- Department of Marine Systems, Tallinn University of Technology, Tallinn, Estonia
A hydrodynamic model coupled with a particle tracking model was used to identify the pathways and accumulation areas of microplastics (MP) in the Gulf of Finland (GoF) over a three-year period (2018-2020). Two key sources, wastewater treatment plants (WWTPs) and rivers, were considered, focusing on polypropylene (PP)/polyethylene (PE) and polyethylene terephthalate (PET) particles sized 20-500 μm. Rivers contribute 76% of total MP entering the gulf, while WWTPs account for the remaining 24%. Most of the MP accumulates inside the gulf and does not drift to the Baltic Proper. The eastern part of the gulf exhibits the highest surface concentrations of particles influenced by the Neva River. In the water column, MP concentrations were notably high in shallow coastal areas, decreasing gradually offshore. Potential MP accumulation zones were identified primarily between longitudes 28°E and 30°E, particularly near the major rivers Narva and Kymi and in the easternmost gulf related to the Neva River discharge. The MP concentrations in the surface layer and water column were higher in winter while settling was more intense in summer. Short-term variability in the surface layer was caused by (sub)mesoscale advection and divergence/convergence, while in the near-bottom layer, strong bottom currents and consequent resuspension elevated the concentrations.
1 Introduction
Microplastics (MP), which are particles smaller than 5 mm, can be found in various aquatic environments, including the oceans, seas, estuaries and rivers (Cole et al., 2011; Jambeck et al., 2015; Setälä et al., 2016; Mishra et al., 2022; Matjašič et al., 2023). The significant increase in plastic production since the early 1970s has raised numerous concerns about plastic pollution in aquatic systems. It has been estimated that over 170 trillion plastic particles are floating in the world’s oceans (Eriksen et al., 2023), and their presence is also increasing in the seabed, coastlines, and marine biota (Barnes et al., 2009; Suaria and Aliani, 2014; Llorca et al., 2020; Matjašič et al., 2023). Despite substantial efforts and initiatives to reduce plastic usage, global annual plastic waste production is projected to continue rising in the coming years. By 2025, the United Nations Sustainable Development Goal 14.1 aims to reduce marine pollution, including plastics.
In Europe, The Marine Strategy Framework Directive (2008/56/EC, European Commission, 2008) (MFSD) identified anthropogenic litter as a dominant pressure and a main source of impact on coastal habitats. The MSFD establishes requirements for the EU (European Union) member states to achieve and maintain a good environmental status in their marine environments, as well as to prevent any future deterioration including the MSFD descriptor D10. In addition, the European Chemical Agency (EGCHA) has proposed restriction of MP in many products within the EU/EEA (European Economic Area) region, with the goal of preventing or minimizing their discharge into the environment (European Chemicals Agency, 2019). In 2021, the European Union also banned single-use plastics within its member states (Harvey and Watts, 2018).
According to GESAMP (2019), the marine environment can be infiltrated by plastic through multiple entry points, including riverine systems, shoreline activities, shipping, and atmospheric deposition. Various studies (Ziajahromi et al., 2016; Mintenig et al., 2017; Kay et al., 2018; Prata, 2018; Schernewski et al., 2020) have highlighted the significant influence of human activities on MP deposition. Among these activities, Wastewater Treatment Plants (WWTPs) are recognized as a significant emission pathway. For example, Municipal WWTPs have shown high efficiency in removing MP (Carr et al., 2016; Talvitie et al., 2017; Gies et al., 2018); however, untreated WWTP effluents exhibit elevated MP concentrations (Sun et al., 2019; Schernewski et al., 2020). Baresel and Olshammar (2019) proposed that MP retention in WWTPs based on their respective treatment stages ranges from 85% to 98% in the Baltic Sea region. Despite this relatively high overall removal efficiency, WWTPs are still considered a significant MP emission pathway in the Baltic Sea region due to the substantial volumes of wastewater they process (Baresel and Olshammar, 2019). In the Baltic Sea, wastewater and stormwater plants are typically separated (Schernewski et al., 2020). Sewer overflows, comprising stormwater and untreated wastewater can substantially contribute to the MP load in the environment (Magnusson, 2016; Dris et al., 2018). During periods of heavy precipitation, stormwater serves as a critical entry point for MP into the aquatic environment. Baresel and Olshammar (2019) suggest that the yearly discharge from sewer overflows is comparable in magnitude to that of treated wastewater.
Several studies have indicated that rivers are a primary source of MP and play a crucial role in transporting plastic waste into oceans (Jambeck et al., 2015; Siegfried et al., 2017; Schrank et al., 2022). Rivers flowing through highly populated cities with significant industrial activity along their banks may serve as an important source of MP in the estuarine bays such as the Gulf of Finland (GoF) in the Baltic Sea (Martyanov et al., 2021). It has been estimated that between 1.15 and 2.41 million tons or more of plastics are deposited annually into oceans via rivers (Lebreton et al., 2017; Schmidt et al., 2017). Numerous studies have discussed the pollution patterns of large rivers and provided insights into the regional and global factors responsible for MP pollution in the water column and sediments (Matjašič et al., 2023). High variability in MP concentration can be seen in both the water column and sediments depending on factors such as sampling methodology, anthropogenic activities and the size of the catchment area (She et al., 2022; Matjašič et al., 2023). Additionally, a significant proportion of marine beach litter is attributed to the input of plastic waste into rivers (Veerasingam et al., 2016). However, it is important to note that this study does not consider river retention in its analysis.
The Baltic Sea, located in northern Europe, is known as one of the largest brackish water bodies in the world (HELCOM, 2023). With a catchment area four time larger than its surface area (372,858 km2) (Marko and Urs, 2013) and an average depth of 55m, the Baltic Sea faces significant challenges related to marine litter (HELCOM, 2023). Coastal areas along the Baltic Sea exhibit significant concentration of beach litter (HELCOM, 2023). Plastic materials make up the most frequently encountered marine litter in the Baltic Sea (HELCOM, 2023). As of 2023, HELCOM’s aim to substantially decrease plastic waste and mitigate its harmful effects on coastal and marine ecosystems remains unfulfilled (HELCOM, 2023). The Baltic Sea receives a substantial volume of water from various rivers, with an average combined flow rate of approximately 14,085 m3/s (Meier and Kauker, 2003). Due to the extended residence time of pollutants in the Baltic Sea during the water renewal period, which can last up to 30 years (Leppäranta and Myrberg, 2009), the pollutants present in the Baltic Sea have a significant impact on the aquatic environment. Consequently, it is reasonable to assume that the Baltic Sea serves as a major hotspot for plastics, primarily through river discharge. Large impacts of riverine inputs as critical pathways for plastics into marine environments has also been identified in other parts of the world (Vianello et al., 2018; Uaciquete et al., 2024).
The GoF is an elongated estuarine basin situated in the northeastern region of the Baltic Sea with an average depth of 37 m and a maximum depth of 123 m (Leppäranta and Myrberg, 2009). The gulf stretches approximately 400 km in length, with a width that varies between 48 and 135 km (Alenius et al., 1998). There is a free water exchange between the GoF and BP at the western border, and fresh water is discharged mostly to the eastern part of the GoF. Several studies have reported the presence of MP in the GoF (Lips et al., 2020; Setälä et al., 2016; Uurasjärvi et al., 2021; Mishra et al., 2022). However, the knowledge about the spatial and temporal variation of MPs in the Baltic Sea is limited (Aigars et al., 2021). In addition, the methodology for acquiring information about MPs can vary based on the instruments utilized, mesh size, sampling depth, and the extent of the sampling area (Mishra et al., 2022; She et al., 2022).
Modeling the movement and fate of MP is particularly relevant in semi-enclosed systems like the Gof, where limited exchange and localized inputs contribute to accumulation of marine debris (Tsiaras et al., 2021). Eulerian and Lagrangian models are commonly used in such simulations (Bigdeli et al., 2022). Lagrangian modeling, also known as particle tracking modeling, tracks individual particles (Siht et al., 2025, in press), while an Eulerian approach considers advection and diffusion at specific locations (Bigdeli et al., 2022). Pärn et al. (2023) employed a combination of a hydrodynamic model and a particle tracking model to understand the transport and fate of marine litter including accumulation areas in the Baltic Sea. Martyanov et al. (2023) considered different initial fall velocities of suspended MP to study their distribution in the eastern GoF. Schernewski et al. (2021) incorporated emission scenarios from WWTPs and combined sewerage plants into their model to estimate the fate of plastics in the Baltic Sea environment. The GETM (General Estuarine Transport Model) ocean circulation model has been utilized in several studies, including those conducted by Schernewski et al. (2021) and Osinski et al. (2020), to analyze the transportation of MP in the Baltic Sea. However, these studies did not include the impact of biofouling on the buoyancy of floating MP and their removal process through sinking and sedimentation (Osinski et al., 2020; Schernewski et al., 2021). Modeling studies in the Baltic Sea (Martyanov et al., 2021; Frishfelds et al., 2022; Murawski et al., 2022), North Sea (Cuttat, 2018), and Mediterranean Sea (Tsiaras et al., 2021) have incorporated biofouling of MP particles that is important to simulate their fate in the marine environment accurately (Murawski et al., 2022).
The objective of this study is to provide an overview of the pathways and accumulation areas of MP in the GoF using a multi-year high-resolution model simulation and realistic loads from the rivers and WWTPs. We chose Lagrangian particle tracking model approach, describing MP as Super-Individuals (SI; (Scheffer et al., 1995) to improve computational efficiency, with each SI representing a group of particles. We have also conducted a series of sensitivity experiments aimed at gaining a deeper insight into the impact of various processes, such as mixing, beaching, resuspension, and biofouling (Siht et al., 2025, in press). In the present study, a 3-year model simulation was conducted to identify potential MP accumulation patterns in the surface layer, water column and sediments.
The paper is organized as follows: it begins with a description of the hydrodynamic model, biogeochemistry model, and Lagrangian particle tracking model, along with MP input data sets. It is followed by an analysis of the model results, aiming to uncover the MP pathways and accumulation areas in the GoF. Finally, the results are discussed, and conclusions are derived.
2 Materials and methods
2.1 Hydrodynamic model and setup
General Estuarine Transport Model (GETM) (Burchard and Bolding, 2002) has been used to simulate the circulation and density fields of the Baltic Sea and GoF in this study. GETM is a hydrostatic, three-dimensional primitive equation model that has embedded adaptive vertical coordinates (Hofmeister et al., 2010; Klingbeil et al., 2018), which significantly reduces the numerical mixing in the simulations (Gräwe et al., 2015). The vertical mixing (viscosity and diffusion) in the GETM is calculated with two equation k-ε model via coupling with General Ocean Turbulence Model (GOTM) (Burchard, 2001; Canuto et al., 2001) and the sub-grid horizontal mixing with Smagorinsky parameterization (Smagorinsky, 1963).
The biogeochemistry model ERGOM (Neumann et al., 2002; Neumann and Schernewski, 2008) is coupled with the hydrodynamic model via Framework Aquatic Biogeochemical Models (FABM; Bruggeman and Bolding, 2014) and has been used to calculate the chlorophyll-a concentration for biofouling of MP in the Gulf of Finland. In short, ERGOM has 12 state variables and describes a nitrogen and phosphorus cycle, although part of the phosphorus is considered with the N:P ratio (Redfield, 1934). More details about the ERGOM model can be found from in (Radtke et al., 2019; Neumann et al., 2022) and references therein.
We are using a three-level nested modelling system. The whole Baltic Sea has been simulated with a horizontal grid step of 1 nautical mile (approximately 1852 m) and 50 adaptive vertical layers (Gräwe et al., 2015). Medium-resolution model based on the settings described in Zhurbas et al. (2018) and Liblik et al. (2020, 2022) has a horizontal grid spacing of 0.5 nautical miles and covers the central Baltic Proper along with the Gulf of Finland and the Gulf of Riga. The high-resolution model covers the Gulf of Finland and has a horizontal grid spacing of 0.125 nautical miles. The number of adaptive layers in medium- and high-resolution runs is 60. Spatially interpolated results with hourly resolution from the coarse-resolution model are used for the boundary conditions in the medium-resolution model and from the medium-resolution model are used for the boundary conditions in the high-resolution model.
Atmospheric forcing at the sea surface (wind stress and heat flux) is calculated offline from the ERA5 re-analysis (Hersbach et al., 2020). Freshwater input to the models is based on the runoff data compiled for the Baltic Model Intercomparison Project (Gröger et al., 2022) by Väli et al. (2019) and Estonian rivers have been corrected by the input estimates from EstModel (https://estmodel.app/en/#/estimates, last access 10.09.2023).
The simulation period for the high-resolution model was from 2018 to mid-2021. The runs were initially started from a motionless state, i.e. current velocity components and sea surface height were set to zero. Previous studies have shown that the adjustment of the wind-driven circulation in the Baltic Sea takes only a few days (e.g. Krauss and Brügge, 1991; Lips et al., 2016).
For more details of the model setup and validation, the reader is referred to (Siht et al 2025, in press).
2.2 Lagrangian particle model
We employed the Lagrangian particle tracking model described by (Siht et al 2025, in review) to track virtual MP particles. The particle tracking model used the 12-hour 3-dimensional output of the high-resolution GETM setup for particle transport. Beyond advection, our model accounted for several additional processes: 1) dispersion, 2) beaching, 3) biofouling, and 4) resuspension.
Our model computed the horizontal diffusion coefficient for particle dispersion based on the current shear velocity, following the Smagorinsky method (Smagorinsky, 1963). Here, we set the Smagorinsky coefficient Cs to 0.2.
Beaching was implemented through a timer-based approach, where particles became beached after a specified duration in the beach zone. In our simulations, all particle types shared a uniform beaching time of 10 days. The beaching zone was defined as the sea cell nearest to the shoreline (i.e., 250m). Resuspension from beaches was not implemented, i.e., once beached, the particle remained still and was effectively removed from the simulation.
Following Murawski et al. (2022), biofouling is described as a saturated growth process that depends on the maximum biofilm thickness and the growth time scale. The biofouling process was initiated when chlorophyll-a concentration exceeded 1.1 mg m-3. In the current simulations, the maximum biofilm thickness was set to 6.7% of the initial particle radius, and the growth time scale was set to 20 days.
