- 1State Key Laboratory of Marine Environmental Science, College of Ocean and Earth Sciences, Xiamen University, Xiamen, China
- 2Department of Biogeochemistry, Max Planck Institute for Marine Microbiology, Bremen, Germany
- 3Department of Geosciences, Princeton University, Princeton, NJ, United States
- 4Graduate School of Oceanography, University of Rhode Island, Narragansett, RI, United States
- 5Marine Operations, Xiamen University, Xiamen, China
- 6Department of Marine and Coastal Sciences, Rutgers University, New Brunswick, NJ, United States
- 7National Oceanography Centre, Southampton, United Kingdom
- 8Aix Marseille Univ, Université de Toulon, CNRS, IRD, MIO UM 110, Marseille, France
- 9Turing Center for Living Systems, Aix-Marseille University, Marseille, France
- 10Nordcee, Department of Biology, University of Southern Denmark, Odense, Denmark
- 11Danish Institute for Advanced Studies (DIAS), University of Southern Denmark, Odense, Denmark
- 12Environmental Studies, Soka University of America, Aliso Viejo, CA, United States
- 13Department of Ocean and Earth Sciences, Old Dominion University, Norfolk, VA, United States
- 14Guangdong Provincial Key Laboratory of Marine Disaster Prediction and Prevention, Institute of Marine Sciences, Shantou University, Shantou, China
- 15State Key Laboratory of Marine Resources Utilization in South China Sea, Hainan University, Haikou, China
Biological dinitrogen (N2) fixation, the energetically expensive conversion of N2 gas to ammonia, plays an essential role in balancing the nitrogen budget in the ocean. Accumulating studies show detectable N2 fixation rates below the euphotic zone in various marine systems, revealing new insights of marine N2 fixation. However, the reported rates are highly variable and frequently fall close to detection limits, raising the question of the ubiquity and significance of N2 fixation in the global dark ocean. Using highly sensitive isotopic labeling incubation including a set of control incubations, we confirm the occurrence of mesopelagic N2 fixation in the South China Sea. Interestingly, we consistently observed that ca. 30% of samples show a significant elevation of 15N in the particulate nitrogen after incubation at most depths (200 - 1000 m). Although this approach does not allow accurate quantification of N2 fixation rates, our data suggest the occurrence of dark N2 fixation yet with highly heterogeneous signals in the mesopelagic zone of the South China Sea. A data compilation of reported N2 fixation in the global dark ocean further reveals that such heterogeneity has also been observed elsewhere, unveiling the ubiquitous heterogeneity in mesopelagic N2 fixation. Thus, we call for more observations to constrain mesopelagic N2 fixation budgets and to understand the underlying mechanism for such heterogeneity.
1 Introduction
Biological nitrogen fixation (N2 fixation) is an important source of new nitrogen to the open ocean by converting inert N2 gas into bioavailable nitrogen (Jickells et al., 2017). N2 fixation was long assumed to occur primarily within the euphotic zone where photoautotrophy can accommodate its high energetic cost (Zehr and Kudela, 2011). Nitrogenase is the enzyme responsible for N2 fixation, and the highly conserved nitrogenase reductase gene (nifH) has been frequently targeted to detect diazotrophs in the ocean (Zehr and Capone, 1996). However, pervasive observations of nifH-containing non-photosynthetic microorganisms in the global ocean (Zehr et al., 1998; Moisander et al., 2008; Delmont et al., 2022) and recent studies demonstrating active N2 fixation via non-cyanobacterial diazotrophs (Harding et al., 2022; Tschitschko et al., 2024) indicate the potential for non-cyanobacterial marine N2 fixation, motivating broader investigations into the range of diazotrophs and their habitats, including in the mesopelagic zone (Bombar et al., 2016; Moisander et al., 2017; Benavides et al., 2018a).
Given the high energetic cost of N2 fixation relative to the assimilation of nitrate (the major form of dissolved inorganic nitrogen (DIN) in the deep ocean; Falkowski, 1983; Zehr and Kudela, 2011), it seems counter-intuitive that this process occurs in nitrate-replete and energy-starved aphotic waters (Moisander et al., 2017). Several hypotheses have been proposed to explain the occurrence of mesopelagic N2 fixation: (1) deep sea diazotrophs may lack the transporters necessary to assimilate or reduce nitrate (Karl et al., 2002; Bombar et al., 2016); (2) N2 fixation may help cells maintain an ideal intracellular redox state (Bentzon-Tilia et al., 2015; Bombar et al., 2016); (3) nitrate could be depleted in aggregates as a result of microbial succession in densely packed microbial consortia (Dekas et al., 2009; Bombar et al., 2016; Chakraborty et al., 2021). Anaerobic microenvironments within particles or lower ambient oxygen concentrations at depth could also be suitable niches for diazotrophs because the high energetic cost largely comes from protecting nitrogenase from being inactivated by oxygen (Postgate, 1970; Paerl and Prufert, 1987; Großkopf and LaRoche, 2012; Farnelid et al., 2018; Riemann et al., 2022).
Several studies have detected N2 fixation in the aphotic water column across a wide range of marine regimes including both hypoxic (Fernandez et al., 2011; Hamersley et al., 2011; Bonnet et al., 2013; Dekaezemacker et al., 2013; Farnelid et al., 2013; Loescher et al., 2014; Löscher et al., 2016; Gradoville et al., 2017; Chang et al., 2019; Selden et al., 2019, 2021b; Saxena et al., 2023) and oxygenated waters (Rahav et al., 2013, 2015; Benavides et al., 2015, 2016, 2018b; Weber, 2015; Gradoville et al., 2017; Mulholland et al., 2019; Shiozaki et al., 2023). Reported rates are highly variable, ranging from below the detection limit up to 35.9 nmol N L-1 d-1 in the oxygen minimum zone in the Eastern Tropical North Pacific (ETNP; Selden et al., 2019), but mostly fall below detection limits when they are assessed (Selden et al., 2021b). Despite the typically low volumetric rates, mesopelagic N2 fixation can contribute up to 100% to integrated water column N2 fixation rates (Benavides et al., 2016). As such, low rates matter for mesopelagic N2 fixation, when integrated with the large volume of aphotic water, and thus, may contribute significantly to global nitrogen budget (Benavides et al., 2018a; Zehr and Capone, 2020).
However, owing to the low volumetric rates of mesopelagic N2 fixation and low concentrations of particulate nitrogen (PN) in the ocean’s interior, care must be taken to ensure that reported rates are representative and above the detection limits of analytical methods (Gradoville et al., 2017; Moisander et al., 2017; White et al., 2020). Mesopelagic N2 fixation rates that are low or close to the detection limit could also be biased by processes other than N2 fixation (e.g., isotopic fractionation during DIN or dissolved organic nitrogen assimilation), and inflated or deflated by errors in elemental analysis–isotope ratio mass spectrometry (EA-IRMS) at low PN amount (White et al., 2020). Thus, methods with sufficient 15N2 enrichment together with controls and precise isotopic measurements are crucial. By far, EA-IRMS is still a major approach to determine the isotopic composition of PN. However, the recommended PN amount for EA-IRMS is 10 μg N, requiring large volume incubations (e.g. the averaged PN concentration of the mesopelagic water in our study was 0.07 µmol N L-1, which results in a minimum volume of 10 L per sample for EA-IRMS) with high human and ship-board cost, limiting a broad measurement of mesopelagic N2 fixation (Gradoville et al., 2017; White et al., 2020). Even the limited measurements show highly variable rates (Benavides et al., 2018b). Moreover, several studies find the change of 15N before and after incubation is highly variable between replicate samples collected from the same depth, hindering the determination of a robust rate (Bonnet et al., 2013; Gradoville et al., 2017). However, it is also improper to conclude the absence of mesopelagic N2 fixation when some of the replicates show reliable elevation of 15N. Overall, the relatively few rate measurements performed up until now, their variability, and the fact that many observations are at or near the limit of analytical/computational detection result in ambiguous understanding of mesopelagic N2 fixation and large uncertainties in their contribution to the global fixed N budget, calling for in depth investigation of this process (Moisander et al., 2017; White et al., 2020; Selden et al., 2021b).
Here, we conducted N2 fixation incubations in the mesopelagic South China Sea (SCS), the largest marginal sea in the western Pacific Ocean. The SCS is a representative oligotrophic marginal sea, although active N2 fixation has been repeatedly reported in the euphotic zone of the SCS, mesopelagic N2 fixation remains to be investigated in the SCS (Wu et al., 2024). Therefore, SCS is an ideal place to test the ubiquity of mesopelagic N2 fixation. To better constrain the isotopic composition of PN under low PN amounts, we implemented an alkaline persulfate digestion (Knapp et al., 2005) coupled with a denitrifier method (the persulfate-denitrifier method hereafter; Sigman et al., 2001; Casciotti et al., 2002; McIlvin and Casciotti, 2011). This method requires only 1.4 μg N (or 100 nmol N) rather than the roughly 10 μg N required when using standard EA-IRMS analysis (White et al., 2020). We also conducted parallel HgCl2-killed and tracer-free controls to account for non-diazotrophic signals possibly reflected in isotopic composition of PN. Using this new method, we were able to report robust mesopelagic N2 fixation signals in individual samples yet with high variabilities between the replicated samples collected from the same depth and between different depths and stations, reflecting detectable but not quantifiable mesopelagic N2 fixation and its heterogeneity. Our new results add to the global data compilation, reveal that heterogeneity is an inherent nature of mesopelagic N2 fixation, and call for more research and new approaches to account for such heterogeneity in estimating the rates and further its contribution to the global N budget.
2 Materials and methods
2.1 Field sampling
Water samples were collected from 4 stations during 2 cruises in the SCS aboard the R/V Tan Kah Kee in August 2018 (KK1806) and July 2019 (KK1905; Figure 1). Salinity, temperature, dissolved oxygen, fluorescence, and photosynthetically active radiation (PAR) data were obtained via a SeaBird conductivity-temperature-depth (CTD) profiler (SBE 911 plus). Water samples for incubations were obtained from a rosette equipped with 24 × 12-L-Niskin bottles.
