- Institute for Chemistry and Biology of the Marine Environment (ICBM), School of Mathematics and Science, Carl von Ossietzky Universität Oldenburg, Oldenburg, Germany
In subterranean estuaries (STE), fresh and saline groundwater introduce dissolved organic matter (DOM) of different origin. This DOM serves as substrate for microorganisms that thrive in the STE. In high-energy beaches with dynamic porewater advection, microbial communities face frequent changes in groundwater composition, even at several meters depth. It is unknown how DOM from deep STE groundwater (> 5 m depth) is transformed by prevailing microbial communities. To address this question, we performed sediment incubations in flow-through reactors (FTRs) with deep (6 m depth) STE groundwater of low (1.6) and high salinity (29.1). FTR setups were sampled daily for quantification of dissolved organic carbon (DOC), and at start and end (day 13) of the incubation for analysis of DOM composition, microbial cell numbers and community composition. Solid-phase extracted DOM was molecularly characterized via ultrahigh-resolution Fourier-transform ion cyclotron resonance mass spectrometry. Both groundwater types contained mainly reworked DOM. Corroborating its presumed origin, the fresh groundwater had a more terrestrial DOM signature with a higher proportion of aromatic compounds compared to the saline groundwater. Over the course of the incubation, DOC concentrations increased primarily due to leaching of sedimentary organic matter, providing an additional source of DOM. In all setups, the DOM composition changed significantly from start to end, and similarly for fresh and saline groundwater. From the ~2700 molecular formulae (MF) detected on day 0, 34-35% were removed during the incubations, demonstrating the potential of deep STE microbial communities to degrade recalcitrant DOM that is supplied with the advective porewater flow. However, a substantial portion of MF (63-64%) was retained in both groundwater types, indicating that a fraction of deep STE-DOM is resistant to removal. Properties of MF that were newly detected on day 13 (26-28%) were indicative of labile DOM. Some of these newly detected MF were also identified in sediment-leachates, suggesting that beach sediments are a source of fresh DOM for the STE microbial communities. It is likely that due to longer groundwater residence time in the STE, continuous leaching and microbial processing shift the molecular composition of released DOM from more labile to more recalcitrant DOM.
1 Introduction
Subterranean estuaries (STEs) are underground mixing zones of fresh groundwater and recirculating seawater in coastal aquifers (Moore, 1999). In contrast to surface estuaries, STEs lack light for photosynthesis and mobile groundwater moves through a static sediment phase. Groundwater flow follows a hydraulic gradient, and flow paths are often influenced by dynamic boundary conditions, such as tides, waves, storm floods, and changing beach morphology (Santos et al., 2012; Robinson et al., 2018; Greskowiak and Massmann, 2021). The water entering the beach aquifer continuously supplies new organic substrates and electron acceptors to the prevailing microbial communities, making STEs active biogeochemical reactors (Anschutz et al., 2009; Kim et al., 2019).
STEs play an essential role in the transformation and degradation of dissolved organic matter (DOM) from both, terrestrial and marine sources (Kim et al., 2012; Seidel et al., 2014; Waska et al., 2021; Adyasari et al., 2023). Since the particulate organic matter (POM) content in beach sediments is generally very low <0.03–0.04% in organic carbon (Beck et al., 2017; Seibert et al., 2019), microbial communities in beach STEs depend on the continuous supply of DOM from the infiltrating seawater and fresh groundwater (Kim et al., 2019; Seidel et al., 2014; Streif, 2002; Waska et al., 2021). Seawater entering the STE transports DOM primarily of marine origin, with a significant portion recently produced by phytoplankton photosynthesis (Thornton, 2014). This marine-derived DOM is generally considered more labile than the aged terrigenous DOM originating from land plants and soils, which is transported by fresh groundwater (Seidel et al., 2015). These different DOM sources serve as carbon and energy supply for STE microbial communities, influencing their composition, diversity, and metabolic activity (Azam et al., 1983; Fuhrman et al., 2015). Microbial communities completely degrade part of the DOM, establishing an important sink for reduced carbon, while they also produce new molecules (Kujawinski et al., 2004; Kim et al., 2012; Koch et al., 2014; Valle et al., 2018) and progressively transform labile into more refractory DOM (Jiao et al., 2011; Dittmar et al., 2021). Thus, microbial activity modifies the bulk properties of DOM and exerts strong influence on the nature of STE-DOM.
Understanding DOM processing in beach STEs is crucial to establish carbon budgets and to assess the effect of submarine groundwater discharge on the coastal ocean. In general, studies conducted on STEs of high-energy beaches that are affected by strong waves (mean significant wave height >1.5m) and high tidal amplitudes (mean tidal range >3.5m) are scarce. Research has mostly focused on low-energy STEs with more stable groundwater flow paths and well-defined reaction zones (Michael et al., 2005; Robinson et al., 2006, 2007a, 2014), and the few studies on high-energy beaches are mostly restricted to the island of Spiekeroog (Beck et al., 2017; Linkhorst et al., 2017; Waska et al., 2019a; Degenhardt et al., 2021; Waska et al., 2021). However, no study has been extended to the deep (>5 m) STEs of high-energy beaches, likely because of the challenges associated with working in such environments (Massmann et al., 2023). Recent research on hydrogeochemical processes (Grünenbaum et al., 2023; Waska et al., 2019b) and numerical groundwater flow and transport models indicate that subsurface flow paths in STEs below high-energy beaches can be heterogeneous. These flow paths extend to tens of meters deep in the STE due to changing boundary conditions related to morpho- and hydrodynamics, leading to strong temporal and spatial variability in biogeochemical conditions even in the deep STE (> 5 m depth) (Greskowiak and Massmann, 2021; Massmann et al., 2023). However, the influence of this heterogeneous groundwater flow patterns in the deep STE on the distribution of DOM and its processing by microbial communities have so far not been explored. Such knowledge is important for delineating the routes of DOM degradation and transformation and, thus, for improving our understanding of the mechanisms behind organic matter cycling in the deep biosphere of the land-sea interface.
In this study, we addressed the question how different DOM substrates from the deep STE are processed by microbial communities. We performed laboratory incubation experiments with sediment and deep groundwater (6 m) from the STE of a mesotidal high-energy beach on Spiekeroog Island (Germany). This island has a freshwater lens formed by the accumulation of meteoric water within the permeable sandy aquifer underlying the island (Seibert et al., 2018). Spiekeroog experiences semi-diurnal tides with a mean tidal range of 2.7 m and high-energy waves of 0.5–2.0 m (Dobrynin et al., 2010), leading to significant tidal oscillations in the coastal aquifer. Tidal pumping drives the infiltration of seawater during high tides and the discharge of fresh groundwater during low tides, creating a dynamic STE within the beach aquifer (Robinson et al., 2007b). The mixing of oxic seawater and anoxic fresh groundwater can induce strong redox gradients within STEs. Recent numerical models indicated that redox dynamics in STEs may occur down to a depth of >10 m, influenced largely by hydrodynamics and morphodynamics (Greskowiak et al., 2023). However, the effects of these changing conditions on biogeochemical reactions have not been explored yet. The study beach site on Spiekeroog Island is temporally equipped with observation wells to extract porewater for biogeochemical studies by the interdisciplinary research unit “Dynamic Deep Subsurface of High-energy Beaches (DynaDeep)” (Massmann et al., 2023). Three multilevel (ML) groundwater wells were installed landward close to the dunes (ML1), seaward at the high-water line (ML2), and close to the low-water line (ML3). Each of these wells has four depths: 6, 12, 18, and 24 m, with 1 m long screens ranging from 5-6, 11-12, 17-18, and 23-24 m depth, respectively, below ground surface at the time of installation (Massmann et al., 2023).
Intertidal beach sediments were sampled and incubated for 13 days in flow-through reactors (FTRs) with groundwater of low salinity (1.6) retrieved from 6 m at ML1 as terrestrial endmember and groundwater of high salinity (29.1) taken at 6 m at ML2 as marine endmember. We hypothesized that (1) fresh and saline groundwater from deep STE differ in molecular DOM composition, and (2) microbial communities process the DOM of fresh and saline groundwater differently.
2 Materials and methods
2.1 Sediment and groundwater sampling from the northern beach of Spiekeroog Island
Sampling was carried out on the north-facing beach of Spiekeroog Island in March 2023 during a period of mostly fair weather and no rain. Beach sediments were collected from the upper 20 cm near the high-water line, approximately 2 m from ML2. A total of twelve liters of unfiltered groundwater with low salinity (1.6) as terrestrial endmember and high salinity (29.1) as marine endmember were retrieved from 6 m depths at ML1 and ML2, respectively, using a submersible pump (Eijkelkamp, Tauchpumpe “Gigant”) and stored in 5 L high-density polyethylene (HDPE; acid-rinsed) canisters. During sampling, dissolved oxygen concentration (O2) and salinity of the groundwater was measured in a flow-through cell connected to the sampling tube and a WTW 3630 IDS multi-sensor device to avoid exposure to O2 during sampling. The dissolved O2 was 6.0 mg/L and 11.0 mg/L in the low and high salinity groundwater respectively, indicating oxic groundwater conditions at the time of sampling. All groundwater and sediment samples were immediately transported to the laboratory at the Institute for Chemistry and Biology of the Marine Environment (ICBM) and stored at 4°C in the dark until setup of FTRs.
2.2 Experimental setup
2.2.1 Flow-through reactors
The sediment was homogenized and 550 ml each transferred into FTRs with an inner diameter of 9 cm and a filled core length of 12 cm. Three sediment-filled FTRs were each flushed with two liters of groundwater of low salinity (1.6) from ML1, another three FTRs with high salinity groundwater (29.1) from ML2. Additionally, control FTRs with artificial seawater (ASW) of respective groundwater salinities as fresh and saline groundwater control (FG-C and SG-C) were included, making up a total of eight FTRs (Supplementary Figure S1). The ASW stock was prepared with a modified recipe of Zech et al. (2009) containing NaCl (24 g L-1), KCl (0.5 g L-1), Na2SO4 (4.8 g L-1), NaHCO3 (0.19 g L-1), MgCl2*6H20 (3 g L-1), NH4Cl (0.25 g L-1) and CaCl2*2H20 (0.15 g L-1) in ultra-pure water, and salinities of 29.1 and 1.6 were prepared by respective dilutions with ultrapure water. The first three salts were combusted (450°C for 4 h) before use to reduce the DOC background of the ASW. The FTRs were positioned vertically and continuously supplied with air-saturated groundwater from the HDPE reservoirs using polypropylene tubing. Advective pore water flow was maintained using a peristaltic pump (ISMATEC IPC4), establishing a flow rate of 25 ml/min. The FTR incubations were conducted under room light and temperature (20°C), and under fully oxic conditions (3 L air space in the reservoir). To monitor dissolved O2 concentration, optodes connected to a Fibox (Fibox3 and oxygen sensors type PSt3 by PreSens) were mounted in the outflow tubing.
2.2.2 Sediment leaching experiment
Five ml of the homogenized beach sand were mixed (by gently shaking the bottle) with 15 ml ASW with the same salinities used in the FTRs (salinity 1.6 and 29.1; four replicates per setup). The contact time between ASW and sediment was 1 h. The supernatant (14 ml) from each replicate was carefully siphoned with clean tubing attached to a syringe (acid washed) and then filtered through pre-cleaned (by thorough rinsing with ultrapure water) 0.2 µm PTFE filters into 30 ml HDPE bottles. The sample was then acidified to pH 2 using HCl (analytical grade) and stored at 4°C in the dark until further analysis.
