- 1Integrative Science Center of Germplasm Creation in Western China (CHONGQING) Science City, Key Laboratory of Freshwater Fish Reproduction and Development (Ministry of Education), College of Fisheries, Southwest University, Chongqing, China
- 2Shandong New Hope Liuhe Group Co., Ltd., Qingdao, China
- 3China Chongqing Fishery Technology Promotion Station, Chongqing Agriculture and Rural Affairs Committee, Chongqing, China
This research was to assess how varying dietary lipid levels influence the growth, body composition, antioxidant capacity of the liver, and the structure of liver and intestine in A. davidianus. The juvenile A. davidianus (initial weight of 34.20 ± 0.27g) were given isonitrogenous diets (crude protein 57%) that contained six different levels of lipid (5.02%, 8.96%, 12.90%, 16.21%, 19.46%, and 22.80%, respectively) for 105 days. The results revealed that WGR, SGR, PRR and muscle RNA/DNA ratio were significantly enhanced by dietary lipid level up to 16.21%, beyond which these parameters declined; FCR exhibited an inverse trend(P<0.05). The maximum activity of intestinal digestive enzymes and superoxide dismutase (SOD) were observed at the 16.21% dietary lipid level, accompanied by a significant reduction in liver malondialdehyde (MDA) concentration (P<0.05). With the increase in dietary lipid level, the lipid content of both the whole body and liver, along with skin collagen content, initially improved before subsequently declining(P<0.05). At the 16.21% dietary lipid level, the height, number, and width of intestinal mucosal folds significantly increase (P<0.05), and intestinal microvilli were more orderly arranged. At the 22.80% dietary lipid level, the liver cells exhibited degradation of the cell membrane, alongside an increased presence of autophagosomes; additionally, the mitochondria demonstrated significant swelling and rounding. Optimal lipid level could enhance feed utilization efficiency and promote both intestinal and liver health in A. davidianus. In contrast, high dietary lipid level has a detrimental effect on the health of A. davidianus. According to WGR, skin collagen content, muscle RNA/DNA ratio, total cholesterol (TCHO) and lipase activity, it has been determined through quadratic curve model analysis that the optimal dietary lipid level for juvenile A. davidianus ranges from 13.3% to 17.5%.
1 Introduction
The giant salamander (Andrias davidianus), commonly known as the baby fish, is the largest amphibian, with a history of more than 350 million years, and is a key species for the development of agricultural industrialization in China (Wang et al., 2004). It has been listed in the World Conservation Union Red List and Appendix I of the Washington Convention (CITES) (Zhang et al., 2002). Additionally, this species holds significant nutritional and medicinal benefits, as its skin, secretions, muscles, blood, and bones are rich in proteins, amino acids, and trace elements, often referred to as ginseng in water (He et al., 2018; Wang et al., 2021). At present, the industrialization of artificial breeding and cultivation of A. davidianus has taken place in various provinces across China; nevertheless, challenges remain regarding feed supply, and there has been limited research conducted on this topic (Cunningham et al., 2015). As the scale of farming continues to grow, the rising cost of breeding and decreasing market price of A. davidianus have made it imperative to advance the development of compound feed and conduct nutrition research.
Lipid has a variety of physiological functions in the metabolic process of fish, such as serving as a component of tissues and cells, supplying energy to the body, promoting the absorption and transportation of lipid-soluble vitamins, and providing essential fatty acids, etc (De Carvalho and Caramujo, 2018). Carnivorous aquatic animals have a poor ability to utilize carbohydrates, therefore protein and lipid are the main source of sustaining their life activities (Guo et al., 2023; Xie et al., 2021). Maintaining suitable dietary lipid level in the feed is beneficial for conserving protein, enhancing growth performance, and improving feed utilization efficiency (Chatzifotis et al., 2010; Mata-Sotres et al., 2022). However, long-term intake excessive lipid could result in liver disorders, cause oxidative stress (Shearer et al., 2012), induce oxidative damage (Yin et al., 2020), suppress immune responses, promote structural abnormalities in both liver and intestine (Zhu et al., 2017), and trigger inflammation (Naiel et al., 2023). Deficiency in dietary intake may result in metabolic disorders, decreased feed utilization efficiency, and inadequate nutrient provision for fish (Meng et al., 2019; Picolo et al., 2021; Qiang et al., 2017; Yan et al., 2015). Overall, the capacity to effectively use lipid differs significantly among aquatic species, as both excessive and inadequate consumption of lipid can affect ideal growth of fish.
Our research team has developed compound feed for A. davidianus, which is under development. Research on nutritional requirements and feed for A. davidianus by our research team has included evaluating protein requirement (Wang et al., 2020), functional feed additives (Lu et al., 2021), and herbal extract (Xu et al., 2021). However, the lipid requirement of A. davidianus and the impact of lipid on their growth and metabolism remain unreported. Considering this, we devised the experiment to examine the effects of varying dietary lipid levels on juvenile A. davidianus. Given the characteristics of feed for A. davidianus, it is essential to store it for extended periods. In this context, soybean oil emerges as a preferable option due to its ease of preservation and significantly lower cost compared to fish oil, aligning more closely with actual production demands. Therefore, we formulated six group of experimental feeds using soybean oil as the main lipid source to assessed their growth, body composition, digestive and absorptive capacity, and antioxidant under different lipid levels. This study aims to elucidate the lipid requirement of juvenile A. davidianus and provide valuable insights for the formulation of artificial feeds tailored to their needs.
2 Materials and methods
2.1 Experimental diets
According to the dietary characteristics of A. davidianus, we selected soybean oil as the lipid source and fish meal as the primary protein source. Consequently, six groups of isonitrogenous (CP57%) feed were prepared (Table 1). The measured lipid levels of feed (dry matter basis) were L1 (5.02%), L2 (8.96%), L3 (12.90%), L4 (16.21%), L5 (19.46%), and L6 (22.80%). The raw feed materials were processed through a 60-mesh sieve before being formed into pellets with a diameter of approximately 4 mm using a soft pellet feed granulator (Model 9FZ-35C from Sichuan Mianfeng Agricultural Machinery Manufacturing Factory). Following this, the pellets were dried in an oven at 40°C and subsequently packaged in a double-layer plastic bag.
2.2 Experimental procedures
The A. davidianus used in this experiment were purchased from the Luyuan Giant Salamander Farm in Hanzhong, Shaanxi Province, China. They were treated with 1% NaCl solution for 5 minutes, followed by a 3-week acclimation period in an indoor freshwater tank where they feeded with basic feed (57% crude protein) before the formal experiment. A total of 144 healthy A. davidianus(34.20 ± 0.27g) were randomly allocated into six experimental groups. Each of these groups was then subdivided into three replicates, consisting of eight A. davidianus per replicate. They were raised in square plastic containers (70×45×17.5cm) sourced from the Aquaculture System at College of Fisheries Southwest University. Clean and replace the water in the tank twice daily, ensuring a consistent depth of 5 cm. Throughout the 105-day experimental period, the A. davidianus were fed for two days (feeding to satiety daily) and then fasted for one day because they need longer time for digesting (Guo et al., 2023). Each day, the leftover feed was collected, and their weight recorded. They were reared and provided the diet in dark condition throughout the 105-day study, maintained at 19-23°C, dissolved oxygen>6.0 mg/L, and ammonia-N concentration<0.10mg/L.
2.3 Sample collection
Our research team determined that the intestinal emptying time in A. davidianus is four days. Therefore, they were deprived of food for four days before being harvested at the conclusion of the experiment. Growth data were gathered by documenting both the weight and the count of individuals in each group. From each tank, five A. davidianus individuals were chosen and anesthetized using 0.1 g/kg of MS-222 (75 mg/L; Sigma, St Louis, MO, USA). Blood was collected from the caudal vein with a 1-mL syringe and subsequently transferred into a centrifuge tube containing EDTA. The samples were then centrifuged (4°C, 4000r/min,10min) to obtain plasma, which was stored at −80°C for future analysis. The viscera of the A. davidianus were subsequently dissected, and during this process, the back muscles, skin, stomach, intestine, and liver were separated and washed with phosphate-buffered saline (PBS, 0.064 mol/L, pH 7.4). Furthermore, the liver and intestine tissues designated for histological analysis were preserved in 4% paraformaldehyde and 2.5% glutaraldehyde. The remaining samples were rapidly frozen in liquid nitrogen and stored in the ultralow temperature refrigerator at −80°C for future utilization. All experiments conducted during this study adhered strictly to the established standard protocols set forth by China regarding the care and use of experimental animals. Additionally, this study received formal approval from the Animal Ethics Committee at Southwest University, affirming that the research methods and practices utilized met the necessary ethical standards for animal welfare.
2.4 Parameters of fish growth performance
The growth metrics were assessed as follows:
Initial body weight (IBW, g/fish)
Finial body weight (FBW, g/fish)
Weight gain rate(WGR, %)=100×(FBW-IBW)/IBW
Specific growth rate (SGR, %)=100×(Ln FBW-Ln IBW)/number of feeding days
Feed conversion ratio (FCR) = Dry feed fed (g)/Wet weight gain (g)
Protein retention ratio(PRR)=(FBW×final fish protein− IBW×initial fish protein)/(dry diet intake feed×feed protein)×100%
2.5 Chemical analysis
2.5.1 Proximate composition analyses
The chemical composition analysis of the experimental feed, whole body, and muscle was conducted to determine the moisture, crude protein, crude lipid, and crude ash content. These were assessed using the following methods: the 105°C oven drying method (GB/T 6435-2006) for moisture, the Kjeldahl nitrogen determination method (GB/T 6432-1994) for crude protein, the Soxhlet extraction method (GB/T 6433-1994) for crude lipid, and the 550°C calcination method (GB/T 6438-1992) for crude ash. Collagen was calculated by detecting the content of hydroxyproline multiplied by 11.0. The content of RNA and DNA were assessed according to the technique outlined by (Buckley, 1979).
2.5.2 Digestive and absorptive enzyme activities
Utilizing kits supplied by the Nanjing Jiancheng Institute of Biological Engineering, we assessed the total activities of protease, lipase, amylase, and Na+-K+-ATPase in the intestinal tissue.
2.5.3 Antioxidant enzymes activities in liver
The level of total antioxidant capacity (T-AOC), total superoxide dismutase (T-SOD), and malondialdehyde (MDA) of the liver were all assessed utilizing commercial kits (Nanjing Jiancheng Bioengineering Institute, Nanjing, China).
2.5.4 Plasma biochemical indexes
The content of triglycerides (TG) and total cholesterol (TCHO) in plasma were assessed using specialized kits (Nanjing Jiancheng Institute of Biological Engineering), following the guidelines provided by the manufacturer.
2.5.5 Preparation of tissue section
The liver and intestine paraffin tissue sections were dewaxed by xylene, followed by hydration with a gradient of ethanol. After staining with hematoxylin and eosin (HE), the light microscope (McAuldie SK210) was employed to examine the internal structure of the sections and capture photographs. The number, length and width of intestinal mucosal folds were measured by image-pro plus.
2.5.6 Transmission electron microscopy and scanning electron microscope for ultrastructural examination
The liver tissue was fixed with osmic acid, dehydrated with acetone, and finally embedded in the capsule. Following staining, the samples were examined with a transmission electron microscope (Hitachi HT 7700) and captured in photographs. The intestinal tissues were fixed using osmium acid solution, after which the liquid was poured off. Following this, the tissues were rinsed with distilled water and subjected to dehydration using an ethanol solution. Subsequently, they were treated twice with propylene oxide, dried at the critical point, coated using an EIKO ion sputtering apparatus, and then observed and photographed with a scanning electron microscope (JSM-6380 LV).
2.6 Statistical analysis
One-Way ANOVA was employed to analyze the experimental data, with Duncan’s multiple comparisons utilized to assess the significance of differences between groups. The statistical significance was established at P<0.05. Results are presented as mean ± standard deviation (SD). All analyses were conducted using SPSS 22.0 on the Windows.
3 Results
3.1 Growth performance
The dietary lipid levels significantly affect the weight gain ratio (WGR), specific growth rate (SGR), feed conversion ratio (FCR), and protein retention ratio (PRR) in A. davidianus (P<0.05). Among these groups, the L4 group (16.21%) exhibited the highest value of FBW WGR, SGR, PRR, and the lowest FCR (P<0.05). WGR in the L4 group increased by 79.5% and 217.2% compared to the L1 (5.02%) and L6 (22.80%) groups, respectively. The RNA/DNA ratio of juvenile A. davidianus reached its highest value in L4 group (16.21%) and lowest value in group L6 (22.80%), even lower than that of the L1 group (Table 2). The quadratic analysis conducted on WGR (Figure 1), muscle RNA/DNA ratio (Figure 2) in relation to dietary lipid levels indicated that the optimal dietary lipid requirement for juvenile A. davidianus were 13.3%, 13.4%, respectively.