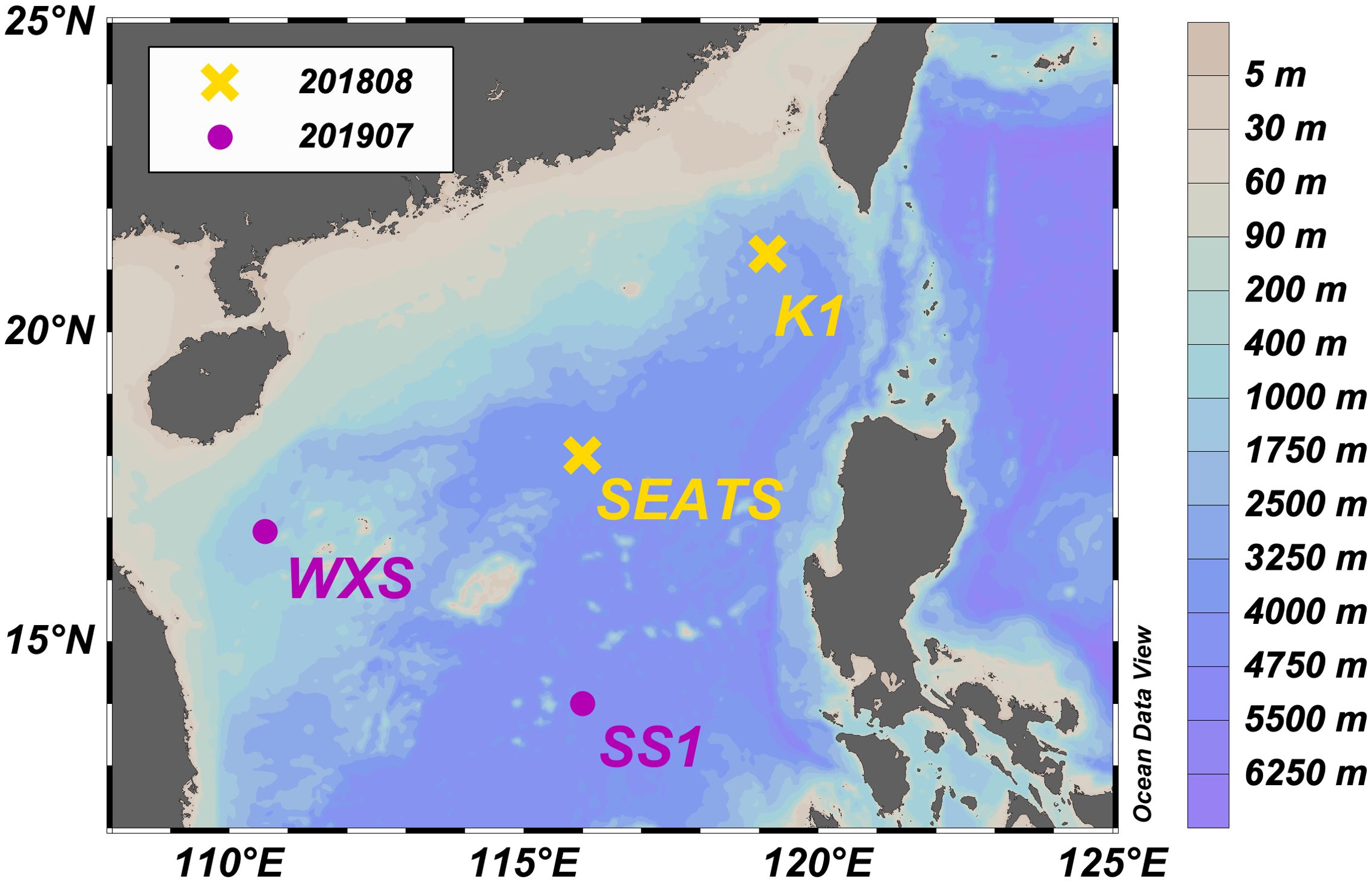
Figure 1. Station map of the two cruises in the SCS. Stations visited in August 2018 are shown in orange and those visited in July 2019 are shown in purple. Background color indicates bathymetry. The map was generated via Ocean Data View (Schlitzer, Reiner, Ocean Data View, odv.awi.de, 2020).
2.2 N2 fixation incubations
N2 fixation was measured by the incorporation of 15N2 into PN (Montoya et al., 1996). All incubations were conducted in the dark and at in situ temperature. Incubations were terminated after 24 to 96 hours by filtration of PN onto pre-combusted 25 mm 0.3 µm GF75 filters (Advantec) under <400 mbar. GF75 filters were used due to their efficiency in catching <0.7 μm diazotrophs (Bombar et al., 2018). All filters were preserved at – 80°C until isotopic composition analysis.
Samples were collected from 4 stations at different depths (K1: 5, 15, 30, 50, 75, 100, 200, 300 and 740 m; SEATS: 5, 15, 30, 50, 75, 100, 200, 300, 705, 1000, 2000 and 3800 m (bottom); SS1: 5, 15, 25, 50, 75, 100, 120, 150, 200, 300, 500, 700, 1000 and 4000 m (bottom); WXS: 5, 200 and 855 m; Figure 1). Sampling at each station included the oxygen minimum depth (OMD; K1: 740 m; SEATS: 705 m; SS1: 700 m; WXS: 855 m), although oxygen concentrations were never severely depleted (> 62 μM). Water samples from above 100 m were collected into acid-cleaned 1 L polycarbonate bottles (Nalgene) and those below 100 m were collected in acid-cleaned 4 L fluorinated polyethylene (FLPE) bottles. After sampling, bottles were kept in the dark using light-blocking black plastic bags until incubations began. Incubations above 200 m at the SEATS, K1 and SS1 stations were started at dusk (17:00 – 19:30). Supplementary Figure 1 provides a schematic plot of the different incubations conducted during both cruises.
During the August 2018 cruise, N2 fixation was measured using the 15N2-enriched seawater method (Mohr et al., 2010). However, this method reportedly reduces atom-% enrichment to 2-5% (White et al., 2020), requiring larger volumes of the enriched seawater for the incubations in environments with low N2 fixation rates. In addition, large 0.2 μm-filtered enriched seawater (as much as 10% v/v) amendments dilute PN, and thus decrease the sensitivity of IRMS measurements. In contrast, the 15N2 bubble method (Montoya et al., 1996), which we employed on the July 2019 cruise, does not dilute PN. However, this method can significantly underestimate N2 fixation rates since the 15N2 bubble can take between 3 to 15 hours to dissolve (Mohr et al., 2010; Wannicke et al., 2018; White et al., 2020). Our 24- to 96-hour incubations were designed to minimize the effect of delayed bubble dissolution as well as provide sufficient time for PN enrichment in 15N (Wannicke et al., 2018). Still, our results should be considered as conservative estimates considering the time for bubble dissolution, and possible cross-feeding and isotope dilution due to the long incubation periods. Details of our experimental design are shown in Table 1 and Supplementary Figure 1. The addition of 15N2 in the incubation bottles was kept <10 15N-atom% for 15N2-enriched seawater method and <20 15N-atom% for 15N2 bubble method. Samples below 200 m were incubated to two destructively sampled time points: 48 and 96 h during the August 2018 cruise and 24 and 48 h during the July 2019 cruise.
The 15N2-enriched seawater amendment used for the August 2018 incubations was made ≤24 h prior to the experiments following previous studies (Shiozaki et al., 2015; Lu et al., 2017, 2019). Seawater was filtered (0.22 μm membrane, Millipore), degassed (STERAPORE 20M1500A membrane, Mitsubishi Rayon Co., Ltd.) and stored in 2 L Tedlar Bags excluding bubbles. 20 mL 15N2 gas (98.9%, Cambridge Isotope Laboratories) was injected and mixed manually until all bubbles dissolved. Either 100 mL or 400 mL of 15N2-enriched seawater was added to 1 L or 4 L incubation bottles, respectively, resulting in 15N2 enrichments of 6 to 10 or 7 to 9 15N-atom%, respectively (assuming complete 15N2 dissolution). Due to limited water sample availability, seawater from the surface and 1000 m was collected in the SCS basin area prior to incubation sampling to make the 15N2-enriched seawater used for 5 to 100 m and 200 to 3800 m incubation samples, respectively. Samples were filtered immediately after tracer addition to determine the initial (T0) 15N-atom% of the PN. Incubations were conducted for 24 h for waters ≤100 m and for 48 and 96 h for waters >100 m under the dark condition and in situ temperature (± 6°C for ≤100 m samples, and ± 2.5°C for all the other samples).
For the 15N2 bubble method used on the July 2019 cruise, incubation bottles were filled with seawater excluding bubbles. Natural samples (considered as T0) were filtered immediately after sampling before tracer addition. Either 1 mL or 10 mL of 15N2 gas (98.9%, Cambridge Isotope Laboratories) equilibrated to atmospheric pressure was added through septum caps to the 1 L and 4 L bottles, respectively. Bottles were then inverted at least 10 times to enhance dissolution. Incubations were conducted in the dark and at in situ temperatures ±2.5°C for 24 h for waters <200 m (except the 5 m sample at WXS station, for which 48-hour incubations were also conducted) and for 24 and 48 h for waters ≥200 m.
2.3 Control experiments
Both tracer-free and HgCl2-killed control incubations were conducted in August 2018 at 200 m, 300 m, 705 m, 1000 m, 2000 m, 3800 m depths at the SEATS station parallel to the N2 fixation incubations. For tracer-free controls, 1 L polycarbonate bottles were fully filled with seawater without any 15N2 addition and incubated as described above to determine any change in 15N-atom% of PN over the course of incubations. For the killed controls, after adding 1 mL ca. 0.2 mol L-1 HgCl2 solution, 100 mL 15N2-enriched seawater was added to 1 L polycarbonate bottles to test whether abiotic processes could change the 15N-atom% of PN over the course of incubations. Controls were incubated and PN samples were collected as described above.
2.4 PN concentration and 15N-atom% determination
Filters collected as described above were freeze-dried and the PN they contained was subsequently oxidized to nitrate using the alkaline persulfate digestion method (Knapp et al., 2005; Yan et al., 2022). The concentration and isotopic composition of this nitrate were then determined via the denitrifier method (Sigman et al., 2001; Casciotti et al., 2002; McIlvin and Casciotti, 2011) using a GasBench IRMS (Delta V, Thermo Scientific). Briefly, potassium peroxodisulfate (K2S2O8, Merck, 105091) was recrystallized three times for purification, then mixed with sodium hydroxide (NaOH, Merck, 106498) and ultrapure water, forming peroxodisulfate reagent (POR) with 6: 6: 100 mass ratio of K2S2O8: NaOH: Milli-Q water. Before each digestion experiment, POR blank was determined by autoclaving POR solution at 105°C for 70 min, and their pH was adjusted to ca. 6 with 6 mol L-1 hydrochloric acid (HCl, Merck, 100317), after which nitrate concentrations were determined using the chemiluminescence method (Braman and Hendrix, 1989). The POR was only used if its N blank was below 2 μM N (Supplementary Figure 2A). A volume of 0.5 mL of POR was added to each sample, which was digested using the same procedure as POR blanks.
