- Department of Estuarine & Delta Systems, Netherlands Institute for Sea Research (NIOZ) Royal Institute for Sea Research, Texel, Netherlands
In ecology, the enumeration of living aquatic unicellular protists is a century-old complicated task. The organisms are small, perhaps motile, and if they are non-motile their vitality is hard to gauge. A respectable technique still in use today is the Serial Dilution Culture-Most Probable Number (MPN) assay that determines the total viable phytoplankton concentration from the highest sample dilutions that exhibit chlorophyll-fluorescence after incubation. However, 99% of extant phytoplankton species have not been shown to grow in MPN tubes (false-negatives) and in ballast water applications, 10-50 µm species can be outcompeted by <10 µm autotrophs in abundance, biomass, and chlorophyll-fluorescence (false-positives). In addition, it is shown that after microscopic identification, contrary to established practice, the concentration of individual species or species-groups (10-50 µm) cannot be derived from standard MPN tables. Examples of a corrected derivation are given for simple (2-3 species) assemblages, but this correction becomes increasingly difficult in more complex mixtures. The MPN assay should not be used as a quantitative method in ecological studies or in applications such as ballast water testing.
1 Introduction
In ecological studies, establishing if an aquatic unicellular protist is dead or alive is a difficult task. Microscopic motile organisms may stop moving but even then, just as non-motile protists, it is difficult to ascertain if the organism is still alive by direct optical observation. Unless there is obvious damage, stains must be used as a sign of vitality. Such vitality probes have been tested using living and killed phytoplankton using microscopy or flow cytometry (Veldhuis et al., 2001; Steinberg et al., 2012). They are indicative of death, such as cell membrane permeability or life, such as intracellular enzyme activity. Unfortunately, there is not one vital stain capable of probing all phytoplankton species. Their fluorescence intensity may be difficult or too low to detect, green-fluorescent detritus may be confusing, and the green fluorescence of the most commonly used probes as SYTOX and FDA can be mistaken with the green autofluorescence some species exhibit (Peperzak and Brussaard, 2011; Steinberg et al., 2012; Lundgreen et al., 2019; Tang et al., 2022).
To reduce the global spread of harmful aquatic organisms, ships’ ballast water needs to be treated to reduce the abundances of living organisms in the discharged water to below an international standard concentration determined by the International Maritime Organization (IMO) and the United States Coast Guard (USCG) (USCG, 2012; IMO, 2018). Ballast water treatment consists of two major groups of technologies. Chemical treatment such as by chlorine delivers fast death, rapidly reduces the concentration of organisms and the intact ones are, due to the dissolvement of detritus, relatively easy identified by vital stains (Stehouwer et al., 2015). UV treatment leads to direct killing at high doses, and cell and DNA damage but also possible repair at lower doses (Helbling et al., 2001; Sinha and Häder, 2002). UV-treated organisms may retain the capability to enzymatically transform the widely used stains FDA and CMFDA, produce green fluorescence and appear vital, while they may not be viable due to treatment-induced DNA damage (First and Drake, 2014; MacIntyre et al., 2018). In that case, they too are no longer able to multiply and cause harmful effects and the goal of treatment has been achieved (Cullen and MacIntyre, 2016; MacIntyre and Cullen, 2016; IMO, 2019).
Aquatic organisms in ballast water are classified by size. The vitality of the largest (>50 µm in “minimum dimension”) organisms is determined by microscopic observation of movement. The second class has a size of ≥10 to 50 µm (10-50 µm) with the lower size limit criticized because the <10 µm size class also contains harmful eukaryotes as well as viruses (Gollasch et al., 2007, 2012; Lundgreen et al., 2019). Both IMO and USCG allow microscopic vital stain analysis of this class, but only IMO has approved the Serial Dilution Culture-Most Probable Number (SDC-MPN or MPN) as viability method for 10-50 µm organisms (IMO, 2019). As will be shown, using MPN for size-restricted phytoplankton results in an increased risk of false-positives. The <10 µm regulations only limit three indicator bacteria.
MPN is a form of grow-out assay in stepwise dilution series that involves weeks of incubation aimed at growing and detecting the viable organisms (Stehouwer et al., 2015; IMO, 2019). The original purpose of MPN was to provide material for the isolation, identification and enumeration of bloom forming species and the non-preservable fraction of the phytoplankton (Allen, 1919; Knight-Jones, 1951; Throndsen, 1978; Andersen and Throndsen, 2003). MPN was not intended to measure the abundance of specific plankton size classes.