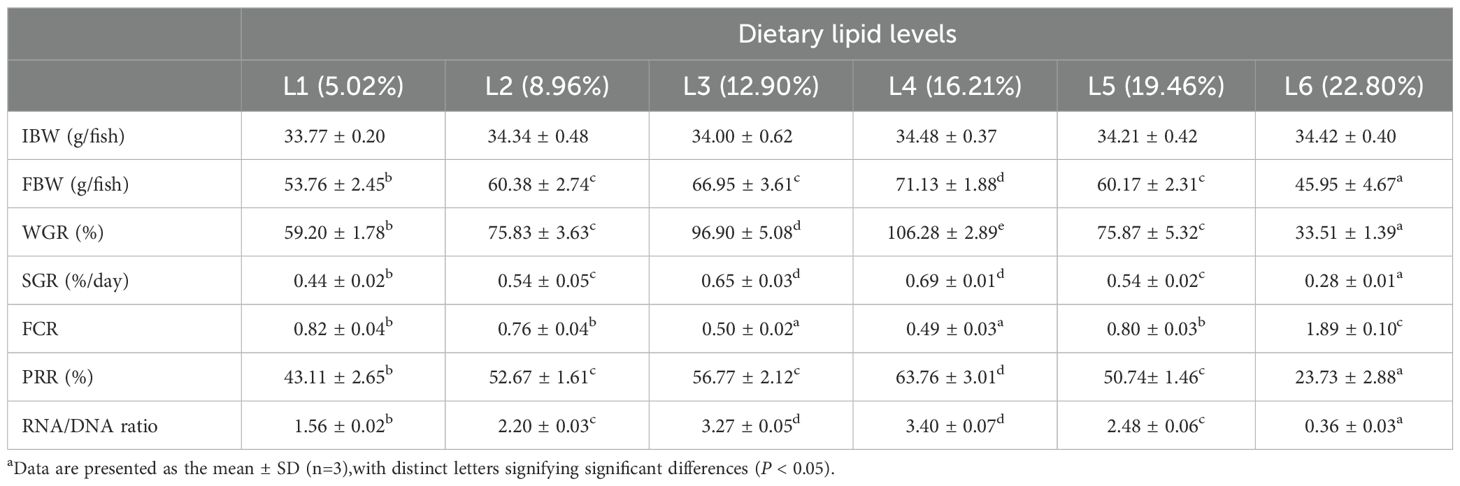
Table 2. Effects of dietary lipid levels on the growth performance and feed utilization of juvenile Andrias davidianus.
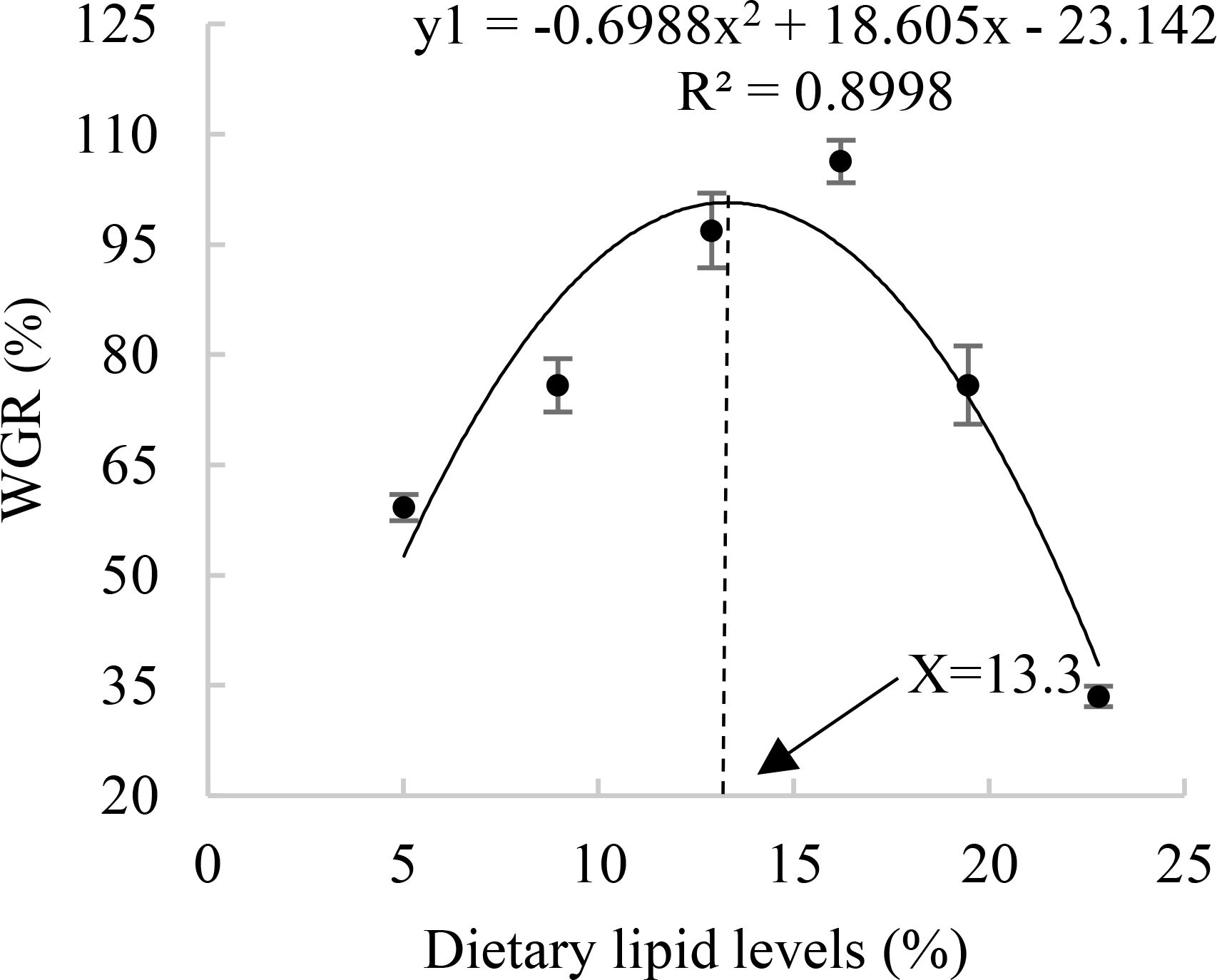
Figure 1. Relationship between the weight growth rate (WGR, %) and the dietary lipid levels of juvenile Andrias davidianus.
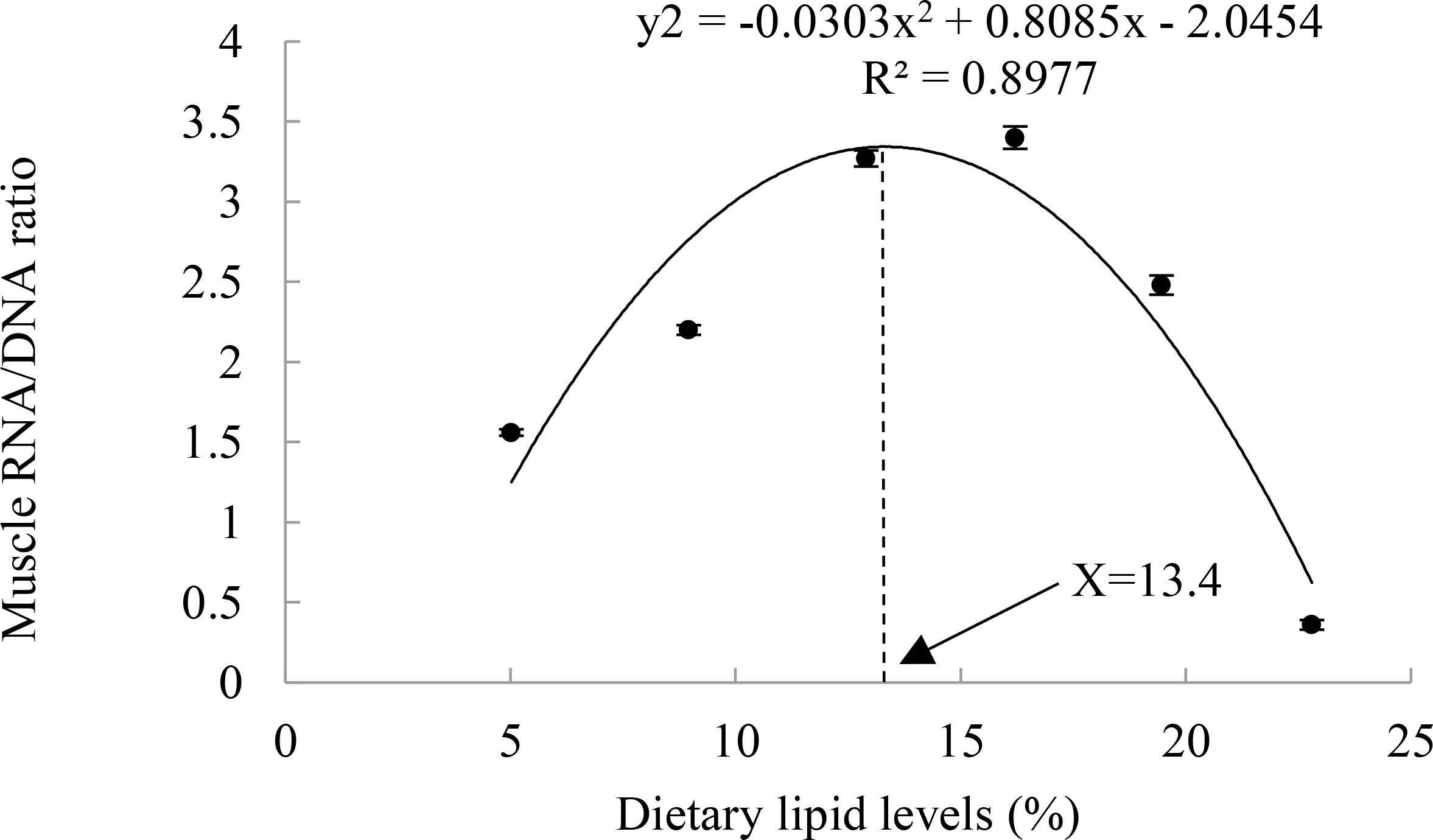
Figure 2. Relationship between muscle RNA/DNA ratio and dietary lipid levels of juvenile Andrias davidianus.
3.2 Proximate composition and collagen content
The whole-body ash and moisture content of A. davidianus were not significantly influenced by the dietary lipid level (P>0.05), whereas crude protein initially rose and subsequently fell as the dietary lipid level increased (P<0.05). As the dietary lipid levels increased, there was a notable decrease in muscle moisture content (P<0.05), while crude protein first climbed and subsequently declined, peaking in the L4 group (16.21%). In contrast to the trend of crude protein, the ash content exhibited an opposite trend.
The lipid level in whole-body and liver of A. davidianus showed an initial increase followed by a decline with the increasing dietary lipid levels. The highest lipid content in the whole body was observed in the L4 group (16.21%), while liver lipid reached its maximum in the L3 group (12.90%). Additionally, the lipid content in both muscle and uropygial gland rose with higher dietary lipid levels, peaking at 16.21% (P<0.05), and subsequently plateaued beyond this level. The maximum skin collagen content was found in the L4(16.21%) group(P<0.05). The quadratic analysis conducted on skin collagen in relation to dietary lipid levels indicated that the optimal dietary lipid requirement for juvenile A. davidianus was 16.0% (Figure 3). No notable variation in muscle collagen level was observed among the groups (P>0.05, Table 3).
