of two decades of research and development. Rev. Fish. Sci. 21, 222–236. doi: 10.1080/10641262.2013.796810
López-Vázquez C., Souto S., Olveira J. G., Riaza A., González Ó., Brea C., et al. (2023). Nervous necrosis virus (NNV) booster vaccination increases Senegalese sole survival and enhances immunoprotection. Animals 13, 2–16. doi: 10.3390/ani13010051
Lu J., Fang W., Huang J., Li S. (2021). The application of genome editing technology in fish. Mar. Life Sci. Technol. 3, 326–346. doi: 10.1007/s42995-021-00091-1
Lu L., Xu H., He Y., Li J. (2011). Protection of grass carp, Ctenopharyngon idellus (Valenciennes), through oral administration of a subunit vaccine against reovirus. J. Fish Dis. 34, 939–942. doi: 10.1111/j.1365-2761.2011.01310.x
Luu V. T., Moon H. Y., Hwang J. Y., Kang B. K., Kang H. A. (2017). Development of recombinant Yarrowia lipolytica producing virus-like particles of a fish nervous necrosis virus. J. Microbiol. 55, 655–664. doi: 10.1007/s12275-017-7218-5
Ma J., Bruce T. J., Jones E. M., Cain K. D. (2019). A review of fish vaccine development strategies: Conventional methods and modern biotechnological approaches. Microorganisms 7, 2–18. doi: 10.3390/microorganisms7110569
Mackinnon A. M. (2005). Use of Arthrobacter davidanieli as a live vaccine against Renibacterium salmoninarum and Piscirickettsia salmonis in salmonids. 121, 189–197.
McKenzie D. J., Axelsson M., Chabot D., Claireaux G., Cooke S. J., Corner R. A., et al. (2016). Conservation physiology of marine fishes: State of the art and prospects for policy. Conserv. Physiol. 4, 1–20. doi: 10.1093/conphys/cow046
Melnick J. L. (1978). Advantages and disadvantages of killed and live poliomyelitis vaccines. Bull. World Health Organ. 56, 21–38.
Miccoli A., Manni M., Picchietti S., Scapigliati G. (2021). State-of-the-art vaccine research for aquaculture use: The case of three economically relevant fish species. Vaccines 9, 1–29. doi: 10.3390/vaccines9020140
Mohamad A., Zamri-Saad M., Amal M. N. A., Al-Saari N., Monir M. S., Chin Y. K., et al. (2021). Vaccine efficacy of a newly developed feed-based whole-cell polyvalent vaccine against vibriosis, streptococcosis and motile aeromonad septicemia in asian seabass, lates calcarifer. Vaccines 9, 2–22. doi: 10.3390/vaccines9040368
Monaghan K. G., Wiktor A., Van Dyke D. L. (2002). Diagnostic testing for prader-willi syndrome and angelman syndrome: A cost comparison. Genet. Med. 4, 448–450. doi: 10.1097/00125817-200211000-00009
Mondal H., Thomas J. (2022). A review on the recent advances and application of vaccines against fish pathogens in aquaculture. Aquac. Int. 30, 1971–2000. doi: 10.1007/s10499-022-00884-w
Muheem A., Shakeel F., Jahangir M. A., Anwar M., Mallick N., Jain G. K., et al. (2016). A review on the strategies for oral delivery of proteins and peptides and their clinical perspectives. Saudi Pharm. J. 24, 414–425. doi: 10.1016/j.jsps.2014.06.004
Munang’andu H. M., Evensen Ø. (2019). Correlates of protective immunity for fish vaccines. Fish Shellfish Immunol. 85, 132–140. doi: 10.1016/j.fsi.2018.03.060
Munang’andu H. M., Fredriksen B. N., Mutoloki S., Brudeseth B., Kuo T. Y., Marjara I. S., et al. (2012). Comparison of vaccine efficacy for different antigen delivery systems for infectious pancreatic necrosis virus vaccines in Atlantic salmon (Salmo salar L.) in a cohabitation challenge model. Vaccine 30, 4007–4016. doi: 10.1016/j.vaccine.2012.04.039
Muñoz-Atienza E. (2021). Systemic and mucosal B and T cell responses upon mucosal vaccination of teleost fish. Front. Immunol. 11. doi: 10.3389/fimmu.2020.622377
Mutoloki S., Munang’andu H. M., Evensen Ø. (2015). Oral vaccination of fish - antigen preparations, uptake, and immune induction. Front. Immunol. 6. doi: 10.3389/fimmu.2015.00519
Naiel M. A. E., Ghazanfar S., Negm S. S., Shukry M., Abdel-Latif H. M. R. (2023). Applications of antimicrobial peptides (AMPs) as an alternative to antibiotic use in aquaculture - A mini-review. Ann. Anim. Sci. 23, 691–701. doi: 10.2478/aoas-2022-0090
Nandal N., Malik R., Dhingra M. (2021). Advances in Biotechnology and Molecular Biology. Eds. Manam V., Shankarishan P.. Weser Books.