Negatively buoyant particles could settle and be resuspended when the critical shear velocity was exceeded. The vertical velocity gained from resuspension was proportional to the local bottom friction velocity.
The simulation period for the particle tracking model was from 2018-02-05 to 2021-01-01. New particle coordinates were calculated with a time step of 600 seconds. At each time step, the current velocity components (and other hydrological parameters) were interpolated in time and space to the exact particle locations. The particle coordinates were saved at 12-hour intervals. A total of approximately 146 million particles were released during the simulation.
2.2.1 Calculations
The concentrations of MP particles in the surface layer were defined for the water layer from the sea surface to the geopotential height of -1 m. The water column was defined as extending from the sea surface to the uppermost layer of the seabed, and the concentrations were integrated over the entire column. The meridionally integrated values refer to the temporal average of integrated values from south to north, which essentially describes the cross-sectional profile of the entire model domain. All mean concentration fields were spatially smoothed with a 2.5 km window to reduce the high-frequency variability. The size of the window aligns with the local baroclinic Rossby radius of approximately 2-4 km (Alenius et al., 2003). The results presented in the current study are based on two model years (2019-2020) after a spin-up period of one year.
2.3 Emission scenarios
2.3.1 Microplastics sources and emission calculations
The fate of MP in the marine environment relies heavily on the density of plastics. Density serves as a determining factor in classifying plastics into two main categories: floating and sinking types. Floating plastics consist of high- and low-density polyethylene (PE) with a density range of 915–970 kg/m3, as well as polypropylene (PP) with a density range of 890–920 kg/m3 (Schernewski et al., 2020). Sinking plastics include rigid polyvinyl chloride (PVC) with a density range of 1300–1450 kg/m3 and polyethylene terephthalate (PET) with a density of 1380 kg/m3 (Schernewski et al., 2020). PP, PE, and PET are the most prevalent plastics observed in aquatic environments (Kooi and Koelmans, 2019). In this study, two main sources of plastics were considered:
1. a. MP inputs from WWTPs were estimated based on the study by Schernewski et al. (2020). The MP load into the GoF catchment (Figures 1A, B) was calculated using treated wastewater discharge data and particle concentrations in raw water. The average minimum and maximum MP concentrations in raw wastewater were based on literature (Schernewski et al., 2020), and we considered maximum MP concentrations (Figure 1C), and river retention was not taken into consideration.
2. b. MP inputs from rivers were selected as another major source of marine plastic pollution because they are responsible for a high level of land-based sources, such as mismanaged waste (Jambeck et al., 2015). The variability in observed MP concentrations in rivers is notable (Constant et al., 2020) partly caused by the choice of sampling method, the type of instrument used, the lower size limit of MPs being sampled, the season during which sampling takes place, and the specific processing and analysis methods employed. According to Schrank et al. (2022), surface water samples from the Danube River had an average concentration of 48.7 particles/m3. The average concentration of plastic in the Têt River was 42 particles/m3 (Constant et al., 2020), while the Narva River had an average of 47 particles/m3 (Lips et al., 2020). Based on the above literature and assuming that only a quarter comes from WWTPs (Schernewski et al., 2020), in this study, we considered the MP amount in the rivers (without contribution of WWTPs) to be 35 particles/m3. We calculated the daily load of MP from river sources (particles/day) by multiplying the concentration of 35 particles/m3 by the river discharge in the GoF (Figure 1D).
The floating and sinking behavior of MP is not only determined by their density but is also influenced by their size and shape. In our study, we focused on MP with a size range of 20–500 μm, which we divided into two classes: 20-200 and 200-500 μm. Based on Schernewski et al. (2021) and Kuddithamby et al. (2024), we assumed that 90% of the MP would fall into the 20-200 μm size class. Additionally, we assumed that the MP had a spherical shape.
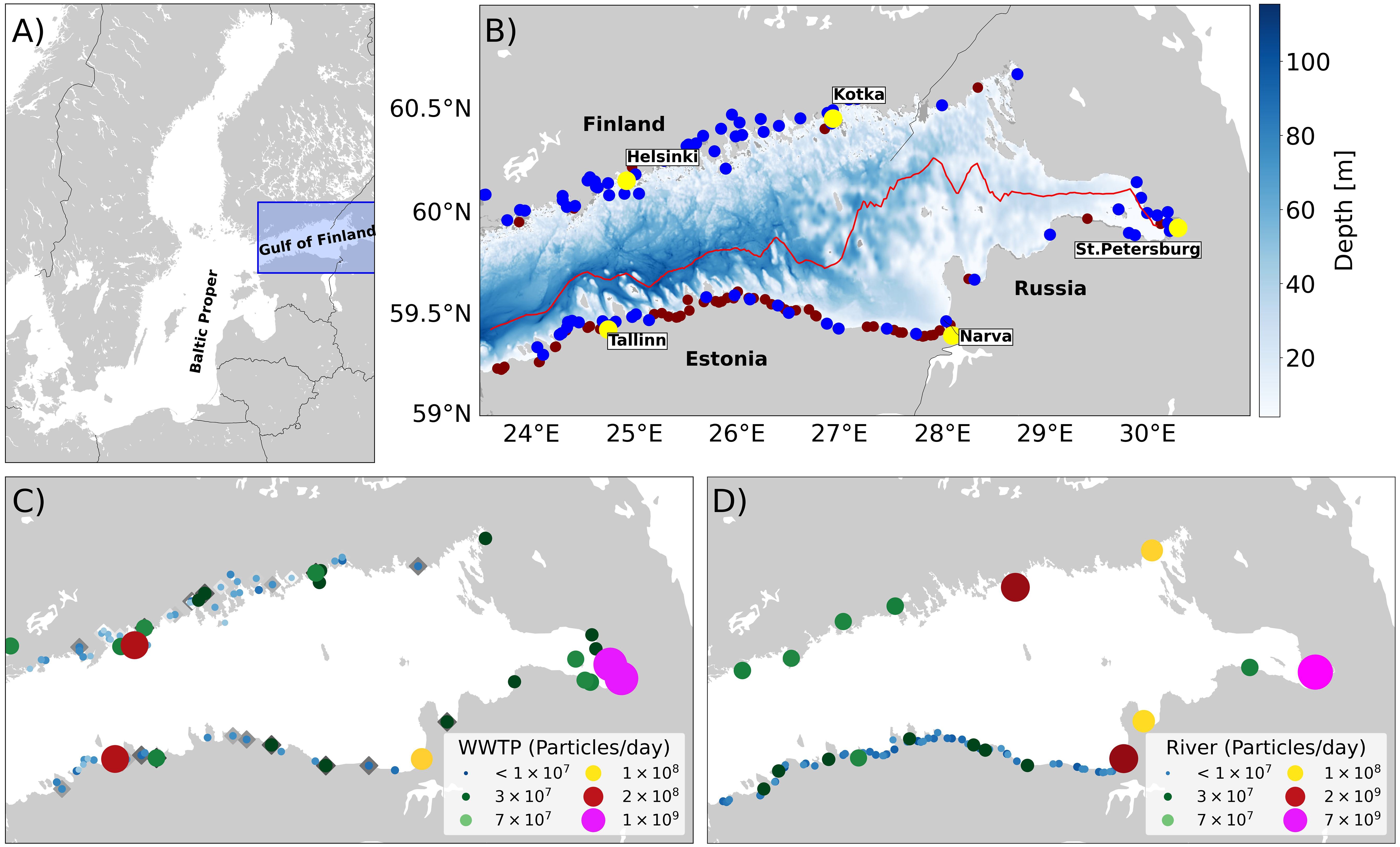
Figure 1. Panel (A, B) represent a map of the Baltic Sea and a map of the WWTPs (blue dots) and river (red dots) emissions points at the coast of the GoF. Yellow dots represent the cities mentioned in the study. The red line indicates the thalweg along the GoF; panels (C, D) display the emissions of PET and PP/PE MP particles (20-500 um) from WWTPs and riverine sources entering the GoF. The diamond markers in panel (1C) represent emissions from inland WWTPs.
2.3.2 Emission scenarios for PP/PE and PET
Calculated emissions from WWTPs and rivers serve as the inputs for the two main scenario runs.
In Scenario 1, the focus was on evaluating the loads of PET and PP/PE from WWTP sources, specifically considering the 20-500 μm MP size fraction. To gain a deeper understanding, this size fraction was further divided into two sub-ranges: small particles (20-200 μm) and large particles (200-500 μm) for both PET and PP/PE.
In Scenario 2, the analysis encompassed PET and PP/PE loads from riverine sources, taking into account the 20-500 μm MP size fractions. Like Scenario 1, this size fraction was divided into two sub-ranges: small particles (20-200 μm) and large particles (200-500 μm) for both PET and PP/PE.
Thus, eight scenario runs were simulated in total, representing two types of MP, two size fractions, and two emission pathways. It is important to note that all scenarios assume a constant daily MP emission throughout the entire simulation period (Table 1), facilitating a thorough evaluation of MP pollution. Thus, eight scenario runs were simulated in total, representing two types of MP, two size fractions, and two emission pathways.
3 Results
3.1 Overall variability of microplastic distribution
The time series of the share of particles in different states is shown in Figure 2. The overall spin-up of the model was relatively fast – after initialization, the share of particles in the water column dropped quickly to approximately 15%, while the share of sedimented particles stabilized between 75 – 80%. Meanwhile, the share of particles at the boundary reached approximately 1%, and the share of beached particles reached 10% during the spin-up period. The small light particles were the most abundant in the water column, and the large heavy particles were the least common (see Figures 2A, B). Most particles that left the GoF (reached the boundary) also belonged to the small light class (3%), whereas the large light particles beached the most (28%). Approximately 65% of the small light and large light particles and 92% and 95% of the small heavy and large heavy particles, respectively, settled after 3 years of simulation.
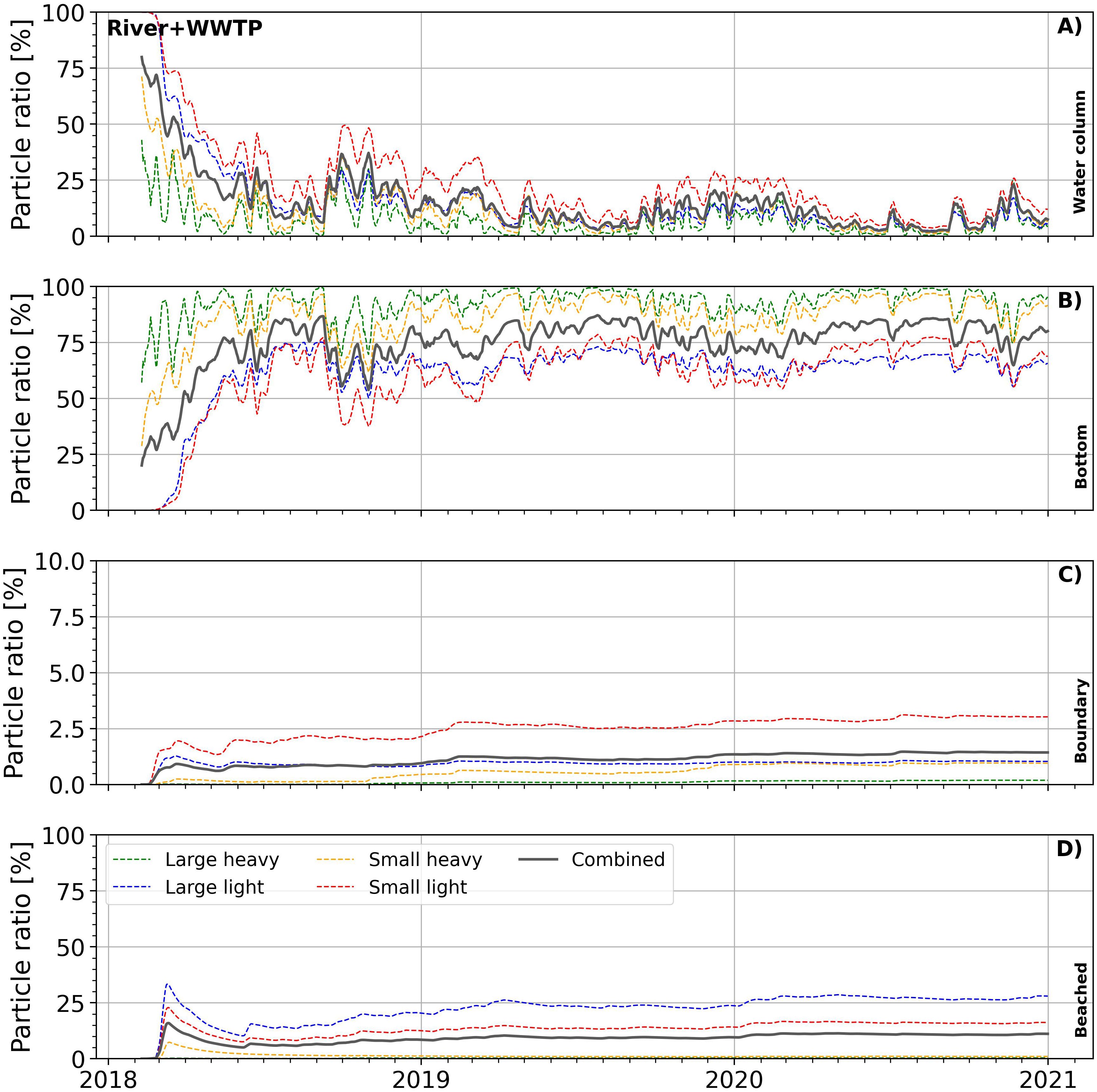
Figure 2. Time series of the particle budget in different classes for the Gulf of Finland. (A) water column, (B) bottom, (C) boundary, and (D) beached. All time series have been smoothed using a 7-day moving window.