2.3 Quantification and molecular characterization of DOM
2.3.1 Sampling and quantification of DOC
Water samples (15 ml) for quantification of dissolved organic carbon (DOC) concentrations in the FTRs were taken daily from the reservoir (Supplementary Figure S1). For molecular characterization of DOM, 50 ml of water was collected from the start (day 0) and end (day 13) of the incubation. The DOC and DOM samples were immediately filtered through pre-combusted (400°C, 4 h) glass microfiber (GMF, 2 µm) and glass fiber filters (GF/F, 0.7 µm) into HDPE bottles, acidified to pH 2 with HCl, and stored in the dark at 4°C until further analysis.
Due to the limited volume of the samples from the leaching experiment, aliquots of 4 ml were diluted with 6 ml ultra-pure water (acidified to pH 2 with HCl) to reach the optimum volume required for DOC analysis. The remaining 10 ml sample was diluted to 30 ml with ultra-pure water (acidified to pH 2 with HCl) to ensure maximum extraction efficiency for DOM analysis.
The DOC and TDN concentrations of all samples were quantified using high-temperature catalytic oxidation on a Shimadzu TOC-VCPH. The quality of the analyses was tested against measured concentrations of the deep-sea Atlantic reference material (DSR; provided by D.A Hansell, University of Miami, FL, USA) with the accepted trueness and precision better than 5%.
2.3.2 Molecular characterization of DOM via Fourier transform ion cyclotron resonance mass spectrometry
All samples were solid-phase extracted (SPE) using styrene divinyl benzene polymer-filled cartridges (Agilent Bond Elut PPL, 100 mg) following the procedure described by Dittmar et al. (2008). Extraction volumes were 50 ml for the FTR samples, 30 ml for the diluted sediment leachates, and respective volumes for one procedural blank (ultrapure water acidified to pH 2) each. After the extraction, the cartridges were rinsed with two cartridge volumes of ultrapure water (pH 2) to remove remaining salts and then dried with nitrogen gas. SPE-DOM was immediately eluted with 1.2 ml of methanol (HPLC-grade) into pre-combusted amber vials and stored in the dark at -20°C until further analysis.
For analysis on the FT-ICR-MS, the carbon concentration of all DOM extracts was adjusted to approximate 2.5 ppm DOC (1:1 mixture of methanol (MS grade) and ultrapure water) for optimal resolution of DOM compounds. Thereafter, all DOM extracts were filtered through 0.2 µm polytetrafluoroethylene polymer (PTFE) filters and analyzed in negative electrospray ionization mode (ESI) on a SolariX FT-ICR-MS (Bruker Daltonik GmbH, Germany) equipped with a 15 Tesla superconducting magnet. The instrument settings and raw data processing were as described by Seidel et al. (2014). The reproducibility of the peak detection and stability of the FT-ICR-MS procedure were controlled by duplicate measurements of each sample, including eight measurements of the deep-sea DOM reference extracted from North Equatorial Pacific Intermediate Water (NEqPIW; https://uol.de/en/icbm/dsr-dom). Procedural blanks (SPE-DOM of ultrapure water acidified to pH 2) were included for quality control of sample processing. Two hundred scans were accumulated for each mass spectrum. The acquired mass spectra were internally calibrated against a NEqPIW-based mass list of confirmed molecular formulae with an error of <0.1 ppm. The calibrated mass spectra were processed using the ICBM-OCEAN free processing tool to assign MF (Merder et al., 2020) and the identified MF were in the mass range between 100 and 800 Da (Supplementary Text S1).
We assembled two datasets for DOM molecular composition. Dataset 1 for the FTR incubation experiment and dataset 2 for the sediment leaching experiment. All data processing described below and in Supplementary Text S1 was performed on each of the two datasets individually because of the different sample processing before and during FT-ICR-MS analysis.
Following the assignment of MF, the mass spectra of each sample were reconstructed and visually inspected. All mass peaks in the spectra considered for further analysis exhibited a Gaussian-like distribution. This approach was used to achieve comparable mass spectra. Blank corrections were performed by removing MF identified in procedural blanks that were higher in intensity than in any sample. MF detected in only a single sample were not considered because they were not reproducibly detected. Isotopologues containing 13C, 15N, and 18O were not further considered as the respective compounds were represented by the monoisotopic MF. Peak intensities of all MF in a sample were then normalized against the sum of the respective sample’s peak intensities. For each sample, we calculated intensity-weighted averages of the modified aromaticity index (AImod; Koch and Dittmar, 2006, 2016), molar ratios (H/C, O/C), and the relative abundance of compound groups (highly unsaturated, unsaturated, saturated, aromatic) as defined in Merder et al. (2020), based on the distribution of relative signal intensities. The identified MF were also grouped according to their heteroatom (N, S, P) content. In addition, we applied four DOM molecular indices: (1) The degradation index (“IDEG”) as an indicator for the level of organic matter degradation (Flerus et al., 2012), (2) the bioformation and transformation index (“Ibio”) as a measure for fresh DOM from recent biological activity (Bercovici et al., 2023b), (3) the DOM molecular lability boundary (“MLB”) as an elemental ratio based measure for DOM lability (D’Andrilli et al., 2015), and (4) the molecular formulae based measure for terrigenous input (“ITerr”; Medeiros et al., 2016).
2.4 Microbial abundance and community composition
2.4.1 Microbial cell counts
Water (48 ml) and sediment (0.5 cm3) samples for total cell counts were taken at start and end of the FTR incubation. The water samples were immediately mixed with 2 ml glutardialdehyde (GDA, 25%) and the sediment samples were fixed in 1 ml GDA (3%). After 30 minutes incubation at 4°C, the samples were stored at -20°C until further processing. For microbial cell counts, 30-50 ml of the fixed groundwater samples were filtered on black PCTE-filters (Ø 25 mm, Whatman) without further treatment. The sediment samples were processed and stained with SybrGreen as described in Lunau et al. (2005). For each sample, a minimum of 300 cells (10-20 view fields) were counted by epifluorescence microscopy (Leica, Leitz DM R).
2.4.2 DNA-extraction, amplification of 16S rRNA genes, sequencing and bioinformatics
Groundwater (250 ml) and sediment (3 ml) samples for DNA-extraction were taken at start and end of the incubation. For the analysis of groundwater communities, the water samples were filtered sequentially using polycarbonate filters (Ø 47 mm, Whatman) with pore sizes of 3 µm and 0.1 µm, and the 0.1 µm filter was used for DNA extraction. For the analysis of sediment-associated communities, DNA was extracted from 0.5 g of sediment. DNA extraction followed a modified protocol of Lueders et al. (2004) and Gabor et al. (2003). To amplify the 16S rRNA gene, primers for sequencing the V4 and V5 region were used with forward 515F-Y (5’-GTG YCA GCM GCC GCG GTA A); reverse 926R (5’-CCG YCA ATT YMT TTR AGT TT) (Parada et al., 2016). Prior to DNA amplification, 2 µl of 1:10 diluted target DNA were mixed with 5 µl Phusion-HF-buffer (5x), 2 µl dNTPs (2.5 mM), 0.75 µl of each primer (10 µM) V4f and V5r, 0.5 µl MgCl2 (50 mM), 0.25 µl Phusion-taq polymerase (2U/µl) and 13.75 µl PCR-water. DNA amplification was performed on a Mastercycler nexus X2 (Eppendorf, Wesseling-Berzdorf, Germany) including following steps: activation at 98°C for 3 min, 25 cycles comprising denaturation at 98°C for 45 seconds, annealing at 50°C for 45 seconds and elongation at 72°C for 90 seconds. The final elongation step was performed at 72°C for 5 minutes. Sequencing was performed with the Illumina NextSeq 1000 sequencing system. The generated 16S rRNA data were processed as described by Tebbe et al. (2022) cutting the forward sequence at 240 bp and the reverse sequence at 200 bp. For taxonomic classification, the database SILVA138 was used. Sequences that were related to mitochondria and chloroplasts were omitted in the subsequent analyses. Additionally, all samples with amplicon sequence variant abundance of more than 0.001% in the complete dataset or a ratio of more than 1% in at least one sample were excluded, whereas, those that had a ratio of more than 0.1% in 2% of the samples or were present in 5% of all samples in any abundance were kept (Milici et al., 2016). The sequences were deposited at the European Nucleotide Archive under the accession number PRJEB80523. For further analyses, read abundances were converted to relative abundances. Statistical analyses were performed in R v. 2022.07.0 (R Core Team, 2022) using the “vegan” package. Non-metric dimensional scaling (NMDS) (k = 2, 999 permutations) was used to visualize Bray-Curtis distances of the microbial abundance distribution in the sediment and groundwater samples. The analysis of similarity (ANOSIM) with 9999 permutations was performed to assess statistical significance regarding the dissimilarity of these samples.
3 Results and discussion
3.1 DOM release during sediment incubations
Prior to incubation, the fresh groundwater (FG) retrieved from 6 m depth close to the dunes had a lower DOC concentration (64 ± 1.2 µmol/L, n=3) than the saline groundwater (SG) from 6 m depth close to the high-water line (98 ± 3.7 µmol/L, n=3). Higher DOC concentrations of SG compared to FG have also been reported for the shallow STE of Spiekeroog North beach (Beck et al., 2017). It is remarkable that both groundwater samples had lower DOC concentrations than those previously reported for the shallow STE, for seawater from the adjacent North Sea, and for groundwater in the island’s freshwater lens (Beck et al., 2017; Waska et al., 2021). This low DOC concentration in STE groundwater is in line with previous suggestions that the Spiekeroog beach STE is a net sink for dissolved organic matter (Waska et al., 2021; Massmann et al., 2023).
During the 13 days of FTR incubation, DOC concentrations increased in both groundwater types (Figure 1). DOC concentrations increased right after the start of the incubations from 64 µmol/L (day 0) to an average of 172 ± 36 µmol/L after day 6 for FG, and from 98 µmol/L (day 0) to an average of 271 ± 15 µmol/L after day 6 for SG. DOC concentrations also increased in the experimental control setups from initial levels of 24 µmol/L to an average of 196 ± 10 µmol/L after day 6 for FG-C, and from 32 µmol/L to an average of 293 ± 21 µmol/L after day 6 for SG-C. This increase in the DOC concentration in both FG and SG, as well as in the ASW controls, indicates an additional DOM source in the FTRs. We did not observe typical contamination signatures, e.g., from softeners, in the DOM spectra. We can also rule out potential DOC contributions from dark carbon fixation because our system was completely oxic (dissolved O2 ranged between 5 and 9 mg/L in all setups) during incubation. The most likely source of additional DOM is release from the sediments.