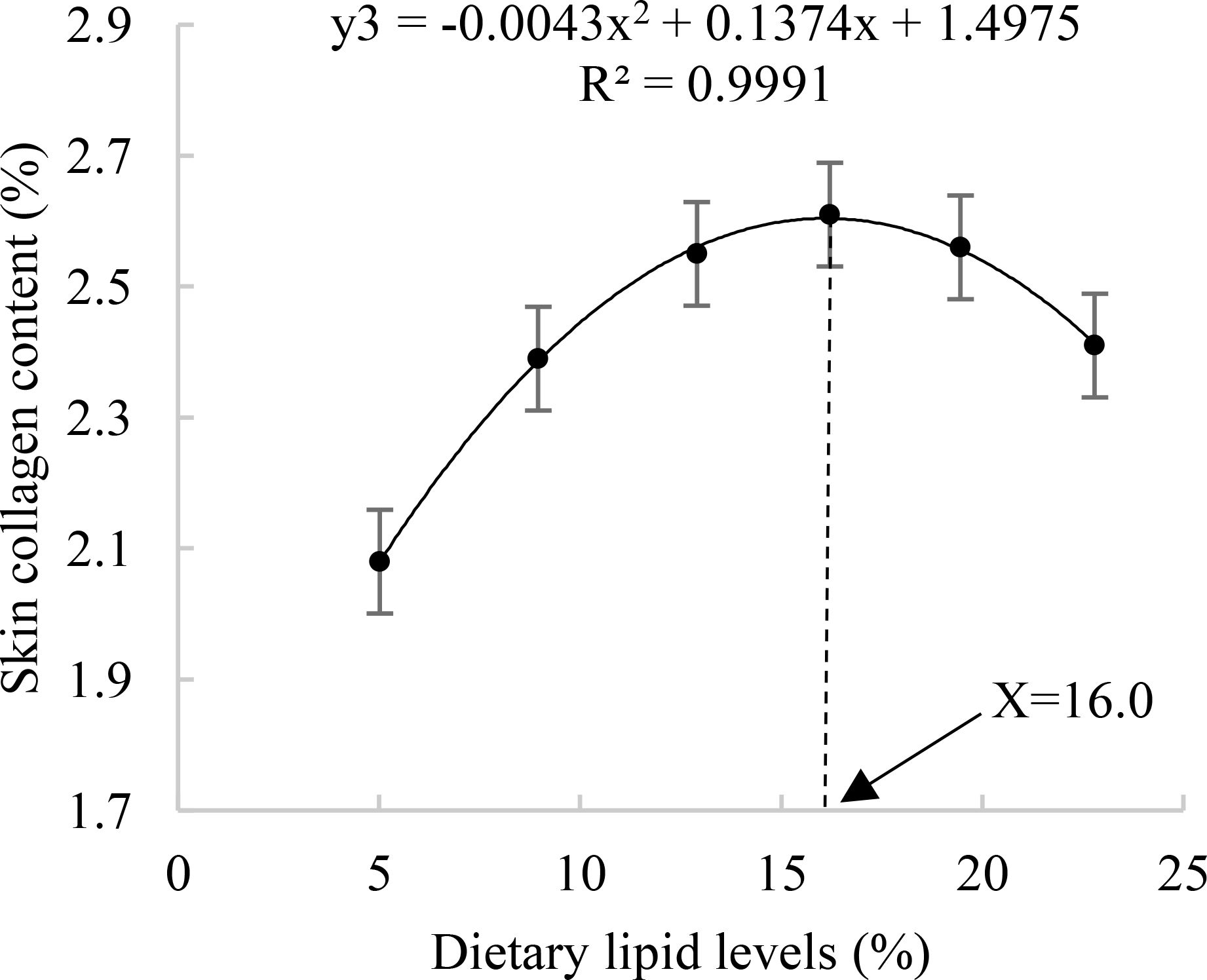
Figure 3. Relationship between skin collagen lipid (%) and dietary lipid levels of juvenile Andrias davidianus.
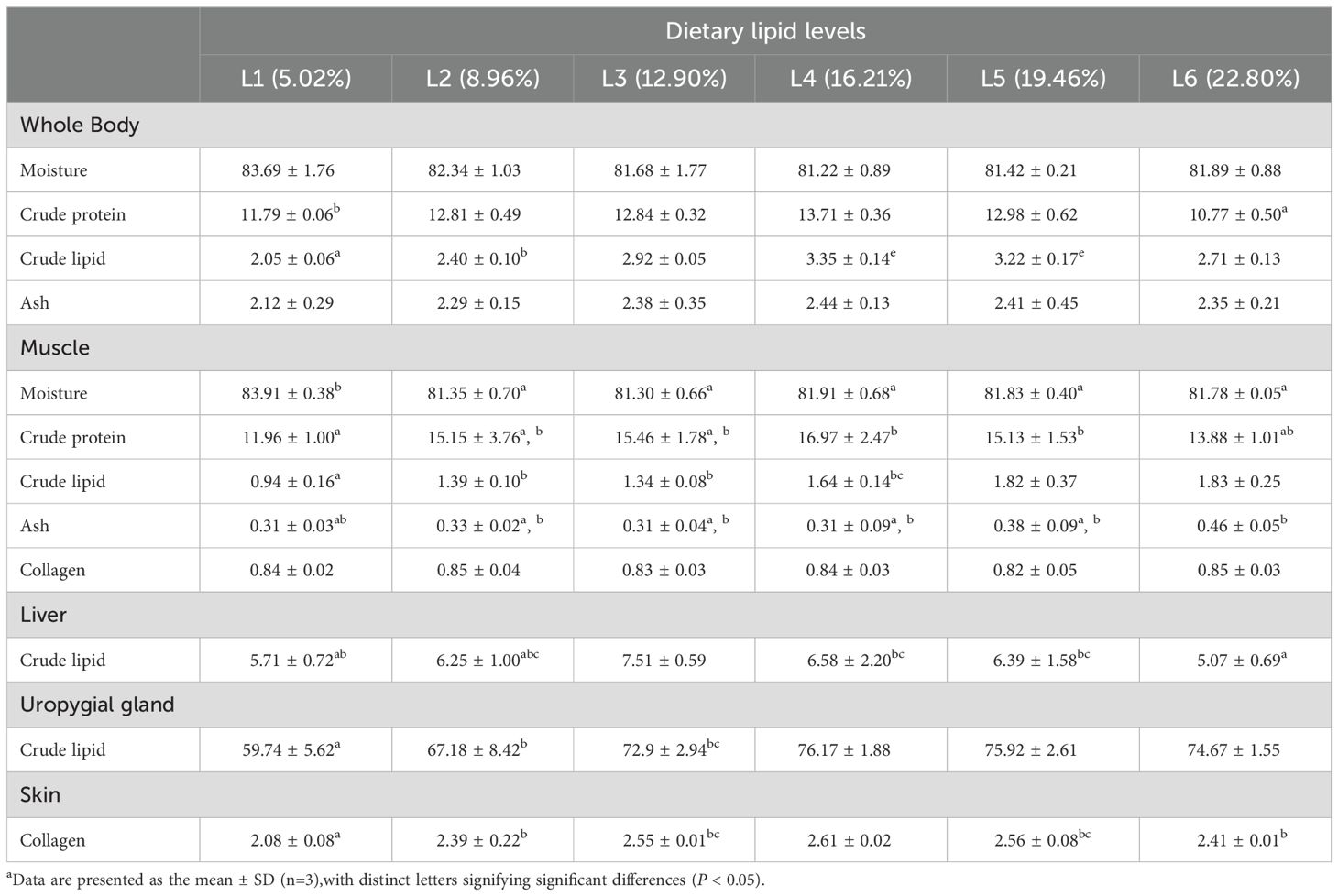
Table 3. Effects of dietary lipid levels on body composition and skin collagen protein of juvenile Andrias davidianus (%).
3.3 Intestinal digestive and absorptive enzyme activity
Dietary lipid levels significantly influenced intestinal trypsin, lipase, amylase, and Na+-K+-ATPase; the L3 group exhibited the highest concentration of trypsin and Na+-K+-ATPase (P<0.05), the maximum activity of the other enzymes was observed in the L4 group. The quadratic analysis conducted on lipase activity in relation to dietary lipid levels indicated that the optimal dietary lipid requirement for juvenile A. davidianus were 17.5% (Table 4; Figure 4).
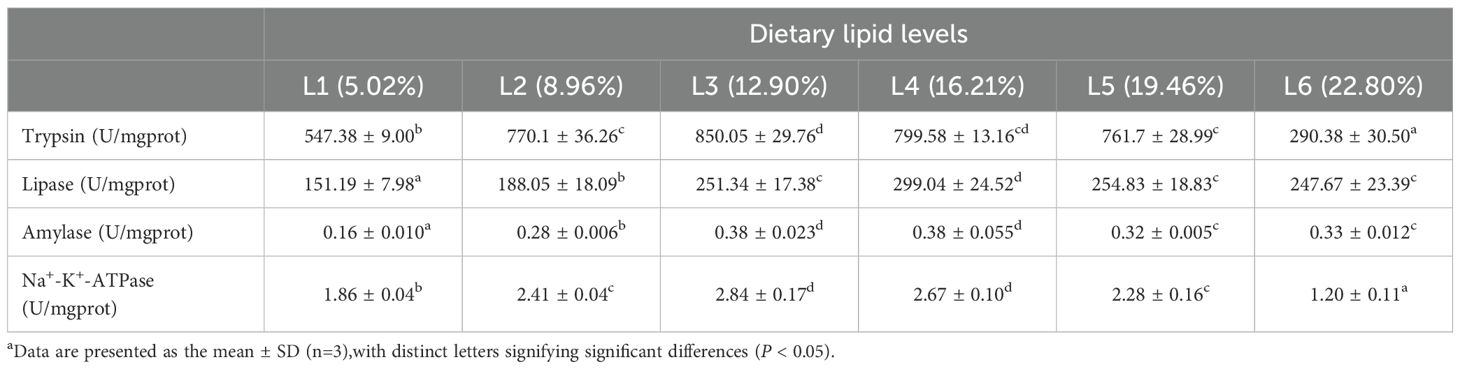
Table 4. Effects of dietary lipid levels on intestinal digestive and absorptive enzyme activity of juvenile A. davidianus.
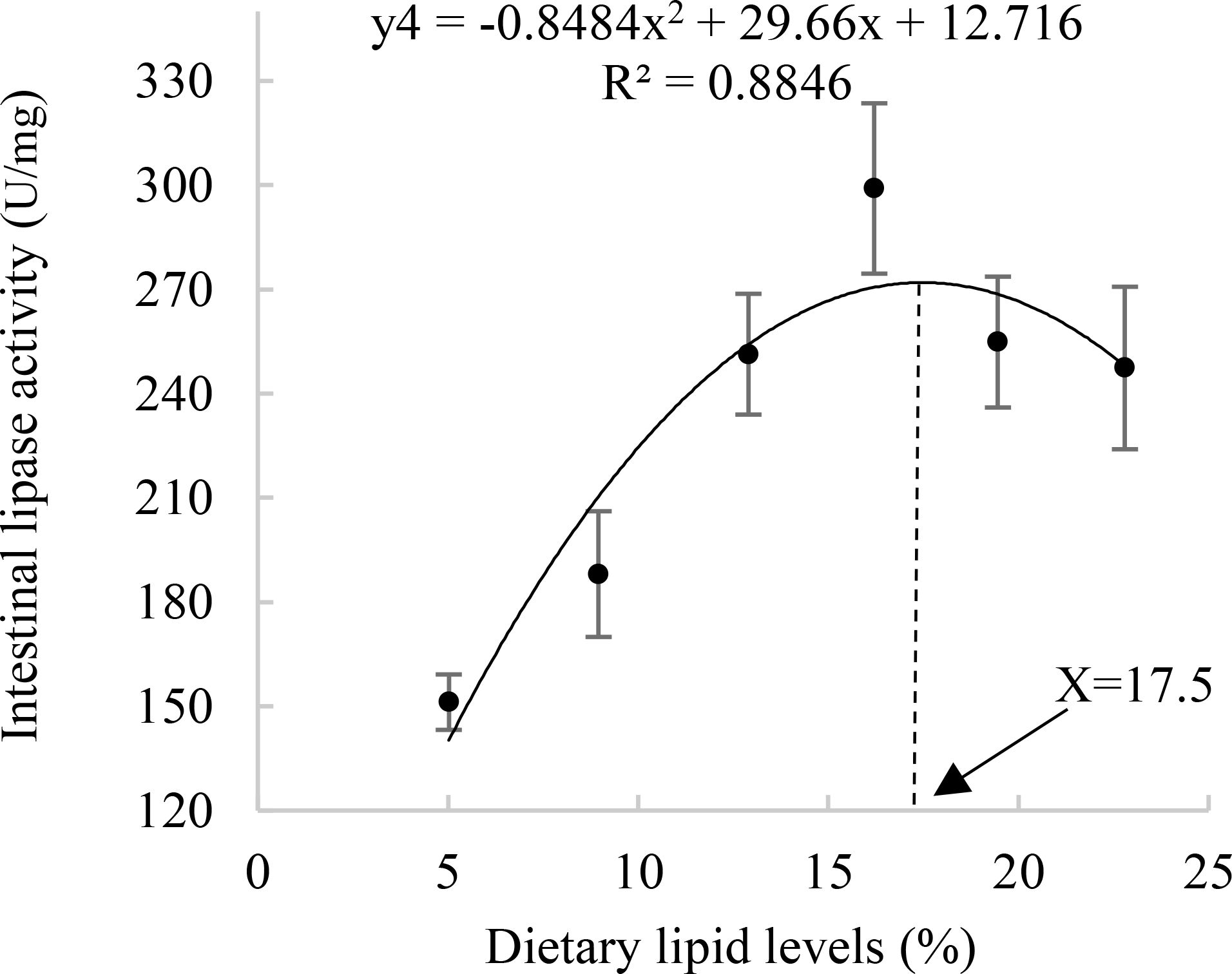
Figure 4. Relationship between Intestinal lipase activity (U/mg) and dietary lipid levels of juvenile Andrias davidianus.
3.4 Intestinal morphology
The height, number, and width of intestinal mucosal folds in juvenile A. davidianus showed remarkably increases in the L4 (16.21%) group when compared to those in L1(5.02%) group and L6 (22.80%) group (P < 0.05, Figure 5; Table 5).