3.1.1 Variability of surface concentrations
The average concentration of MP particles in the surface layer is shown in Figure 3. The concentrations were larger for the light particles (PP/PE) compared to heavy particles (PET) but high for both types near major coastal sources (Figures 3E, F). Since the riverine input to the Russian part was six-fold greater than the combined input to the Estonian and Finland parts, the highest concentrations of riverine-origin MP were in the eastern part of the gulf (Figures 3A, B). The WWTP-origin particle concentrations were high in the vicinity of larger cities (St. Petersburg, Helsinki and Tallinn, Figures 3C, D). The overall mean riverine origin particle concentrations for PP/PE within the model domain were about 3.8 particles/m2, but the maximum values exceeded 50 particles/m2 in the eastern part of the gulf (Figure 3B). The PET particles did not disperse as extensively as PP/PE particles from the eastern part of the gulf towards the west. PET particles from WWTPs were primarily gathered near Helsinki and in the eastern part of the gulf (Figure 3C). PP/PE particles from WWTPs had a similar distribution to the PET, but dispersion was higher, and the impact of Tallinn was more pronounced. On average, the PET and PP/PE particles released from WWTPs had concentrations of 0.1 and 1.0 particles/m2 within the model domain, respectively. When considering particles from both rivers and WWTPs, the average surface concentrations for PET and PP/PE particles were 1.4 particles/m2 and 4.8 particles/m2, respectively. The findings indicate that most of the MP particles in the central gulf are predominantly retained near their source areas, with limited long-distance transport.
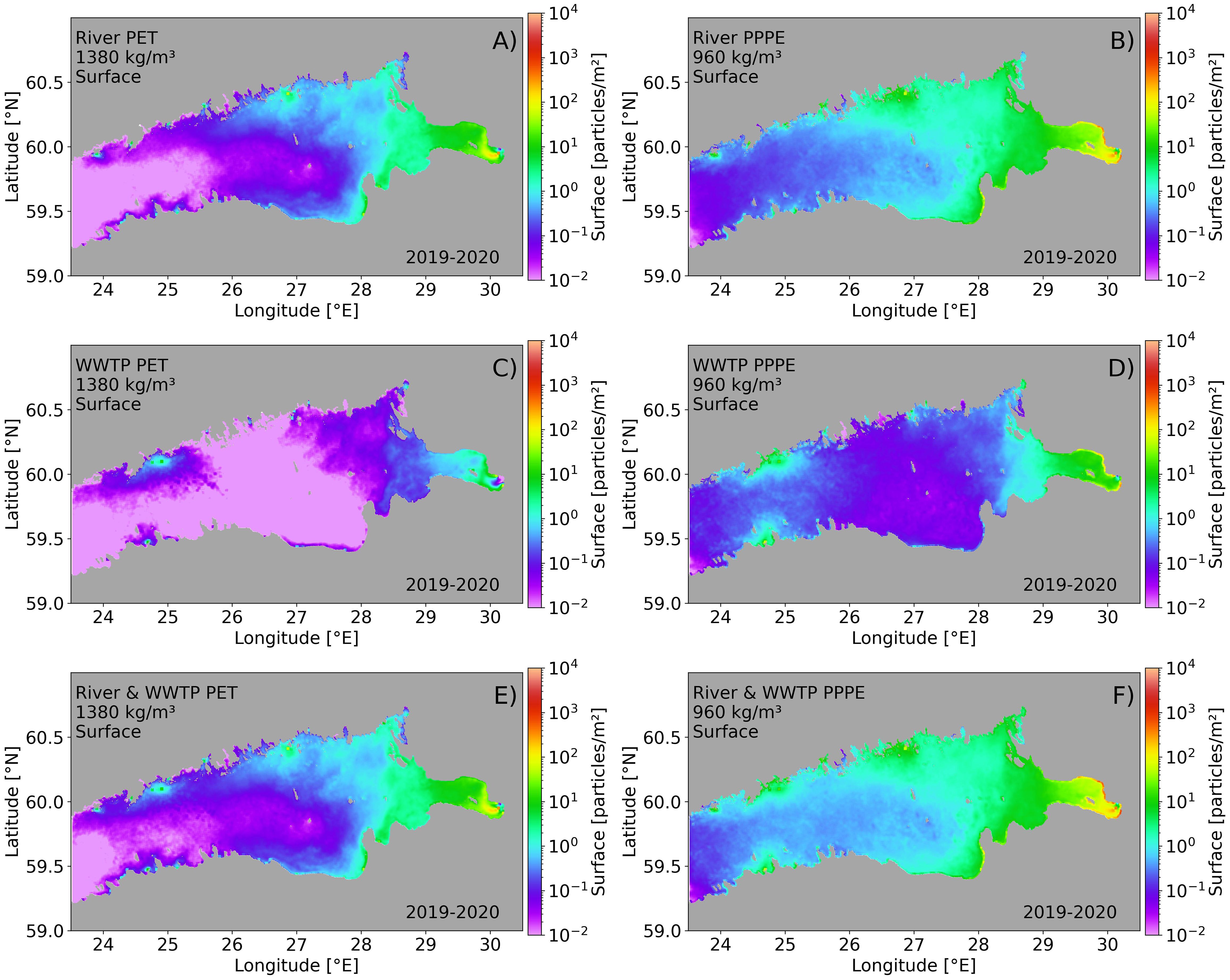
Figure 3. Mean concentrations of PET and PP/PE MP particles (20 - 500 μm) in the surface layer of the GoF in 2019-2020. Panels (A, B) represent the riverine origin PET and PP/PE particles; panels (C, D) WWTP origin PET and PP/PE particles; panels (E, F) display composite maps of different origin PET and PP/PE particles.
3.1.2 Water column
Figure 4 provides an overview of the mean spatial distributions of vertically integrated MP amounts in the water column during 2019 and 2020. The occurrence of particles in the water column was larger in the eastern part than in the western part of the gulf, where concentrations were much smaller for both the riverine and WWTP-origin particles. Heavy particles tended to remain closer to sources (rivers and WWTPs) in the eastern part, but high integrated concentrations can also be seen along the thalweg of the gulf in the western part.
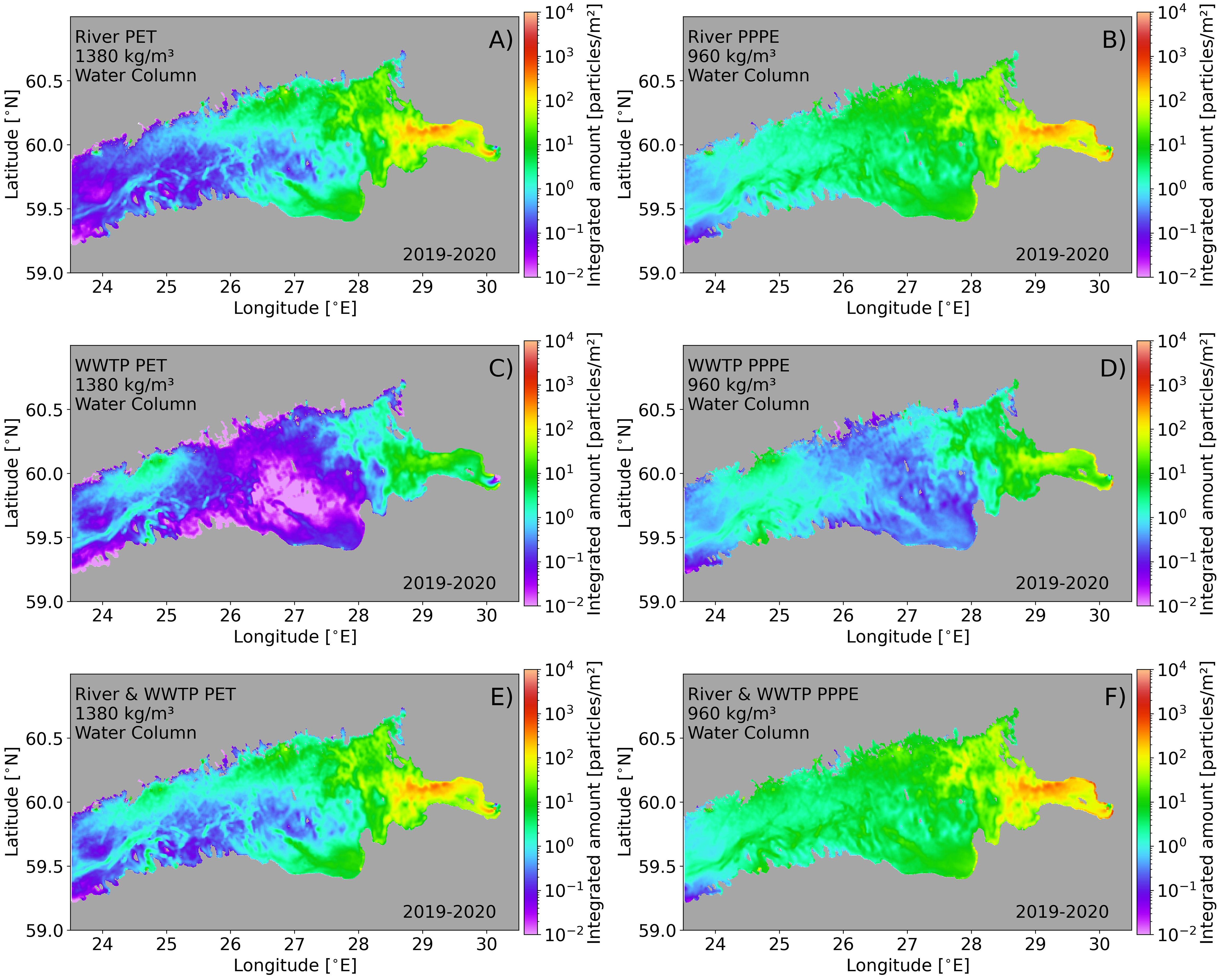
Figure 4. Mean spatial distribution of vertically integrated concentrations of PET and PP/PE (20-500 mm) in the GoF in 2019-2020. Panels (A, B) represent the riverine origin PET and PP/PE particles; panels (C, D) WWTP origin PET and PP/PE particles; panels (E, F) display composite maps of different origin PET and PP/PE particles.
The model data suggests that a gradual decrease in MP concentration was likely influenced by both the configuration of the gulf and the distance from the main sources (Figure 4). Particularly in shallower areas, to the east from 28°E, there was an elevated presence of riverine PET and PP/PE particles (Figures 4A, B). Conversely, in the region to the west from 28°E, where the Neva River has less influence, lower concentrations of riverine-origin PET and PP/PE particles were observed. The mean concentrations of WWTP-origin particles were higher in both the eastern and western regions, with lower concentrations between 26°E and 28°E (Figures 4C, D). This variation in WWTP-origin PET and PP/PE particles is likely due to the locations of the main input sources. The region between 26°E and 28°E receives less pollution from WWTPs, resulting in lower concentrations of WWTP-origin particles in this area.
Figure 5 represents the meridionally integrated concentrations from south to north in the water column during 2019 and 2020. From the surface to the seabed, PET particles had lower concentrations, while PP/PE particles were more prevalent, likely due to the higher density of PET particles, causing them to sink more quickly (Figures 5A–D). A characteristic vertical distribution of particles with high concentrations near the sea surface and the seabed and a minimum in the intermediate water layer is revealed for both particle types in the deeper areas of the gulf.
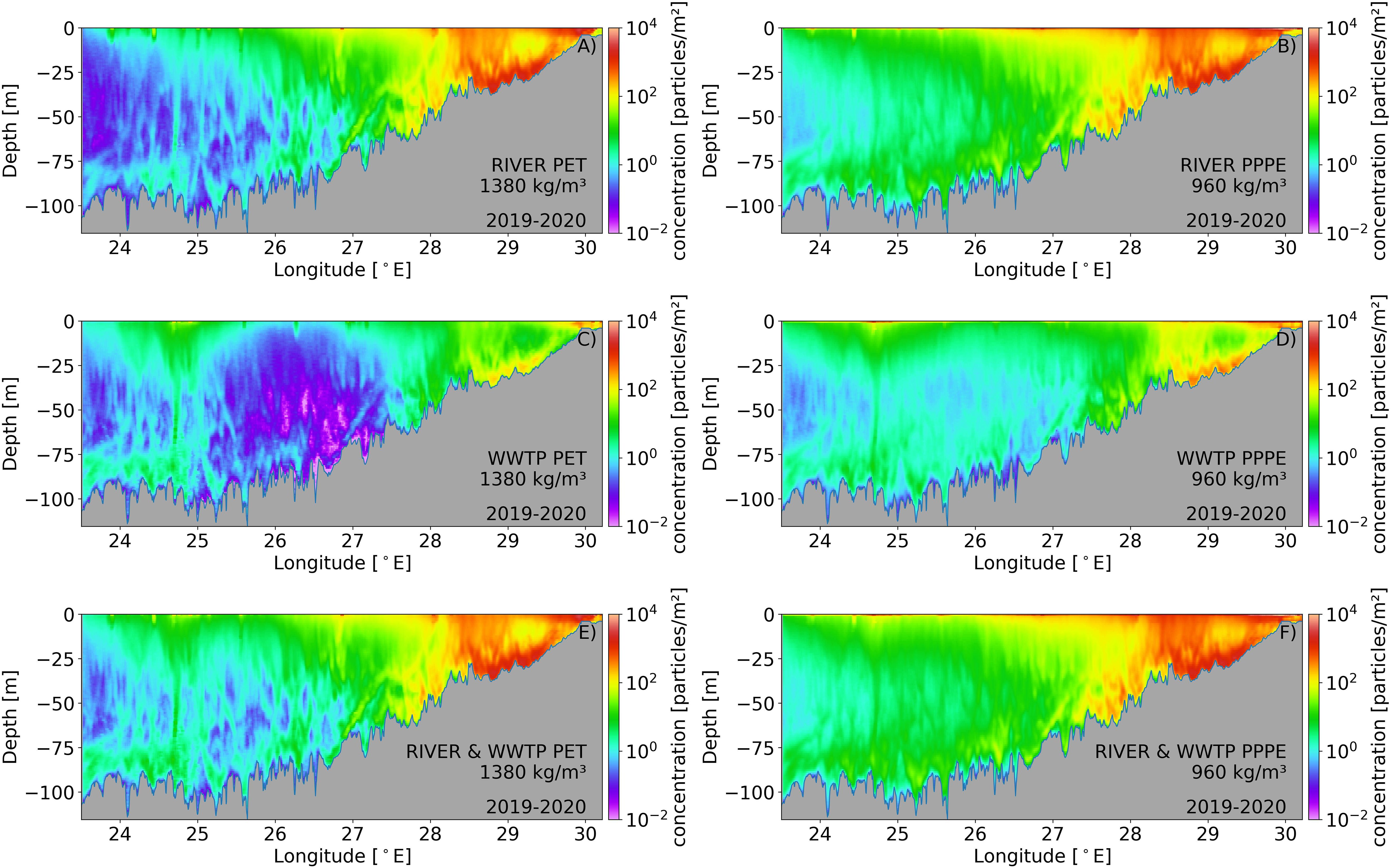
Figure 5. Mean meridionally integrated concentration of PET and PP/PE MP particles (20 - 500 μm) in the water column of the GoF in 2019-2020. Panels (A, B) represent the riverine origin PET and PP/PE particles; panels (C, D) WWTP origin PET and PP/PE particles; panels (E, F) display composite maps of different origin PET and PP/PE particles. The bathymetry data along the latitude axis is represented as the maximum depth values for each longitude coordinate.