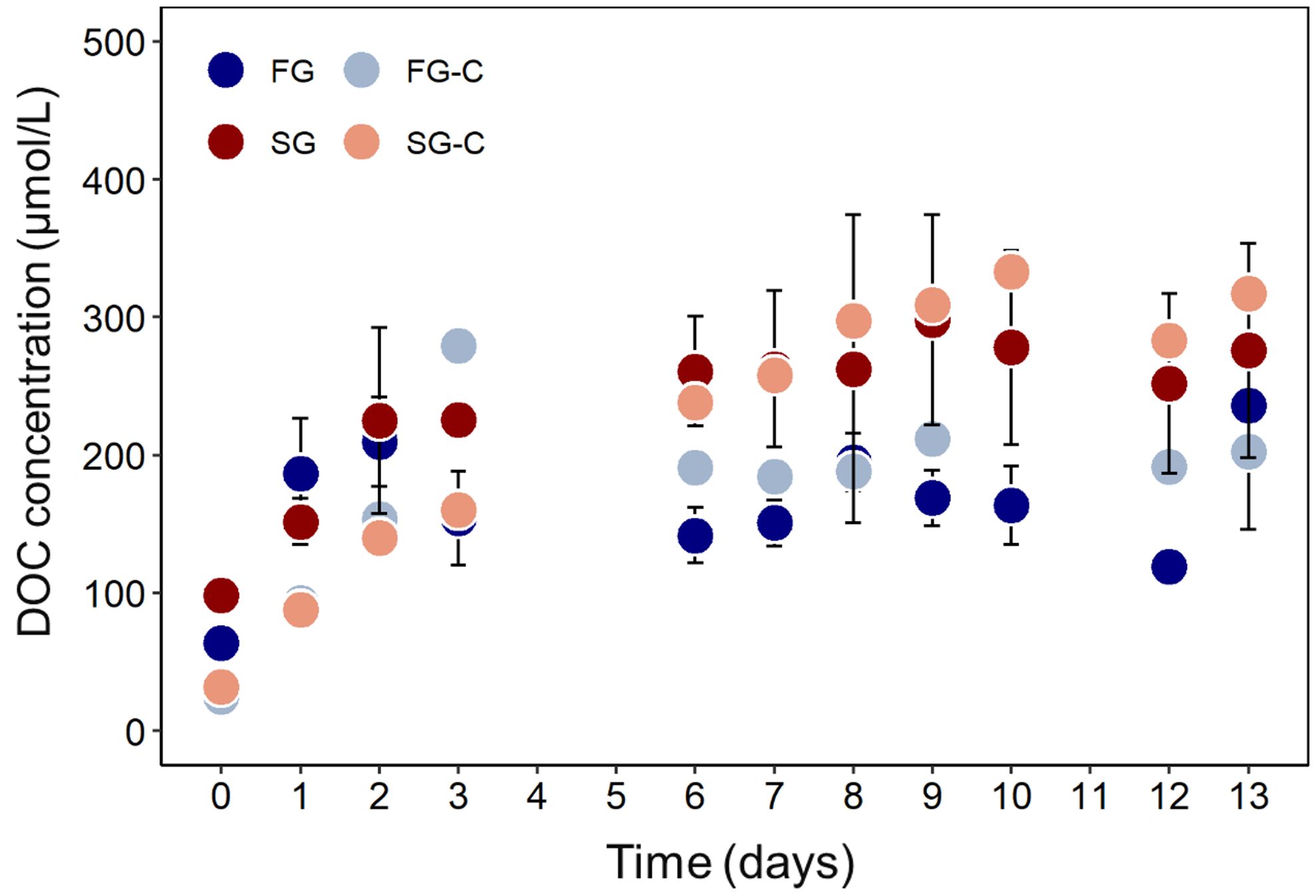
Figure 1. DOC concentration in the fresh groundwater (FG) and respective artificial seawater control (FG-C, salinity 1.6), saline groundwater (SG) and respective artificial seawater control (SG-C, salinity 29.1), during the 13-days sediment incubations in flow through reactors. Error bars for FG and SG represent standard deviation of three replicate setups.
In a recent study, Adyasari et al. (2023) also observed elevated DOC concentrations in porewater after incubation, primarily from particulate organic matter (POM) degradation in organic-rich Mobile Bay STE sediments. The continuous flushing of sediments with groundwater during the incubation could lead to continuous leaching and release of sedimentary organic matter into the DOM pool of our FG and SG reservoirs. Despite the low stock of particulate organic carbon of <0.04% in Spiekeroog beach sands (Beck et al., 2017), mobilization of only tiny fractions of this POM into the DOM pool could significantly increase the DOC concentrations in the porewater. In our leaching experiment, ASW leached ~ 0.1 µmol DOC per ml of sediment in both setups (salinity 1.6 and 29.1). Considering the sediment and groundwater volumes in our FTR incubations, such a release can explain an increase in DOC concentrations of 25 - 30 µmol/L in the groundwater reservoirs (Supplementary Table S1). However, this calculation is based on the DOC released during short sediment–water contact time of 1 h and does not consider the prolonged impact of continuous flushing of the sediments with groundwater at the pumping rhythm. It is likely that DOM was continuously mobilized from the sediments especially in the first 2-3 days during the FTR incubations, as also reported by Adyasari et al. (2023) in their long-term incubation experiment. In the natural environment of the Spiekeroog STE, this sediment-derived DOC apparently does not accumulate in the groundwater, as we do not observe increased DOC concentrations in deep groundwater. We therefore conclude that the STE is not only a sink for DOM introduced with the fresh and saline groundwater, but also a sink for sediment-derived DOM.
3.2 Response of microbial communities to fresh and saline groundwater
Concurrent with the increased DOC concentration, microbial cell numbers increased from 2.6 × 103 cells/ml on day 0 to 2.6 × 105 cells/ml on day 13 in the FG, and from 1.5 × 103 cells/ml to 2.8 × 104 cells/ml on day 13 in the SG, indicating microbial growth (Supplementary Figure S2). Cell numbers also increased in the experimental control setups from initially sterile to cell numbers of 2.8 × 103 cells/ml and 1.1 × 103 cells/ml on day 13 in the low and high salinity ASW, respectively. The initial sediments used for the incubation were not sterile, microbial cell numbers were 1.7 × 107 cells/ml sediment. We assume that sediment bacteria seeded the ASW of both salinities. We can rule out that the increase in cell numbers in the aqueous phase was solely due to accumulation of detached sediment bacteria, as the microbial community composition of the aqueous phase in all setups (FG, SG, ASW) was significantly different (ANOSIM; P 0.001) from that of the sediment (Figure 2). Microbial growth in the aqueous phase was likely facilitated by the release of growth substrates (DOM) from the sediments. Assuming an average bacterial carbon content of 20 fg/cell (Fukuda et al., 1998) and a growth yield of 40% (Bell and Kuparinen, 1984), the observed increase in bacterial cell numbers required a maximum of 1.1 µmol DOC per liter of groundwater, which is less than 1% of the observed net increase in DOC concentrations. Based on this calculation, we can rule out that the rather constant DOC concentrations after day 6 were the result of efficient DOC removal by microorganisms, but rather indicate a depletion of the mobilizable DOM reservoir.
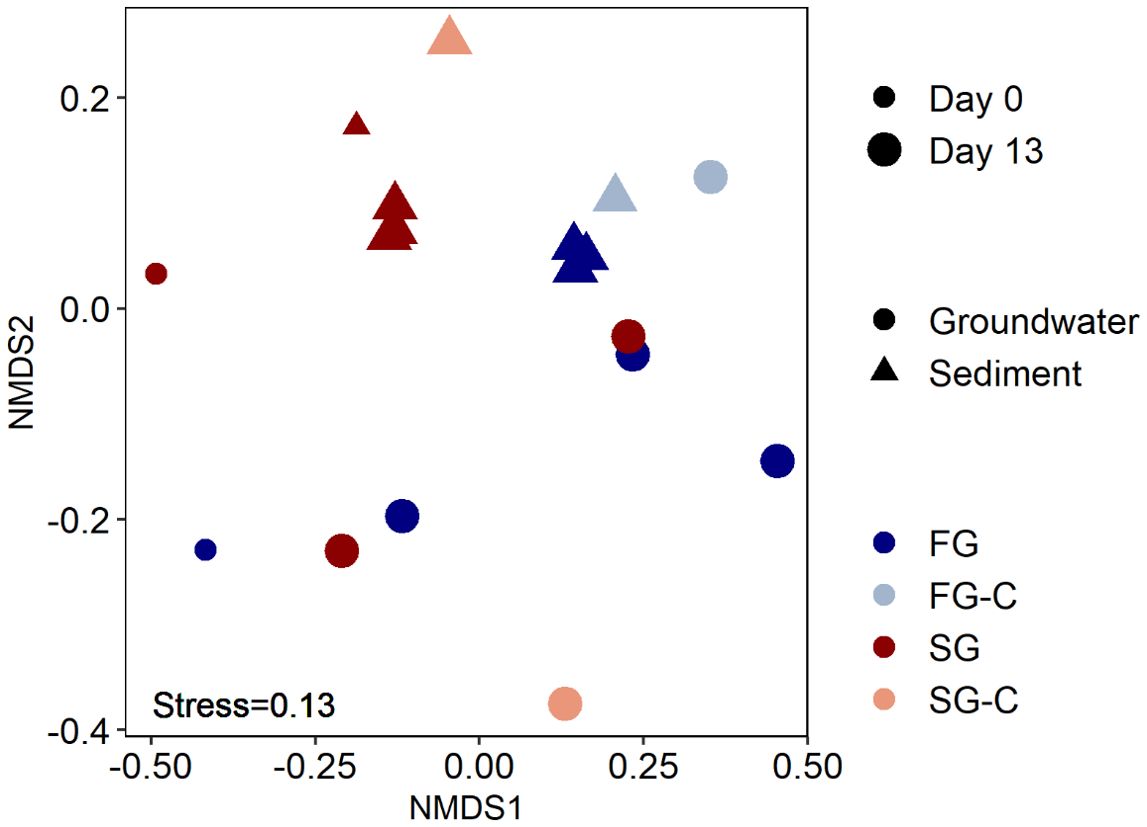
Figure 2. Non-metric multidimensional scaling (NMDS) showing the differences based on Bray-Curtis distance similarities in microbial community compositions of fresh groundwater (FG) and respective artificial seawater control (FG-C, salinity 1.6), saline groundwater (SG) and respective artificial seawater control (SG-C, salinity 29.1) at the start (day 0) and end (day 13) of flow through reactor incubations.
Cell counts in the sediments of all setups (FG, SG, FG-C, and SG-C) did not change considerably or even decreased during the 13 days of incubation (Supplementary Figure S2). However, there was a noticeable shift in the sediment microbial community composition during the incubation. The sediments flushed with FG established a different community structure compared to those flushed with SG, however flushing with FG had a more pronounced impact than SG (Figure 2). This was expected, as the sediment was collected at the high-water line and is frequently exposed to saline seawater. Nevertheless, the communities in the SG-flushed sediments changed slightly due to decreasing relative abundance of Nitrosopumilaceae and Aeromonadaceae at the expense of Rhodobacteraceae (Figure 3). Members of the Rhodobacteraceae are known to degrade phytoplankton-derived organic matter (Tada et al., 2017), which is frequently introduced during seawater flooding of the beach, while the groundwater in the FTRs was likely depleted in fresh DOM. In the sediment of the control setup, SG-C, bacteria related to Flavobacteriaceae showed a distinct increase. Members of this family are capable of degrading biopolymers, which are part of the high molecular mass fraction of DOM (Kirchman, 2002). In the FG-flushed sediment, the changes were mainly due to an increase of bacteria related to fast growing Comamonadaceae and Pseudomonadaceae, and a decrease of archaea related to Nitrosopumilaceae (Figure 3). Similar shifts were observed in the sediments flushed with ASW of the respective groundwater salinities (SG-C and FG-C), suggesting that salinity is a major control of the sediment community composition in the STE (Kimbrel et al., 2018). The increase in Comamonadaceae and Pseudomonadaceae indicates a shift towards bacteria commonly involved in the degradation of complex and recalcitrant DOM. These families are characterized by high metabolic versatility, enabling efficient utilization of diverse organic substrates and rapid response to resource fluctuations. Members of both families are capable of releasing extracellular enzymes to break down complex DOM molecules into smaller, more bioavailable forms. For instance, Pseudomonas species are known to degrade recalcitrant compounds, including aromatic hydrocarbons (Palleroni et al., 2010) while the Comamonadaceae have been associated with humic-like DOM (Broman et al., 2019).