The N introduced by the POR was <1 nmol (<4% of the total sample N). The isotopic composition of blanks in each batch were reproducible. Likewise, the filter blanks contained 11.9 ± 2.7 nmol N (Supplementary Figure 2C), accounting for 14.0 ± 4.5% and 4.6 ± 3.3% of the N content of the samples in the August 2018 and July 2019 cruises, respectively. The low and reproducible contribution of the POR and filters reduced uncertainties, lowered detection limits for measured sample N, and can be subtracted as well.
The N recovery (102 ± 3.6%) and reproducibility of this digestion method were determined using known amounts of urea and EDTA (Supplementary Figure 2B). δ15N was -1.5 ± 0.3‰ for urea and -0.4 ± 0.1‰ for EDTA. These were not significantly different from δ15N values acquired via EA-IRMS which were -2.0 ± 0.03‰ and -0.4 ± 0.05‰ for urea and EDTA, respectively (Supplementary Figure 2B). Both recovery rates and δ15N values were stable between 90 and 2000 nmol of urea and EDTA (Supplementary Figure 2B), suggesting a negligible isotope fractionation effect during the digestion process. Together, the low blank and stable recovery of added N during the digestion experiment enabled a precise measurement of the concentration and isotopic composition of PN down to 90 nmol PN, which was the minimum tested value in this study. Although the minimum amount of N required for stable δ15N using the denitrifier method can be as low as 5 nmol (Supplementary Figure 2D). However, in order to minimize biases caused by blanks of GF75 and POR (total 11.9 ± 2.7 nmol N), our recommended amount of PN for this method is ≥100 nmol.
The original 15N-atom% and PN amount data obtained in this study are available in Supplementary Table 1.
2.5 Analytical considerations for dark N2 fixation
Reliable N2 fixation signals come from both sufficient PN amount for isotopic determination and the increase of 15N-PN exceeding the minimum acceptable change. As mentioned above, our recommended amount of PN for the persulfate-denitrifier method is ≥100 nmol. However, not all the samples exceeded this recommended amount. Considering that more PN amount leads to less impact of blanks and more stable isotopic analysis by IRMS, we divide samples into 3 intervals according to the PN amount on the filters, i.e. <100 nmol N, 100 - 400 nmol N, and >400 nmol N. For each interval, we calculated averaged 15N-atom% of PN and their standard deviations for T0s from the N2 fixation incubations that fall into each interval. Then, the minimum acceptable changes for N2 fixation for each interval were set to be the averaged 15N-atom% + 3 times the standard deviation. In this way, we account for both natural variability and analytical errors. We consider that N2 fixation occurs when the 15N-atom% enrichment of PN after incubation surpasses the minimum acceptable change. In addition, rates were only considered quantifiable when the averaged 15N-atom% after incubation exceeded 10 times the standard deviation of replicates. As a result, even though we detected N2 fixation signals, there is no quantifiable N2 fixation rate below 200 m. Therefore, no rates were calculated here.
2.6 Data compilation
We collected available dark N2 fixation rate data (depth ≥200 m) from previous studies listed in Table 2. We combined studies in similar geographical regions, and only included those with more than 5 data points for representative comparison. As a result, we compiled 8 studies, i.e. Gradoville et al. (2017) for ALOHA station in north Pacific subtropical gyre, Benavides et al. (2016) for Mediterranean Sea, Selden et al. (2019) for Eastern Tropical North Pacific, Bonnet et al. (2013) and Löscher et al. (2016) for Eastern Tropical South Pacific, Benavides et al. (2018b) for Western Tropical South Pacific, Shiozaki et al. (2023) for Eastern South Pacific, and Saxena et al. (2023) for Bay of Bengal. It is also worth noting that N2 fixation rates from ALOHA were all below the minimum quantifiable rates (Gradoville et al., 2017). The data used for compilation are shown in Supplementary Table 2.
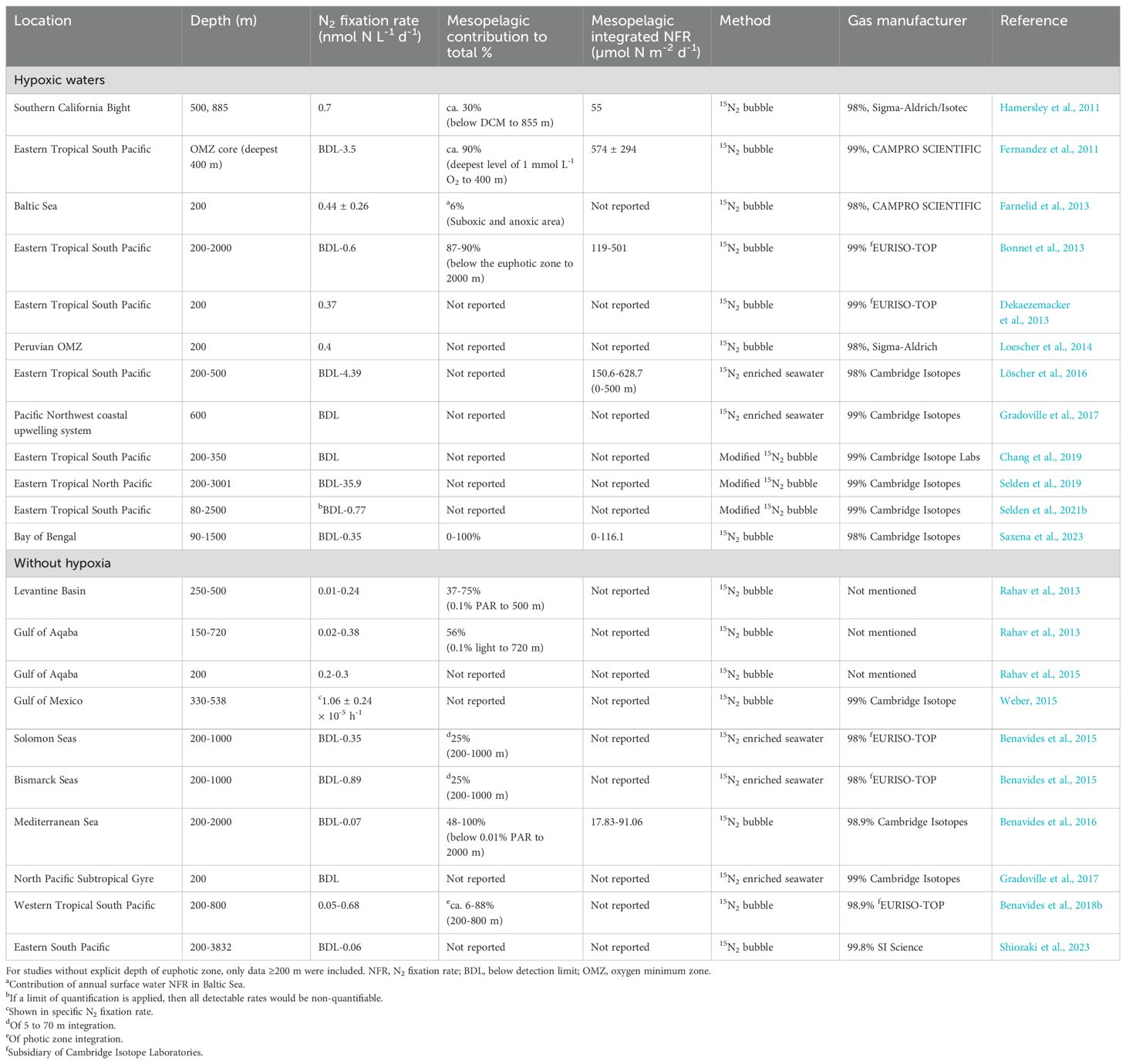
Table 2. Compilation of N2 fixation rate measurements conducted below the euphotic zone (mesopelagic N2 fixation) using 15N2 labeling techniques.
3 Results and discussion
3.1 Detectable but not quantifiable mesopelagic N2 fixation in the South China Sea
We investigated mesopelagic N2 fixation in the South China Sea basin from 200 m until around 4000 m bottom depths. In order to distinguish the N2 fixation signal from other processes that might have resulted in 15N-atom% change of PN, we conducted parallel control incubations with no tracer addition and with 15N2 tracer but killed with HgCl2 at SEATS station from 200 m to the bottom depth of 3800 m. Our results show that only two samples in all the samples of the control experiment (<3%) exceeded the minimum acceptable change of 15N-atom% for PN, which is defined according to the T0s in N2 fixation incubations (Figure 2). This supports the applicability of the above defined minimum acceptable 15N-atom% change, and also indicates that processes other than N2 fixation had negligible effect on the 15N-atom% of PN during the incubations. By comparison, more than 20% of the ≥200 m samples after parallel 15N2 incubation had a 15N-atom% of PN above the minimum acceptable change (Figure 3), showing the occurrence of active N2 fixation at mesopelagic depths, but that could not be quantified due to the large variation between replicates. Moreover, statistical analyses show that 15N-atom% of PN at T1 and T2 are significantly higher than those at T0 (t-Test: samples assuming unequal variances, p < 0.05), whereas no significant difference was found in the control experiments (Figure 4). This further confirms the robustness of N2 fixation signals, and negligible effect of other processes on 15N-atom% of PN.