3.1.3 Accumulation of particles
Sedimentation of particles of different origins and classes is shown in Figure 6. Sedimentation of the MP has occurred almost on the whole seabed of the gulf. The largest concentrations in the seabed are in the eastern part of the gulf, similar to those in the water column. The heavy particles (PET) tend to sink quickly and accumulate in the coastal areas, with notable amounts observed in Neva Bay, Narva Bay and near Kotka on the northern coast. Relatively high values were also in deeper areas of the central part of the gulf (Figure 6A). WWTP-origin PET particles had the highest values in the western and eastern parts of the gulf, while lower accumulation rates were revealed between 26.5 and 28°E (Figure 6C).
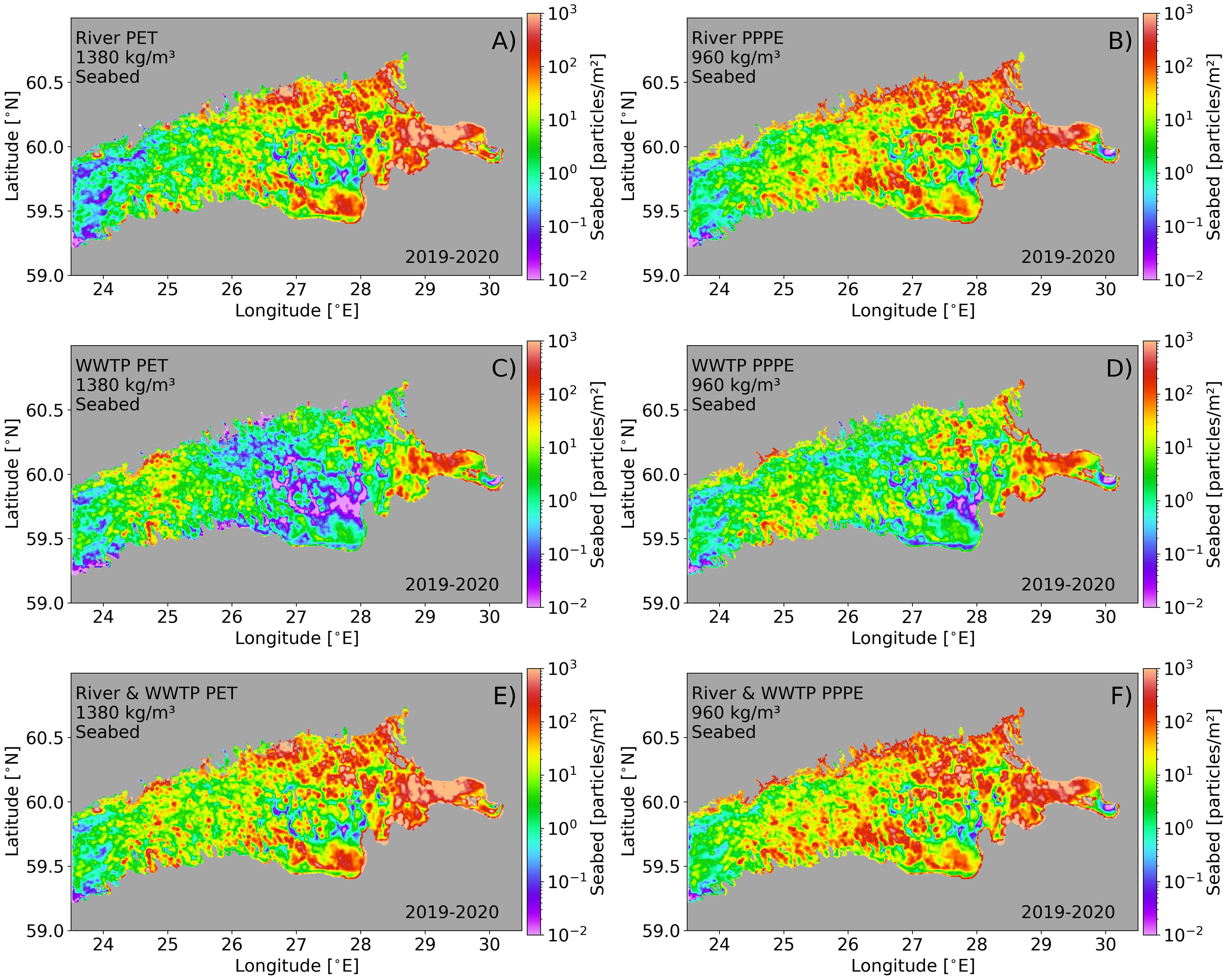
Figure 6. Mean spatial concentrations of PET and PP/PE MP particles (20 - 500 μm) on the seabed of the GoF in 2019-2020. Panels (A, B) represent the riverine origin PET and PP/PE particles; panels (C, D) WWTP origin PET and PP/PE particles; panels (E, F) display composite maps of different origin PET and PP/PE particles.
The composite maps indicate the overall accumulation (Figures 6E, F). In principle, the highest concentrations of both heavy and light particles were in the easternmost part of the gulf and Narva Bay. There is a tendency for higher concentrations near the coastline along the southern coast, while in the northern part, the sedimentation is more homogenous (Figure 6F).
3.1.4 Beaching of particles
The accumulation of particles on beaches around the GoF is shown in Figure 7. The whole coastline of the gulf has been impacted by the MP, although the load varied in space. Overall, more light particles beached compared to the heavy particles – the average number of beached particles was nearly 20 times higher for the light particles. Nevertheless, a relatively high number of heavy particles from rivers (> 105 particles/m) have beached on the southern shore of Neva Bay. A high number of light particles from rivers have beached in Neva Bay and Narva Bay, and along the Finnish coastline; particles from WWTPs are also numerous around Neva Bay, inside Tallinn Bay, in the vicinity of Helsinki, and at some spots along the northern coast.
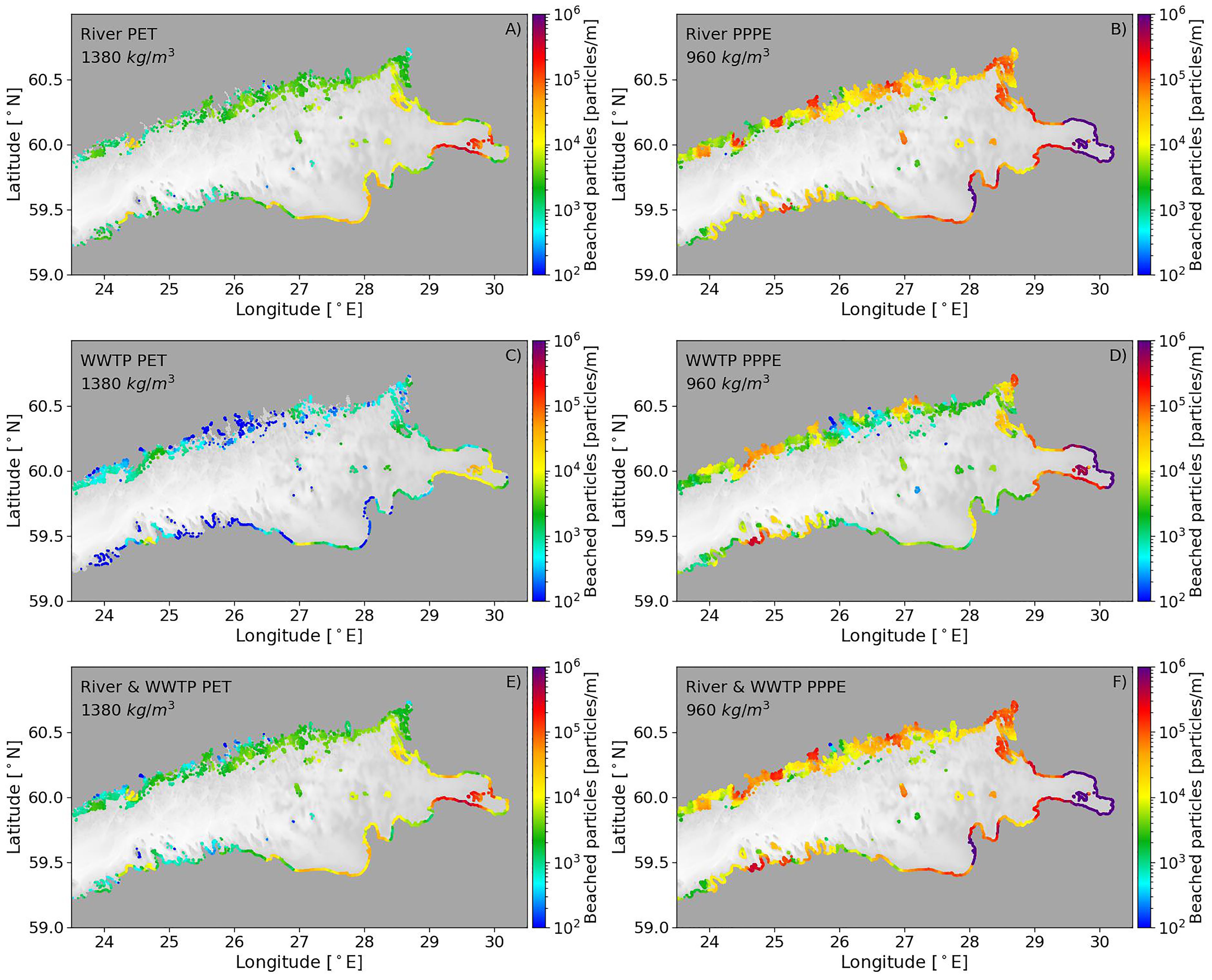
Figure 7. Distribution of particle accumulation on beaches over the period 2019–2020. Panels (A, B) represent the PET and PP/PE particles, respectively, from rivers; panels (C, D) focus on PET and PP/PE particles sourced from WWTPs; panels (E, F) display PET and PP/PE particles sourced from both rivers and WWTPs. The values have been spatially smoothed with a window length of 10 km.
3.2 Seasonal variability
3.2.1 Seasonal dynamics
Figures 8 and 9 illustrate the seasonal variations of PET and PP/PE particles in the surface layer, water column and sediments during the summer and winter months in the GoF.
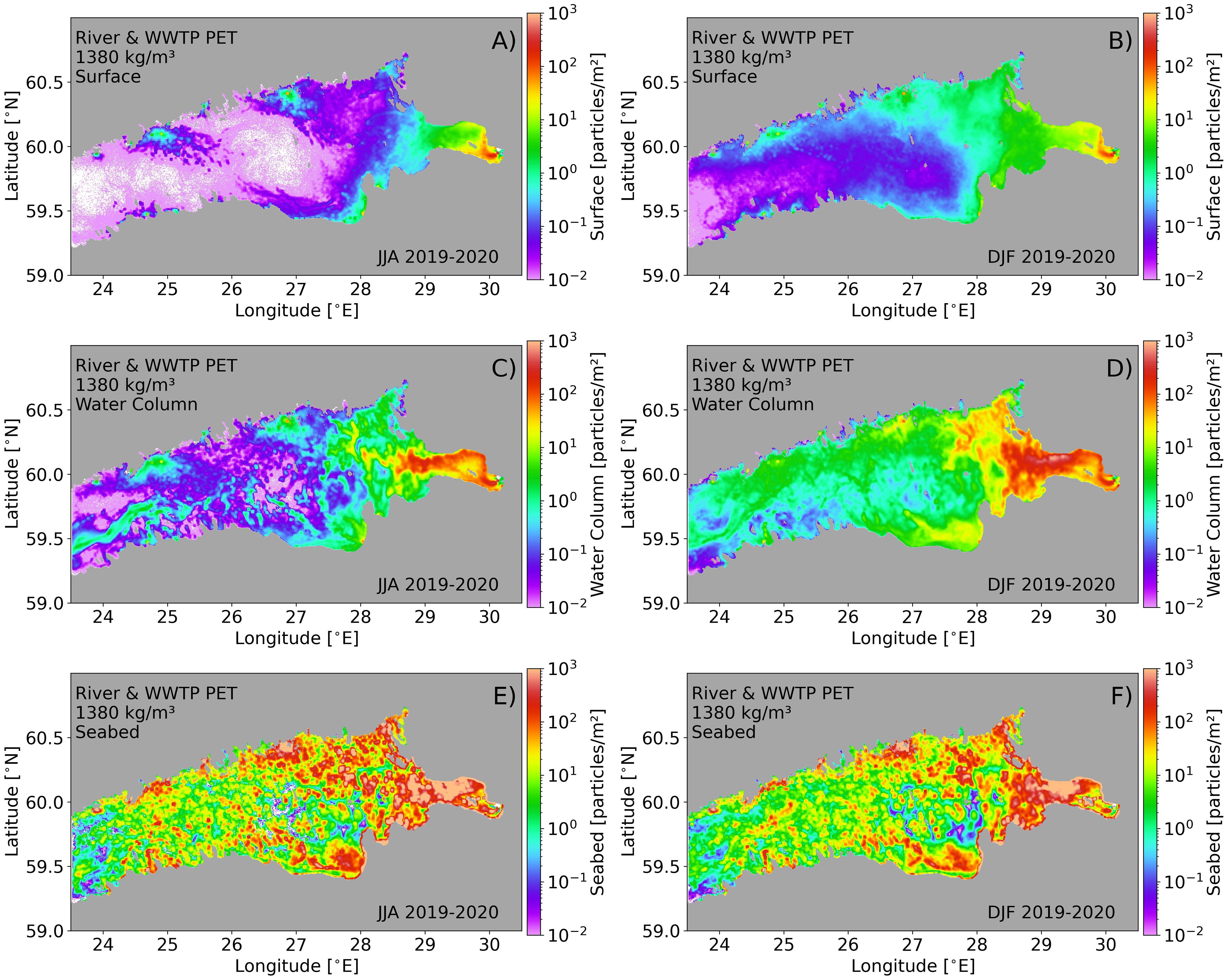
Figure 8. Mean spatial concentration of PET MP particles (20 - 500 μm) sourced from both rivers and WWTPs in different layers of the GoF during the summer months JJA (June, July, and August, panels (A, C, E) and winter months DJF (December, January, and February, panels (B, D, F). Panels (A, B) illustrate the concentration in the surface layer; panels (C, D) focus on concentration in the water column; panels (E, F) showcase the concentration in the bottom layer.