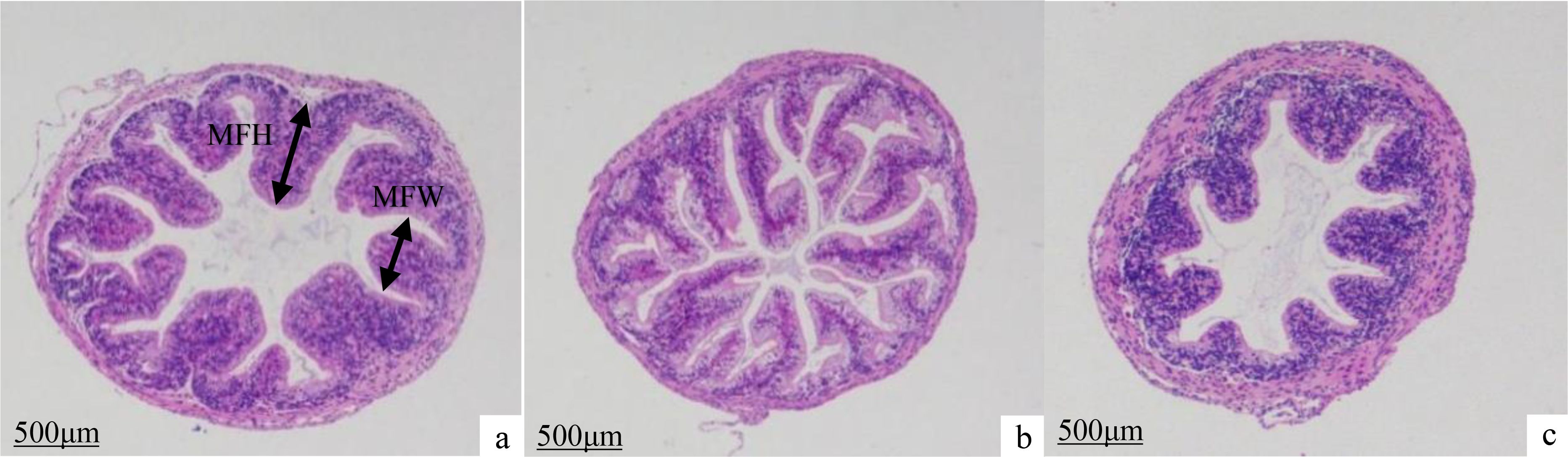
Figure 5. Effects of dietary lipid levels on intestinal morphology of juvenile Andrias davidianus, HE staining was performed. (A) L1 (5.02%), (B) L4 (16.21%) (C) L6 (22.80%), MFH, mucosal fold heigh; MFH, mucosal fold width.
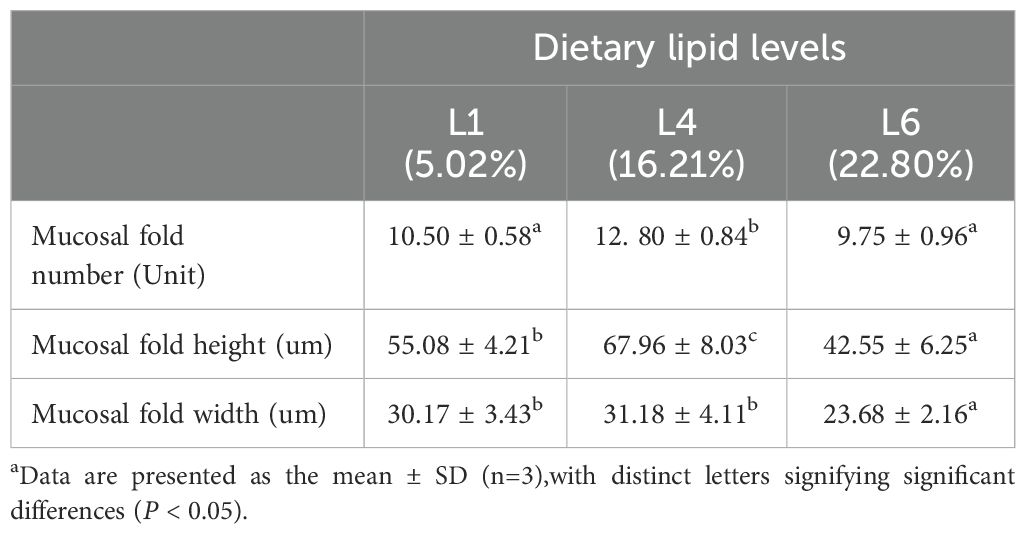
Table 5. Effects of dietary lipid level on intestinal morphology indices of juvenile Andrias davidianus.
Intestinal microvilli in the 16.21% group were more neatly arranged and densely packed, with matchhead-like heads of the microvilli (Figure 6B), compared with those in the L1(Figure 6A) and L6 group (Figure 6C). Although the heads of the microvilli in the L1 group were seen to be matchhead-like but not full, and the heads of the microvilli was severely damaged in the L6 group.
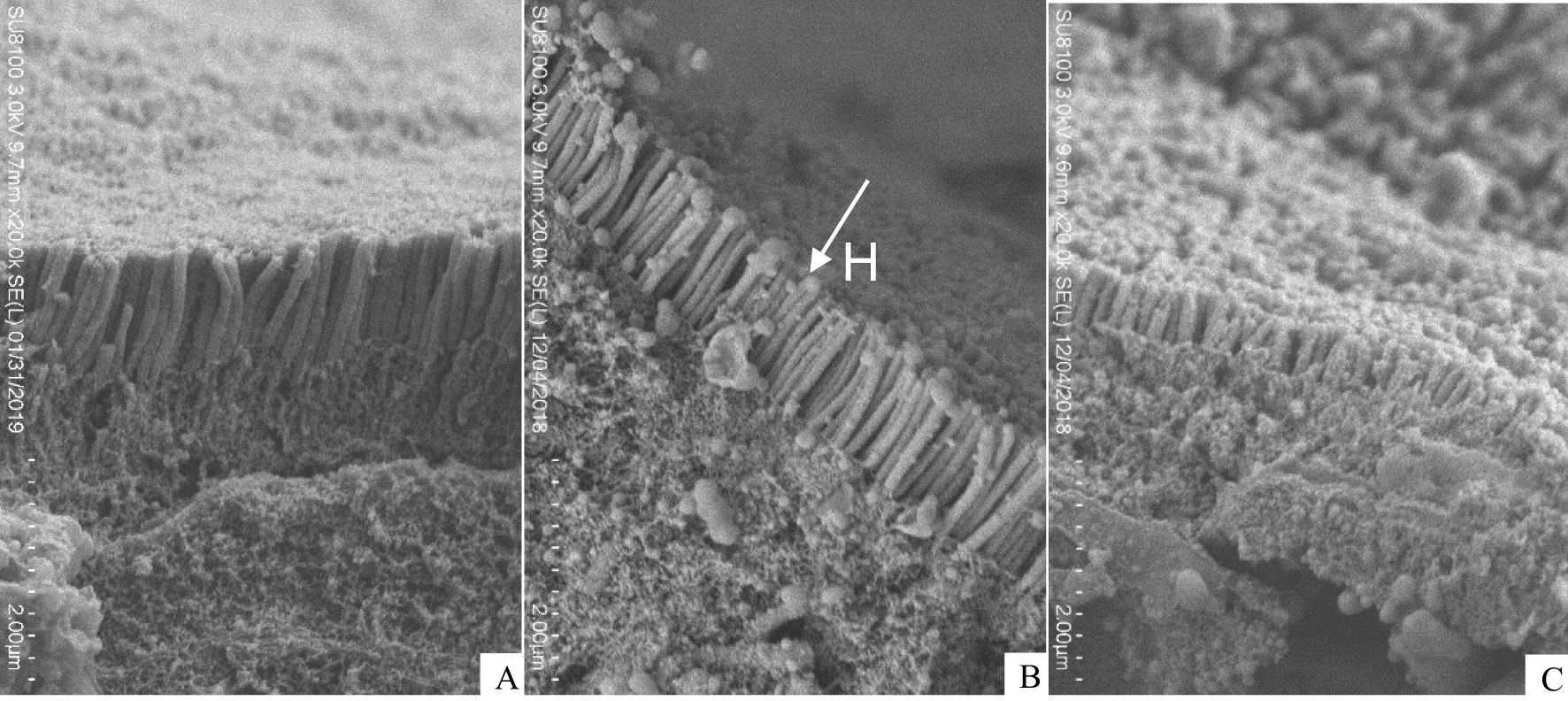
Figure 6. Scanning electron microscope (SEM) of juvenile Andrias davidianus. (A) L1 (5.02%), (B) L4 (16.21%), (C) L6 (22.80%), H: microvilli head.
3.5 Plasma lipid metabolism indexes
Both triglycerides (TG) and total cholesterol (TCHO) in the plasma of A. davidianus, initially increased significantly with rising dietary lipid levels, followed by a notable decrease, with maximum values observed between the L3 and L4 groups (P<0.05, Figure 7). The quadratic analysis conducted on Total cholesterol (TCHO) in relation to dietary lipid levels indicated that the optimal dietary lipid requirement for juvenile A. davidianus was 13.83% (Figure 8).
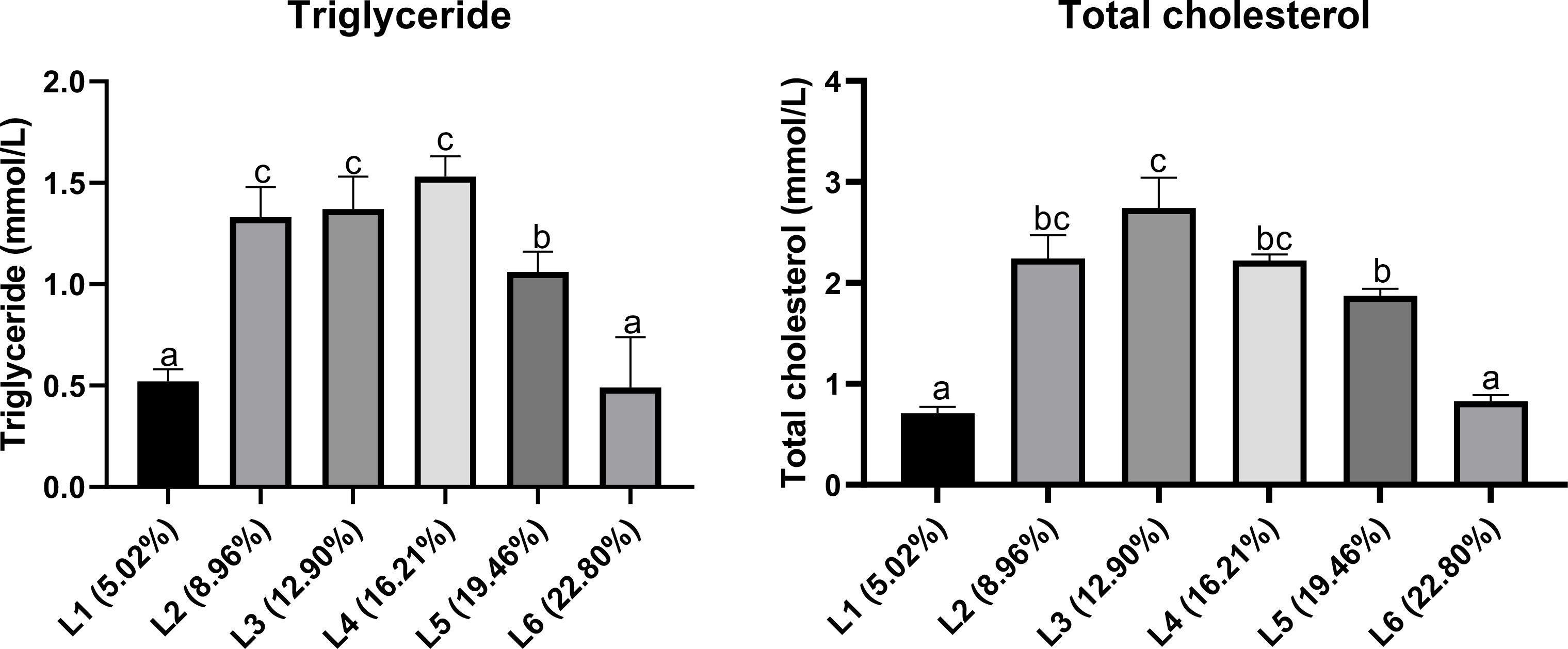
Figure 7. Effects of dietary lipid levels on plasma lipid metabolism indexes of juvenile Andrias davidianus. Values (mean ± SE) in the same row with different superscripts are significantly different using Duncan’s test (p ≤ 0.05).