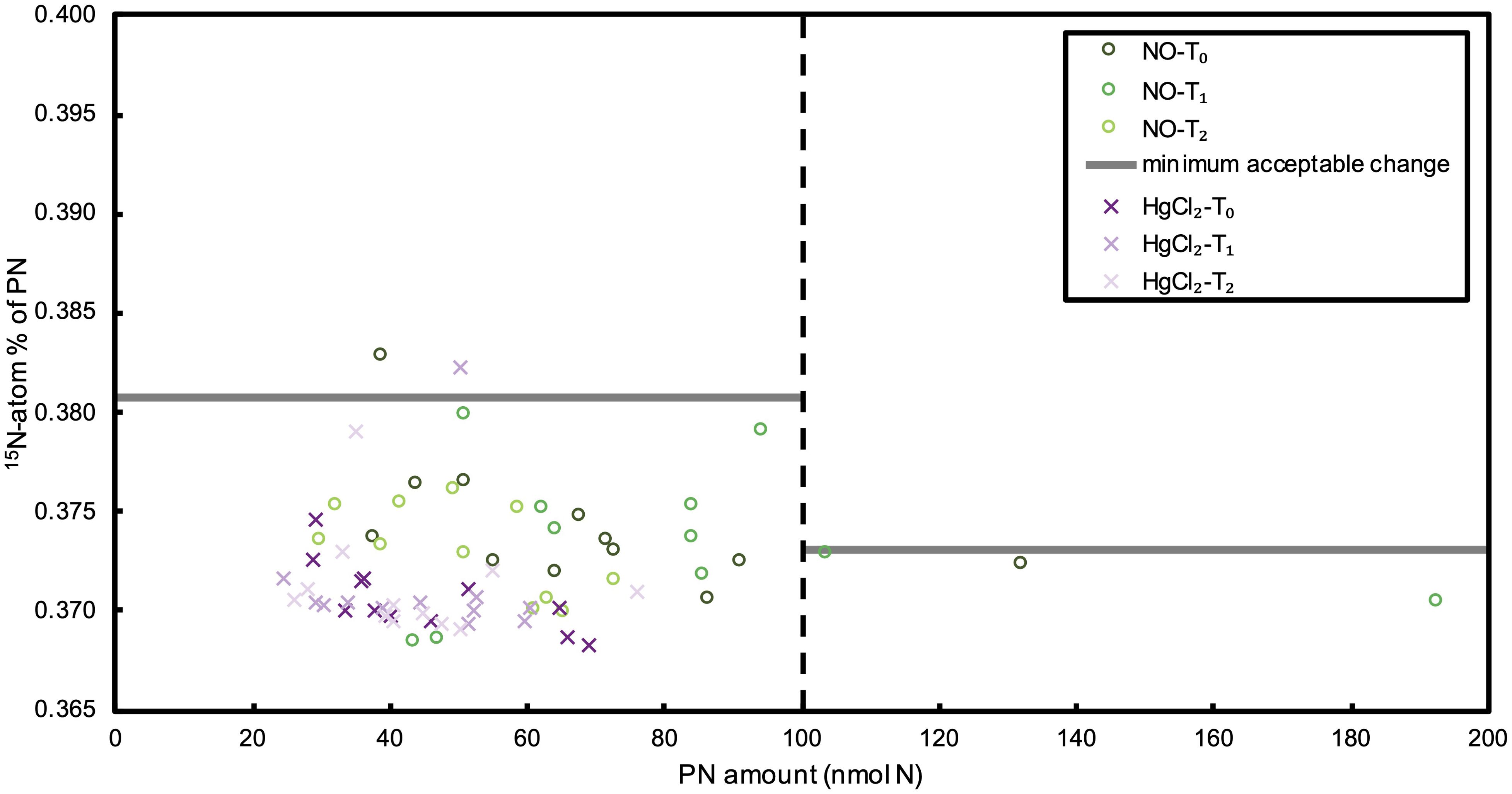
Figure 2. 15N-atom% of PN in no tracer (NO) and killed (HgCl2) control incubations at SEATS station 200 - 3800 m. The x-axis indicates the PN amount on the sample filters, and the vertical dashed line indicates PN amount of 100 nmol N. Horizontal gray lines represent the minimum acceptable 15N-atom% change for different intervals.
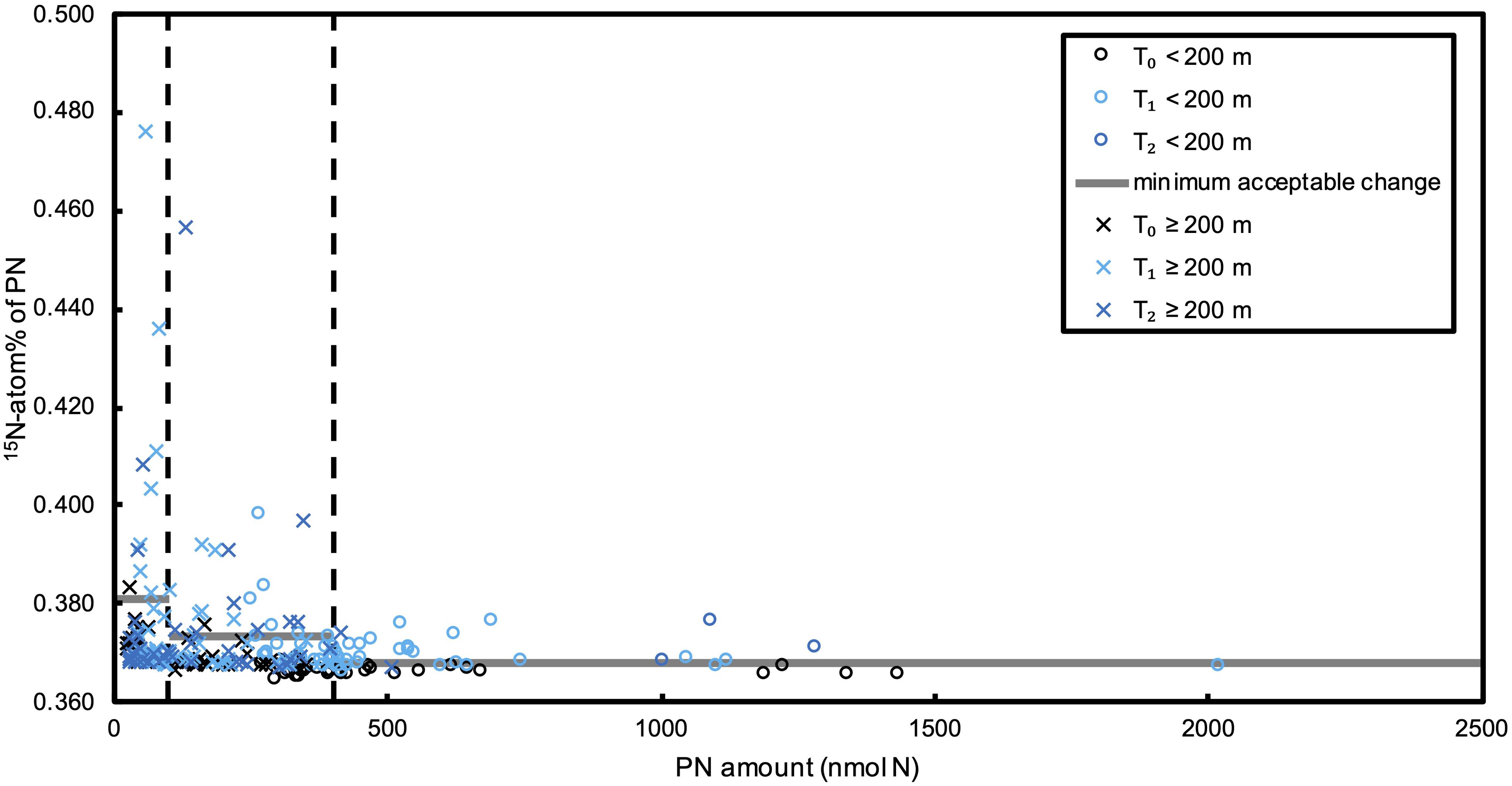
Figure 3. 15N-atom% of PN in N2 fixation incubations at all incubation depths. The x-axis indicates the PN amount on the sample filters, and the vertical dashed lines indicate PN amount of 100 and 400 nmol N. Horizontal gray lines represent the minimum acceptable 15N-atom% change for different intervals.
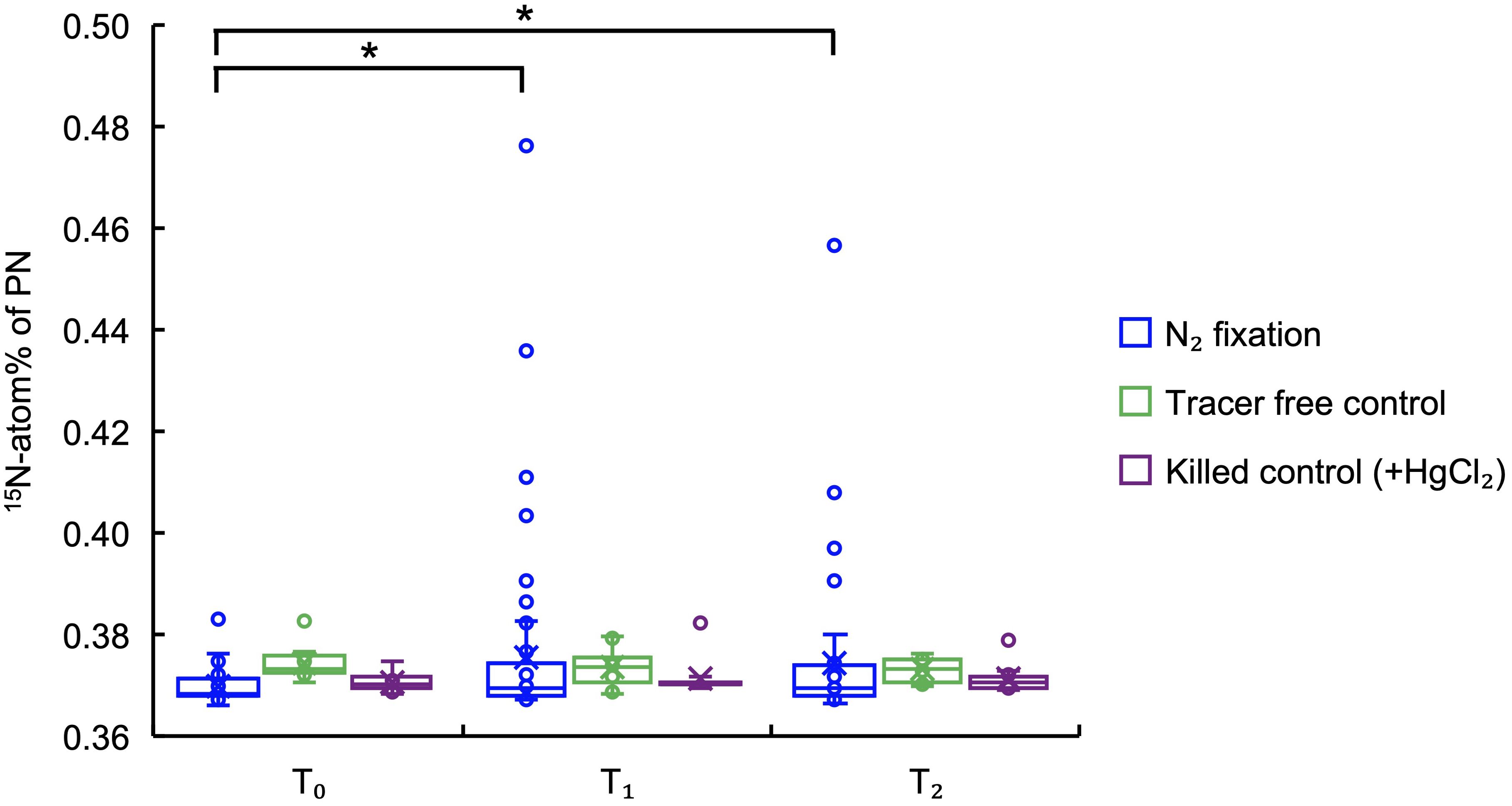
Figure 4. Box plot of 15N-atom% of PN ≥200 m under N2 fixation (blue), tracer free control (green), and killed control (+HgCl2, purple) incubations. Black solid lines with asterisks (*) on top represent statistically significantly different groups, i.e. T1s and T2s in N2 fixation incubations are significantly different from T0s (t-Test: samples assuming unequal variances, p < 0.05).