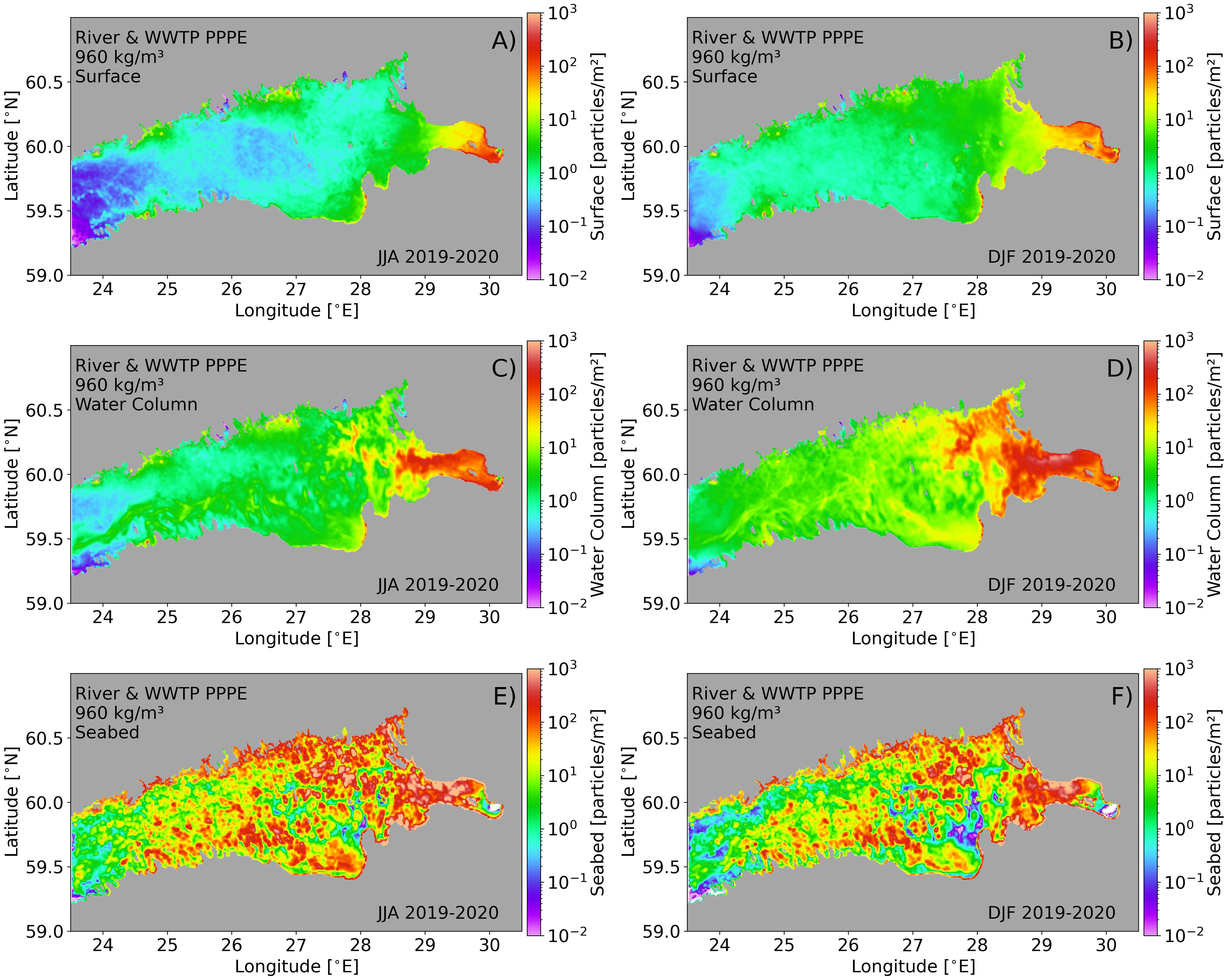
Figure 9. Mean spatial concentration of PP/PE MP particles (20 - 500 μm) sourced from both rivers and WWTPs in different layers of the GoF during the summer months JJA (June, July, and August) and winter months DJF (December, January, and February). Panels (A, B) illustrate the concentration in the surface layer; panels (C, D) focus on concentration in the water column; panels (E, F) showcase the concentration in the bottom layer.
During the summer months (JJA, June-August), PET MP concentrations near the surface were elevated along the northern and southern coasts, with the highest concentrations near St. Petersburg (Figure 8A). Despite lower river runoff and MP loads in winter (DJF, December-February), surface PET concentrations were higher in winter compared to summer (Figure 8B). A similar tendency was observed in the water column, where vertically integrated PET concentrations were higher in winter, likely due to reduced sedimentation and stronger resuspension driven by winter currents. In contrast, the settled particle concentrations were higher in summer, driven by enhanced biofilm formation and stratification (Figures 8E, F).
The mean surface concentration of PP/PE particles was higher in summer than in winter (Figures 9A, B), with higher levels observed in Narva Bay and along the Tallinn and Helsinki coasts. This winter increase likely reflects reduced sedimentation due to weaker biofilm growth. In contrast, summer concentrations were lower near the surface but higher on the seabed, primarily driven by enhanced biofilm formation and stratification, which promote particle settling (Figures 9C–F).
3.3 Short-term variability
The impact of an upwelling event along the northern coast is shown in Figure 10. There was already a small upwelling visible along the northern coast on 18 July (Figure 10A), the surface concentrations (Figure 10D) were strongly inhomogeneous, with the highest values in the easternmost areas of the gulf and patches also appearing along the northern coast and central part of the gulf. As the upwelling intensified on 22 July (Figure 10B), particles began to advert offshore, forming significant surface patches of MP concentrations in the central gulf (Figure 10E). During the upwelling peak (24th of July), a large patch of particles was advected southwards, particularly in the areas with colder temperatures, i.e. in the upwelling zone (Figure 10F).
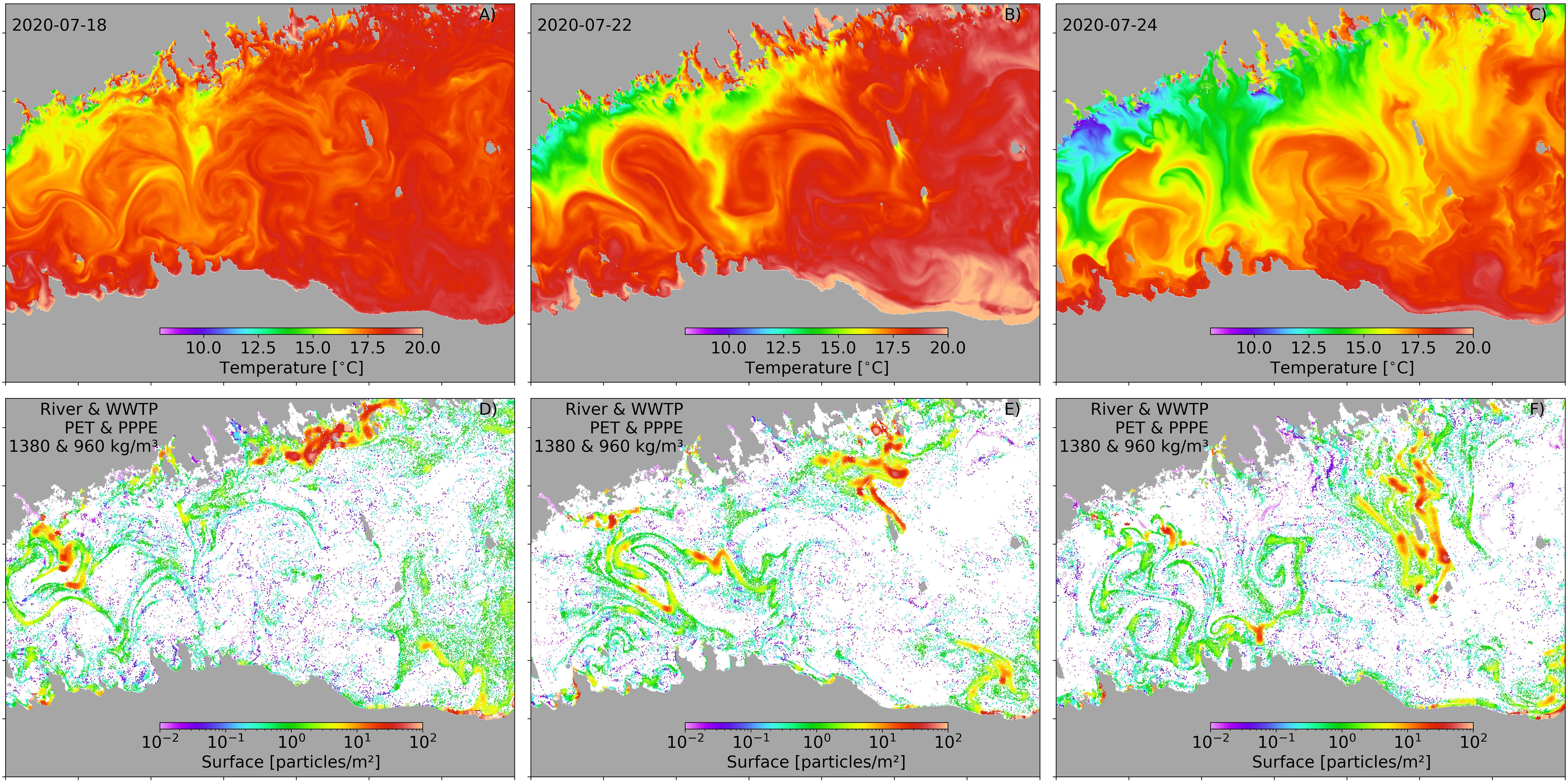
Figure 10. Sea Surface Temperature (SST) maps (A–C) and surface layers (D–F) during Upwelling conditions along the Finnish coast in the GoF.
The impact of near-bottom currents on particle concentrations in the 5 m thick layer above the seabed is shown in Figure 11. Stronger near-bottom currents observed on December 6 (Figure 11B) caused increased resuspension of particles from the seabed, as evidenced by higher concentrations in the near-bottom layer (Figure 11E). On December 7, as the bottom currents relaxed (Figure 11C), resuspension levels decreased moderately (Figure 11F). This suggests that while stronger currents lead to particle resuspension, the eventual relaxation of currents allows particles to settle back onto the seabed over time.
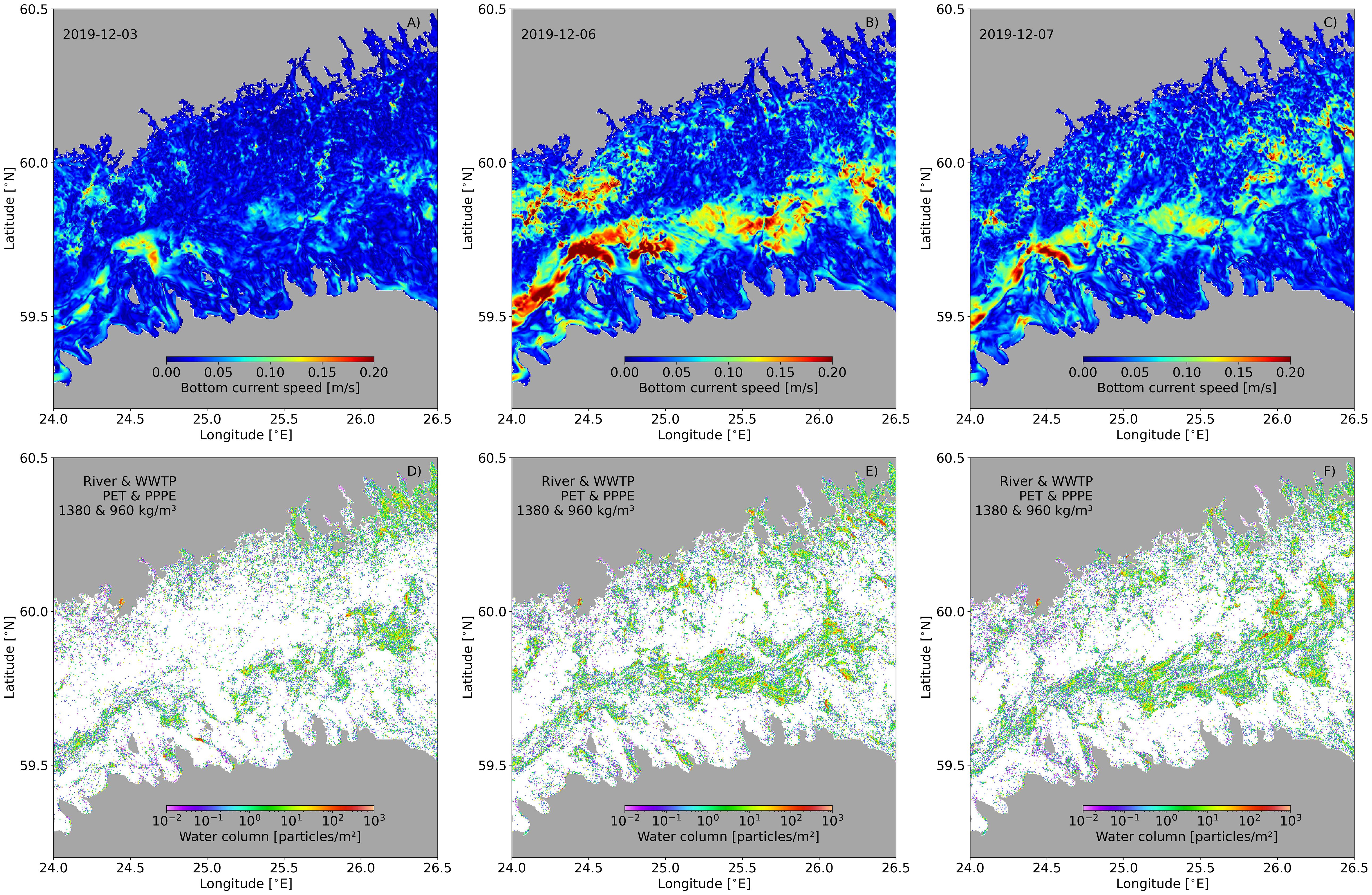
Figure 11. Snapshots of bottom currents (A–C), and water column particles integrated within 5 meters above the seabed (D–F) in the GoF.