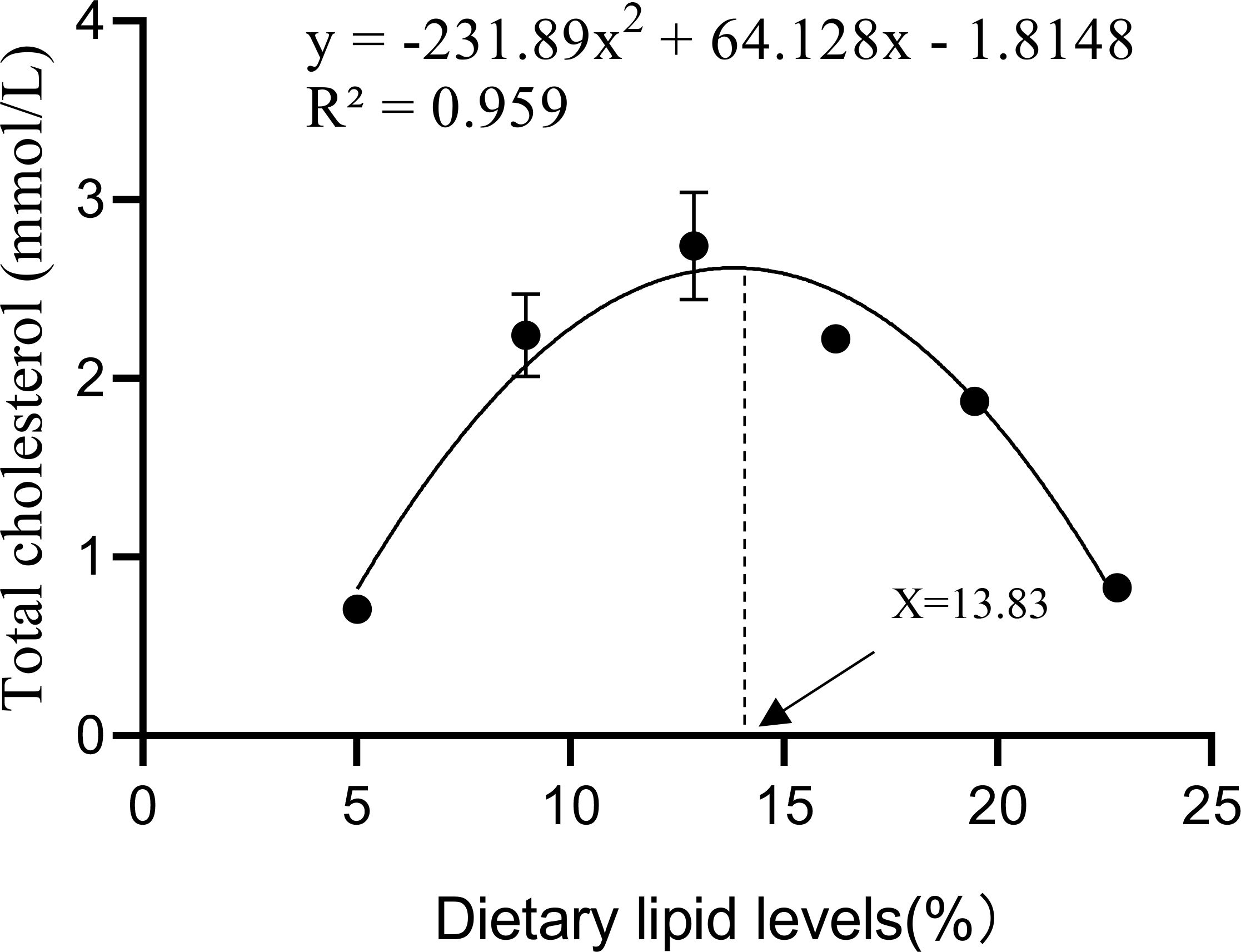
Figure 8. Relationship between the Total cholesterol (mmol/L) and dietary lipid levels of juvenile Andrias davidianus.
3.6 Liver antioxidant indices
As shown in Figure 9, highest SOD and T-AOC activities was noted in the L4(18.1%) group and then decreased with a further increase in lipid(P<0.05). MDA content decreased with the increasing lipid levels in the diet up to16.21% and then increased with further inclusion of lipid in the diet (P<0.05).
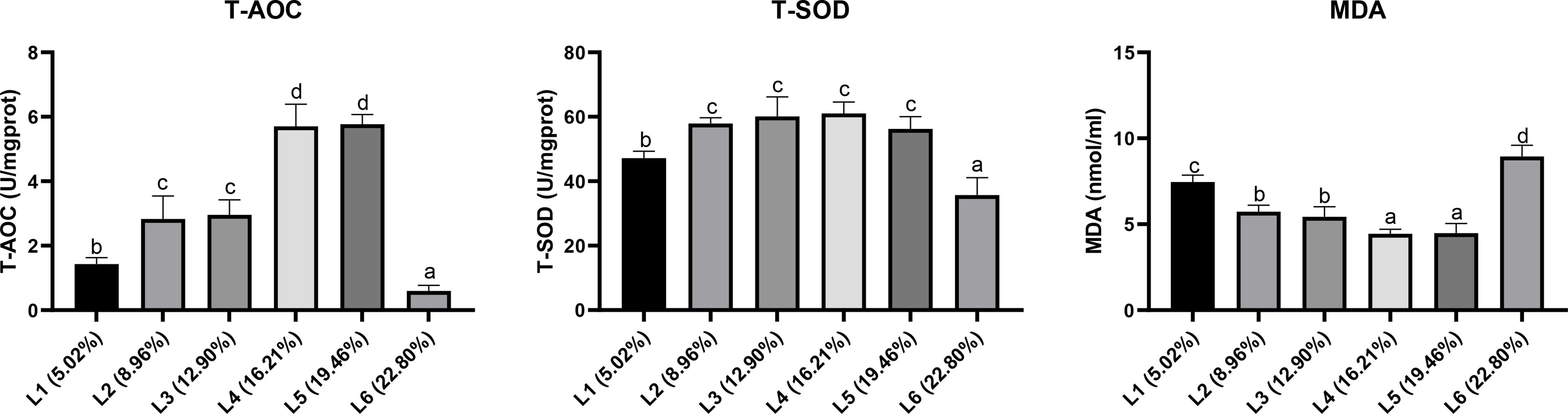
Figure 9. Effects of dietary lipid levels on liver antioxidant indices of juvenile Andrias davidianus. Values (mean ± SE) in the same row with different superscripts are significantly different using Duncan’s test (p ≤ 0.05).
3.7 Liver morphology
Compared with the L1 group (Figure 10A), the L4 group (Figure 10B) exhibited a small amount of brownish-yellow pigment phagocytosed by macrophages (Figure 10B, red arrow) and well-aligned hepatocytes, with no additional lesions observed. Conversely, the L6 group has extensive hepatocyte swelling with loose cytoplasm and vacuolization (Figure 10C, black arrow), diffuse central venous as well as periportal hepatocyte necrosis in the confluent area, and a substantial quantity of brownish-yellow pigment phagocytosed by macrophages was evident (Figure 10C, red arrow).
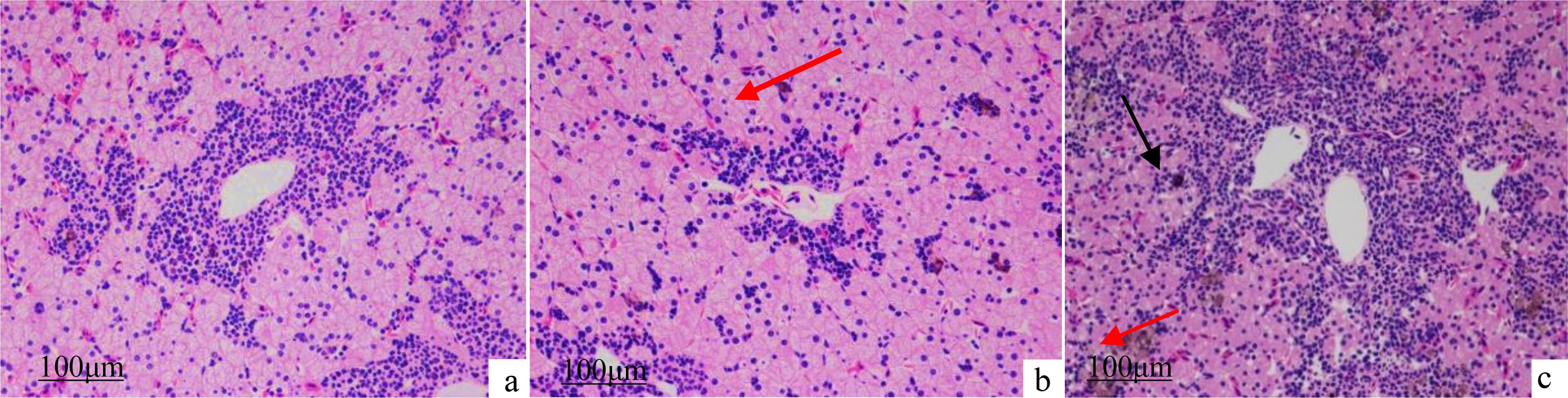
Figure 10. Effects of dietary lipid levels on liver morphology of juvenile Andrias davidianus, HE staining was performed. (A) L1 (5.02%), (B) L4 (16.21%) (C) L6 (22.80%), red arrow: brownish-yellow pigment, black arrow: hepatocyte swelling.
Ultrastructural analysis revealed that the livers of A. davidianus in the L1 group appeared normal, characterized by hepatocytes with large, centrally located round nuclei and moderate amounts of cytoplasm (Figure 11D). In contrast, the hepatocytes found in the L4 group showed a significant buildup of lipid droplets in addition to the occurrence of autophagosomes (Figure 11E). The livers of A. davidianus fed the high-lipid diet exhibited numerous irregularities. In the L6 group, there was an increase in hepatocyte autophagosomes accompanied by a decrease in lipid droplets. Additionally, the nucleus was displaced towards the cell periphery, while the cell membrane exhibited signs of degradation and the cytoplasmic volume was reduced. Furthermore, mitochondria in the L6 group were notably swollen and rounded (Figure 11F).
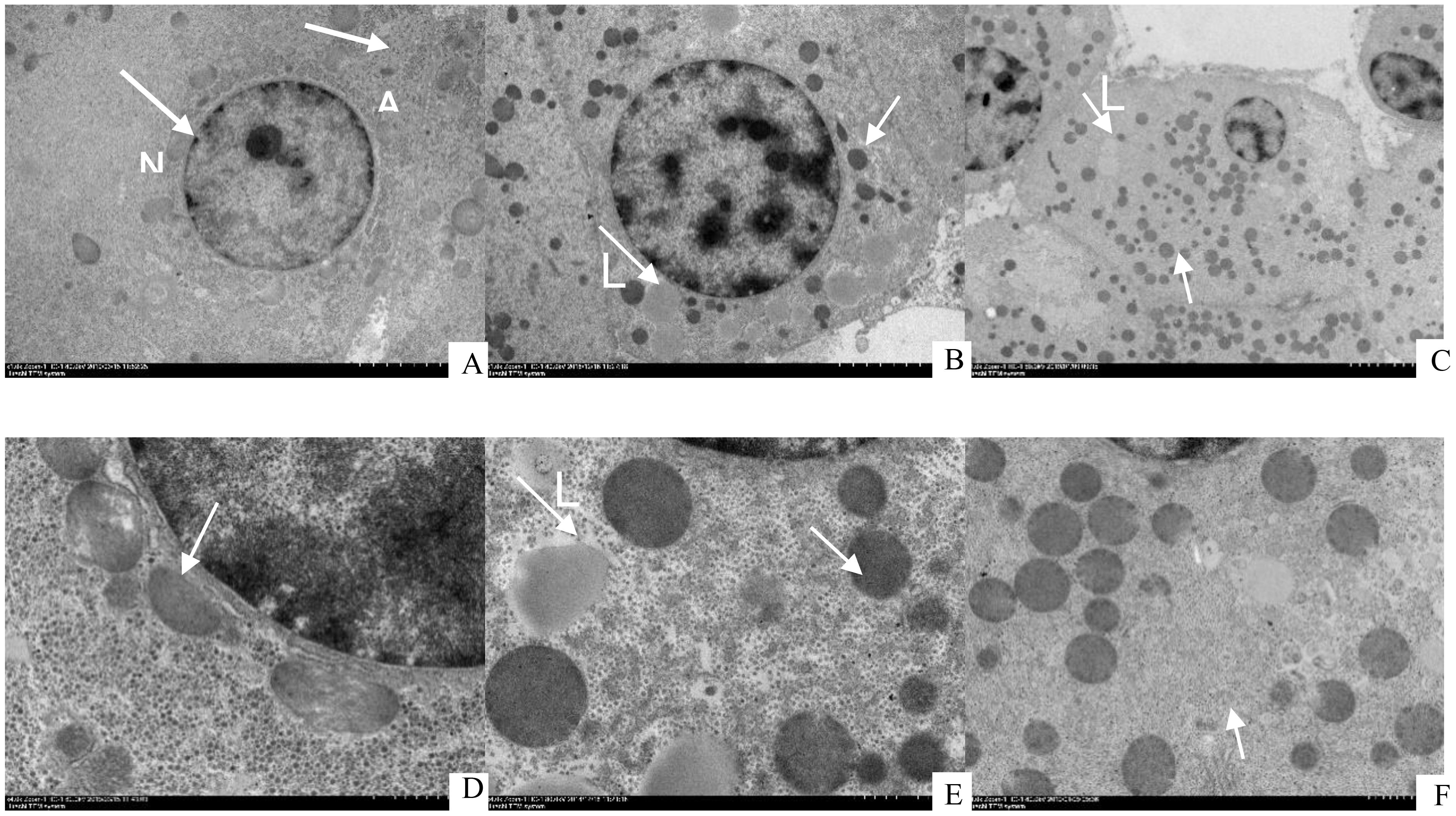
Figure 11. Transmission electron microscope (TEM) images of juvenile Andrias davidianus (A) L1 (5.02%), (B) L4 (16.21%), (C) L6 (22.80%), (D) L1 (5.02%), (E) L4 (16.21%), (F) L6 (22.80%), N, Nucleus; A, Autophagosome; M, Mitochondria.