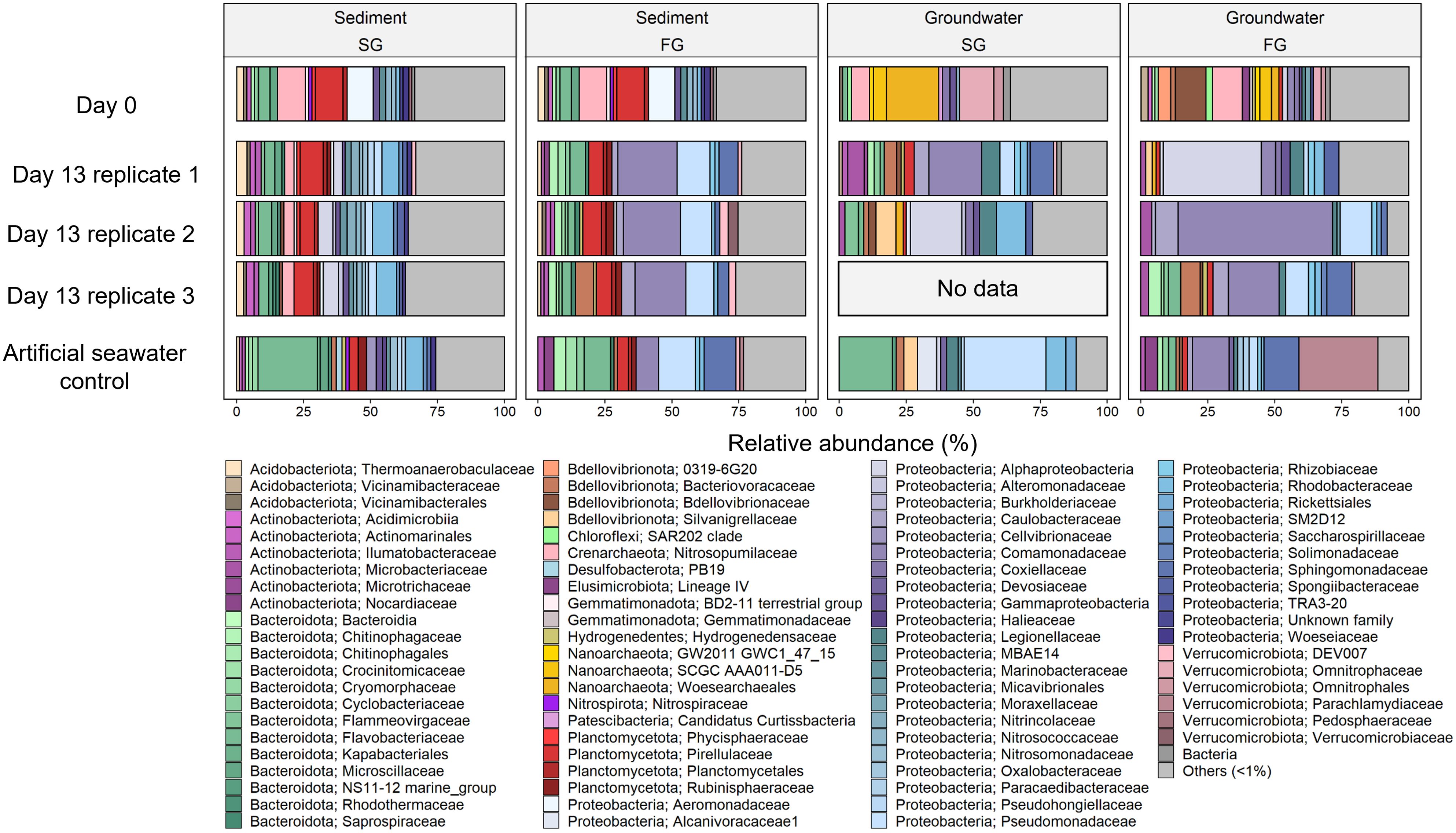
Figure 3. Relative abundance of microbial community composition of the fresh groundwater (FG), saline groundwater (SG) and their respective artificial seawater controls (FG-C and SG-C), and the sediment flushed with the different groundwater types and their respective artificial seawater controls at the start (day 0) and end (day 13) of flow through reactor incubations.
The microbial community composition of the groundwaters (FG and SG) was significantly different at day 0 (ANOSIM; p = 0.02) (Figure 2) which could also be attributed to a dominant influence of salinity, although other factors likely contribute to the establishment of groundwater microbial communities (Lozupone and Knight, 2007). Comamonadaceae were also present in the groundwater of both salinities and increased in relative abundance at end of the incubation, while ultra-small bacteria and archaea assigned to Nanoarchaeota (FG and SG) and Bdellvibrionaceae (FG) diminished (Figure 3). In contrast to the sediment-associated communities that changed very reproducibly in replicate setups, the microbial communities in the aqueous phase changed differently in the replicate setups (ANOSIM; p = 0.01). This diverging development could be due to various stochastic effects such as random initial population distributions or micro-environmental fluctuation (Zhou et al., 2013; Johansen et al., 2019).
3.3 Differential DOM composition of fresh and saline groundwater
The total number of assigned MF was 2694 in fresh groundwater DOM (FG-DOM) and 2715 in saline groundwater DOM (SG-DOM). FG- and SG-DOM shared 85-86% of the assigned MF, while 14% and 15% of the total assigned MF were exclusively present in each groundwater type, respectively. Considering the relative signal abundance of detected MF, the distribution of DOM molecular groups (saturated, unsaturated, highly unsaturated, and aromatic compounds) was similar in both groundwater types (Figure 4A). FG- and SG-DOM had high relative contributions of less labile, more reworked DOM compounds; up to 86% and 90% were highly unsaturated compounds, and 6% and 4% were unsaturated compounds, respectively. The remaining DOM pool consisted of aromatic compounds and negligible contributions (< 0.5%) of saturated compounds (Figure 4A). The high percentage of shared MF and the similar relative abundance distribution of compound groups between FG and SG indicate that reworking during transport in the deep STE significantly shapes the molecular DOM composition, resulting in more uniform signatures (Zark and Dittmar, 2018). Nonetheless, compared to SG-DOM, FG-DOM had a higher relative abundance of aromatic compounds (Figure 4A) and ITerr was higher (Figure 4B), indicating a higher contribution of terrestrial DOM. This finding corroborates a previous study by Waska et al. (2021) who observed that shallow groundwater DOM at the dune base is enriched with aromatic compounds originating from buried plant material (e.g., dune grass and beach wrack mainly composed of Kelp) and inputs from the island freshwater lens. Given that the FG was sampled from the well located close to the gray dunes, it likely receives a significant amount of groundwater DOM from the island freshwater lens and organic matter from vascular plant leachates from the dune belt (Streif, 2002; Waska et al., 2021). In contrast, the SG was sampled from the well located at the intertidal zone of the beach that constantly receives seawater infiltrating at tidal rhythm. This could explain why the SG-DOM has less terrestrial DOM signature compared to the FG-DOM. We also observed high relative abundance of nitrogen containing compounds in the SG-DOM (21% in SG and 16% in FG-DOM) which is a typical feature of marine DOM (Amon et al., 2001). In general, the chemical makeup of the SG-DOM is similar to what is reported for beach porewater samples associated with high salinity (Waska et al., 2021), consistent with a deep injection of seawater near the high water line.
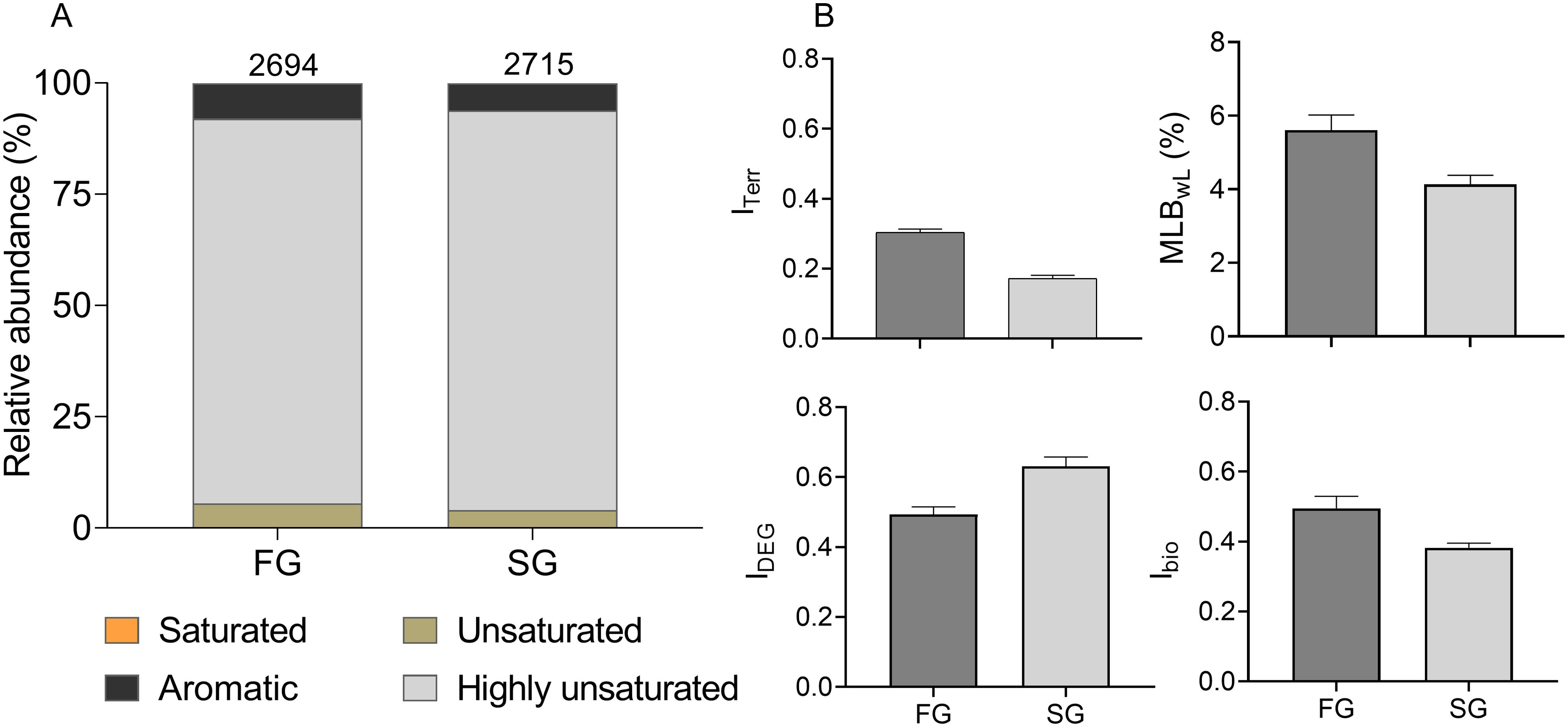
Figure 4. Bar plots showing the relative abundance of chemical categories (A) and the molecular indices (B) of the fresh groundwater (FG) and saline groundwater (SG) DOM before the start of the flow through reactor incubations (day 0). Categories are based on molecular formulae and were obtained with ICBM-OCEAN (Merder et al., 2020). The numbers above the bar plots represent the number of molecular formulae belonging to each group. The relative abundance of saturated compounds is unresolved in this plot because of its low contribution.