4 Discussion
In this study, results from a multi-year (2018-2020) high-resolution model experiment were employed. This model system incorporated hydrodynamic, biogeochemical and Lagrangian particle tracking models to identify the MP pollution’s pathways and accumulation zones within the GoF in the surface layer, water column, coastline and bottom layer. Our research utilized the existing datasets for MP distribution estimates and employed advanced modeling techniques.
We considered two distinct particle types: (1) PET particles with density greater than sea water and (2) PP/PE particles with density less than sea water. In addition, particles within a size range of 20 to 500 um were considered and further categorized into small (20 - 200) and large (200 - 500) particles. Generally, this is well supported by literature. PP, PE, PET, PVC, and PS are the most common polymers found worldwide (Vermeiren et al., 2016; Geyer et al., 2017; Kooi and Koelmans, 2019). In China, Lv et al. (2019) found the following polymer shares: PP (15%), PE (18%), PET (47%) and PS (20%) in the raw wastewater. In the Mediterranean, out of the total MP observed, Pedrotti et al. (2016) reported that around 86-97% share accounted for the following polymers: PP, PE and polyamides. In our study, we used PP, PE and PET due to their abundance in the environment and as they cover a wide range of densities from 900 to 1300 kg/m3. We used the previous load estimates from Schernewski et al. (2020), which included WWTP locations and emissions exclusively used within the GoF catchment area. Based on the available literature (Schrank et al., 2022; Constant et al., 2020), we have considered mean MP particles found in the rivers. Nevertheless, substantial uncertainties still exist regarding emissions from the WWTPs and MP presence in rivers.
The Baltic Sea receives land-based MPs from rivers and coastal catchment areas (Murawski et al., 2022). The GoF is under considerable anthropogenic pressure, and as a result, the levels of pollutants, including the MP in water and biota are higher compared to the neighboring basins such as the Gulf of Riga or Baltic Proper (Mishra et al., 2022; Kuprijanov et al., 2024). In addition, our results indicate that most of the MP particles entering the GoF do not spread to the Baltic Proper, but instead accumulate within the GoF.
Due to prevailing cyclonic surface circulation in the GoF, floating litter tends, in general, to drift towards the Baltic Proper in higher abundances along the northern coast (Pärn et al., 2023). Our study reveals that the easternmost part of the GoF exhibits the highest levels of MP pollution in the surface layer. Similar tendencies for the GoF have been shown by other modelling studies (e.g. Murawski et al., 2022; Pärn et al., 2023) due to the large freshwater input from the Neva River. Pollution levels were notably reduced in the central gulf, with concentrations at least an order of magnitude smaller. Rivers discharge freshwater and substances in amounts not typically found in seawater (e.g. Hetland and Hsu, 2013) and, as a result, river plumes, small or large, with high concentrations of tracers and plume fronts in the vicinity of sources are formed. Five river plume fronts were noticeable in the study area associated with the Neva River, Luga River, Narva River and Kymi River (Suursaar et al., 2021). Nevertheless, none of these fronts are stationary as they undergo spatial excursions and other dynamic transformations (Suursaar et al., 2021), and consequently, we could not detect regions with persistently high MP concentrations at these frontal regions. However, such convergent density fronts are characterized by relatively large vertical velocities (D’Asaro et al., 2020) that likely restrict MP transport over long horizontal distances. Additionally, quasi-persistent eddy activity may contribute to such a high number of plastics near the emission areas (Andrejev et al., 2004; Pärn et al., 2023).
Our simulations revealed that both the PET and PP/PE particles predominantly accumulated in the seabed in close proximity to coastal regions and emission points. The accumulation areas of lighter PP/PE particles extended further offshore. Due to their negative buoyancy, PET particles consistently descend into the water column, ultimately settling on the seabed, particularly near coastal areas. Recent research, using an Eulerian modeling approach, also reported the accumulation of PET particles in shallow coastal waters of the Baltic Sea (Schernewski et al., 2020). In contrast, PP/PE particles, initially buoyant, remained suspended longer before sinking. This coastal accumulation was particularly evident near riverine sources such as the Neva River estuary and the Narva Bay. The shallow nature of the GoF, with an average depth of 37 m (Leppäranta and Myrberg, 2009), contributes to higher deposition rates in these areas. Kuprijanov et al. (2021) reported similar patterns of hazardous substance accumulation in shallow areas, such as Neva Bay and Finnish coastal inlets, though MP accumulation may persist longer in deeper areas.
Resuspension events, driven by near-bottom currents > 30 cm s-1, occasionally > 50 cm s-1 (Liblik et al., 2013; Rasmus et al., 2015; Suhhova et al., 2018), or wave-induced shear stress (Jönsson et al., 2005), can remobilize MPs from sediments back into the water column gulf. This dynamic interplay of deposition and resuspension highlights the importance of hydrodynamic forces in redistributing MPs in the current study.
The beaching of particles occurred almost throughout the entire GoF. The hotspots were mostly in bays with limited access to the open sea, e.g. Neva Bay, Tallinn Bay, and multiple locations on the northern coast. Previous studies have highlighted similar trends, with higher particle accumulation near the major rivers and urban areas, such as St. Petersburg and the Gulf of Riga (Schernewski et al., 2021). Our results show that light particles had substantially higher beaching rates compared to heavy particles, likely due to differences in their buoyancy and settling dynamics. Likely, a lot of the heavy PET particles settled in the shallow areas quicker than in 10 days required for a particle to be considered beached as defined in the simulations in the present study. Therefore, a significantly smaller amount of PET was found on beaches compared to light particles. These findings underline the importance of focused monitoring and sampling near key pollution sources, such as the Bay of Tallinn, to validate the simulation results and improve understanding of beaching dynamics.
The model effectively replicates the seasonal variability of MP concentrations observed in the GoF. Spring and summer are periods of heightened biological activity in the Baltic Sea (e.g. Lips et al., 2014). MP concentrations in the surface layer are lower in summer than winter were consistent with observations in the northern Baltic Proper and the GoF (Mishra et al., 2022). Furthermore, a recent modeling study conducted in Neva Bay, which used a different modeling approach compared to our study, reported a 10-fold decrease in the surface concentration during the summer months compared to the winter (Martyanov et al., 2021). This seasonal pattern reflects the combined influence of biofouling, hydrodynamics and stratification. In summer, phytoplankton blooms promote biofouling, enhancing the sinking rates of MPs and further leading to their accumulation on the seabed. Vertical stratification, while necessary for biofouling, also acts as a barrier, potentially trapping MPs withing the thermocline (Uurasjärvi et al., 2021). By late summer, the decay of thermocline and reduced primary production lead to less biofilm formation and reduced organic matter (Almroth-Rosell et al., 2011; Liblik and Lips, 2011) which may slow MP sinking.
In contrast, during winter, reduced biofouling, weaker stratification and stronger hydrodynamic activity contribute to higher MP concentrations at the surface. Stronger near-bottom currents can also resuspend particles from the seabed, maintaining elevated MP levels in the water column.
A wind-induced coupled coastal upwelling-downwelling event, which is frequent in the GoF (e.g. Lips et al., 2009; Uiboupin and Laanemets, 2009; Laanemets et al., 2011; Väli et al., 2011; Liblik and Lips, 2017) was selected as a case study. Previous studies have proposed that coastal upwelling may result in relatively low concentrations of MPs in coastal waters (de Lucia et al., 2014; Desforges et al., 2014; Mishra et al., 2022) while high concentration patches can form in the convergence zone of coastal downwelling (Mishra et al., 2022). In contrast, La Daana et al. (2017) found no significant difference in MP concentrations between Benguela upwelling sites and other non-upwelling sites.
During the upwelling events, the distribution in the surface layers indicated large particle concentrations converged in the sub-mesoscale stripes or small eddy-like features in the upwelling frontal zone, where the Rossby numbers (not shown) and temperature gradients were high (Figure 10). Previously, Väli et al. (2017, 2018) showed the convergence of particles in the GoF at the locations of the high Rossby number and sub-mesoscale activity. A recent modelling study (Väli et al., 2024) indicated the frequent occurrence of sub-mesoscale activity in the GoF. Observations of the temporal changes of MP concentrations are very challenging in such estuarine systems, where, on the one hand, the MP input from land is high, and, on the other hand, strong thermohaline gradients exist.
Previous studies have shown that episodic events, such as storms or wind-driven upwelling, can generate bottom currents, which can disturb bottom sediments, resuspending MPs into the overlying water column (Osinski et al., 2020; Zhou et al., 2021). In areas with high sedimentation rates, where MPs accumulate on the seabed, even short-term increases in current velocity have been shown to result in the release of particles back into the water column (Kane and Clare, 2019). Our results mirror this process, where we observed a marked decrease in sedimented MP concentrations and an increase in water column particles following periods of intensified bottom currents.
5 Conclusions and summary
We applied a combination of hydrodynamic, biogeochemical, and Lagrangian particle tracking models to trace MP pathways and identify potential accumulation zones in the GOF. This three-year simulation, with sub-mesoscale permitting horizontal resolution, revealed MP concentrations at the surface, within the water column, and accumulation on the seabed. The results provide critical insights into MP distribution pathways, spatial heterogeneity and the influence of hydrodynamics on MP transport and accumulation.
The study revealed that approximately 75% of MP particles settle on the seabed, making it the primary accumulation area in the GoF. Around 10% of particles were beached, with notable accumulation in Neva Bay, Narva Bay, and parts of the Finnish coastline. Only 1% of MP particles exited the gulf through the western boundary, while 14% remained suspended in the water column, influenced by episodic resuspension events. These findings highlight the GoF’s role as a significant retention zone for MPs due to its semi-enclosed geography and hydrodynamic conditions.
MP concentrations were highest near major coastal and riverine sources, particularly in the eastern part of the gulf. Surface concentrations of light particles exceeded those of heavy particles, especially in proximity to WWTPs and river mouths. The zonally integrated concentrations demonstrated higher values in the shallower eastern areas and a marked decrease west of 28°E. This spatial variability underscores the influence of anthropogenic inputs and local hydrodynamics on MP distribution.
Hydrodynamic processes played a key role in shaping MP transport and redistribution in the GoF. Upwelling and downwelling events, as well as episodic intensification of bottom currents, significantly influenced the redistribution of MPs. Strong bottom currents resuspended settled MPs into the water column, temporarily increasing their concentrations. These dynamic processes emphasize the importance of monitoring MPs across all layers of the marine environment – not just the surface layer but also the water column and seabed, to fully understand their transport and fate.
This study provides a scientific basis for policymakers to regulate MP emissions from WWTPs, manage riverine inputs, and address urban coastal pollution. By identifying hotspot areas, such as Neva Bay and Narva Bay, the findings can help prioritize resources for pollution control. Future monitoring efforts in the GoF and Baltic Sea should extend beyond the surface layer to include the water column and seabed, incorporating multipoint sampling at various depths, especially near emission sources. Additionally, mitigation measures should focus on the eastern gulf, where MP concentrations are consistently elevated.
Data availability statement
The raw data supporting the conclusions of this article will be made available by the authors, without undue reservation.
Author contributions
AM: Conceptualization, Data curation, Investigation, Methodology, Software, Validation, Visualization, Writing – original draft, Writing – review & editing. ES: Conceptualization, Data curation, Investigation, Methodology, Software, Validation, Visualization, Writing – original draft, Writing – review & editing. GV: Conceptualization, Data curation, Formal analysis, Funding acquisition, Investigation, Methodology, Software, Supervision, Validation, Visualization, Writing – review & editing. TL: Conceptualization, Data curation, Formal analysis, Funding acquisition, Project administration, Supervision, Validation, Visualization, Writing – review & editing. NB: Conceptualization, Resources, Validation, Writing – review & editing. UL: Conceptualization, Formal analysis, Funding acquisition, Methodology, Project administration, Validation, Visualization, Writing – review & editing.
Funding
The author(s) declare that financial support was received for the research, authorship, and/or publication of this article. This work was supported by the Estonian Research Council grant PRG602 and the JPI Oceans project RESPONSE (funded by the Ministry of the Environment of Estonia and the Estonian Research Council).
Acknowledgments
Allocation of computing time from HPC at Tallinn University of Technology is gratefully acknowledged. The GETM community at Leibniz Institute of Baltic Sea research (IOW, Warnemünde) is acknowledged for the code maintenance and support. Prof. Gerald Schernewski (IOW) is acknowledged for providing MP load data for the GoF.
Conflict of interest
The authors declare that the research was conducted in the absence of any commercial or financial relationships that could be construed as a potential conflict of interest.
Generative AI statement
The author(s) declare that no Generative AI was used in the creation of this manuscript.
Publisher’s note
All claims expressed in this article are solely those of the authors and do not necessarily represent those of their affiliated organizations, or those of the publisher, the editors and the reviewers. Any product that may be evaluated in this article, or claim that may be made by its manufacturer, is not guaranteed or endorsed by the publisher.