Newman S. G. (1993). Bacterial vaccines for fish. Annu. Rev. Fish Dis. 3, 145–185. doi: 10.1016/0959-8030(93)90033-8
Nishizawa T., Iida H., Takano R., Isshiki T., Nakajima K., Muroga K. (2002). Genetic relatedness among Japanese, American and European isolates of viral hemorrhagic septicemia virus (VHSV) based on partial G and P genes. Dis. Aquat. Organ. 48, 143–148. doi: 10.3354/dao048143
Nitimulyo K. H., Isnansetyo A., Triyanto T., Murdjani M., Sholichah L. (2005). Effectiveness of polyvalent vaccines for controlling vibriosis in rat group (Cromileptes altivelis). J. Perikan. Univ. Gadjah Mada 7, 95. doi: 10.22146/jfs.9056
Noad R., Roy P. (2003). Virus-like particles as immunogens. Trends Microbiol. 11, 438–444. doi: 10.1016/S0966-842X(03)00208-7
Nunnally B. K., Turula V. E., Sitrin R. D. (2015). Vaccine analysis: Strategies, principles, and control. Berlin Heidelberg, Germany: Springer-Verlag Berlin Heidelberg. doi: 10.1007/978-3-662-45024-6
Nusbaum K. E., Smith B. F., DeInnocentes P., Bird R. C. (2002). Protective immunity induced by DNA vaccination of channel catfish with early and late transcripts of the channel catfish herpesvirus (IHV-1). Vet. Immunol. Immunopathol. 84, 151–168. doi: 10.1016/S0165-2427(01)00399-3
Papadopoulos P., Bitchava Κ., Tzironi E., Athanassopoulou F., Παπαδόπουλος Π., Μπιτχαβά Κ., et al. (2008). Fish vaccination. doi: 10.12681/jhvms.14965
Preena P. G., Swaminathan T. R., Kumar V. J. R., Singh I. S. B. (2020). Antimicrobial resistance in aquaculture: a crisis for concern. Biologia (Bratisl). 75, 1497–1517. doi: 10.2478/s11756-020-00456-4
Purcell S. W., Hair C. A., Mills D. J. (2012). Sea cucumber culture, farming and sea ranching in the tropics: Progress, problems and opportunities. Aquaculture 368–369, 68–81. doi: 10.1016/j.aquaculture.2012.08.053
Radhakrishnan A., Vaseeharan B., Ramasamy P., Jeyachandran S. (2023). Oral vaccination for sustainable disease prevention in aquaculture—an encapsulation approach. Aquac. Int. 31, 867–891. doi: 10.1007/s10499-022-01004-4
Rees S. E., Ashley M., Evans L., Mangi S., Sheehan E. V., Mullier T., et al. (2021). An evaluation of the social and economic impact of a Marine Protected Area on commercial fisheries. Fish. Res. 235, 1–30. doi: 10.1016/j.fishres.2020.105819
Ringø E., Olsen R. E., Jensen I., Romero J., Lauzon H. L. (2014). Application of vaccines and dietary supplements in aquaculture: possibilities and challenges. Rev. Fish Biol. Fish. 24, 1005–1032. doi: 10.1007/s11160-014-9361-y
Rombout J. H. W. M., Kiron V. (2014). Mucosal Vaccination of Fish. John Wiley Sons, Ltd. 9780470674. 6, 56–67. doi: 10.1002/9781118806913.ch6
Ryan F. J., Hope C. M., Masavuli M. G., Lynn M. A., Mekonnen Z. A., Yeow A. E. L., et al. (2022). Long-term perturbation of the peripheral immune system months after SARS-CoV-2 infection. BMC Med. 20, 1–23. doi: 10.1186/s12916-021-02228-6