4 Discussion
Numerous studies have evidenced that the ideal dietary lipid level differ among various fish species (NRC, 2011). This research indicated that the dietary lipid level had a considerable impact on the growth performance of juvenile A. davidianus. Comparable results were observed in Rainbow Trout, Oncorhynchus mykiss (Chen et al., 2023), Rockfish, Acipenser schrenckii (Li et al., 2023a), white seabass, Atractoscion nobilis (López et al., 2009), silver barb, Puntius gonionotus (Mohanta et al., 2008). Notably, the WGR and SGR reached their peak at the dietary lipid level of 16.21%. Juvenile A. davidianus fed high lipid diet appear growth depression may because of the damage to the intestinal structure resulting in decreased digestion and absorption (Chang et al., 2018). In addition, high-lipid diet could cause abnormal lipid metabolism in liver, resulting in pathological changes of liver. Through quadratic regression analysis of weight gain rate (WGR), muscle RNA/DNA ratio, lipase activity, total cholesterol and skin collagen content with dietary lipid level concluded that the optimal dietary lipid level for juvenile A. davidianus is 13.3%-17.5%.
Increasing dietary lipid levels has been shown to promote growth and protein utilization (Ai et al., 2004; Han et al., 2014). The experimental findings indicate that the optimal levels of FCR and PRR were achieved at the dietary lipid level of 16.21%. While growth and feed efficiency indicate the protein-sparing benefits of higher lipid content, the PRR may be influenced when the dietary lipid surpasses 16.21%. Furthermore, the synthesis of proteins relies on RNA, which normally maintains a consistent level of DNA within cells (Clemmesen, 1994). The RNA/DNA ratio governs the ability of cell to generate RNA and synthesize protein, and the decrease of RNA/DNA ratio indicates that there are factors influencing growth of juvenile A. davidianus. In the present experiment, the RNA/DNA ratio increased to 16.21% with the dietary inclusion of lipid. This finding implies that such dietary lipid level favor optimal protein synthesis in A. davidianus, subsequently enhancing growth performance. This result is consistent with the earlier studies in largemouth bass, Micropterus salmoides (Guo et al., 2019), totoaba, Totoaba macdonaldi (Perez-Velazquez et al., 2017). Appropriate lipid intake can increase the metabolic energy of A. davidianus, improve protein utilization efficiency, and promote growth.
Collagen, abundantly in the skin, bones, muscles, and various other tissues, represents the predominant structural protein found in all animal species (Shoulders and Raines, 2009). The result of this experiment shows that the collagen of A. davidianus skin is higher than that of muscle. This study has demonstrated that optimal dietary lipid level is conducive to the synthesis of skin collagen, while excessive or insufficient lipid levels exert adverse effects. This phenomenon may be attributed to the fact that when lipid level is too low, the body primarily uses lipid to provide energy for the synthesis of skeletal muscles and other proteins (Guillard and Schwörer, 2024). Conversely, excessively high lipid level could lead to significant damage to the intestinal and liver structures, impairing intestinal digestion and absorption, as well as causing liver dysfunction. This impairment reduces the ability to metabolize lipid, consequently decreasing the availability of dietary fatty acids and inhibiting collagen synthesis (Meng et al., 2006). The result of this experiment showed a quadratic relationship between skin collagen and dietary lipid levels, with an optimal lipid level of 16.0%. Nevertheless, the exact mechanism by which appropriate lipid level promote collagen formation requires further investigation.
After being digested and absorbed, the lipid is used for tissue construction and energy storage, mainly in viscera, liver, muscles and other tissues (Guo et al., 2019). In contrast, the lipid of A. davidianus is mainly concentrated in the tail, in which unsaturated fatty acids account for 71.3%. Numerous earlier studies have shown that excessive dietary lipid level in lumpfish, Cyclopterus lumpus (Berge et al., 2023), rockfish, Sebastes schlegeli (Li et al., 2023b), and red-tailed catfish, Hemibagrus wyckioides (Hung et al., 2017), can lead to an accumulation of lipid in the visceral cavity and liver tissue, which in turn raises the whole body lipid concentration. This findings indicated that higher dietary lipid level resulted in reduced lipid content in both the liver and body of juvenile A.davidianus. This phenomenon may be due to the damage of liver function caused by metabolic disorder and the obstruction of lipid synthesis, as confirmed by the liver antioxidant indices. The lipid content of muscle and uropygial gland remained stable following elevated dietary lipid, suggesting that the liver of A. davidianus is more sensitive compared to other tissues. This sensitivity represents a significant limiting factor in its capacity to effectively utilize lipid resources.
Intestinal digestive enzyme activity and intestinal tissue development directly reflect the ability of the intestine to digest and absorb nutrients (Ghasemi et al., 2020; Sesay et al., 2016). The findings indicated a significant inhibition of trypsin activity in the intestine with the consumption of the high-lipid diet, as evidenced by a decrease in activity when dietary lipid levels exceeded 12.90%. Furthermore, earlier research has demonstrated that increased lipid content was associated with increased lipase activity in larval sea bass, Dicentrarchus labrax (Morais et al., 2004), teleost pejerrey, Odontesthes bonariensis (Gómez-Requeni et al., 2013), and Atlantic salmon, Salmo salar (Krogdahl et al., 2015). It suggests that the A. davidianus could adapt to the increasing lipid levels in the diet by regulating their own lipase activity, but when the dietary lipid level exceeded 16.21%, the lipase activity decreased significantly. These results are related to the species of experimental animals, feed composition and living environment. Based on the liver antioxidant index and transmission electron microscopy analysis, it was observed that the liver of A. davidianus experienced impairment because of the high-lipid diet, potentially leading to compromised bile secretion and decreased availability of substrates for lipase binding. Comparable outcomes have been identified in the whiteleg shrimp, Litopenaeus vannamei investigation (Hamidoghli et al., 2019).
The intestine, as the largest immune system and digestive organ in the body, could selectively absorb essential nutrients that aid in combating external pathogens and other detrimental substances (Lee et al., 2017). The interior wall of intestine, whose morphological integrity is the basis for the function of intestine, is composed of folds, villi and microvilli. The increase in mucosal fold height and number implies an increase in nutrient-absorbing surface area (Ramos et al., 2017). In the present investigation, appropriate dietary lipid can improve the intestinal morphology of fish by increasing the width and height of intestinal mucosal fold. Additionally, the findings from the electron microscope analysis an overabundance of dietary lipid hindered the development of intestinal villi in A. davidianus, leading to a reduction in absorptive surface area. Moreover, Na+-K+-ATPases are proteins embedded in cell membranes that provide energy for ion transport by hydrolyzing ATPases (Gorini et al., 2002), thus enhancing the active transport and absorption capacity of amino acids, glucose and other nutrients in the intestine (Kopecka-Pilarczyk, 2010). It has been demonstrated that Na+-K+-ATPase activity is directly related to intestinal digestion and absorption capacity (Almansa et al., 2003). The experimental results show that appropriate lipid inclusion in the diet can improve the activity of Na+-K+-ATPase in the intestine of A. davidianus, thus promoting the absorption and deposition of nutrients.
Triglyceride (TG) is mainly involved in the production and storage of energy in the body, serving as a crucial substrate for energy metabolism. TCHO is the raw material for the synthesis of bile acids, vitamin D, and steroid hormones, and is also involved in the formation of cell membranes. TCHO and TG in plasma are regarded as key indicators connected to lipid metabolism, providing insight into the nutritional condition of fish. Earlier research efforts concerning various fish species have observed that TG and TCHO consistently rose as dietary lipid levels increased (Du et al., 2006; Hansen et al., 2008; Yan et al., 2015). In the current investigation, a markedly reduced concentration of TG and TCHO in plasma was observed in the lowest dietary lipid group. Additionally, as the dietary lipid level rose, the content of TG and TCHO exhibited a rising tendency. This observation suggests that the transport of endogenous lipid becomes more active in reaction to elevated dietary lipid level (Du et al., 2005). However, when the dietary lipid level exceeds 16.21%, the level of TCHO and TG decreases significantly. Similar conclusion to the present experiment were reached in the studies of the Hiphaestus fuliginosus) (Wang et al., 2010) and Sebastiscus marmoratus (Shi et al., 2013), in which the reduction of TG and TCHO levels in the blood could be the result of bile secretion abnormality or liver dysfunction.
The process of lipid metabolism in the liver is intricate, and abnormalities at any stage can result in disorder associated with lipid metabolism (Karavia et al., 2013). Excessive lipid diet leads to cell swelling, nuclear translocation, accumulation of lipid droplets (Cao et al., 2018), and hepatocellular hypertrophy with increased cell diameters (Ragab et al., 2015). The impacts have been comprehensively documented among different fish species (Lin and Yeh, 2022; Zhao et al., 2023). These changes in liver of A. davidianus in this study also support this assertion. During cell growth and development, intracellular biomolecules and damaged organelles can be degraded and recycled by cellular autophagy (Van den Bossche et al., 2017). Under normal physiological condition, autophagy actively participates in cellular differentiation, growth, and development, as well as in the prevention of neurodegeneration and the regulation of immune responses at the cellular level (Papáčková and Cahová, 2014). Additionally, autophagy can be upregulated in response to stressors such as starvation, hypoxia, and DNA damage, thereby has offering cellular protective mechanisms. However, if cellular autophagy is overactive, it can damage normal organelles and cause autophagic death (Murray and Wynn, 2011). Significant changes in the ultrastructural composition were noted after the intake of diet excessive in lipid in the current research. As the level of feed lipid increased, the number of autophagosomes in liver cells also rose, and the mitochondria in the highest lipid level exhibited swelling and rounding. The result suggests that high-lipid diet leads to oxidative stress in the liver of A. davidianus, resulting in enhanced cellular autophagy, liver damage and significant growth inhibition. A deeper insight into these histological alterations in the liver could be essential for exploring metabolic disorders (Lu et al., 2013).
Chronic consumption of high-lipid diet results in the accumulation of lipid within cells, which in turn causes an overproduction of reactive oxygen species (ROS) (Schieber and Chandel, 2014). These ROS can trigger permanent alterations in both cells and tissues (Chen et al., 2024). This study found that MDA content in A. davidianus livers increased significantly as dietary fat content increased, suggesting that extremely high dietary lipid content increases lipid peroxidation (Mas-Bargues et al., 2021; Baskol et al., 2007). T-AOC reflects the total antioxidant level of the organism, and SOD, as an important antioxidant enzyme in the antioxidant system of aquatic animals. Reactive oxygen species, including superoxide anion radicals, free oxygen, and H2O2, can be efficiently scavenged by them, helping to reduce the harm caused to tissues and cells by free radicals resulting from PUFA peroxidation (Valko et al., 2004). In the current investigation, the activity of those enzymes increased when dietary lipid levels increased from 5.02% to 16.21% and declined when dietary lipid levels exceeded 16.21%. The increase in enzyme activity might be the result of maintaining the proper ratio of ROS to antioxidant, shielding the liver cells from their harmful effects (Paital and Chainy, 2010) Therefore, to mitigate significant oxidative stress, it is anticipated to uphold elevated levels of antioxidant enzyme production. When the generation of reactive oxygen species (ROS) exceeds the capacity of antioxidant defenses, organisms experience oxidative stress, leading to detrimental effects (Bize et al., 2008). Consequently, the antioxidant enzymes displayed a declining trend when the A. davidianus was fed diet beyond 16.21% lipid level, indicating the possibility of oxidative stress. Comparable findings were observed in prior studies involving different species (Deng et al., 2021; Ebadi et al., 2021; Liou et al., 2023).
5 Conclusion
Through quadratic regression analysis using WGR, skin collagen content, muscle RNA/DNA ratio, total cholesterol (TCHO), and lipase activity as indicators, it was determined that the optimal lipid level of A. davidianus is 13.3% to 17.5%. This dietary lipid level can significantly improve skin collagen content, antioxidant capacity and the health of intestine and liver, thereby positively influencing grow perform. Diet that contains either insufficient or excessive lipid can adversely affect health of A. davidianus, and excessive lipid diet has the most serious impact. Specifically, high-lipid diet result in lipid metabolism disorder, oxidative stress, and detrimental effects on the intestinal and liver health of A. davidianus.
Data availability statement
The original contributions presented in the study are included in the article/supplementary material. Further inquiries can be directed to the corresponding author.