References
Aigars J., Barone M., Suhareva N., Putna-Nimane I., Dimante-Deimantovica I. (2021). Occurrence and spatial distribution of microplastics in the surface waters of the Baltic Sea and the Gulf of Riga. Mar. pollut. Bull. 172, 112860. doi: 10.1016/j.marpolbul.2021.112860
Alenius P., Myrberg K., Nekrasov A. (1998). The physical oceanography of the Gulf of Finland: a review. Boreal Environ. Res. 3, 97–125.
Alenius P., Nekrasov A., Myrberg K. (2003). Variability of the baroclinic Rossby radius in the Gulf of Finland. Cont. Shelf Res. 23, 563–573. doi: 10.1016/S0278-4343(03)00004-9
Almroth-Rosell E., Eilola K., Hordoir R., Meier H. E. M., Hall P. O. J. (2011). Transport of fresh and resuspended particulate organic material in the Baltic Sea—a model study. J. Mar. Syst. 87, 1–12. doi: 10.1016/j.jmarsys.2011.02.005
Andrejev O., Myrberg K., Alenius P., Lundberg P. A. (2004). Mean circulation and water exchange in the Gulf of Finland—a study based on three-dimensional modelling. Boreal Environ. Res. 9, 1.
Baresel C., Olshammar M. (2019). On the importance of sanitary sewer overflow on the total discharge of microplastics from sewage water. J. Environ. Prot. (Irvine,. Calif). 10, 1105–1118. doi: 10.4236/jep.2019.109065
Barnes D. K. A., Galgani F., Thompson R. C., Barlaz M. (2009). Accumulation and fragmentation of plastic debris in global environments. Philos. Trans. R. Soc B Biol. Sci. 364, 1985–1998. doi: 10.1098/rstb.2008.0205
Bigdeli M., Mohammadian A., Pilechi A., Taheri M. (2022). Lagrangian modeling of marine microplastics fate and transport: the state of the science. J. Mar. Sci. Eng. 10, 481. doi: 10.3390/jmse10040481
Bruggeman J., Bolding K. (2014). A general framework for aquatic biogeochemical models. Environ. Model. Software 61, 249–265. doi: 10.1016/j.envsoft.2014.04.002
Burchard H. (2001). Applied turbulence modelling in marine waters. Springer. doi: 10.5194/gmd-15-8613-2022
Burchard H., Bolding K. (2002). GETM: A general estuarine transport model, scientific documentation, european commission, joint research centre. Inst. Environ. Sustain. 12262, 25.
Canuto V. M., Howard A., Cheng Y., Dubovikov M. S. (2001). Ocean turbulence. Part I: One-point closure model—Momentum and heat vertical diffusivities. J. Phys. Oceanogr. 31, 1413–1426. doi: 10.1175/1520-0485(2001)031<1413:OTPIOP>2.0.CO;2
Carr S. A., Liu J., Tesoro A. G. (2016). Transport and fate of microplastic particles in wastewater treatment plants. Water Res. 91, 174–182. doi: 10.1016/j.watres.2016.01.002
Cole M., Lindeque P., Halsband C., Galloway T. S. (2011). Microplastics as contaminants in the marine environment: a review. Mar. pollut. Bull. 62, 2588–2597. doi: 10.1016/j.marpolbul.2011.09.025
Constant M., Ludwig W., Kerhervé P., Sola J., Charrière B., Sanchez-Vidal A., et al. (2020). Microplastic fluxes in a large and a small Mediterranean river catchments: The Têt and the Rhône, Northwestern Mediterranean Sea. Sci. Total Environ. 716, 136984. doi: 10.1016/j.scitotenv.2020.136984
Cuttat F. M. R. (2018). Marine transport of plastic fragments from dolly ropes used in demersal fisheries in the North Sea. (Denmark: DTU Aqua)
D’Asaro E. A., Carlson D. F., Chamecki M., Harcourt R. R., Haus B. K., Fox-Kemper B., et al. (2020). Advances in observing and understanding small-scale open ocean circulation during the Gulf of Mexico Research Initiative Era. Front. Mar. Sci. 7. doi: 10.3389/fmars.2020.00349
de Lucia G. A., Caliani I., Marra S., Camedda A., Coppa S., Alcaro L., et al. (2014). Amount and distribution of neustonic micro-plastic off the western Sardinian coast (Central-Western Mediterranean Sea). Mar. Environ. Res. 100, 10–16. doi: 10.1016/j.marenvres.2014.03.017
Desforges J.-P. W., Galbraith M., Dangerfield N., Ross P. S. (2014). Widespread distribution of microplastics in subsurface seawater in the NE Pacific Ocean. Mar. pollut. Bull. 79, 94–99. doi: 10.1016/j.marpolbul.2013.12.035
Dris R., Gasperi J., Tassin B. (2018). Sources and fate of microplastics in urban areas: a focus on Paris megacity. Freshw. microplastics Emerg. Environ. Contam. 69–83. doi: 10.1007/978-3-319-61615-5_4
Eriksen M., Cowger W., Erdle L. M., Coffin S., Villarrubia-Gómez P., Moore C. J., et al. (2023). A growing plastic smog, now estimated to be over 170 trillion plastic particles afloat in the world’s oceans—Urgent solutions required. PloS One 18, e0281596. doi: 10.1371/journal.pone.0281596
European Chemicals Agency. (2019). Annex XV Restriction Report: Proposal for a Restriction – Intentionally Added Microplastics.
Frishfelds V., Murawski J., She J. (2022). Transport of microplastics from the daugava estuary to the Open Sea. Front. Mar. Sci. 9. doi: 10.3389/fmars.2022.886775
GESAMP (2019). Guidelines on the monitoring and assessment of plastic litter and microplastics in the 632 ocean. GESAMP Rep. Stud. Ser. 99, 130.
Geyer R., Jambeck J. R., Law K. L. (2017). Production, use, and fate of all plastics ever made. Sci. Adv. 3, e1700782. doi: 10.1126/sciadv.1700782
Gies E. A., LeNoble J. L., Noël M., Etemadifar A., Bishay F., Hall E. R., et al. (2018). Retention of microplastics in a major secondary wastewater treatment plant in Vancouver, Canada. Mar. pollut. Bull. 133, 553–561. doi: 10.1016/j.marpolbul.2018.06.006
Gräwe U., Naumann M., Mohrholz V., Burchard H. (2015). Anatomizing one of the largest saltwater inflows into the B altic S ea in D ecember 2014. J. Geophys. Res. Ocean. 120, 7676–7697. doi: 10.1002/2015JC011269
Gröger M., Placke M., Meier H. E., Börgel F., Brunnabend S.-E., Dutheil C., et al. (2022). The Baltic Sea Model Intercomparison Project (BMIP)–a platform for model development, evaluation, and uncertainty assessment. Geosci. Model. Dev. 15, 8613–8638. doi: 10.5194/gmd-15-8613-2022
HELCOM (2023). “State of the Baltic Sea. Third HELCOM holistic assessment 2016-2021,” in Baltic sea environment proceedings. Helsinki, Finland: HELCOM. Available at: https://helcom.fi/post_type_publ/holas3_sobs.
Hersbach H., Bell B., Berrisford P., Hirahara S., Horányi A., Muñoz-Sabater J., et al. (2020). The ERA5 global reanalysis. Q. J. R. Meteorol. Soc 146, 1999–2049. doi: 10.1002/qj.3803
Hetland R. D., Hsu T. J. (2013). “Freshwater and sediment dispersal in large river plumes,” in Biogeochem. Dyn. Large river-coastal interfaces linkages with glob. Clim. Chang. Eds. Bianchi T. S., Allison M. A., Cai ,. W.-J. (Springer, New York, USA), 55–85.
Hofmeister R., Burchard H., Beckers J.-M. (2010). Non-uniform adaptive vertical grids for 3D numerical ocean models. Ocean Model. 33, 70–86. doi: 10.1016/j.ocemod.2009.12.003
Jambeck J. R., Geyer R., Wilcox C., Siegler T. R., Perryman M., Andrady A., et al. (2015). Plastic waste inputs from land into the ocean. Sci. (80-.). 347, 768–771. doi: 10.1126/science.1260352
Jönsson A., Danielsson Å., Rahm L. (2005). Bottom type distribution based on wave friction velocity in the Baltic Sea. Cont. Shelf Res. 25, 419–435. doi: 10.1016/j.csr.2004.09.011
Kane I. A., Clare M. A. (2019). Dispersion, accumulation, and the ultimate fate of microplastics in deep-marine environments: a review and future directions. Front. Earth Sci. 7. doi: 10.3389/feart.2019.00080
Kay P., Hiscoe R., Moberley I., Bajic L., McKenna N. (2018). Wastewater treatment plants as a source of microplastics in river catchments. Environ. Sci. pollut. Res. 25, 20264–20267. doi: 10.1007/s11356-018-2070-7
Klingbeil K., Lemarié F., Debreu L., Burchard H. (2018). The numerics of hydrostatic structured-grid coastal ocean models: State of the art and future perspectives. Ocean Model. 125, 80–105. doi: 10.1016/j.ocemod.2018.01.007
Kooi M., Koelmans A. A. (2019). Simplifying microplastic via continuous probability distributions for size, shape, and density. Environ. Sci. Technol. Lett. 6, 551–557. doi: 10.1021/acs.estlett.9b00379
Krauss W., Brügge B. (1991). Wind-produced water exchange between the deep basins of the Baltic Sea. J. Phys. Oceanogr. 21, 373–384. doi: 10.1175/1520-0485(1991)021<0373:WPWEBT>2.0.CO;2
Kuddithamby G., ALMEDA R., Vianello A., Lorenz C., Iordachescu L., Papacharalampos K., et al. (2024). Does water column stratification influence the vertical distribution of microplastics? Environ Pollut. 340, 122865. doi: 10.1016/j.envpol.2023.122865
Kuprijanov I., Buhhalko N., Eriksson U., Sjöberg V., Rotander A., Kolesova N., et al. (2024). A case study on microlitter and chemical contaminants: assessing biological effects in the southern coast of the Gulf of Finland (Baltic Sea) using the mussel Mytilus trossulus as a bioindicator. Mar. Environ. Res., 106628. doi: 10.1016/j.marenvres.2024.106628
Kuprijanov I., Väli G., Sharov A., Berezina N., Liblik T., Lips U., et al. (2021). Hazardous substances in the sediments and their pathways from potential sources in the eastern Gulf of Finland. Mar. pollut. Bull. 170, 112642. doi: 10.1016/j.marpolbul.2021.112642
Laanemets J., Väli G., Zhurbas V., Elken J., Lips I., Lips U. (2011). Simulation of mesoscale structures and nutrient transport during summer upwelling events in the Gulf of Finland in 2006. Boreal Environ. Res. 16, 15.
La Daana K. K., Officer R., Lyashevska O., Thompson R. C., O’Connor I. (2017). Microplastic abundance, distribution and composition along a latitudinal gradient in the Atlantic Ocean. Mar. pollut. Bull. 115, 307–314. doi: 10.1016/j.marpolbul.2016.12.025
Lebreton L. C. M., van der Zwet J., Damsteeg J.-W., Slat B., Andrady A., Reisser J. (2017). River plastic emissions to the world’s oceans. Nat. Commun. 8, 15611. doi: 10.1038/ncomms15611
Leppäranta M., Myrberg K. (2009). Physical oceanography of the baltic sea. (Berlin, Germany: Springer Science & Business Media). doi: 10.1007/978-3-540-79703-6
Liblik T., Laanemets J., Raudsepp U., Elken J., Suhhova I. (2013). Estuarine circulation reversals and related rapid changes in winter near-bottom oxygen conditions in the Gulf of Finland, Baltic Sea. Ocean Sci. 9, 917–930. doi: 10.5194/os-9-917-2013
Liblik T., Lips U. (2011). Characteristics and variability of the vertical thermohaline structure in the Gulf of Finland in summer. Boreal Environ. Res. 16. 73–83.
Liblik T., Lips U. (2017). Variability of pycnoclines in a three-layer, large estuary: the Gulf of Finland. Boreal Environ. Res. 22, 27.
Liblik T., Väli G., Lips I., Lilover M.-J., Kikas V., Laanemets J. (2020). The winter stratification phenomenon and its consequences in the Gulf of Finland, Baltic Sea. Ocean Sci. 16, 1475–1490. doi: 10.5194/os-16-1475-2020
Liblik T., Väli G., Salm K., Laanemets J., Lilover M.-J., Lips U. (2022). Quasi-steady circulation regimes in the Baltic Sea. Ocean Sci. Discuss. 2022, 1–37. doi: 10.5194/os-18-857-2022
Lips I., Lips U., Liblik T. (2009). Consequences of coastal upwelling events on physical and chemical patterns in the central Gulf of Finland (Baltic Sea). Cont. Shelf Res. 29, 1836–1847. doi: 10.1016/j.csr.2009.06.010
Lips I., Rünk N., Kikas V., Meerits A., Lips U. (2014). High-resolution dynamics of the spring bloom in the Gulf of Finland of the Baltic Sea. J. Mar. Syst. 129, 135–149. doi: 10.1016/j.jmarsys.2013.06.002
Lips I., Turov P., Lind K., Buhhalko N., Thennakoon H. (2020). Mikroplasti allikad ja levikuteed Eesti rannikumerre, potentsiaalne mõju pelaagilistele ja bentilistele organismidele. Report 4-1/18/30. Tallinn: Tallinn University of Technology, Department of Marine Systems; p. 1–46.
Lips U., Zhurbas V., Skudra M., Väli G. (2016). A numerical study of circulation in the Gulf of Riga, Baltic Sea. Part I: Whole-basin gyres and mean currents. Cont. Shelf Res. 112, 1–13. doi: 10.1016/j.csr.2015.11.008
Lips I., Turov P., Lind K., Buhhalko N., Thennakoon H. (2020). Mikroplasti allikad ja levikuteed Eesti rannikumerre, potentsiaalne mõju pelaagilistele ja bentilistele organismidele. Report 4-1/18/30. Tallinn: Tallinn University of Technology, Department of Marine Systems; p. 1–46.