3.2 Mesopelagic N2 fixation shows great heterogeneity
With detectable mesopelagic N2 fixation signals revealed by 15N-atom% of PN, we also observed great variation in those signals. The 15N-atom% of PN in T1 and T2 samples are obviously more variable than those in control experiments, while the variation in T0s are similar across different treatments (Figure 4), being indicative of highly variable mesopelagic N2 fixation between different stations, depths, and even between the replicated samples collected from the same depths. Of course, the difference between stations might be also partly affected by the different incubation settings between the two cruises, while variabilities within replicates and between depths are not subject to such incubation differences. It is worth noting that most of the 15N-atom% change of the mesopelagic samples after incubation were still within the minimum acceptable value, suggesting sporadic occurrence of N2 fixation. However, while 15N-atom% of PN in T1 and T2 are significantly higher than those in T0 in N2 fixation incubations, we observed no significant difference between 15N-atom% of PN in T1 and T2 (Figure 4). This is also likely due to the heterogeneity in the abundance and composition of N2-fixing microorganisms, which results in large variation of 15N-atom% in both T1 and T2 samples, and leads to a lack of significant differences in the time series incubation. The high heterogeneity of mesopelagic N2 fixation observed here is also consistent with previous observations showing high heterogeneity of marine particles in composition, which may provide variable conditions for N2 fixation (Iversen and Lampitt, 2020; Ho et al., 2022; Comstock et al., 2024). Based on the high variation in 15N-atom% between the replicated samples, we further speculate uneven distribution, community composition and activities of N2-fixing microorganisms in mesopelagic waters.
We then quantified the frequency of samples showing significant increase of 15N-atom% in replicates after incubations to see whether there are any vertical patterns. Generally, the result showed that samples from <200 m depths had a higher possibility of catching N2 fixing signals compared to greater depths (≥200 m, Figure 5). This could be due to the presence of cyanobacterial diazotrophs (e.g. UCYN-B) that fix nitrogen in the dark or non-cyanobacterial diazotrophs that do not directly require light for energy or both (Chen et al., 2019; Turk-Kubo et al., 2023; Masuda et al., 2024). Only between 15 - 75 m (corresponding to the depth range with highest N2 fixation in the South China Sea; Lu et al., 2019; Wen et al., 2022) did we find incubations in which all of the replicates showed detectable N2 fixation. However, even in the euphotic zone, there were many depths at which some of the replicates showed no detectable N2 fixation, reinforcing that the heterogeneity of N2 fixation extends to the euphotic zone. The lack of detectable N2 fixation in some euphotic zone incubations may also be due to dark incubation conditions limiting autotrophic N2 fixation by cyanobacteria, or the low isotope sensitivity caused by high PN concentrations (White et al., 2020). In fact, at 5 m in WXS station, the only <200 m depth time course, we observed significant linear production of 15N-PN from N2 fixation even though only T2 replicates passed the minimum acceptable change (Supplementary Figure 3). This suggests that our conservative calculation of the detection limit for N2 fixation may be masking measurable N2 fixation. At mesopelagic depths, no depth had detectable N2 fixation signals in >50% of replicates, while we consistently observed N2 fixation signals in 1 - 3 of the 4 - 8 replicates (12.5 - 50%) between 200 m and 1000 m, except for 1000 m at SS1 station that had no replicate with N2 fixation signal. Interestingly, we observed that around 30% of replicates at most depths exhibited detectable N2 fixation signals, an observation, which was surprisingly stable regardless of the different methods and replicates we used. The stably low frequency of detectable N2 fixation signals, therefore, reveals the inherent nature of heterogeneity in mesopelagic N2 fixing microorganisms. It seems that no matter how many replicates we have, with the huge heterogeneity observed, the widely accepted quantification criteria, i.e. 10 times of the standard deviation of replicates, cannot be met (White et al., 2020). Unfortunately, we haven’t found a way yet to overcome this heterogeneity for robust rate calculation. However, with the recurrence of detectable N2 fixation signals, we suggest the occurrence of mesopelagic N2 fixation in the SCS despite that the rates were not quantifiable due to the large variation of 15N-atom% between the replicated samples. More studies are needed to understand the heterogeneity of mesopelagic N2 fixation and its causes, and further how to obtain representative rates under such heterogeneity. In contrast, below 1000 m (2000 m and 3800 m in SEATS station and 4000 m of SS1 station), only one sample showed an increase of 15N-atom% above the minimum acceptable change, implying no detectable dark N2 fixation at greater depths than 1000 m in the SCS. To date, N2 fixation at the deep ocean (>1000 m) was only observed in several studies conducted in the Eastern Tropical North Pacific (Selden et al., 2019), Eastern South Pacific (Bonnet et al., 2013; Shiozaki et al., 2023), Mediterranean Sea (Benavides et al., 2016), and the Bay of Bengal (Saxena et al., 2023).
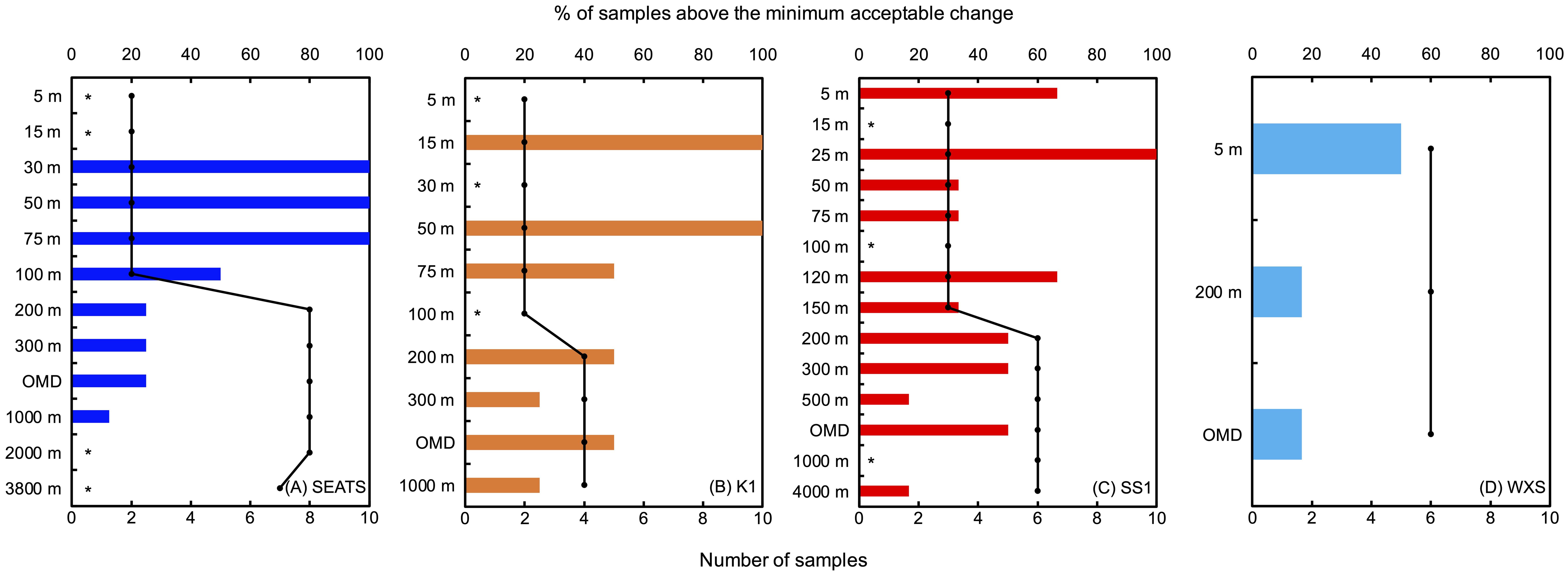
Figure 5. Proportion of 15N-atom% of PN after incubation above the minimum acceptable change in SEATS (A), K1 (B), SS1 (C) and WXS (D) stations (bar plots). Scatter plots indicate how many points were sampled after incubations, i.e. the sum of T1 and T2 (if sampled) replicates. Bar plots indicate the percentage of sampled 15N-atom% of PN surpassed the minimum acceptable change. Asterisks (*) indicate that all of the 15N-atom% of PN after incubations was below the minimum acceptable change (0%).
3.3 Global data compilation
To compare dark N2 fixation rates measured in the global ocean, we further compiled mesopelagic N2 fixation rates from 8 studies reported globally, which revealed a great heterogeneity across different geological regimes (Table 2; Figure 6). Reported N2 fixation rates ranged between below detection limit to 35.9 nmol N L-1 d-1 (Table 2), revealing great variability among studies and ocean basins. More than 80% of compiled N2 fixation rates were below 0.2 nmol N L-1 d-1 with around one third reported as below detection limit. High rates of >2 nmol N L-1 d-1 were observed occasionally in the Eastern Pacific (Figure 6), mostly associated with low oxygen conditions (Löscher et al., 2016; Selden et al., 2019). However, high rates are extremely patchy with the vast majority of mesopelagic N2 fixation rates in the oxygen minimum zones still comparable to those in the oxygenated areas. This indicates that the low oxygen condition favors the activity of diazotroph, but the occurrence of dark N2 fixation is likely regulated by multiple factors rather than oxygen only.