Ethics statement
The animal study was approved by Southwest University’s Animal Ethics Committee. The study was conducted in accordance with the local legislation and institutional requirements.
Author contributions
XY: Formal analysis, Investigation, Methodology, Resources, Visualization, Writing – review & editing, Software. LP: Data curation, Supervision, Writing – review & editing. JY: Writing – review & editing, Data curation, Formal analysis, Software. SW: Data curation, Formal analysis, Investigation, Methodology, Resources, Writing – original draft. YL: Funding acquisition, Supervision, Writing – review & editing. MZ: Funding acquisition, Project administration, Resources, Supervision, Writing – review & editing. XZ: Project administration, Supervision, Writing – review & editing. YX: Project administration, Supervision, Validation, Writing – review & editing. LL: Funding acquisition, Supervision, Visualization, Writing – review & editing.
Funding
The author(s) declare financial support was received for the research, authorship, and/or publication of this article. This research received valuable support from Integrative Science Center of Germplasm Creation in Western China (CHONGQING) Science City, Chongqing Social Livelihood Science and Technology Innovation Project(cstc2016shmszx80084), Chongqing Ecological Fishery Industry System and Chongqing Fishery Technology Innovation Union (2023).
Acknowledgments
We express our sincere gratitude to Z.F. Li and D. Zhou for their vital assistance throughout the experimental process. Furthermore, we acknowledge Y.S. Chen and J.K. Ma for their essential contributions in conducting the chemical analysis involved in our study. Their expertise and efforts were instrumental in achieving accurate results.
Conflict of interest
Authors YL and MZ were employed by the company Shandong New Hope Liuhe Group Co., Ltd.
The remaining authors declare that the research was conducted in the absence of any commercial or financial relationships that could be construed as a potential conflict of interest.
Generative AI statement
The author(s) declare that no Generative AI was used in the creation of this manuscript.
Publisher’s note
All claims expressed in this article are solely those of the authors and do not necessarily represent those of their affiliated organizations, or those of the publisher, the editors and the reviewers. Any product that may be evaluated in this article, or claim that may be made by its manufacturer, is not guaranteed or endorsed by the publisher.
References
Ai Q. H., Mai K. S., Li H. T., Zhang C. X., Zhang L., Duan Q. Y., et al. (2004). Effects of dietary protein to energy ratios on growth and body composition of juvenile Japanese seabass, Lateolabrax japonicas. Aquaculture 516, 230–507. doi: 10.1016/j.aquaculture.2003.09.040
Almansa E., Sánchez J. J., Cozzi S., Rodríguez C., Díaz M. (2003). Temperature-activity relationship for the intestinal Na+-K+-ATPase of Sparus aurata. A role for the phospholipid microenvironment? J. Comp. Physiol. B 173, 231–237. doi: 10.1007/s00360-003-0327-y
Baskol G., Atmaca H., Tanriverdi F., Baskol M., Kocer D., Bayram F. (2007). Oxidative stress and enzymatic antioxidant status in patients with hypothyroidism before and after treatment. Exp. Clin. Endocrinol. diabetes: Off. journal German Soc. Endocrinol. [and] German Diabetes Assoc. 115, 522–526. doi: 10.1055/s-2007-981457
Berge G. M., Zhou W., Johansen L. H., Chikwati E., Kortner T. M., Lein I., et al. (2023). Effects of dietary lipid level on growth, digestive physiology and disease resistance in lumpfish (Cyclopterus lumpus). Aquaculture 566, 739209. doi: 10.1016/j.aquaculture.2022.739209
Bize P., Devevey G., Monaghan P., Doligez B., Christe P. (2008). Fecundity and survival in relation to resistance to oxidative stress in a free-living bird. Ecology 89, 2584–2593. doi: 10.1890/07-1135.1
Buckley L. J. (1979). Relationships between RNA–DNA ratio, prey density, and growth rate in atlantic cod (Gadus morhua) larvae. J. Fisheries Res. Board Canada 36, 1497–1502. doi: 10.1139/f79-217
Cao X. F., Liu W. B., Zheng X. C., Yuan X. Y., Wang C. C., Jiang G. Z. (2018). Effects of high-fat diets on growth performance, endoplasmic reticulum stress and mitochondrial damage in blunt snout bream Megalobrama amblycephala. Aquaculture Nutr. 25, 97–109. doi: 10.1111/anu.12834
Chang J., Niu H. X., Jia Y. D., Li S. G., Xu G. F. (2018). Effects of dietary lipid levels on growth, feed utilization, digestive tract enzyme activity and lipid deposition of juvenile Manchurian trout, Brachymystax lenok (Pallas). Aquacult Nutr. 24, 694–701. doi: 10.1111/anu.12598
Chatzifotis S., Panagiotidou M., Papaioannou N., Pavlidis M., Nengas I., Mylonas C. C. (2010). Effect of dietary lipid levels on growth, feed utilization, body composition and serum metabolites of meagre (Argyrosomus regius) juveniles. Aquaculture 307, 65–70. doi: 10.1016/j.aquaculture.2010.07.002
Chen C., Tian H. Y., Liu X. B., Dai Y. S., Wen X. B., Zhao H. H., et al. (2024). Effects of dietary lipid levels on growth, lipid metabolism, fatty acid composition and antioxidant capacity of juvenile greasyback shrimp (Metapenaeus ensis). Aquaculture Rep. 36, 102146. doi: 10.1016/j.aqrep.2024.102146
Chen Y. F., Cao K. L., Huang H. F., Li X. Q., Leng X. J. (2023). Dietary effects of lipid and protein levels on growth, feed utilization, lipid metabolism, and antioxidant capacity of triploid rainbow trout (Oncorhynchus mykiss). Aquaculture Nutr. 2023, 1–13. doi: 10.1155/2023/8325440
Clemmesen C. (1994). The effect of food availability, age or size on the RNA/DNA ratio of individually measured herring larvae: laboratory calibration. Mar. Biol. 118, 377–382. doi: 10.1007/BF00350294
Cunningham A. A., Turvey S. T., Zhou F., Meredith H. M. R., Guan W., Liu X., et al. (2015). Development of the Chinese giant salamander Andrias davidianus farming industry in Shaanxi Province, China: conservation threats and opportunities. Oryx 50, 265–273. doi: 10.1017/s0030605314000842
De Carvalho C. C. C. R., Caramujo M. J. (2018). The various roles of fatty acids. Molecules (Basel Switzerland) 23, 2583. doi: 10.3390/molecules23102583
Deng J. M., Zhang X. D., Sun Y., Zhang L., Mi H. F. (2021). Optimal dietary lipid requirement for juvenile Asian red-tailed catfish (Hemibagrus wyckioides): Dietary lipid level for Hemibagrus wyckioides. Aquaculture Rep. 20, 100666. doi: 10.1016/j.aqrep.2021.100666
Du Z. Y., Clouet P., Zheng W. H., Degrace P., Tian L. X., Liu Y. J. (2006). Biochemical hepatic alterations and body lipid composition in the herbivorous grass carp (Ctenopharyngodon idella) fed high-fat diets. Br. J. Nutr. 95, 905–915. doi: 10.1079/bjn20061733
Du Z. Y., Liu Y. J., Tian L. X., Wang J. T., Wang Y., Liang G. Y. (2005). Effect of dietary lipid level on growth, feed utilization and body composition by juvenile grass carp (Ctenopharyngodon idella). Aquaculture Nutr. 11, 139–146. doi: 10.1111/j.1365-2095.2004.00333.x
Ebadi H., Zakeri M., Mousavi S. M., Yavari V., Souri M. (2021). The interaction effects of dietary lipid, vitamin E and vitamin C on growth performance, feed utilization, muscle proximate composition and antioxidant enzyme activity of white leg shrimp (Litopenaeus vannamei). Aquaculture Res. 52, 2048–2060. doi: 10.1111/are.15056
Ghasemi N., Imani A., Noori F., Shahrooz R. (2020). Ontogeny of digestive tract of Stellate sturgeon (Acipenser stellatus) from hatching to juvenile stage: Digestive enzymes activity, stomach and proximal intestine. Aquaculture 519, 734751. doi: 10.1016/j.aquaculture.2019.734751
Gómez-Requeni P., Bedolla-Cázares F., Montecchia C., Zorrilla J., Villian M., Toledo-Cuevas E. M., et al. (2013). Effects of increasing the dietary lipid levels on the growth performance, body composition and digestive enzyme activities of the teleost pejerrey (Odontesthes bonariensis). Aquaculture 416–417, 15–22. doi: 10.1016/j.aquaculture.2013.08.027
Gorini A., Canosi U., Devecchi E., Geroldi D., Villa R. F. (2002). ATPases enzyme activities during ageing in different types of somatic and synaptic plasma membranes from rat frontal cerebral cortex. Prog. Neuropsychopharmacol. Biol. Psychiatry 26, 81–90. doi: 10.1016/s0278-5846(01)00233-0
Guillard J., Schwörer S. (2024). Metabolic control of collagen synthesis. Matrix Biol. 133, 43–56. doi: 10.1016/j.matbio.2024.07.003
Guo Y. H., Ma J. K., Xu H. Z., Li W. L., Liu C. J., Lu H., et al. (2023). Effects of different dietary lipid sources (perilla, fish, and soybean oils) on growth, lipid metabolism, antioxidant, and immune status in Chinese giant salamander (Andrias davidianus). Front. Mar. Sci. 10. doi: 10.3389/fmars.2023.1139651
Guo J. L., Zhou Y. L., Zhao H., Chen W. Y., Chen Y. J., Lin S. M. (2019). Effect of dietary lipid level on growth, lipid metabolism and oxidative status of largemouth bass, Micropterus salmoides. Aquaculture 506, 394–400. doi: 10.1016/j.aquaculture.2019.04.007
Hamidoghli A., Won S., Aya F. A., Yun H., Bae J., Jang I., et al. (2019). Dietary lipid requirement of whiteleg shrimp Litopenaeus vannamei juveniles cultured in biofloc system. Aquaculture Nutr. 26, 603–612. doi: 10.1111/anu.13021
Han T., Li X., Wang J., Hu S., Jiang Y., Zhong X. (2014). Effect of dietary lipid level on growth, feed utilization and body composition of juvenile giant croaker Nibea japonica. Aquaculture 434, 145–150. doi: 10.1016/j.aquaculture.2014.08.012
Hansen J. V., Berge G. M., Hillestad M., Krogdahl S., Galloway T. F., Holm H., et al. (2008). Apparent digestion and apparent retention of lipid and fatty acids in Atlantic cod (Gadus morhua) fed increasing dietary lipid levels. Aquaculture 284, 159–166. doi: 10.1016/j.aquaculture.2008.07.043
He D., Zhu W. M., Zeng W. M., Lin J., Ji Y., Wang Y., et al. (2018). Nutritional and medicinal characteristics of Chinese giant salamander (Andrias davidianus) for applications in healthcare industry by artificial cultivation: A review. Food Sci. Hum. Wellness 7, 1–10. doi: 10.1016/j.fshw.2018.03.001
Hung L. T., Binh V. T. T., Thanh Truc N. T., Tham L. H., Ngoc Tran T. (2017). Effects of dietary protein and lipid levels on growth, feed utilization and body composition in red-tailed catfish juveniles (Hemibagrus wyckioides, Chaux & Fang 1949). Aquaculture Nutr. 23, 367–374. doi: 10.1111/anu.12401
Karavia E. A., Papachristou D. J., Kotsikogianni I., Triantafyllidou I. E., Kypreos K. E. (2013). Lecithin/cholesterol acyltransferase modulates diet-induced hepatic deposition of triglycerides in mice. J. Nutr. Biochem. 24, 567–577. doi: 10.1016/j.jnutbio.2012.02.007
Kopecka-Pilarczyk J. (2010). Effect of exposure to polycyclic aromatic hydrocarbons on Na +–K +-ATPase in juvenile gilthead seabream Sparus aurata. J. Fish Biol. 76, 716–722. doi: 10.1111/j.1095-8649.2009.02503.x
Krogdahl S., Sundby A., Holm H. (2015). Characteristics of digestive processes in Atlantic salmon (Salmo salar). Enzyme pH optima, chyme pH, and enzyme activities. Aquaculture 449, 27–36. doi: 10.1016/j.aquaculture.2015.02.032
Lee S., Katya K., Park Y., Won S., Seong M., Hamidoghli A., et al. (2017). Comparative evaluation of dietary probiotics Bacillus subtilis WB60 and Lactobacillus plantarum KCTC3928 on the growth performance, immunological parameters, gut morphology and disease resistance in Japanese eel, Anguilla japonica. Fish Shellfish Immunol. 61, 201–210. doi: 10.1016/j.fsi.2016.12.035