Llorca M., Álvarez-Muñoz D., Ábalos M., Rodríguez-Mozaz S., Santos L. H., León V. M., et al. (2020). Microplastics in Mediterranean coastal area: Toxicity and impact for the environment and human health. Trends Environ. Anal. Chem. 27, e00090. doi: 10.1016/j.teac.2020.e00090
Lv X., Dong Q., Zuo Z., Liu Y., Huang X., Wu W.-M. (2019). Microplastics in a municipal wastewater treatment plant: Fate, dynamic distribution, removal efficiencies, and control strategies. J. Clean. Prod. 225, 579–586. doi: 10.1016/j.jclepro.2019.03.321
Magnusson K. (2016). Microlitter in sewage treatment systems: A Nordic perspective on waste water treatment plants as pathways for microscopic anthropogenic particles to marine systems. Nordic Council Ministers. doi: 10.6027/TN2016-510
Marko F., Urs B. (2013). Mass balance of perfluoroalkyl acids in the baltic sea. Environmental Science & Technology. 47, 4088–4095. doi: 10.1021/es400174y
Martyanov S. D., Isaev A. V., Ryabchenko V. A. (2021). Model estimates of microplastic potential contamination pattern of the eastern Gulf of Finland in 2018. Oceanologia 65, 86–99. doi: 10.1016/j.oceano.2021.11.006
Martyanov S. D., Ryabchenko V. A., Ershova A. A., Eremina T. R., Martin G.. (2023). On the assessment of microplastic distribution in the eastern part of the Gulf of Finland. Fundamental and Applied Hydrophysics, 16(1), 5–14. doi: 10.7868/S2073667323010020
Matjašič T., Mori N., Hostnik I., Bajt O., Viršek M. K. (2023). Microplastic pollution in small rivers along rural–urban gradients: Variations across catchments and between water column and sediments. Sci. Total Environ. 858, 160043. doi: 10.1016/j.scitotenv.2022.160043
Meier H. E. M., Kauker F. (2003). Modeling decadal variability of the Baltic Sea: 2. Role of freshwater inflow and large-scale atmospheric circulation for salinity. J. Geophys. Res. Ocean. 108. doi: 10.1029/2003JC001799
Mintenig S. M., Int-Veen I., Löder M. G. J., Primpke S., Gerdts G. (2017). Identification of microplastic in effluents of waste water treatment plants using focal plane array-based micro-Fourier-transform infrared imaging. Water Res. 108, 365–372. doi: 10.1016/j.watres.2016.11.015
Mishra A., Buhhalko N., Lind K., Lips I., Liblik T., Väli G., et al. (2022). Spatiotemporal variability of microplastics in the Eastern Baltic Sea. Front. Mar. Sci. 9. doi: 10.3389/fmars.2022.875984
Murawski J., She J., Frishfelds V. (2022). Modelling drift and fate of microplastics in the Baltic Sea. Front. Mar. Sci. 1656. doi: 10.3389/fmars.2022.886295
Neumann T., Fennel W., Kremp C. (2002). Experimental simulations with an ecosystem model of the Baltic Sea: a nutrient load reduction experiment. Global Biogeochem. Cycles 16, 1–7. doi: 10.1029/2001GB001450
Neumann T., Radtke H., Cahill B., Schmidt M., Rehder G. (2022). Non-Redfieldian carbon model for the Baltic Sea (ERGOM version 1.2)–implementation and budget estimates. Geosci. Model. Dev. 15, 8473–8540. doi: 10.5194/gmd-15-8473-2022
Neumann T., Schernewski G. (2008). Eutrophication in the Baltic Sea and shifts in nitrogen fixation analyzed with a 3D ecosystem model. J. Mar. Syst. 74, 592–602. doi: 10.1016/j.jmarsys.2008.05.003
Osinski R. D., Enders K., Gräwe U., Klingbeil K., Radtke H. (2020). Model uncertainties of a storm and their influence on microplastics and sediment transport in the Baltic Sea. Ocean Sci. 16, 1491–1507. doi: 10.5194/os-16-1491-2020
Pärn O., Moy D. M., Stips A. (2023). Determining the distribution and accumulation patterns of floating litter in the Baltic Sea using modelling tools. Mar. pollut. Bull. 190, 114864. doi: 10.1016/j.marpolbul.2023.114864
Pedrotti M. L., Petit S., Elineau A., Bruzaud S., Crebassa J.-C., Dumontet B., et al. (2016). Changes in the floating plastic pollution of the Mediterranean Sea in relation to the distance to land. PloS One 11, e0161581. doi: 10.1371/journal.pone.0161581
Prata J. C. (2018). Microplastics in wastewater: State of the knowledge on sources, fate and solutions. Mar. pollut. Bull. 129, 262–265. doi: 10.1016/j.marpolbul.2018.02.046
Radtke H., Lipka M., Bunke D., Morys C., Woelfel J., Cahill B., et al. (2019). Ecological ReGional Ocean Model with vertically resolved sediments (ERGOM SED 1.0): coupling benthic and pelagic biogeochemistry of the south-western Baltic Sea. Geosci. Model. Dev. 12, 275–320. doi: 10.5194/gmd-12-275-2019
Rasmus K., Kiirikki M., Lindfors A. (2015). Long-term field measurements of turbidity and current speed in the Gulf of Finland leading to an estimate of natural resuspension of bottom sediment. Boreal Environ. Res. 20, 735.
Redfield A. C. (1934). On the proportions of organic derivatives in sea water and their relation to the composition of plankton. (Liverpool, United Kingdom: University Press of Liverpool).
Scheffer M., Baveco J. M., DeAngelis D. L., Rose K. A., van Nes Eh. (1995). Super-individuals a simple solution for modelling large populations on an individual basis. Ecol. Modell. 80, 161–170. doi: 10.1016/0304-3800(94)00055-M
Schernewski G., Radtke H., Hauk R., Baresel C., Olshammar M., Oberbeckmann S. (2021). Urban microplastics emissions: effectiveness of retention measures and consequences for the Baltic Sea. Front. Mar. Sci. 8. doi: 10.3389/fmars.2021.594415
Schernewski G., Radtke H., Hauk R., Baresel C., Olshammar M., Osinski R., et al. (2020). Transport and behavior of microplastics emissions from urban sources in the Baltic Sea. Front. Environ. Sci. 8. doi: 10.3389/fenvs.2020.579361
Schmidt C., Krauth T., Wagner S. (2017). Export of plastic debris by rivers into the sea. Environ. Sci. Technol. 51, 12246–12253. doi: 10.1021/acs.est.7b02368
Schrank I., Löder M. G. J., Imhof H. K., Moses S. R., Heß M., Schwaiger J., et al. (2022). Riverine microplastic contamination in southwest Germany: A large-scale survey. Front. Earth Sci. 10. doi: 10.3389/feart.2022.794250
Setälä O., Magnusson K., Lehtiniemi M., Norén F. (2016). Distribution and abundance of surface water microlitter in the Baltic Sea: a comparison of two sampling methods. Mar. Pollut. Bull. 110, 177–183. doi: 10.1016/j.marpolbul.2016.06.065
She J., Buhhalko N., Lind K., Mishra A., Kikas V., Costa E., et al. (2022). Uncertainty and consistency assessment in multiple microplastic observation datasets in the Baltic Sea. Front. Mar. Sci. 9. doi: 10.3389/fmars.2022.886357
Siegfried M., Koelmans A. A., Besseling E., Kroeze C. (2017). Export of microplastics from land to sea. A modelling approach. Water Res. 127, 249–257. doi: 10.1016/j.watres.2017.10.011
Siht E., Väli G., Liblik T., Mishra A., Buhhalko N., Lips U. (2025). Modeling the pathways of microplastics 797 in the Gulf of Finland, Baltic Sea - sensitivity of parametrizations. Ocean Dynamics. Ocean Dynamics 75, 9. doi: 10.1007/s10236-024-01649-0
Smagorinsky J. (1963). General circulation experiments with the primitive equations: I. The basic experiment. Mon. Weather Rev. 91, 99–164. doi: 10.1175/1520-0493(1963)091<0099:GCEWTP>2.3.CO;2
Suaria G., Aliani S. (2014). Floating debris in the mediterranean sea. Mar. pollut. Bull. 86, 494–504. doi: 10.1016/j.marpolbul.2014.06.025
Suhhova I., Liblik T., Lilover M.-J., Lips U. (2018). A descriptive analysis of the linkage between the vertical stratification and current oscillations in the Gulf of Finland. Boreal Environ. Res. 23, 83–103.
Sun J., Dai X., Wang Q., Van Loosdrecht M. C. M., Ni B.-J. (2019). Microplastics in wastewater treatment plants: Detection, occurrence and removal. Water Res. 152, 21–37. doi: 10.1016/j.watres.2018.12.050
Suursaar Ü., Elken J., Belkin I. M. (2021). Fronts in the Baltic Sea: A review with a focus on its north-eastern part. Chem. Oceanogr. Front. Zo., 143–181. doi: 10.1007/698_2021_813
Talvitie J., Mikola A., Koistinen A., Setälä O. (2017). Solutions to microplastic pollution–Removal of microplastics from wastewater effluent with advanced wastewater treatment technologies. Water Res. 123, 401–407. doi: 10.1016/j.watres.2017.07.005
Tsiaras K., Hatzonikolakis Y., Kalaroni S., Pollani A., Triantafyllou G. (2021). Modeling the pathways and accumulation patterns of micro-and macro-plastics in the Mediterranean. Front. Mar. Sci. 8. doi: 10.3389/fmars.2021.743117
Uaciquete D., Mitsunaga K., Aoyama K., Kitajima K., Chiba T., Jamal D. L., et al. (2024). Microplastic abundance in the semi-enclosed Osaka Bay, Japan. Environ. Sci. pollut. Res. 31, 49455–49467. doi: 10.1007/s11356-024-34444-x
Uiboupin R., Laanemets J. (2009). Upwelling characteristics derived from satellite sea surface temperature data in the Gulf of Finland, Baltic Sea. Boreal Environ. Res. 14, 297–304.
Uurasjärvi E., Pääkkönen M., Setälä O., Koistinen A., Lehtiniemi M. (2021). Microplastics accumulate to thin layers in the stratified Baltic Sea. Environ. pollut. 268, 115700. doi: 10.1016/j.envpol.2020.115700
Väli G., Meier H. E. M., Liblik T., Radtke H., Klingbeil K., Gräwe U., et al. (2024). Submesoscale processes in the surface layer of the central Baltic Sea: a high-resolution modelling study. Oceanologia. 66, 78–90. doi: 10.1016/j.oceano.2023.11.002
Väli G., Meier M., Dieterich C., Placke M. (2019). River runoff forcing for ocean modeling withinthe Baltic Sea Model Intercomparison Project. Meereswiss. 113, 1–26. doi: 10.12754/msr-2019-0113
Väli G., Zhurbas V., Laanemets J., Elken J. (2011). Simulation of nutrient transport from different depths during an upwelling event in the Gulf of Finland. Oceanologia 53, 431–448. doi: 10.5697/oc.53-1-TI.431
Vali G., Zhurbas V. M., Laanemets J., Lips U. (2018). Clustering of floating particles due to submesoscale dynamics: a simulation study for the Gulf of Finland, Baltic Sea. Фундаментальная и прикладная гидрофизика 11, 21–35. doi: 10.7868/s2073667318020028
Väli G., Zhurbas V., Lips U., Laanemets J. (2017). Submesoscale structures related to upwelling events in the Gulf of Finland, Baltic Sea (numerical experiments). J. Mar. Syst. 171, 31–42. doi: 10.1016/j.jmarsys.2016.06.010
Veerasingam S., Saha M., Suneel V., Vethamony P., Rodrigues A. C., Bhattacharyya S., et al. (2016). Characteristics, seasonal distribution and surface degradation features of microplastic pellets along the Goa coast, India. Chemosphere 159, 496–505. doi: 10.1016/j.chemosphere.2016.06.056
Vermeiren P., Muñoz C. C., Ikejima K. (2016). Sources and sinks of plastic debris in estuaries: a conceptual model integrating biological, physical and chemical distribution mechanisms. Mar. pollut. Bull. 113, 7–16. doi: 10.1016/j.marpolbul.2016.10.002
Vianello A., Da Ros L., Boldrin A., Marceta T., Moschino V. (2018). First evaluation of floating microplastics in the Northwestern Adriatic Sea. Environ. Sci. pollut. Res. 25, 28546–28561. doi: 10.1007/s11356-018-2812-6
Zhou Q., Tu C., Yang J., Fu C., Li Y., Waniek J. J. (2021). Trapping of microplastics in halocline and turbidity layers of the semi-enclosed Baltic Sea. Front. Mar. Sci. 8. doi: 10.3389/fmars.2021.761566
Zhurbas V., Väli G., Golenko M., Paka V. (2018). Variability of bottom friction velocity along the inflow water pathway in the Baltic Sea. J. Mar. Syst. 184, 50–58. doi: 10.1016/j.jmarsys.2018.04.008
Keywords: microplastic, microplastic pathways, hydrodynamic modeling, Lagrangian particles, GETM, ERGOM, Gulf of Finland, Baltic Sea
Citation: Mishra A, Siht E, Väli G, Liblik T, Buhhalko N and Lips U (2025) Mapping microplastic pathways and accumulation zones in the Gulf of Finland, Baltic Sea – insights from modeling. Front. Mar. Sci. 11:1524585. doi: 10.3389/fmars.2024.1524585
Received: 07 November 2024; Accepted: 23 December 2024;
Published: 20 January 2025.
Edited by:
Meilin Wu, Chinese Academy of Sciences (CAS), ChinaReviewed by:
Marcos D. Mateus, Instituto Superior Técnico - Universidade de Lisboa, PortugalMeng Chuan Ong, University of Malaysia Terengganu, Malaysia
Copyright © 2025 Mishra, Siht, Väli, Liblik, Buhhalko and Lips. This is an open-access article distributed under the terms of the Creative Commons Attribution License (CC BY). The use, distribution or reproduction in other forums is permitted, provided the original author(s) and the copyright owner(s) are credited and that the original publication in this journal is cited, in accordance with accepted academic practice. No use, distribution or reproduction is permitted which does not comply with these terms.
*Correspondence: Arun Mishra, YXJ1bi5taXNocmFAdGFsdGVjaC5lZQ==