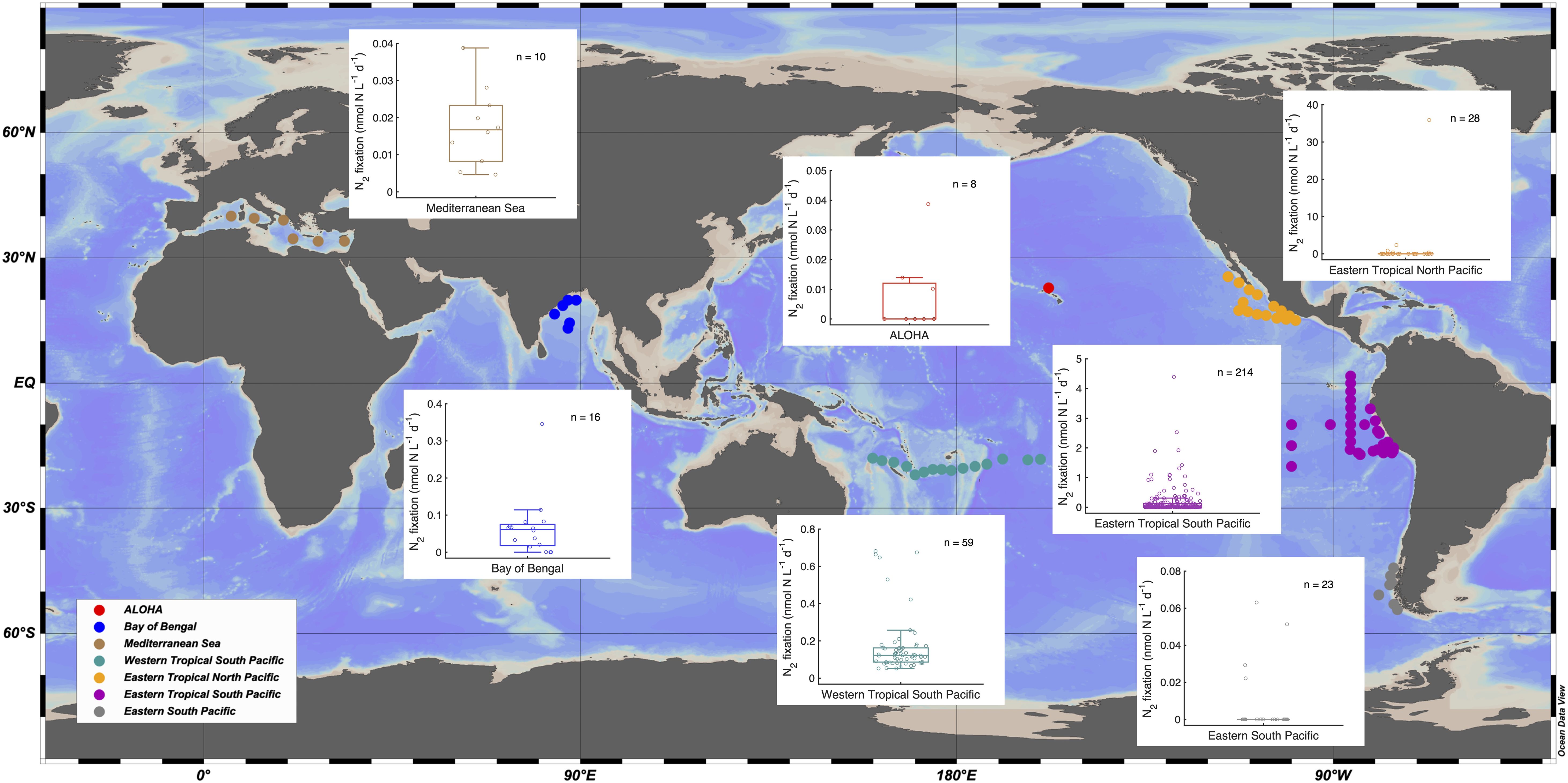
Figure 6. Compilation of globally reported mesopelagic N2 fixation rates shown in box plots with sampling stations plotted on the map. Data sources are indicated in 2.6. The numbers of data points included in each box plot are shown on the top right corner. It is worth noting that the N2 fixation rates in the ALOHA station were all below the minimum quantifiable rates (Gradoville et al., 2017).
Locally, reported mesopelagic N2 fixation rates can also vary across magnitudes even within a single study (Table 2). For example, the rates in the Eastern Tropical North Pacific varied from below detection limit to 35.9 nmol N L-1 d-1, yielding no statistical differences with any other regions in Figure 6, possibly due to the high variability. Localized heterogeneity in N2 fixation was also reported for epipelagic oceans, which might be caused by variabilities in environmental parameters (Messer et al., 2015; Selden et al., 2021a). Similarly, mesopelagic N2 fixation might also be regulated by various local diazotrophic communities and environmental conditions, such as sinking particles, bioavailability of dissolved organic matter, dissolved oxygen, and eddies (Löscher et al., 2016; Selden et al., 2019; Chakraborty et al., 2021). Therefore, heterogeneity of mesopelagic N2 fixation reveals the coincidence of N2 fixing microorganisms and the environmental conditions that allow them to fix N2.
Temporal dynamics of mesopelagic N2 fixation are seldomly reported due to limited observations. In terms of seasonal variabilities, Hamersley et al. (2011) reported highest mesopelagic N2 fixation rates in winter mixed period in Southern California Bight, while contrasting results were observed from Gulf of Aqaba and Levantine Basin with higher rates in the stratified periods than the mixed periods (Rahav et al., 2013, 2015). Interannual variabilities of mesopelagic N2 fixation were only reported for the most extensively studied site, Eastern Tropical South Pacific, which might be largely related to upwelling intensity (Selden et al., 2021b).
The great heterogeneity between studies might also come from the different methods used for rate determination (Selden et al., 2021b), as N2 fixation measurements developed rapidly during the past decades (White et al., 2020). Therefore, unifying and standardizing the measurements for future research are crucial for intercomparison of the results. Even though we were not able to apply, we are prone to the modified bubble method (Klawonn et al., 2015) as injecting large amounts of 15N2 as bubbles are applicable with minor disturbance to the samples and stable 15N2 enrichments throughout the incubation.
Even with low rates, mesopelagic N2 fixation holds the potential to contribute significantly to the global N budget when integrated with the large volume of mesopelagic oceans. For example, even though the rates in the Mediterranean Sea were all below 0.04 nmol N L-1 d-1, the integrated rates of mesopelagic N2 fixation were comparable to those in the euphotic zone (Benavides et al., 2016). However, the integrated rates are often obtained via a limited number of rate measurements integrated by vertically hundreds of meters. Even though the mesopelagic ocean is not as dynamic as the euphotic ocean, it is far from homogenous (Robinson et al., 2010; Baltar et al., 2012; Rigonato et al., 2023). Special care should be taken to avoid over- or under- extrapolation. Therefore, the best practice for obtaining representative rates and robust extrapolation into the global N budget under such heterogeneity needs to be established.
4 Conclusion and future perspectives
With carefully designed control incubations and sophisticated measurements, we were able to detect mesopelagic N2 fixation in the South China Sea down to 1000 m depth. We consistently observed that around 30% of the replicates at mesopelagic depths exhibited an N2 fixation signal, although the high variability prevented the quantification of representative rates. Detectable signals also present great variability across depths and stations. Global data compilation also shows regional and global heterogeneity in mesopelagic N2 fixation with variations of up to three orders of magnitude. Therefore, we conclude that heterogeneity is the inherent nature of mesopelagic N2 fixation, which prevents us from concrete rate determination as well as the extrapolation into integrated rates and global budgets.
Studies of mesopelagic N2 fixation are still scarce, which limits our understanding of global mesopelagic N2 fixation. Most of the studies are from the Pacific Ocean, and there are, as yet, no such studies carried out in the Atlantic ocean and polar regions. The temporal and spatial distribution of mesopelagic N2 fixation is still poorly understood. Future research should explore mesopelagic N2 fixation in not yet covered geographical areas, and further focus on the temporal variabilities in regions of high rates, which will shed more light on the pervasiveness of mesopelagic N2 fixation globally. In addition, coastal waters undergoing intensified deoxygenation (Breitburg et al., 2018; Zhang et al., 2023) may also be a new niche for non-cyanobacterial diazotroph. Further, developing standardized methods and calculations is also of urgent need to comprehend the heterogeneous rates and develop reliable budgets of mesopelagic N2 fixation globally. On the other hand, the mechanisms and environmental factors influencing mesopelagic N2 fixation remain to be elucidated. For example, dissolved organic matter has been assumed to affect mesopelagic N2 fixation, while the results are not consistent between different studies and the underlying mechanism remains hard to reconcile (Benavides et al., 2015; Selden et al., 2019). Lastly, further studies should be conducted to establish the link between observed rates and corresponding diazotrophs.
Data availability statement
The original contributions presented in the study are included in the article/Supplementary Material. Further inquiries can be directed to the corresponding author.
Author contributions
SW: Writing – review & editing, Writing – original draft, Methodology, Investigation, Formal analysis, Conceptualization. XW: Writing – review & editing, Supervision, Conceptualization. MD: Writing – review & editing, Formal analysis. XC: Writing – review & editing, Investigation. CS: Writing – review & editing, Investigation. MB: Writing – review & editing, Investigation. SB: Writing – review & editing, Investigation. CL: Writing – review & editing, Investigation. MRH: Writing – review & editing, Investigation. MM: Writing – review & editing, Investigation. XY: Writing – review & editing, Investigation. SK: Writing – review & editing, Supervision, Resources, Funding acquisition, Conceptualization.
Funding
The author(s) declare financial support was received for the research, authorship, and/or publication of this article. This study was supported by the National Natural Science Foundation of China (NSFC no. 92058204, 92258302). SW is also supported by the Ph.D. Fellowship of the State Key Laboratory of Marine Environmental Science at Xiamen University and the China Scholarship Council.
Acknowledgments
Many thanks to all the cruise members on R/V Tan Kah Kee during Aug 2018 and Jul 2017 cruises (KK1806 and KK1905). Special thanks to Gaute Lavik and Wiebke Mohr for their helpful and inspiring discussions on this manuscript, Zhixiong Huang for EA-IRMS standard data, Wenbin Zou and Li Tian for experimental help. Our dataset has appeared as a preprint in Biogeosciences Discussions (Wu et al., 2021), while we’ve already withdrawn the preprint in 2021 and completely redesigned and rewrote the manuscript. We also appreciate the handling editor, XL, and two reviewers for their constructive comments.
Conflict of interest
The authors declare that the research was conducted in the absence of any commercial or financial relationships that could be construed as a potential conflict of interest.
The author(s) declared that they were an editorial board member of Frontiers, at the time of submission. This had no impact on the peer review process and the final decision.
Publisher’s note
All claims expressed in this article are solely those of the authors and do not necessarily represent those of their affiliated organizations, or those of the publisher, the editors and the reviewers. Any product that may be evaluated in this article, or claim that may be made by its manufacturer, is not guaranteed or endorsed by the publisher.