Although the FG-DOM had a more terrestrial DOM signature than the SG-DOM, consistent with the expected source-specific DOM signature, the MLB indicates that the DOM in both groundwater types was already highly degraded. As a general paradigm, MLB divides the MF into two groups encompassing more or less labile DOM, derived from calculated hydrogen saturation of molecular formulae (D’Andrilli et al., 2015). Environmental data from open ocean to freshwater environments indicate that marine DOM generally has a higher MLB than freshwater DOM (D’Andrilli et al., 2015; Bercovici et al., 2023b) due to higher relative abundance of bioavailable carbon fractions, such as proteins, amino sugars, and lipid-like organic matter which have higher degrees of hydrogen saturation (Chipman et al., 2010; D’Andrilli et al., 2013). The MLBwL (weighted distribution percentages) of FG- and SG-DOM (Figure 4B) were far lower than those of algal marine-derived organic matter (MLBwL = 27.2; D’Andrilli et al., 2015), but similar to MLBwL of degraded bottom seawater from the Weddell Sea, Gulf of Mexico (D’Andrilli et al., 2015), Pacific and Circumpolar deep ocean water (Bercovici et al., 2023b) and our NEqPIW reference (MLBwL = 3.8). The low MLBwL of both FG-DOM and SG-DOM (Figure 4B) in the deep STE is indicative of highly reworked DOM. This finding was further supported by the molecular indices Ibio and IDEG which assess the transformation and degradation state of DOM. The Ibio is an indicator for recent biological production and transformation of DOM that was established based on microbially produced and modified DOM, encompassing semi-labile to semi-refractory DOM (Bercovici et al., 2023b). The Ibio of both FG- and SG-DOM (Figure 4B) were higher than Ibio reported for the deep Atlantic and Pacific oceans (0.15 < Ibio < 0.30; Bercovici et al., 2023a) and our NEqPIW reference sample (Ibio; 0.2), indicating that the recent microbial transformation of STE groundwater DOM was more intense than that of deep ocean DOM. In addition, the IDEG of both FG- and SG-DOM, was similar to more degraded deep ocean water (Bercovici et al., 2023b) and our NEqPIW reference (IDEG = ~0.8). This confirms that both groundwater types are dominated by semi-labile and/or recalcitrant DOM. Longer groundwater residence time has been linked to aged/reworked DOM, for example, Waska et al. (2021), found increasing relative contributions of highly unsaturated and aromatic compounds to the DOM pool with increasing porewater age in the shallow subsurface of the Spiekeroog STE. The estimated water travel time from the dune to the low water line (Grünenbaum et al., 2020) at the study site (Spiekeroog beach) is 16–18 years. This long groundwater residence time allows enough time for the degradation or transformation of the STE groundwater DOM from more labile to more recalcitrant composition irrespective of its source. And because these DOM fractions are persistent on time scales of 4000-6000 years (Hansell et al., 2012), it is reasonable to expect their accumulation in the deep STE over time.
Although both FG- and SG-DOM are highly degraded, the SG-DOM seems to be more degraded than the FG-DOM based on its comparatively lower average MLBwL, Ibio and higher IDEG than the FG-DOM. This finding contradicts our initial expectation that seawater carries more fresh and labile DOM to the beach STE. However, as the seawater DOM arriving in the deep (6 m) STE is already quite reworked, it is likely that any labile DOM compounds that might have been introduced by the seawater were already selectively degraded before reaching the deep STE.
3.4 Change in DOM composition of the fresh and saline groundwater after incubation
After the incubation, there was no significant (TTEST, p > 0.05) change in the total number of detected MF in both groundwater types. The number of detected MF had only slightly decreased from the initial of 2694 to 2563 on day 13 in FG-DOM, and from 2715 to 2653 on day 13 in the SG-DOM, but the molecular DOM composition had changed distinctly. We identified MF within the DOM pool of both groundwater types that (1) were significantly decreased (p < 0.05) in relative abundance or removed entirely at the end of the incubation (lost), (2) remained unchanged after the incubation (core), (3) significantly increased (p < 0.05) in relative abundance or were present in the end but not at the start of the incubation (gained). It is noteworthy that in the lost group we only consider the sink for the groundwater DOM while we do not have information on the fraction of DOM that is leached from the sediment and turned over during the incubation.
The core group made up 64% and 63% of the total number of detected MF in the FG- and SG-DOM, respectively, on day 13. It contained high proportions of highly unsaturated compounds (54% in the FG and 59% in the SG-DOM), unsaturated compounds (5% in the FG and 4% in the SG-DOM), and aromatic compounds (6% in the FG and 4% in the SG-DOM) compared to the lost and gained groups (Figures 5C, D). This core group of MFs in both groundwater types has molecular characteristics similar to published DOM compositions from the deep Atlantic and Pacific oceans (core group; Bercovici et al., 2023a) and the refractory marine DOM (cluster 3) in adjacent North Sea surface water (Merder et al., 2021). The island of stability is a group of MFs found across the deep Atlantic and deemed most stable due to the positive correlation with 14C age (Lechtenfeld et al., 2014). We found that 71 -75% of the MF present in this island stability were also present in FG- and SG-DOM, respectively, indicating that the core group mostly reflected the refractory DOM background. The core group also showed a huge overlap with MF found in deep Pacific DOM (52% - 60% of the core MF were also found in the NEqPIW reference). This implies that a substantial fraction of the STE-DOM resists rapid microbial degradation and persists over time scales of groundwater residence times. However, some of this recalcitrant DOM in the deep STE could be more susceptible to other degradation processes such as photodegradation, once it leaves the STE and enters the coastal ocean (McDonough et al., 2022).
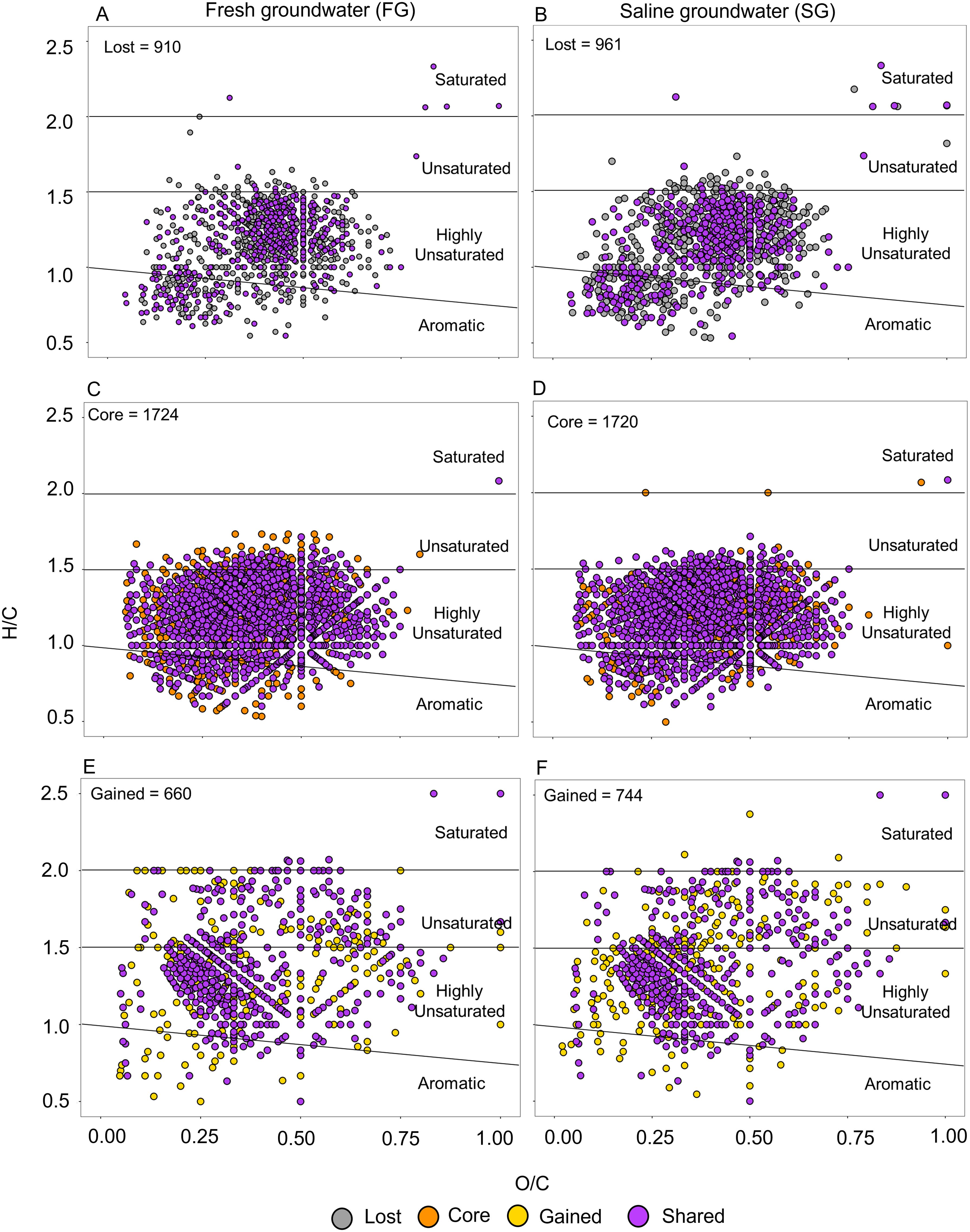
Figure 5. Van Krevelen plots for (A, B) the lost and (C, D) the core, and (E, F) gained groups in the fresh groundwater (FG, lefthand plots) and saline groundwater (SG, righthand plots). The purple color shows shared molecular formulae between FG and SG for each group. The black lines divide different DOM compound groups based on their H/C and O/C ratios (Merder et al., 2020).
The gained fraction accounted for 26% and 28% of the total number of detected MF in FG-DOM and SG-DOM at the end of the incubation, respectively. This group consisted of higher proportions of unsaturated compounds (9% in FG-DOM and 11% in SG-DOM) and negligible (<0.5%) proportions of aromatic compounds compared to the core and lost groups (Figures 5E, F). Moreover, it had higher MLBwL (Supplementary Table S2) than the core and lost groups, and also the bulk groundwater before incubation (day 0), implying that this gained fraction was more bioavailable (D’Andrilli et al., 2015). Assuming that these newly occurring MF are introduced from leaching of sedimentary material, it is reasonable to assume that the release of DOM from the sediments fuels microbial activity in the STE.
The MF lost during the incubation comprised 34% and 35% of the total number of detected MF in FG-DOM and SG-DOM, at the start of the incubation, respectively. This group had lower relative proportions of highly unsaturated (28% in FG-DOM and 30% in SG-DOM), aromatic (1.9% in FG-DOM and 1.7% in SG-DOM), and negligible (<0.5%) proportions of unsaturated compounds compared to the core group (Figures 5A, B). The mean mass and oxygen content of these lost MF were substantially higher compared to the core and gained groups (Supplementary Table S2). In general, the loss of compounds from groundwater containing mostly aged and reworked DOM suggests that there is continuous degradation of recalcitrant DOM in the deep STE. Such continuous degradation of a fraction of presumably refractory DOM has also been observed in the deep ocean (Bercovici et al., 2023a) and in crustal aquifers underlying the deep ocean (Shah Walter et al., 2018). The addition of more labile sediment-derived DOM could increase microbial activity, potentially leading to concurrent breakdown of more recalcitrant DOM in the groundwater (“priming effect”; Guenet et al., 2010). However, while in this study we focus on the microbial interactions with the DOM, microbial processing may not be the sole reason for the observed loss of DOM compounds within the comparably short timeframe of 13-days incubation. We cannot exclude that DOM was also removed through abiotic processes such as adsorption to mineral surfaces. Coagulation of DOM with iron oxides preferentially removes larger and oxygen-rich molecules (Riedel et al., 2012; Gomez-Saez et al., 2015; Linkhorst et al., 2017), which matches the molecular characteristics of the lost fraction. However, as both sediment and water used in our FTRs were fully oxidized, we assume that coagulation with newly formed metal oxides was negligible.