Schönherz A. A., Forsberg R., Guldbrandtsen B., Buitenhuis A. J., Einer-Jensen K. (2018). Introduction of viral hemorrhagic septicemia virus into freshwater cultured rainbow trout is followed by bursts of adaptive evolution. J. Virol. 92, 1–20. doi: 10.1128/jvi.00436-18
Shahid N., Daniell H. (2016). Plant-based oral vaccines against zoonotic and non-zoonotic diseases. Plant Biotechnol. J. 14, 2079–2099. doi: 10.1111/pbi.12604
Shoemaker C. A., Klesius P. H., Drennan J. D., Evans J. J. (2011). Efficacy of a modified live Flavobacterium columnare vaccine in fish. Fish & Shellfish Immunology. 30, 304–308. doi: 10.1016/j.fsi.2010.11.001
Smith A. J., Adams M. B., Crosbie P. B. B., Nowak B. F., Bridle A. R. (2022). Size-dependent resistance to amoebic gill disease in naïve atlantic salmon (Salmo salar). Fish Shellfish Immunol. 122, 437–445. doi: 10.1016/j.fsi.2022.02.035
Sommerset I., Krossøy B., Biering E., Frost P. (2005a). Vaccines for fish in aquaculture. Expert Rev. Vaccines 4, 89–101. doi: 10.1586/14760584.4.1.89
Sommerset I., Skern R., Biering E., Bleie H., Fiksdal I. U., Grove S., et al. (2005b). Protection against Atlantic halibut nodavirus in turbot is induced by recombinant capsid protein vaccination but not following DNA vaccination. Fish Shellfish Immunol. 18, 13–29. doi: 10.1016/j.fsi.2004.03.006
Stentiford A., Bateman G., Hinchliffe I. (2020). Sustainable aquaculture through the One Health lens. Nat. Food 1 (8), 468–474. doi: 10.1038/s43016-020-0127-5
Su H., Yakovlev I. A., van Eerde A., Su J., Clarke J. L. (2021). Plant-produced vaccines: future applications in aquaculture. Front. Plant Sci. 12. doi: 10.3389/fpls.2021.718775
Subasinghe R. P., Delamare-Deboutteville J., Mohan C. V., Phillips M. J. (2019). Vulnerabilities in aquatic animal production. Rev. Sci. Tech. 38, 423–436. doi: 10.20506/rst.38.2.2996
Tamer C., Cavunt A., Durmaz Y., Ozan E., Kadi H., Kalayci G., et al. (2021). Inactivated infectious pancreatic necrosis virus (IPNV) vaccine and E. coli-expressed recombinant IPNV-VP2 subunit vaccine afford protection against IPNV challenge in rainbow trout. Fish Shellfish Immunol. 115, 205–211. doi: 10.1016/j.fsi.2021.06.002
Tammas I., Bitchava K., Gelasakis A. I. (2024). Transforming aquaculture through vaccination: A review on recent developments and milestones. Vaccines 12, 1–38. doi: 10.3390/vaccines12070732
Terech-Majewska E. (2016). Improving disease prevention and treatment in controlled fish culture. Arch. Polish Fish. 24, 115–165. doi: 10.1515/aopf-2016-0013
Thu Lan N. G., Salin K. R., Longyant S., Senapin S., Dong H. T. (2021). Systemic and mucosal antibody response of freshwater cultured Asian seabass (Lates calcarifer) to monovalent and bivalent vaccines against Streptococcus agalactiae and Streptococcus iniae. Fish Shellfish Immunol. 108, 7–13. doi: 10.1016/j.fsi.2020.11.014
Tournaire M., Lepercq J. (1995). Avantages et inconvenients des conferences de consensus. Contracept. Fertil. Sex 23, 587–589.