Supplementary material
The Supplementary Material for this article can be found online at: https://www.frontiersin.org/articles/10.3389/fmars.2024.1495649/full#supplementary-material
References
Baltar F., Arístegui J., Gasol J. M., Herndl G. J. (2012). Microbial functioning and community structure variability in the mesopelagic and epipelagic waters of the subtropical northeast Atlantic Ocean. Appl. Environ. Microbiol. 78, 3309–3316. doi: 10.1128/aem.07962-11
Benavides M., Bonnet S., Berman-Frank I., Riemann L. (2018a). Deep into oceanic N2 fixation. Front. Mar. Sci. 5. doi: 10.3389/fmars.2018.00108
Benavides M., Bonnet S., Hernández N., Martínez-Pérez A. M., Nieto-Cid M., Álvarez-Salgado X. A., et al. (2016). Basin-wide N2 fixation in the deep waters of the Mediterranean Sea. Glob. Biogeochem. Cycles 30, 952–961. doi: 10.1002/2015gb005326
Benavides M., Moisander P. H., Berthelot H., Dittmar T., Grosso O., Bonnet S. (2015). Mesopelagic N2 fixation related to organic matter composition in the Solomon and Bismarck Seas (Southwest Pacific). PloS One 10, e0143775. doi: 10.1371/journal.pone.0143775
Benavides M., Shoemaker K. M., Moisander P. H., Niggemann J., Dittmar T., Duhamel S., et al. (2018b). Aphotic N2 fixation along an oligotrophic to ultraoligotrophic transect in the western tropical South Pacific Ocean. Biogeosciences 15, 3107–3119. doi: 10.5194/bg-15-3107-2018
Bentzon-Tilia M., Severin I., Hansen L. H., Riemann L. (2015). Genomics and ecophysiology of heterotrophic nitrogen-fixing bacteria isolated from estuarine surface water. mBio 6, e00929–e00915. doi: 10.1128/mbio.00929-15
Bombar D., Paerl R. W., Anderson R., Riemann L. (2018). Filtration via conventional glass fiber filters in 15N2 tracer assays fails to capture all nitrogen-fixing prokaryotes. Front. Mar. Sci. 5. doi: 10.3389/fmars.2018.00006
Bombar D., Paerl R. W., Riemann L. (2016). Marine non-cyanobacterial diazotrophs: moving beyond molecular detection. Trends Microbiol. 24, 916–927. doi: 10.1016/j.tim.2016.07.002
Bonnet S., Dekaezemacker J., Turk-Kubo K. A., Moutin T., Hamersley R. M., Grosso O., et al. (2013). Aphotic N2 fixation in the eastern tropical South Pacific Ocean. PloS One 8, e81265. doi: 10.1371/journal.pone.0081265
Braman R. S., Hendrix S. A. (1989). Nanogram nitrite and nitrate determination in environmental and biological materials by vanadium(III) reduction with chemiluminescence detection. Anal. Chem. 61, 2715–2718. doi: 10.1021/ac00199a007
Breitburg D., Levin L. A., Oschlies A., Grégoire M., Chavez F. P., Conley D. J., et al. (2018). Declining oxygen in the global ocean and coastal waters. Science 359, eaam7240. doi: 10.1126/science.aam7240
Casciotti K. L., Sigman D. M., Hastings M. G., Böhlke J. K., Hilkert A. (2002). Measurement of the oxygen isotopic composition of nitrate in seawater and freshwater using the denitrifier method. Anal. Chem. 74, 4905–4912. doi: 10.1021/ac020113w
Chakraborty S., Andersen K. H., Visser A. W., Inomura K., Follows M. J., Riemann L. (2021). Quantifying nitrogen fixation by heterotrophic bacteria in sinking marine particles. Nat. Commun. 12, 4085. doi: 10.1038/s41467-021-23875-6
Chang B. X., Jayakumar A., Widner B., Bernhardt P., Mordy C. W., Mulholland M. R., et al. (2019). Low rates of dinitrogen fixation in the eastern tropical South Pacific. Limnol. Oceanogr. 64, 1913–1923. doi: 10.1002/lno.11159
Chen T.-Y., Chen Y. L., Sheu D.-S., Chen H.-Y., Lin Y.-H., Shiozaki T. (2019). Community and abundance of heterotrophic diazotrophs in the northern South China Sea: Revealing the potential importance of a new alphaproteobacterium in N2 fixation. Deep Sea Res. Part : Oceanogr. Res. Pap. 143, 104–114. doi: 10.1016/j.dsr.2018.11.006
Comstock J., Henderson L. C., Close H. G., Liu S., Vergin K., Worden A. Z., et al. (2024). Marine particle size-fractionation indicates organic matter is processed by differing microbial communities on depth-specific particles. ISME Commun. 4 (1), ycae090. doi: 10.1093/ismeco/ycae090
Dekaezemacker J., Bonnet S., Grosso O., Moutin T., Bressac M., Capone D. G. (2013). Evidence of active dinitrogen fixation in surface waters of the eastern tropical South Pacific during El Niño and La Niña events and evaluation of its potential nutrient controls. Glob. Biogeochem. Cycles 27, 768–779. doi: 10.1002/gbc.20063
Dekas A. E., Poretsky R. S., Orphan V. J. (2009). Deep-Sea Archaea fix and share nitrogen in methane-consuming microbial consortia. Science 326, 422–426. doi: 10.1126/science.1178223
Delmont T. O., Karlusich J. J. P., Veseli I., Fuessel J., Eren A. M., Foster R. A., et al. (2022). Heterotrophic bacterial diazotrophs are more abundant than their cyanobacterial counterparts in metagenomes covering most of the sunlit ocean. ISME J. 16, 927–936. doi: 10.1038/s41396-021-01135-1
Falkowski P. G. (1983). Nitrogen in the marine environment. IV: Methods, 839–868. doi: 10.1016/b978-0-12-160280-2.50031-6
Farnelid H., Bentzon-Tilia M., Andersson A. F., Bertilsson S., Jost G., Labrenz M., et al. (2013). Active nitrogen-fixing heterotrophic bacteria at and below the chemocline of the central Baltic Sea. ISME J. 7, 1413–1423. doi: 10.1038/ismej.2013.26
Farnelid H., Turk-Kubo K., Ploug H., Ossolinski J. E., Collins J. R., Mooy B. A. S. V., et al. (2018). Diverse diazotrophs are present on sinking particles in the North Pacific Subtropical Gyre. ISME J. 13, 170–182. doi: 10.1038/s41396-018-0259-x
Fernandez C., Farías L., Ulloa O. (2011). Nitrogen fixation in denitrified marine waters. PLoS One 6, e20539. doi: 10.1371/journal.pone.0020539
Gradoville M. R., Bombar D., Crump B. C., Letelier R. M., Zehr J. P., White A. E. (2017). Diversity and activity of nitrogen-fixing communities across ocean basins. Limnol. Oceanogr. 62, 1895–1909. doi: 10.1002/lno.10542
Großkopf T., LaRoche J. (2012). Direct and indirect costs of dinitrogen fixation in crocosphaera watsonii WH8501 and possible implications for the nitrogen cycle. Front. Microbiol. 3. doi: 10.3389/fmicb.2012.00236
Hamersley M., Turk K., Leinweber A., Gruber N., Zehr J., Gunderson T., et al. (2011). Nitrogen fixation within the water column associated with two hypoxic basins in the Southern California Bight. Aquat. Microb. Ecol. 63, 193–205. doi: 10.3354/ame01494
Harding K. J., Turk-Kubo K. A., Mak E. W. K., Weber P. K., Mayali X., Zehr J. P. (2022). Cell-specific measurements show nitrogen fixation by particle-attached putative non-cyanobacterial diazotrophs in the North Pacific Subtropical Gyre. Nat. Commun. 13, 6979. doi: 10.1038/s41467-022-34585-y
Ho Q. N., Fettweis M., Spencer K. L., Lee B. J. (2022). Flocculation with heterogeneous composition in water environments: A review. Water Res. 213, 118147. doi: 10.1016/j.watres.2022.118147
Iversen M. H., Lampitt R. S. (2020). Size does not matter after all: No evidence for a size-sinking relationship for marine snow. Prog. Oceanogr. 189, 102445. doi: 10.1016/j.pocean.2020.102445
Jickells T. D., Buitenhuis E., Altieri K., Baker A. R., Capone D., Duce R. A., et al. (2017). A reevaluation of the magnitude and impacts of anthropogenic atmospheric nitrogen inputs on the ocean. Glob. Biogeochem. Cycles 31, 289–305. doi: 10.1002/2016gb005586
Karl D., Michaels A., Bergman B., Capone D., Carpenter E., Letelier R., et al. (2002). Dinitrogen fixation in the world’s oceans. Biogeochemistry 57–58, 47–98. doi: 10.1023/a:1015798105851
Klawonn I., Lavik G., Böning P., Marchant H. K., Dekaezemacker J., Mohr W., et al. (2015). Simple approach for the preparation of 15–15N2-enriched water for nitrogen fixation assessments: evaluation, application and recommendations. Front. Microbiol. 6. doi: 10.3389/fmicb.2015.00769
Knapp A. N., Sigman D. M., Lipschultz F. (2005). N isotopic composition of dissolved organic nitrogen and nitrate at the Bermuda Atlantic Time-series Study site. Glob. Biogeochem. Cycles 19. doi: 10.1029/2004gb002320
Loescher C. R., Großkopf T., Desai F. D., Gill D., Schunck H., Croot P. L., et al. (2014). Facets of diazotrophy in the oxygen minimum zone waters off Peru. ISME J. 8, 2180–2192. doi: 10.1038/ismej.2014.71
Löscher C. R., Bourbonnais A., Dekaezemacker J., Charoenpong C. N., Altabet M. A., Bange H. W., et al. (2016). N2 fixation in eddies of the eastern tropical South Pacific Ocean. Biogeosciences 13, 2889–2899. doi: 10.5194/bg-13-2889-2016
Lu Y., Wen Z., Shi D., Chen M., Zhang Y., Bonnet S., et al. (2017). Effect of light on N2 fixation and net nitrogen release of Trichodesmium in a field study. Biogeosciences 15, 1–12. doi: 10.5194/bg-15-1-2018
Lu Y., Wen Z., Shi D., Lin W., Bonnet S., Dai M., et al. (2019). Biogeography of N2 fixation influenced by the western boundary current intrusion in the South China Sea. J. Geophys. Res.: Oceans 124, 6983–6996. doi: 10.1029/2018jc014781
Masuda T., Mareš J., Shiozaki T., Inomura K., Fujiwara A., Prášil O. (2024). Crocosphaera watsonii – A widespread nitrogen-fixing unicellular marine cyanobacterium. J. Phycol. 60, 604–620. doi: 10.1111/jpy.13450