In general, we observed a similar change in the composition of FG- and SG-DOM after the incubation. This is also evident in the visual representation of van Krevelen diagrams of lost (Figures 5A, B), core (Figures 5C, D), and gained groups (Figures 5E, F) of both groundwater types. There was a similar distribution of DOM compounds with up to 74-75% shared MF in the core, and 42-45% shared MF in the lost group between the FG- and SG-DOM. It is remarkable that deep STE groundwaters originating from distinct sources and carrying different DOM share such similar DOM chemical composition after incubation. This similarity in the composition of lost and core groups between FG- and SG-DOM indicates that the microorganisms processed a similar fraction of DOM irrespective of its source. This also implies that despite the development of different microbial community composition in the sediments during the 13 days incubation, these communities tend to transform a similar fraction of the provided DOM independent of its source. There was also a 62-70% overlap of MF in the gained group between FG- and SG-DOM, which suggests a similar input source during the incubation. As this DOM fraction is not dependent on the initial groundwater DOM composition, it is likely introduced into the groundwater from sediment leaching.
3.5 Mobilizable DOM from sediments
We assume that the increased DOC concentrations and the gain of DOM compounds (gained group) in both groundwater types at the end of our incubation is due to sediment leaching. For the molecular distinction of DOM derived from pure sediment leaching in our incubation, we compared DOM molecular compositions in the leachates from our leaching experiment (ASW-leachates) to those of our FTR control setups (ASW-controls) of high salinity (SG-C) and low salinity (FG-C), as well as to the gained group of the groundwater incubations (FG and SG).
Approximately half (46-47%) of the MF detected in the FTR-controls were also present in the ASW-leachates, confirming that release from sediments is indeed a source of additional DOM in our incubations. However, a large fraction of the MF detected in the FTR controls (53% for FG-C and 54% for SG-C) was not found in the respective ASW leachates (Supplementary Figure S3). There was also a difference in the relative abundance of compound groups between the FTR controls and ASW leachates (Figure 6). The ASW leachates were enriched in unsaturated (58% for low salinity and 48% for high salinity) compounds compared to the FTR- controls. The MLBwL of ASW leachates (61% for low salinity and 51% for high salinity) was significantly higher than that of the FTR controls (28% for FG-C and 20% for SG-C). On the other hand, DOM in the FTR-controls consisted of higher relative proportions of aromatic compounds (7% for FG-C and 10% for SG-C) and up to two times higher proportions of highly unsaturated compounds (65% for FG-C and 70% for SG-C) compared to the ASW leachates. The difference in DOM composition of ASW leachates and FTR controls could be due to the different sediment-water contact times during the leaching experiment (1 hour) and the FTR incubations (13 days). Apparently, initial leaching mobilized potentially more labile DOM compared to the continuous leaching in the FTR-controls.
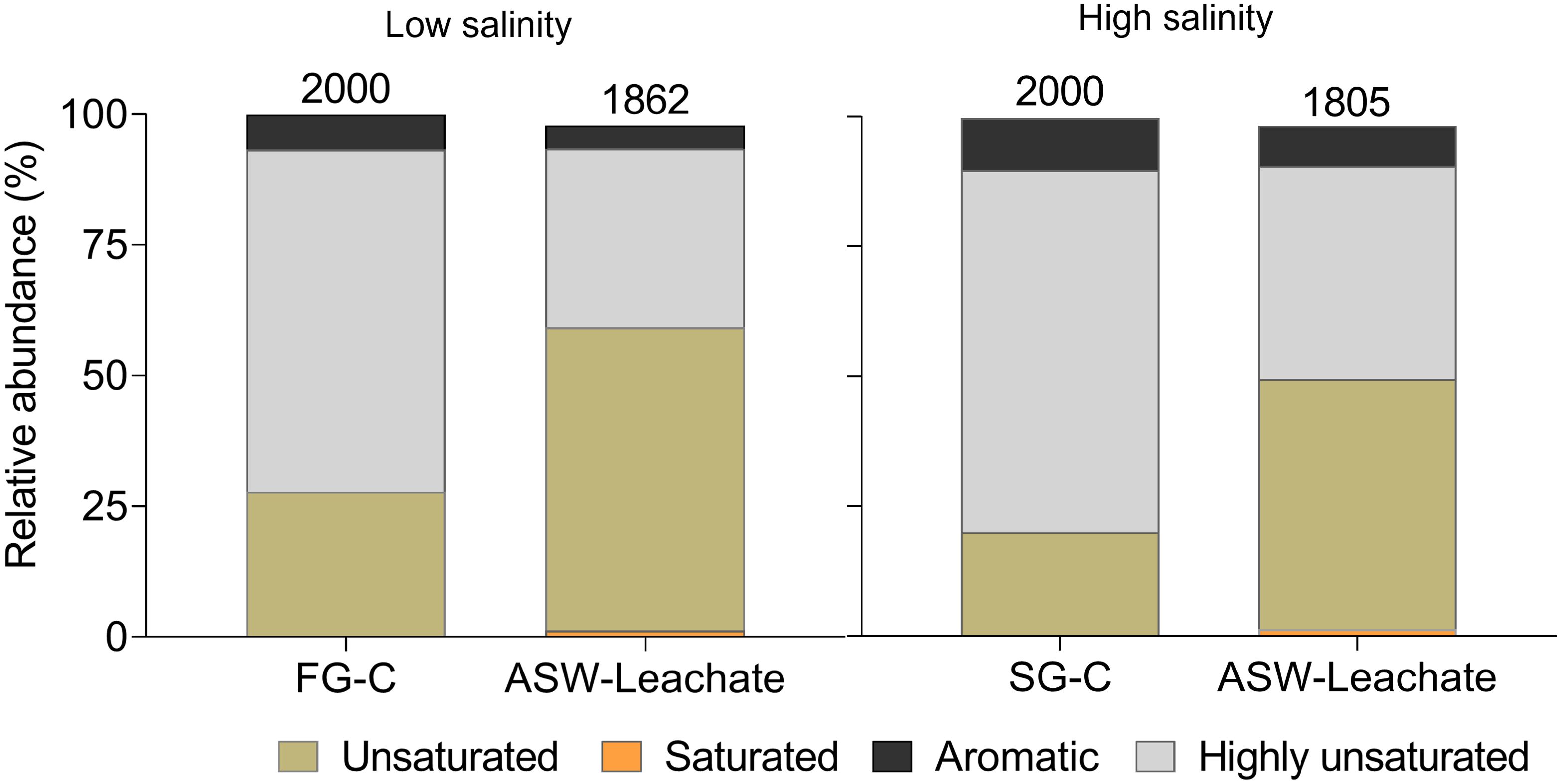
Figure 6. Bar plots showing the relative abundance of chemical categories of the FTR-controls (FG-C and SG-C) and ASW-leachates of low salinity and high salinity. Categories are based on molecular formulae and were obtained with ICBM-OCEAN (Merder et al., 2020). The numbers above the bar plots represent the number of molecular formulae belonging to each group. Due to the difference in the quality of DOM analysis of FG-C and SG-C samples, for this comparison, we considered the same total number of molecular formulae (2000) for each. These 2000 molecular formulae were those with the highest peak intensity detected in each of the samples.
The accumulation of more aromatic compounds in the FTR-controls due to longer and continuous sediment leaching could potentially have diluted or overprinted the initially more labile DOM signature in the FTR-controls. However, as we observed microbial growth in the FTR controls, the DOM signature of the FTR-controls is likely a result of both leaching and continuous microbial reworking of more labile DOM into more recalcitrant DOM (Jiao et al., 2011; Dittmar et al., 2021). Previous studies also report the selective preservation of less labile molecules, such as aromatic DOM, and carboxyl-rich alicyclic acids (CRAM) during long-term incubation experiments (Lechtenfeld et al., 2015; Valle et al., 2018; Lian et al., 2021). We conclude that in the FTR-control setups, we have a combined signature of DOM leached from the sediments and of microbially transformed DOM. This conclusion is consistent with higher Ibio values in the FTR-controls compared to the ASW-leachates (Supplementary Figure S4), indicating a higher degree of recent microbial activity (Bercovici et al., 2023b). The occurrence of microbial growth in the control FTRs makes it challenging to disentangle the contribution of leached DOM to the overall change in DOM composition during the incubations. The gained MF of the groundwater incubations had a comparatively small overlap (16-17%) with the MF detected in the ASW-leachates, indicating efficient transformation of the sediment-derived MF by the prevailing microbes. Alternatively, as most (62-66%) of the MF detected in the sediment leachates were already present in the groundwater used for the incubations which might be expected as the groundwater continuously flushes STE sediments; they were not identified as gained.
4 Conclusion
Our study shows that the “aged” DOM from different groundwater sources in the deep STE of Spiekeroog high-energy beach still undergoes degradation, and that the microbial communities of the beach STE thrive on a similar fraction of DOM independent of its source. The potential contribution of DOM released from sediments, as an additional substrate for microbial communities, has not been assessed in previous studies. We show that beach sediments are significant sources of DOM to the STE microbial communities, and that the lability of sediment-derived DOM may largely depend on the extent of sediment leaching and/or microbial processing. The release of DOM from sediments likely affects the overall degradation of organic matter in the deep STE and stimulates growth of specific microorganisms. Therefore, it is important to constrain the quantity of sedimentary DOM release, to determine the origin and age of mobilizable DOM, and to elucidate the mechanisms governing its mobilization or retention over extended periods in the STE.
Data availability statement
The datasets presented in this study can be found in online repositories. The names of the repository/repositories and accession number(s) can be found below: https://www.ebi.ac.uk/ena, PRJEB80523.
Author contributions
GA: Conceptualization, Formal analysis, Visualization, Writing – original draft, Writing – review & editing. SB: Conceptualization, Formal analysis, Visualization, Writing – review & editing. BE: Conceptualization, Writing – review & editing, Funding acquisition, Supervision. JN: Conceptualization, Funding acquisition, Supervision, Writing – review & editing.
Funding
The author(s) declare financial support was received for the research, authorship, and/or publication of this article. This study was conducted within the research unit FOR 5094: The Dynamic Deep subsurface of high-energy beaches (DynaDeep), funded by the German Research Foundation (Deutsche Forschungsgemeinschaft, DFG).
Acknowledgments
The authors wish to thank the student assistants during field and laboratory work; Jennifer Onunze, Olukunle Stevens, Matthew Lawrence, Abdullah Furkan Özcan, Oluwatosin Oluwole. We are also grateful to Ina Ulber, Matthias Friebe, Katrin Klaproth, Heike Simon, Mandy Knutzen, and Frank Meyerjürgens from ICBM for their assistance with DOC, DOM and microbial community composition analyses. We are grateful for the infrastructure installed by the DynaDeep project and to all the DynaDeep scientists and project partners.