Välimaa A. L., Mäkinen S., Mattila P., Marnila P., Pihlanto A., Mäki M., et al. (2019). Fish and fish side streams are valuable sources of high-value components. Food Qual. Saf. 3, 209–226. doi: 10.1093/fqsafe/fyz024
Van Muiswinkel W. B. (2008). A history of fish immunology and vaccination I. The early days. Fish Shellfish Immunol. 25, 397–408. doi: 10.1016/j.fsi.2008.02.019
Ventola C. L. (2015). The antibiotic resistance crisis: part 1: causes and threats. Pharmacy and Therapeutics 40 (4), 277–283.
Vinay T. N., Bhat S., Gon Choudhury T., Paria A., Jung M. H., Shivani Kallappa G., et al. (2018). Recent advances in application of nanoparticles in fish vaccine delivery. Rev. Fish. Sci. Aquac. 26, 29–41. doi: 10.1080/23308249.2017.1334625
Walsh H. L., Gerdts V., Babiuk L. A. (2020). Veterinary and aquatic diseases. Adv. Exp. Med. Biol. - Adv. Microbiol. Infect. Dis. Public Heal. 3, 811–830. doi: 10.1016/B978-0-12-811924-2.00048-1
Waltzek T. B., Kelley G. O., Stone D. M., Way K., Hanson L., Fukuda H., et al. (2005). Koi herpesvirus represents a third cyprinid herpesvirus (CyHV-3) in the family herpesviridae. J. Gen. Virol. 86, 1659–1667. doi: 10.1099/vir.0.80982-0
Wang Q., Ji W., Xu Z. (2020). Current use and development of fish vaccines in China. Fish Shellfish Immunol. 96, 223–234. doi: 10.1016/j.fsi.2019.12.010
Wessel Ø., Haugland Ø., Rode M., Fredriksen B. N., Dahle M. K., Rimstad E. (2018). Inactivated Piscine orthoreovirus vaccine protects against heart and skeletal muscle inflammation in Atlantic salmon. J. Fish Dis. 41, 1411–1419. doi: 10.1111/jfd.12835
Williams T. C., Ayrapetyan M., Oliver J. D. (2015). Molecular and physical factors that influence attachment of vibrio vulnificus to chitin. Appl. Environ. Microbiol. 81, 6158–6165. doi: 10.1128/AEM.00753-15
Wolf K., Quimby M. C. (1962). Established eurythermic line of fish cells in vitro. Science 135, 1065–1066. doi: 10.1126/science.135.3508.1065
Yusibov V., Streatfield S. J., Kushnir N. (2011). Clinical development of plant-produced recombinant pharmaceuticals: Vaccines, antibodies and beyond. Hum. Vaccin. 7, 313–321. doi: 10.4161/hv.7.3.14207
Zhang Z. (2021). Research advances on tilapia streptococcosis. Pathogens 10. doi: 10.3390/pathogens10050558
Keywords: aquaculture, sea ranching, vaccine, disease control, sustainable fisheries, antimicrobial resistance
Citation: Alfatat A, Amoah K, Cai J, Huang Y, Fachri M, Lauden HN, Lyu S, Wang X, Maulu S, Asiedu B and Syaifiuddin S (2025) Sustainable aquaculture and sea ranching with the use of vaccines: a review. Front. Mar. Sci. 11:1526425. doi: 10.3389/fmars.2024.1526425
Received: 11 November 2024; Accepted: 03 December 2024;
Published: 10 January 2025.
Edited by:
Mohammed Fouad El Basuini, Tanta University, EgyptReviewed by:
Akram Shehata, Alexandria University, EgyptMayada Alhoshy, Fujian Agriculture and Forestry University, China
Copyright © 2025 Alfatat, Amoah, Cai, Huang, Fachri, Lauden, Lyu, Wang, Maulu, Asiedu and Syaifiuddin. This is an open-access article distributed under the terms of the Creative Commons Attribution License (CC BY). The use, distribution or reproduction in other forums is permitted, provided the original author(s) and the copyright owner(s) are credited and that the original publication in this journal is cited, in accordance with accepted academic practice. No use, distribution or reproduction is permitted which does not comply with these terms.
*Correspondence: Kwaku Amoah, YW1vYWhrMjAxMEB5YWhvby5jb20=; Xuefeng Wang, eHVlZmVuZzE5OTlAMTI2LmNvbQ==