The IMO MPN assay aims to detect the chlorophyll-fluorescence of reproducing autotrophic 10-50 µm organisms. The most abundant species is expected to grow in the highest dilution where in two weeks’ time even one viable cell grows sufficiently to pass the fluorescence detection limit. The standard scenario (IMO, 2019) is as follows: make 10-fold dilutions of the sample, take five draws of 1 mL from each dilution, and mix each draw with a nutrient culture medium in tubes, then incubate all tubes in the light to stimulate growth (Figure 1). The assumption is made that species are homogeneously distributed in the dilutions, that they are randomly selected and pipetted into tubes and that all viable cells will grow under the culture conditions offered. The last assumption led Andersen and Throndsen (2003) to note that MPN will deliver minimum values because different species may have various culture requirements. Allen (1919) who performed the first dilution culture assay already commented that many species could not be cultured under the conditions he offered.
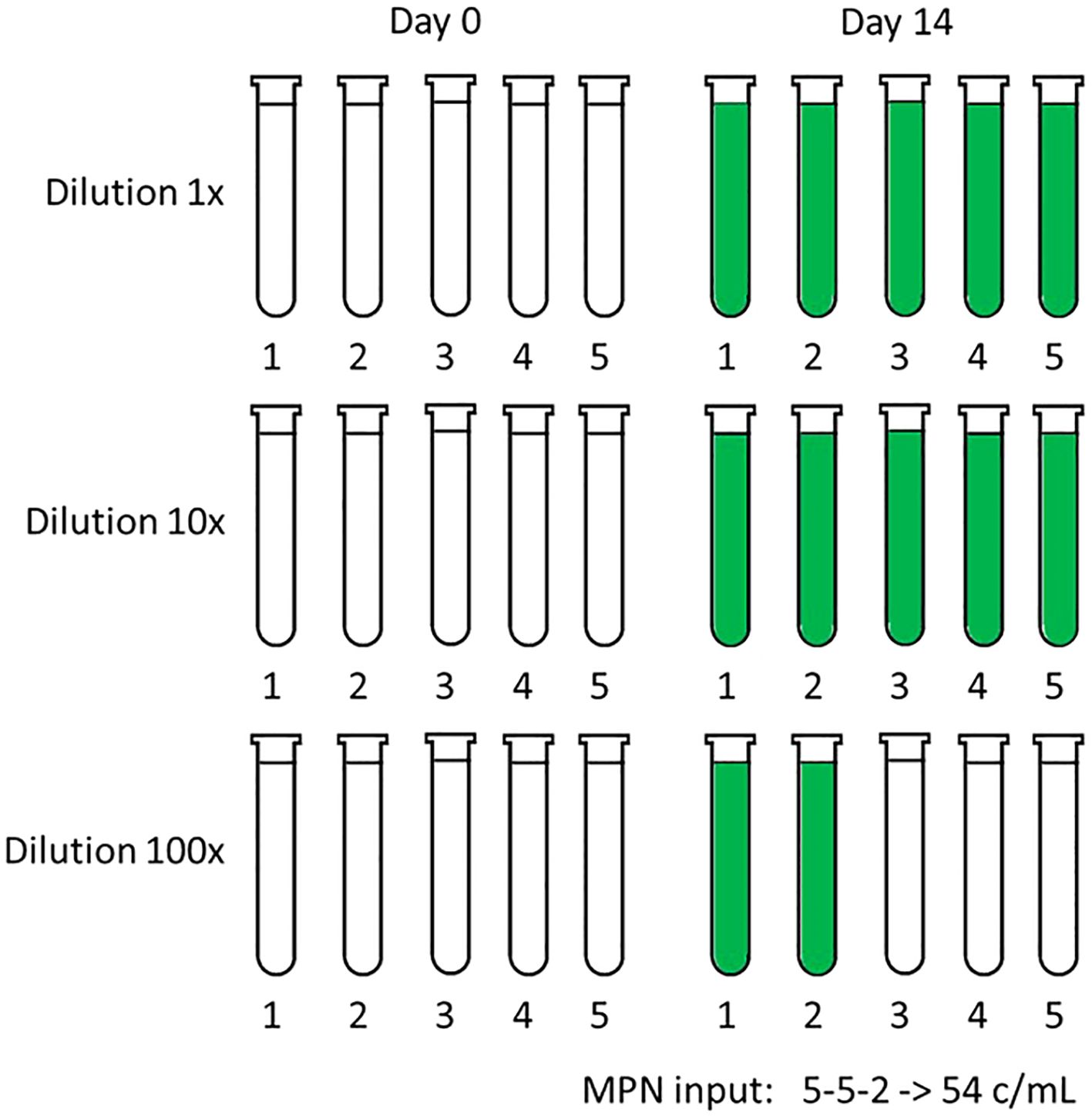
Figure 1. Example of the standard MPN matrix with three dilution factors: 1x (undiluted sample), 10x and 100x diluted sample. Each dilution is dispensed in five tubes with culture medium. The dilutions are made on day 0 when the incubation in the light is started. Tubes are scored positive if the measured chlorophyl-fluorescence on day 14 is greater than four times the standard deviation of the five initial fluorescence measurements of that dilution. In this example, two out of five tubes at 100x dilution are positive. Calculation of the total cell concentration is performed by inputting 5-5-2 in a MPN calculator or table, see Supplementary Table 1, which results in 54 c/mL with a lower-higher 95% confidence interval of 16-190 c/mL.