Li Y., Li W. T., Luo L., Ren Y. Y., Xing W., Xu G. L., et al. (2023b). Dietary lipid levels affect growth performance, lipid metabolism, antioxidant and immune status of Amur sturgeon, Acipenser schrenckii. Aquaculture Rep. 33, 101796. doi: 10.1016/j.aqrep.2023.101796
Li P. Y., Song Z. D., Huang L., Sun Y. Z., Sun Y. M., Wang X. Y., et al. (2023a). Effects of dietary protein and lipid levels in practical formulation on growth, feed utilization, body composition, and serum biochemical parameters of growing rockfish sebastes schlegeli. Aquaculture Nutr. 2023, 9970252. doi: 10.1155/2023/9970252
Lin Y., Yeh C. (2022). Effects of dietary lipid levels on growth, lipid deposition, oxidative stress and hepatic morphological changes in giant grouper, Epinephelus lanceolatus. Aquaculture Res. 53, 2431–2438. doi: 10.1111/are.15760
Liou C. H., To V. A., Zhang Z. F., Lin Y. H. (2023). The effect of dietary lecithin and lipid levels on the growth performance, body composition, hemolymph parameters, immune responses, body texture, and gene expression of juvenile white shrimp (Litopenaeus vannamei). Aquaculture 567, 739260. doi: 10.1016/j.aquaculture.2023.739260
López L. M., Durazo E., Viana M. T., Drawbridge M., Bureau D. P. (2009). Effect of dietary lipid levels on performance, body composition and fatty acid profile of juvenile white seabass, Atractoscion nobilis. Aquaculture 289, 101–105. doi: 10.1016/j.aquaculture.2009.01.003
Lu H., Li Z. F., Huang X. Z., Li H., Zhai X. L., Xue Y., et al. (2021). Effects of mulberry leaf extract and 1-deoxynojirimycin on growth performance, liver function and immune ability of giant salamander (Andrias davidianus). Chin. J. Anim. Nutr. 33, 584–593. doi: 10.3969/j.issn.1006-267x.2021.01.059
Lu K. L., Xu W. N., Li X. F., Liu W. B., Wang L. N., Zhang C. N. (2013). Hepatic triacylglycerol secretion, lipid transport and tissue lipid uptake in blunt snout bream (Megalobrama amblycephala) fed high-fat diet. Aquaculture 408-409, 160–168. doi: 10.1016/j.aquaculture.2013.06.003
Mas-Bargues C., Escrivá C., Dromant M., Borrás C., Viña J. (2021). Lipid peroxidation as measured by chromatographic determination of malondialdehyde. Human plasma reference values in health and disease. Arch. Biochem. biophysics 709, 108941. doi: 10.1016/j.abb.2021.108941
Mata-Sotres J. A., Flores-Salas C., Skrzynska A. K., Tinajero A., Araújo B. C., Viana M. T. (2022). Lipid metabolism in juvenile of Yellowtail, Seriola dorsalis fed diets containing different lipid levels. Aquaculture 550, 737870. doi: 10.1016/j.aquaculture.2021.737870
Meng Y. Q., Qian K. K., Ma R., Liu X. H., Han B. Y., Wu J., et al. (2019). Effects of dietary lipid levels on sub-adult triploid rainbow trout (Oncorhynchus mykiss): 1. Growth performance, digestive ability, health status and expression of growth-related genes. Aquaculture 513, 734394. doi: 10.1016/j.aquaculture.2019.734394
Meng X. R., Zhang W. P., Zhang Y. P. (2006). The effect of specific aliphatic acid to the collagen coagulation of the habbit’s scar. Chin. Med. Factory Mine 19, 3. doi: 10.3969/j.issn.1674-8182.2006.02.007
Mohanta K., Mohanty S., Jena J., Sahu N. (2008). Optimal dietary lipid level of silver barb, Puntius gonionotus fingerlings in relation to growth, nutrient retention and digestibility, muscle nucleic acid content and digestive enzyme activity. Aquaculture Nutr. 14, 350–359. doi: 10.1111/j.1365-2095.2007.00542.x
Morais S., Cahu C., Zambonino-Infante J. L., Robin J., Rønnestad I., Dinis M. T., et al. (2004). Dietary TAG source and level affect performance and lipase expression in larval sea bass (Dicentrarchus labrax). Lipids 39, 449–458. doi: 10.1007/s11745-004-1250-2
Murray P. J., Wynn T. A. (2011). Protective and pathogenic functions of macrophage subsets. Nat. Rev. Immunol. 11, 723–737. doi: 10.1038/nri3073
Naiel M. A. E., Negm S. S., Ghazanfar S., Shukry M., Abdelnour S. A. (2023). The risk assessment of high-fat diet in farmed fish and its mitigation approaches: A review. J. Anim. Physiol. Anim. Nutr. 107, 948–969. doi: 10.1111/jpn.13759
NRC (2011). “Lipids,” in Nutrient requirements of fish and shrimp (The National Academies Press, Washington DC), 102–134. doi: 10.1007/s10499-011-9480-6
Paital B., Chainy G. B. N. (2010). Antioxidant defenses and oxidative stress parameters in tissues of mud crab (Scylla serrata) with reference to changing salinity. Comp. Biochem. Physiol. Part C: Toxicol. Pharmacol. 151, 142–151. doi: 10.1016/j.cbpc.2009.09.007
Papáčková Z., Cahová M. (2014). Important role of autophagy in regulation of metabolic processes in health, disease and aging. Physiol. Res. 63, 409–420. doi: 10.33549/physiolres.932684
Perez-Velazquez M., Minjarez-Osorio C., González-Félix M. L. (2017). Effect of dietary lipid level on growth performance, feed utilization and body composition of totoaba, Totoaba macdonaldi (Gilbert 1890). Aquac Res. 48, 2607–2617. doi: 10.1111/are.13002
Picolo V. L., Quadros V. A., Canzian J., Grisolia C. K., Goulart J. T., Pantoja C., et al. (2021). Short-term high-fat diet induces cognitive decline, aggression, and anxiety-like behavior in adult zebrafish. Prog. Neuropsychopharmacol. Biol. Psychiatry 110, 0278–5846. doi: 10.1016/j.pnpbp.2021.110288
Qiang J., He J., Yang H., Sun Y. L., Tao Y. F., Xu P., et al. (2017). Dietary lipid requirements of larval genetically improved farmed tilapia, Oreochromis niloticus (L.), and effects on growth performance, expression of digestive enzyme genes, and immune response. Aquac Res. 48, 2827–2840. doi: 10.1111/are.13117
Ragab S. M. M., Elghaffar S. K. A., El-Metwally T. H., Badr G., Mahmoud M. H., Omar H. M. (2015). Effect of a high fat, high sucrose diet on the promotion of non-alcoholic fatty liver disease in male rats: the ameliorative role of three natural compounds. Lipids Health Dis. 14, 83. doi: 10.1186/s12944-015-0087-1
Ramos M. A., Batista S., Pires M. A., Silva A. P., Pereira L. F., Saavedra M. J., et al. (2017). Dietary probiotic supplementation improves growth and the intestinal morphology of Nile tilapia. Animal: an Int. J. Anim. bioscience 11, 1259–1269. doi: 10.1017/S1751731116002792
Schieber M., Chandel N. S. (2014). ROS function in redox signaling and oxidative stress. Curr. Biol. 24, R453–R462. doi: 10.1016/j.cub.2014.03.034
Sesay D. F., Habte-Tsion H. M., Zhou Q. L., Ren M. C., Xie J., Liu B., et al. (2016). Effects of dietary folic acid on the growth, digestive enzyme activity, immune response and antioxidant enzyme activity of blunt snout bream (Megalobrama amblycephala) fingerling. Aquaculture 452, 142–150. doi: 10.1016/j.aquaculture.2015.10.026
Shearer G. C., Savinova O. V., Harris W. S. (2012). Fish oil -how does it reduce plasma triglycerides? Biochim. Biophys. Acta 1821, 843–851. doi: 10.1016/j.bbalip.2011.10.011
Shi Z. H., Yue Y. F., Peng S. M., Li Y. H., Sun P., Yin F., et al. (2013). Effects of dietary lipid levels on serum biochemistry indices, immunity, and antioxidant activity in Sebastiscus marmoratus. J. Fish. Sci. China 20 (1), 101–107. doi: 10.3724/SP.J.1118.2013.00101
Shoulders M. D., Raines R. T. (2009). Collagen structure and stability. Annu. Rev. Biochem. 78, 929–958. doi: 10.1146/annurev.biochem.77.032207.120833
Valko M., Izakovic M., Mazur M., Rhodes C. J., Telser J. (2004). Role of oxygen radicals in DNA damage and cancer incidence. Mol. Cell. Biochem. 266, 37–56. doi: 10.1023/B:MCBI.0000049134.69131.89
Van den Bossche J., O’Neill L., Menon D. (2017). Macrophage immunometabolism: where are we (Going)? Trends Immunol. 38, 395–406. doi: 10.1016/j.it.2017.03.001
Wang A. Y., Han B., Song L. P., Zhang Y. H., Pan X. L., Hu B., et al. (2010). Effects of dietary lipids on the serum biochemical indices of sooty grunter. J. Zhejiang Ocean University: Natural Science. 29, 222–226.
Wang S., Li Z. F., Li H., Li X. L., Lei D. H., Zhu C. K., et al. (2020). Dietary protein requirement of juvenile giant salamander (Andrias davidianus). J. fisheries China 44, 99–110. doi: 10.11964/jfc.20181111533
Wang W. B., Liu P., Dou L. L., Ping W., Ning Y., Xi R. M., et al. (2021). A survey of Chinese giant salamander (Andrias davidianus). Acta hydrobiologica Sin. 45, 464–472. doi: 10.7541/2021.2020.184
Wang X. M., Zhang K. J., Wang Z. H., Ding Y. Z., Wu W., Huang S. (2004). The decline of the Chinese giant salamander Andrias davidianus and implications for its conservation. Oryx 38, 197–202. doi: 10.1017/S0030605304000341
Xie S. W., Lin Y. Y., Wu T., Tian L. X., Liang J. J., Tan B. (2021). Dietary lipid levels affected growth performance, lipid accumulation, inflammatory response and apoptosis of Japanese seabass (lateolabraxjaponicus). Aquaculture Nutr. 27, 807–816. doi: 10.1111/anu.13225
Xu H. Z., Li W. L., Li L., Chen Y. J., Zhai X. L., Xue Y., et al. (2021). Perilla leaves can promote the growth and some physiological functions of chinese giant salamander (Andrias davidianus). Acta Hydrobiologica Sin. 45, 774–780. doi: 10.7541/2021.2020.088
Yan J., Liao K., Wang T. J., Mai K. S., Xu W., Ai Q. H. (2015). Dietary Lipid Levels Influence Lipid Deposition in the Liver of Large Yellow Croaker (Larimichthys crocea) by Regulating Lipoprotein Receptors, Fatty Acid Uptake and Triacylglycerol Synthesis and Catabolism at the Transcriptional Level. PloS One 10, e0129937. doi: 10.1371/journal.pone.0129937
Yin P., Xie S., Zhuang Z., He X., Tang X., Tian L., et al. (2020). Dietary supplementation of bile acid attenuates adverse effects of high-fat diet on growth performance, antioxidant ability, lipid accumulation and intestinal health in juvenile largemouth bass (Micropterus salmoides). Aquaculture 531, 735864. doi: 10.1016/j.aquaculture.2020.735864
Zhang K. J., Wang X. M., Wu W., Wang Z. H., Huang S. (2002). Advances in conservation biology of Chinese giant salamander. Biodiversity Sci. 10, 291–297. doi: 10.17520/biods.2002040
Zhao W. L., Luo J. X., Fang F., Zhu T. T., Xie S. C., Yang Z., et al. (2023). Differential regulatory effects of dietary lipid level on growth, antioxidant capacity and expression of genes involved in lipid and glucose metabolism of juvenile mud crab Scylla paramamosain. Aquaculture Rep. 29, 101520. doi: 10.1016/j.aqrep.2023.101520
Keywords: Andrias davidianus, lipid level, antioxidant capacity, growth performance, liver
Citation: Yan X, Pan L, Yu J, Wang S, Li Y, Zhao M, Zhai X, Xue Y and Luo L (2024) Effects of dietary lipid levels on growth, antioxidant capacity, intestinal and liver structure of juvenile giant salamander (Andrias davidianus). Front. Mar. Sci. 11:1515014. doi: 10.3389/fmars.2024.1515014
Received: 22 October 2024; Accepted: 25 November 2024;
Published: 20 December 2024.
Edited by:
Christian Larbi Ayisi, University of Environment and Sustainable Development, GhanaReviewed by:
Amit Ranjan, Tamil Nadu Fisheries University, IndiaXianyong Bu, Ocean University of China, China
Copyright © 2024 Yan, Pan, Yu, Wang, Li, Zhao, Zhai, Xue and Luo. This is an open-access article distributed under the terms of the Creative Commons Attribution License (CC BY). The use, distribution or reproduction in other forums is permitted, provided the original author(s) and the copyright owner(s) are credited and that the original publication in this journal is cited, in accordance with accepted academic practice. No use, distribution or reproduction is permitted which does not comply with these terms.
*Correspondence: Li Luo, bHVvbGkxOTcyQDE2My5jb20=