McIlvin M. R., Casciotti K. L. (2011). Technical updates to the bacterial method for nitrate isotopic analyses. Anal. Chem. 83, 1850–1856. doi: 10.1021/ac1028984
Messer L. F., Mahaffey C., Robinson C. M., Jeffries T. C., Baker K. G., Isaksson J. B., et al. (2015). High levels of heterogeneity in diazotroph diversity and activity within a putative hotspot for marine nitrogen fixation. ISME J. 10, 1499–1513. doi: 10.1038/ismej.2015.205
Mohr W., Großkopf T., Wallace D. W. R., LaRoche J. (2010). Methodological underestimation of oceanic nitrogen fixation rates. PloS One 5, e12583. doi: 10.1371/journal.pone.0012583
Moisander P. H., Beinart R. A., Voss M., Zehr J. P. (2008). Diversity and abundance of diazotrophic microorganisms in the South China Sea during intermonsoon. ISME J. 2, 954–967. doi: 10.1038/ismej.2008.51
Moisander P. H., Benavides M., Bonnet S., Berman-Frank I., White A. E., Riemann L. (2017). Chasing after non-cyanobacterial nitrogen fixation in marine pelagic environments. Front. Microbiol. 8. doi: 10.3389/fmicb.2017.01736
Montoya J. P., Voss M., Kahler P., Capone D. G. (1996). A simple, high-precision, high-sensitivity tracer assay for N(inf2) fixation. Appl. Environ. Microbiol. 62, 986–993. doi: 10.1128/aem.62.3.986-993.1996
Mulholland M. R., Bernhardt P. W., Widner B. N., Selden C. R., Chappell P. D., Clayton S., et al. (2019). High rates of N2 fixation in temperate, western North Atlantic coastal waters expand the realm of marine diazotrophy. Glob. Biogeochem. Cycles 33, 826–840. doi: 10.1029/2018gb006130
Paerl H. W., Prufert L. E. (1987). Oxygen-poor microzones as potential sites of microbial N 2 fixation in nitrogen-depleted aerobic marine waters. Appl. Environ. Microbiol. 53, 1078–1087. doi: 10.1128/aem.53.5.1078-1087.1987
Rahav E., Bar-Zeev E., Ohayon S., Elifantz H., Belkin N., Herut B., et al. (2013). Dinitrogen fixation in aphotic oxygenated marine environments. Front. Microbiol. 4. doi: 10.3389/fmicb.2013.00227
Rahav V., Herut B., Mulholland M., Belkin N., Elifantz H., Berman-Frank I. (2015). Heterotrophic and autotrophic contribution to dinitrogen fixation in the Gulf of Aqaba. Mar. Ecol. Prog. Ser. 522, 67–77. doi: 10.3354/meps11143
Riemann L., Rahav E., Passow U., Grossart H.-P., de Beer D., Klawonn I., et al. (2022). Planktonic aggregates as hotspots for heterotrophic diazotrophy: the plot thickens. Front. Microbiol. 13. doi: 10.3389/fmicb.2022.875050
Rigonato J., Budinich M., Murillo A. A., Brandão M. C., Karlusich J. J. P., Soviadan Y. D., et al. (2023). Ocean-wide comparisons of mesopelagic planktonic community structures. ISME Commun. 3, 83. doi: 10.1038/s43705-023-00279-9
Robinson C., Steinberg D. K., Anderson T. R., Arístegui J., Carlson C. A., Frost J. R., et al. (2010). Mesopelagic zone ecology and biogeochemistry – a synthesis. Deep Sea Res. Part II: Top. Stud. Oceanogr. 57, 1504–1518. doi: 10.1016/j.dsr2.2010.02.018
Saxena H., Sahoo D., Nazirahmed S., Chaudhari D., Rahi P., Kumar S., et al. (2023). The Bay of Bengal: an enigmatic diazotrophic niche. J. Geophys. Res.: Biogeosciences 128. doi: 10.1029/2023jg007687
Selden C. R., Chappell P. D., Clayton S., Macías-Tapia A., Bernhardt P. W., Mulholland M. R. (2021a). A coastal N2 fixation hotspot at the Cape Hatteras front: Elucidating spatial heterogeneity in diazotroph activity via supervised machine learning. Limnol. Oceanogr. 66, 1832–1849. doi: 10.1002/lno.11727
Selden C. R., Mulholland M. R., Bernhardt P. W., Widner B., Macías-Tapia A., Ji Q., et al. (2019). Dinitrogen fixation across physico-chemical gradients of the eastern tropical North Pacific oxygen deficient zone. Glob. Biogeochem. Cycles 33, 1187–1202. doi: 10.1029/2019gb006242
Selden C. R., Mulholland M. R., Widner B., Bernhardt P., Jayakumar A. (2021b). Toward resolving disparate accounts of the extent and magnitude of nitrogen fixation in the Eastern Tropical South Pacific oxygen deficient zone. Limnol. Oceanogr. 66, 1950–1960. doi: 10.1002/lno.11735
Shiozaki T., Hirai M., Kondo F., Sato T., Sato M., Iriarte J. L., et al. (2023). Heterogeneous diazotroph communities in the subtropical-subantarctic transition and aphotic zones off the coast of Patagonia, eastern South Pacific Ocean. J. Geophys. Res.: Biogeosciences 128. doi: 10.1029/2023jg007683
Shiozaki T., Nagata T., Ijichi M., Furuya K. (2015). Nitrogen fixation and the diazotroph community in the temperate coastal region of the northwestern North Pacific. Biogeosciences 12, 4751–4764. doi: 10.5194/bg-12-4751-2015
Sigman D. M., Casciotti K. L., Andreani M., Barford C., Galanter M., Böhlke J. K. (2001). A bacterial method for the nitrogen isotopic analysis of nitrate in seawater and freshwater. Anal. Chem. 73, 4145–4153. doi: 10.1021/ac010088e
Tschitschko B., Esti M., Philippi M., Kidane A. T., Littmann S., Kitzinger K., et al. (2024). Rhizobia–diatom symbiosis fixes missing nitrogen in the ocean. Nature 630, 899–904. doi: 10.1038/s41586-024-07495-w
Turk-Kubo K. A., Gradoville M. R., Cheung S., Cornejo-Castillo F. M., Harding K. J., Morando M., et al. (2023). Non-cyanobacterial diazotrophs: global diversity, distribution, ecophysiology, and activity in marine waters. FEMS Microbiol. Rev. 47, fuac046. doi: 10.1093/femsre/fuac046
Wannicke N., Benavides M., Dalsgaard T., Dippner J. W., Montoya J. P., Voss M. (2018). New perspectives on nitrogen fixation measurements using 15N2 gas. Front. Mar. Sci. 5. doi: 10.3389/fmars.2018.00120
Weber S. C. (2015). From Rivers to Natural Gas: the Influence of Allochthonous Inputs on Marine Nitrogen Fixation and the Carbon Cycle. dissertation. (Georgia Institute of Technology).
Wen Z., Browning T. J., Dai R., Wu W., Li W., Hu X., et al. (2022). The response of diazotrophs to nutrient amendment in the South China Sea and western North Pacific. Biogeosciences 19, 5237–5250. doi: 10.5194/bg-19-5237-2022
White A. E., Granger J., Selden C., Gradoville M. R., Potts L., Bourbonnais A., et al. (2020). A critical review of the 15N2 tracer method to measure diazotrophic production in pelagic ecosystems. Limnol. Oceanogr.: Methods 18, 129–147. doi: 10.1002/lom3.10353
Wu S., Du M., Wan X. S., Selden C., Benavides M., Bonnet S., et al. (2021). Insights into nitrogen fixation below the euphotic zone: trials in an oligotrophic marginal sea and global compilation. Biogeosciences Discuss. 2021, 1–31. doi: 10.5194/bg-2021-104
Wu S., Yan X., Tang J.-M., Tan E., Luo L., Tong S., et al. (2024). Nitrogen cycling in China marginal seas: Progress and challenges. Mar. Chem. 265, 104421. doi: 10.1016/j.marchem.2024.104421
Yan X., Yang J.-Y. T., Xu M. N., Wang H., Dai M., Kao S.-J. (2022). Nitrogen isotope constraint on the zonation of multiple transformations between dissolved and particulate organic nitrogen in the Changjiang plume. Sci. Total Environ. 818, 151678. doi: 10.1016/j.scitotenv.2021.151678
Zehr J. P., Capone D. G. (1996). Problems and promises of assaying the genetic potential for nitrogen fixation in the marine environment. Microb. Ecol. 32, 263–281. doi: 10.1007/bf00183062
Zehr J. P., Capone D. G. (2020). Changing perspectives in marine nitrogen fixation. Science 368, eaay9514. doi: 10.1126/science.aay9514
Zehr J. P., Kudela R. M. (2011). Nitrogen cycle of the open ocean: from genes to ecosystems. Annu. Rev. Mar. Sci. 3, 197–225. doi: 10.1146/annurev-marine-120709-142819
Zehr J. P., Mellon M. T., Zani S. (1998). New nitrogen-fixing microorganisms detected in oligotrophic oceans by amplification of nitrogenase ( nifH ) genes. Appl. Environ. Microbiol. 64, 3444–3450. doi: 10.1128/aem.64.9.3444-3450.1998
Zhang X., Yao C., Zhang B., Tan W., Gong J., Wang G., et al. (2023). Dynamics of benthic nitrate reduction pathways and associated microbial communities responding to the development of seasonal deoxygenation in a coastal mariculture zone. Environ. Sci. Technol. 57, 15014–15025. doi: 10.1021/acs.est.3c03994
Keywords: nitrogen fixation, mesopelagic zone, heterogeneity, South China Sea, global compilation
Citation: Wu S, Wan XS, Du M, Chen X, Selden CR, Benavides M, Bonnet S, Löscher CR, Hamersley MR, Mulholland MR, Yan X and Kao S-J (2024) Heterogeneity of nitrogen fixation in the mesopelagic zone of the South China Sea. Front. Mar. Sci. 11:1495649. doi: 10.3389/fmars.2024.1495649
Received: 13 September 2024; Accepted: 15 October 2024;
Published: 11 November 2024.
Edited by:
Xianbiao Lin, Ocean University of China, ChinaReviewed by:
Zhibing Jiang, Ministry of Natural Resources, ChinaZongxiao Zhang, Xinjiang Normal University, China
Copyright © 2024 Wu, Wan, Du, Chen, Selden, Benavides, Bonnet, Löscher, Hamersley, Mulholland, Yan and Kao. This is an open-access article distributed under the terms of the Creative Commons Attribution License (CC BY). The use, distribution or reproduction in other forums is permitted, provided the original author(s) and the copyright owner(s) are credited and that the original publication in this journal is cited, in accordance with accepted academic practice. No use, distribution or reproduction is permitted which does not comply with these terms.
*Correspondence: Shuh-Ji Kao, c2prYW9AaGFpbmFudS5lZHUuY24=