Conflict of interest
The authors declare that the research was conducted in the absence of any commercial or financial relationships that could be construed as a potential conflict of interest.
Generative AI statement
The author(s) declare that no Generative AI was used in the creation of this manuscript.
Publisher’s note
All claims expressed in this article are solely those of the authors and do not necessarily represent those of their affiliated organizations, or those of the publisher, the editors and the reviewers. Any product that may be evaluated in this article, or claim that may be made by its manufacturer, is not guaranteed or endorsed by the publisher.
Supplementary material
The Supplementary Material for this article can be found online at: https://www.frontiersin.org/articles/10.3389/fmars.2024.1501781/full#supplementary-material
References
Adyasari D., Dimova N., Waska H., Chadhain S. N. (2023). Dissolved organic matter and nutrient processing in organic-rich subterranean estuaries: Implications for future land use and climate scenarios. Geochim. Cosmochim. Acta 362, 65–76. doi: 10.1016/j.gca.2023.10.025
Amon R. M. W., Fitznar H.-P., Benner R. (2001). Linkages among the bioreactivity, chemical composition, and diagenetic state of marine dissolved organic matter. Limnol. Oceanogr 46, 287–297. doi: 10.4319/lo.2001.46.2.0287
Anschutz P., Smith T., Mouret A., Deborde J., Bujan S., Poirier D., et al. (2009). Tidal sands as biogeochemical reactors. Estuar. Coast. Shelf Sci. 84, 84–90. doi: 10.1016/j.ecss.2009.06.015
Azam F., Fenchel T., Field J. G., Gray J. S., Meyer-Reil L.-A., Thingstad F. (1983). The ecological role of water-column microbes in the sea. Mar. Ecol. Prog. Ser. Oldend 10, 257–263. doi: 10.3354/meps010257
Beck M., Reckhardt A., Amelsberg J., Bartholomä A., Brumsack H.-J., Cypionka H., et al. (2017). The drivers of biogeochemistry in beach ecosystems: a cross-shore transect from the dunes to the low-water line. Mar. Chem. 190, 35–50. doi: 10.1016/j.marchem.2017.01.001
Bell R. T., Kuparinen J. (1984). Assessing phytoplankton and bacterioplankton production during early spring in Lake Erken, Sweden. Appl. Environ. Microbiol. 48, 1221–1230. doi: 10.1128/aem.48.6.1221-1230.1984
Bercovici S. K., Dittmar T., Niggemann J. (2023a). Processes in the surface ocean regulate dissolved organic matter distributions in the deep. Global Biogeochem. Cycles 37, e2023GB007740. doi: 10.1029/2023GB007740
Bercovici S. K., Wiemers M., Dittmar T., Niggemann J. (2023b). Disentangling biological transformations and photodegradation processes from marine dissolved organic matter composition in the global ocean. Environ. Sci. Technol. 57, 21145–21155. doi: 10.1021/acs.est.3c05929
Broman E., Asmala E., Carstensen J., Pinhassi J., Dopson M. (2019). Distinct coastal microbiome populations associated with autochthonous-and allochthonous-like dissolved organic matter. Front. Microbiol. 10, 2579. doi: 10.3389/fmicb.2019.02579
Chipman L., Podgorski D., Green S., Kostka J., Cooper W., Huettel M. (2010). Decomposition of plankton-derived dissolved organic matter in permeable coastal sediments. Limnol. Oceanogr 55, 857–871. Available online at: https://www.jstor.org/stable/40985152.
D’Andrilli J., Cooper W. T., Foreman C. M., Marshall A. G. (2015). An ultrahigh-resolution mass spectrometry index to estimate natural organic matter lability. Rapid Commun. Mass Spectrom 29, 2385–2401. doi: 10.1002/rcm.7400
D’Andrilli J., Foreman C. M., Marshall A. G., McKnight D. M. (2013). Characterization of IHSS Pony Lake fulvic acid dissolved organic matter by electrospray ionization Fourier transform ion cyclotron resonance mass spectrometry and fluorescence spectroscopy. Org. Geochem 65, 19–28. doi: 10.1016/j.orggeochem.2013.09.013
Degenhardt J., Merder J., Heyerhoff B., Simon H., Engelen B., Waska H. (2021). Cross-shore and depth zonations in bacterial diversity are linked to age and source of dissolved organic matter across the intertidal area of a sandy beach. Microorganisms 9, 1720. doi: 10.3390/microorganisms9081720
Dittmar T., Koch B., Hertkorn N., Kattner G. (2008). A simple and efficient method for the solid-phase extraction of dissolved organic matter (SPE-DOM) from seawater. Limnol. Oceanogr. Methods 6, 230–235. doi: 10.4319/lom.2008.6.230
Dittmar T., Lennartz S. T., Buck-Wiese H., Hansell D. A., Santinelli C., Vanni C., et al. (2021). Enigmatic persistence of dissolved organic matter in the ocean. Nat. Rev. Earth Environ. 2, 570–583. doi: 10.1038/s43017-021-00183-7
Dobrynin M., Gayer G., Pleskachevsky A., Günther H. (2010). Effect of waves and currents on the dynamics and seasonal variations of suspended particulate matter in the North Sea. J. Mar. Syst. 82, 1–20. doi: 10.1016/j.jmarsys.2010.02.012
Flerus R., Lechtenfeld O. J., Koch B. P., McCallister S. L., Schmitt-Kopplin P., Benner R., et al. (2012). A molecular perspective on the ageing of marine dissolved organic matter. Biogeosciences 9, 1935–1955. doi: 10.5194/bg-9-1935-2012
Fuhrman J. A., Cram J. A., Needham D. M. (2015). Marine microbial community dynamics and their ecological interpretation. Nat. Rev. Microbiol. 13, 133–146. doi: 10.1038/nrmicro3417
Fukuda R., Ogawa H., Nagata T., Koike I. (1998). Direct determination of carbon and nitrogen contents of natural bacterial assemblages in marine environments. Appl. Environ. Microbiol. 64, 3352–3358. doi: 10.1128/AEM.64.9.3352-3358.1998
Gabor E. M., de Vries E. J., Janssen D. B. (2003). Efficient recovery of environmental DNA for expression cloning by indirect extraction methods. FEMS Microbiol. Ecol. 44, 153–163. doi: 10.1016/S0168-6496(02)00462-2
Gomez-Saez G. V., Riedel T., Niggemann J., Pichler T., Dittmar T., Bühring S. I. (2015). Interaction between iron and dissolved organic matter in a marine shallow hydrothermal system off Dominica Island (Lesser Antilles). Mar. Chem. 177, 677–686. doi: 10.1016/j.marchem.2015.10.003
Greskowiak J., Massmann G. (2021). The impact of morphodynamics and storm floods on pore water flow and transport in the subterranean estuary. Hydrol. Process 35, e14050. doi: 10.1002/hyp.14050
Greskowiak J., Seibert S. L., Post V. E. A., Massmann G. (2023). Redox-zoning in high-energy subterranean estuaries as a function of storm floods, temperatures, seasonal groundwater recharge and morphodynamics. Estuar. Coast. Shelf Sci. 290, 108418. doi: 10.1016/j.ecss.2023.108418
Grünenbaum N., Greskowiak J., Sültenfuß J., Massmann G. (2020). Groundwater flow and residence times below a meso-tidal high-energy beach: a model-based analyses of salinity patterns and 3H-3He groundwater ages. J. Hydrol 587, 124948. doi: 10.1016/j.jhydrol.2020.124948
Grünenbaum N., Günther T., Greskowiak J., Vienken T., Müller-Petke M., Massmann G. (2023). Salinity distribution in the subterranean estuary of a meso-tidal high-energy beach characterized by Electrical Resistivity Tomography and direct push technology. J. Hydrol 617, 129074. doi: 10.1016/j.jhydrol.2023.129074
Guenet B., Danger M., Abbadie L., Lacroix G. (2010). Priming effect: bridging the gap between terrestrial and aquatic ecology. Ecology 91, 2850–2861. doi: 10.1890/09-1968.1
Hansell D. A., Carlson C. A., Schlitzer R. (2012). Net removal of major marine dissolved organic carbon fractions in the subsurface ocean. Global Biogeochem. Cycles 26, GB1016. doi: 10.1029/2011GB004069
Jiao N., Herndl G. J., Hansell D. A., Benner R., Kattner G., Wilhelm S. W., et al. (2011). The microbial carbon pump and the oceanic recalcitrant dissolved organic matter pool. Nat. Rev. Microbiol. 9, 555. doi: 10.1038/nrmicro2386-c5
Johansen R., Albright M., Gallegos-Graves L. V., Lopez D., Runde A., Yoshida T., et al. (2019). Tracking replicate divergence in microbial community composition and function in experimental microcosms. Microb. Ecol. 78, 1035–1039. doi: 10.1007/s00248-019-01368-w
Kim K. H., Michael H. A., Field E. K., Ullman W. J. (2019). Hydrologic shifts create complex transient distributions of particulate organic carbon and biogeochemical responses in beach aquifers. J. Geophysical Research: Biogeosciences 124, 3024–3038. doi: 10.1029/2019JG005114
Kim T.-H., Waska H., Kwon E., Suryaputra I. G. N., Kim G. (2012). Production, degradation, and flux of dissolved organic matter in the subterranean estuary of a large tidal flat. Mar. Chem. 142, 1–10. doi: 10.1016/j.marchem.2012.08.002
Kimbrel J. A., Ballor N., Wu Y.-W., David M. M., Hazen T. C., Simmons B. A., et al. (2018). Microbial community structure and functional potential along a hypersaline gradient. Front. Microbiol. 9, 1492. doi: 10.3389/fmicb.2018.01492
Kirchman D. L. (2002). The ecology of Cytophaga–Flavobacteria in aquatic environments. FEMS Microbiol. Ecol. 39, 91–100. doi: 10.1016/S0168-6496(01)00206-9
Koch B. P., Dittmar T. (2006). From mass to structure: An aromaticity index for high-resolution mass data of natural organic matter. Rapid Commun. mass Spectrom 20, 926–932. doi: 10.1002/rcm.v20:5
Koch B. P., Dittmar T. (2016). Erratum: from mass to structure: an aromaticity index for high-resolution mass data of natural organic matter. Rapid Commun. Mass Spectrom 30, 250–250. doi: 10.1002/rcm.v30.1
Koch B. P., Kattner G., Witt M., Passow U. (2014). Molecular insights into the microbial formation of marine dissolved organic matter: recalcitrant or labile? Biogeosciences 11, 4173–4190. doi: 10.5194/bg-11-4173-2014
Kujawinski E. B., Del Vecchio R., Blough N. V., Klein G. C., Marshall A. G. (2004). Probing molecular-level transformations of dissolved organic matter: insights on photochemical degradation and protozoan modification of DOM from electrospray ionization Fourier transform ion cyclotron resonance mass spectrometry. Mar. Chem. 92, 23–37. doi: 10.1016/j.marchem.2004.06.038
Lechtenfeld O. J., Hertkorn N., Shen Y., Witt M., Benner R. (2015). Marine sequestration of carbon in bacterial metabolites. Nat. Commun. 6, 6711. doi: 10.1038/ncomms7711
Lechtenfeld O. J., Kattner G., Flerus R., McCallister S. L., Schmitt-Kopplin P., Koch B. P. (2014). Molecular transformation and degradation of refractory dissolved organic matter in the Atlantic and Southern Ocean. Geochim. Cosmochim. Acta 126, 321–337. doi: 10.1016/j.gca.2013.11.009
Lian J., Zheng X., Zhuo X., Chen Y., He C., Zheng Q., et al. (2021). Microbial transformation of distinct exogenous substrates into analogous composition of recalcitrant dissolved organic matter. Environ. Microbiol. 23, 2389–2403. doi: 10.1111/1462-2920.15426
Linkhorst A., Dittmar T., Waska H. (2017). Molecular fractionation of dissolved organic matter in a shallow subterranean estuary: the role of the iron curtain. Environ. Sci. Technol. 51, 1312–1320. doi: 10.1021/acs.est.6b03608
Lozupone C. A., Knight R. (2007). Global patterns in bacterial diversity. Proc. Natl. Acad. Sci. 104, 11436–11440. doi: 10.1073/pnas.0611525104
Lueders T., Manefield M., Friedrich M. W. (2004). Enhanced sensitivity of DNA-and rRNA-based stable isotope probing by fractionation and quantitative analysis of isopycnic centrifugation gradients. Environ. Microbiol. 6, 73–78. doi: 10.1046/j.1462-2920.2003.00536.x
Lunau M., Lemke A., Walther K., Martens-Habbena W., Simon M. (2005). An improved method for counting bacteria from sediments and turbid environments by epifluorescence microscopy. Environ. Microbiol. 7, 961–968. doi: 10.1111/j.1462-2920.2005.00767.x
Massmann G., Abarike G., Amoako K., Auer F., Badewien T. H., Berkenbrink C., et al. (2023). The DynaDeep observatory–a unique approach to study high-energy subterranean estuaries. Front. Mar. Sci. 10, 1189281. doi: 10.3389/fmars.2023.1189281
McDonough L. K., Andersen M. S., Behnke M. I., Rutlidge H., Oudone P., Meredith K., et al. (2022). A new conceptual framework for the transformation of groundwater dissolved organic matter. Nat. Commun. 13, 2153. doi: 10.1038/s41467-022-29711-9
Medeiros P. M., Seidel M., Niggemann J., Spencer R. G. M., Hernes P. J., Yager P. L., et al. (2016). A novel molecular approach for tracing terrigenous dissolved organic matter into the deep ocean. Global Biogeochem. Cycles 30, 689–699. doi: 10.1002/2015GB005320
Merder J., Freund J. A., Feudel U., Hansen C. T., Hawkes J. A., Jacob B., et al. (2020). ICBM-OCEAN: processing ultrahigh-resolution mass spectrometry data of complex molecular mixtures. Anal. Chem. 92, 6832–6838. doi: 10.1021/acs.analchem.9b05659
Merder J., Röder H., Dittmar T., Feudel U., Freund J. A., Gerdts G., et al. (2021). Dissolved organic compounds with synchronous dynamics share chemical properties and origin. Limnol. Oceanogr 66, 4001–4016. doi: 10.1002/lno.v66.11
Michael H. A., Mulligan A. E., Harvey C. F. (2005). Seasonal oscillations in water exchange between aquifers and the coastal ocean. Nature 436, 1145–1148. doi: 10.1038/nature03935
Milici M., Deng Z.-L., Tomasch J., Decelle J., Wos-Oxley M. L., Wang H., et al. (2016). Co-occurrence analysis of microbial taxa in the Atlantic Ocean reveals high connectivity in the free-living bacterioplankton. Front. Microbiol. 7, 649. doi: 10.3389/fmicb.2016.00649
Moore W. S. (1999). The subterranean estuary: a reaction zone of ground water and sea water. Mar. Chem. 65, 111–125. doi: 10.1016/S0304-4203(99)00014-6
Palleroni N. J., Pieper D. H., Moore E. R. B. (2010). “Microbiology of Hydrocarbon-Degrading Pseudomonas.” in Handbook of Hydrocarbon and Lipid Microbiology, ed. Timmis K. N.. doi: 10.1007/978-3-540-77587-4_129
Parada A. E., Needham D. M., Fuhrman J. A. (2016). Every base matters: assessing small subunit rRNA primers for marine microbiomes with mock communities, time series and global field samples. Environ. Microbiol. 18, 1403–1414. doi: 10.1111/emi.2016.18.issue-5
Riedel T., Biester H., Dittmar T. (2012). Molecular fractionation of dissolved organic matter with metal salts. Environ. Sci. Technol. 46, 4419–4426. doi: 10.1021/es203901u
Robinson C., Gibbes B., Carey H., Li L. (2007a). Salt-freshwater dynamics in a subterranean estuary over a spring-neap tidal cycle. J. Geophys. Res. Ocean 112. doi: 10.1029/2006JC003888
Robinson C., Gibbes B., Li L. (2006). Driving mechanisms for groundwater flow and salt transport in a subterranean estuary. Geophys. Res. Lett. 33, L03402. doi: 10.1029/2005GL025247
Robinson C., Li L., Barry D. A. (2007b). Effect of tidal forcing on a subterranean estuary. Adv. Water Resour 30, 851–865. doi: 10.1016/j.advwatres.2006.07.006
Robinson C., Xin P., Li L., Barry D. A. (2014). Groundwater flow and salt transport in a subterranean estuary driven by intensified wave conditions. Water Resour. Res. 50, 165–181. doi: 10.1002/2013WR013813
Robinson C. E., Xin P., Santos I. R., Charette M. A., Li L., Barry D. A. (2018). Groundwater dynamics in subterranean estuaries of coastal unconfined aquifers: Controls on submarine groundwater discharge and chemical inputs to the ocean. Adv. Water Resour 115, 315–331. doi: 10.1016/j.advwatres.2017.10.041
RStudio Team. (2022). RStudio: Integrated Development Environment for R. (Boston, MA: RStudio, PBC). Availabe online at: http://www.rstudio.com/.
Santos I. R., Eyre B. D., Huettel M. (2012). The driving forces of porewater and groundwater flow in permeable coastal sediments: A review. Estuar. Coast. Shelf Sci. 98, 1–15. doi: 10.1016/j.ecss.2011.10.024
Seibert S. L., Greskowiak J., Prommer H., Böttcher M. E., Massmann G. (2019). Modeling of biogeochemical processes in a barrier island freshwater lens (Spiekeroog, Germany). J. Hydrol 575, 1133–1144. doi: 10.1016/j.jhydrol.2019.05.094
Seibert S. L., Holt T., Reckhardt A., Ahrens J., Beck M., Pollmann T., et al. (2018). Hydrochemical evolution of a freshwater lens below a barrier island (Spiekeroog, Germany): the role of carbonate mineral reactions, cation exchange and redox processes. Appl. Geochemistry 92, 196–208. doi: 10.1016/j.apgeochem.2018.03.001
Seidel M., Beck M., Greskowiak J., Riedel T., Waska H., Suryaputra I. G. N. A., et al. (2015). Benthic-pelagic coupling of nutrients and dissolved organic matter composition in an intertidal sandy beach. Mar. Chem. 176, 150–163. doi: 10.1016/j.marchem.2015.08.011
Seidel M., Beck M., Riedel T., Waska H., Suryaputra I. G. N. A., Schnetger B., et al. (2014). Biogeochemistry of dissolved organic matter in an anoxic intertidal creek bank. Geochim. Cosmochim. Acta 140, 418–434. doi: 10.1016/j.gca.2014.05.038
Shah Walter S. R., Jaekel U., Osterholz H., Fisher A. T., Huber J. A., Pearson A., et al. (2018). Microbial decomposition of marine dissolved organic matter in cool oceanic crust. Nat. Geosci 11, 334–339. doi: 10.1038/s41561-018-0109-5
Streif H. (2002). “The Pleistocene and Holocene development of the southeastern North Sea basin and adjacent coastal areas,” in Climate development and history of the North Atlantic Realm (Springer-Verlag Berlin Heidelberg GmbH), 387–397.
Tada Y., Nakaya R., Goto S., Yamashita Y., Suzuki K. (2017). Distinct bacterial community and diversity shifts after phytoplankton-derived dissolved organic matter addition in a coastal environment. J. Exp. Mar. Bio. Ecol. 495, 119–128. doi: 10.1016/j.jembe.2017.06.006
Tebbe D. A., Geihser S., Wemheuer B., Daniel R., Schäfer H., Engelen B. (2022). Seasonal and zonal succession of bacterial communities in North Sea salt marsh sediments. Microorganisms 10, 859. doi: 10.3390/microorganisms10050859
Thornton D. C. O. (2014). Dissolved organic matter (DOM) release by phytoplankton in the contemporary and future ocean. Eur. J. Phycol 49, 20–46. doi: 10.1080/09670262.2013.875596
Valle J., Gonsior M., Harir M., Enrich-Prast A., Schmitt-Kopplin P., Bastviken D., et al. (2018). Extensive processing of sediment pore water dissolved organic matter during anoxic incubation as observed by high-field mass spectrometry (FTICR-MS). Water Res. 129, 252–263. doi: 10.1016/j.watres.2017.11.015
Waska H., Brumsack H.-J., Massmann G., Koschinsky A., Schnetger B., Simon H., et al. (2019a). Inorganic and organic iron and copper species of the subterranean estuary: Origins and fate. Geochim. Cosmochim. Acta 259, 211–232. doi: 10.1016/j.gca.2019.06.004
Waska H., Greskowiak J., Ahrens J., Beck M., Ahmerkamp S., Böning P., et al. (2019b). Spatial and temporal patterns of pore water chemistry in the inter-tidal zone of a high energy beach. Front. Mar. Sci. 6, 154. doi: 10.3389/fmars.2019.00154
Waska H., Simon H., Ahmerkamp S., Greskowiak J., Ahrens J., Seibert S. L., et al. (2021). Molecular traits of dissolved organic matter in the subterranean estuary of a high-energy beach: indications of sources and sinks. Front. Mar. Sci. 8, 607083. doi: 10.3389/fmars.2021.607083
Zark M., Dittmar T. (2018). Universal molecular structures in natural dissolved organic matter. Nat. Commun. 9, 3178. doi: 10.1038/s41467-018-05665-9
Zech H., Thole S., Schreiber K., Kalhöfer D., Voget S., Brinkhoff T., et al. (2009). Growth phase-dependent global protein and metabolite profiles of Phaeobacter gallaeciensis strain DSM 17395, a member of the marine Roseobacter-clade. Proteomics 9, 3677–3697. doi: 10.1002/pmic.200900120
Keywords: dissolved organic matter (DOM), subterranean estuary (STE), microbial community, groundwater, flow-through reactors, mobilizable DOM, high-energy beach
Citation: Abarike GA, Brick S, Engelen B and Niggemann J (2024) Different dissolved organic matter sources sustain microbial life in a sandy beach subterranean estuary – an incubation study. Front. Mar. Sci. 11:1501781. doi: 10.3389/fmars.2024.1501781
Received: 25 September 2024; Accepted: 02 December 2024;
Published: 17 December 2024.
Edited by:
Nicholas David Ward, Pacific Northwest National Laboratory (DOE), United StatesReviewed by:
Jeonghyun Kim, Jeju National University, Republic of KoreaMorgane Derrien, Universidad de O’Higgins, Chile
Copyright © 2024 Abarike, Brick, Engelen and Niggemann. This is an open-access article distributed under the terms of the Creative Commons Attribution License (CC BY). The use, distribution or reproduction in other forums is permitted, provided the original author(s) and the copyright owner(s) are credited and that the original publication in this journal is cited, in accordance with accepted academic practice. No use, distribution or reproduction is permitted which does not comply with these terms.
*Correspondence: Jutta Niggemann, anV0dGEubmlnZ2VtYW5uQHVvbC5kZQ==