An approved, fast, and simple method to monitor growth after experimental manipulation is by measuring chlorophyll-fluorescence in multiple fluorimeter tubes (Brand et al., 1981; Peeters and Peperzak, 1990). MPN employs this method and the number of growth-positive tubes in the highest dilution is used to calculate the original sample cell concentration according to a table (Anonymous, 1939; Andersen and Throndsen, 2003), or an Excel™ calculator (https://standards.iso.org/iso/7218/) (Jarvis et al., 2010). Several old and new MPN tables and calculators are listed and compared in Supplementary Table 1. A detailed description of the MPN method is given by Andersen and Throndsen (2003). The MPN outcomes are stepwise concentrations, depending on the dilution factor and the number of tubes per dilution (Figure 2). This is in contrast to microscopic counting that has increments of 1 c/mL.
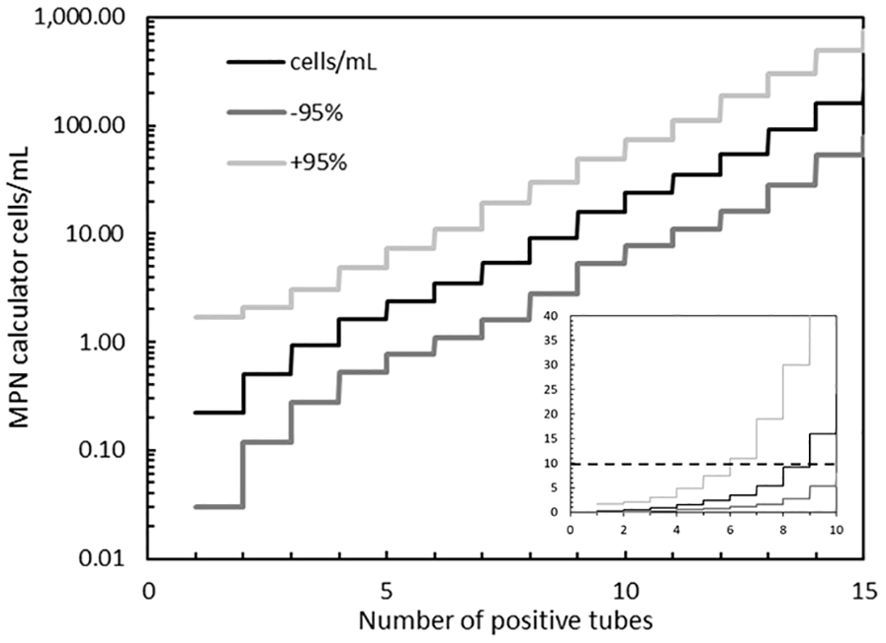
Figure 2. Cell concentrations and 95% confidence intervals (± 95% c.i.) at increasing number of positive tubes, calculated for a tenfold diluted sample that is dispensed in 5 tubes. The example in Figure 1 has 12 positive tubes (5 + 5 + 2). The horizontal dashed line in the inset shows the ballast water non-compliant concentration of 10 c/mL, lying between tubes 8 (9 c/mL, +95% c.i. = 30 c/mL) and tube 9 (16 c/mL, +95% c.i. = 49 c/mL). Note the log scale in the main figure and the linear scale in the inset. Also note that the Jarvis et al. (2010) ISO7218 calculator, see Supplementary Table 1, needs a blank row at a higher dilution to calculate the concentration (denoted as >) and +95% c.i. when all five tubes in the highest dilution tested are positive (e.g. input = 5 + 5 + 5+0, instead of 5 + 5 + 5), otherwise the result (at 5 + 5 + 5) will be twice ∞.
In ballast water testing, a MPN assay is not only used for the 10-50 µm sized phytoplankton eukaryotes, but also for the enumeration of two IMO-regulated indicator microbes Escherichia coli and the enterococci. An important difference in the methods between the eukaryote and the bacteria MPNs is that the latter are incubated at specific temperatures and in growth-specific culture media that inhibit non-target bacteria. In other words, the bacteria MPN assays are specifically designed for the growth of one species (E. coli) or one species-group (enterococci). In contrast, the phytoplankton eukaryote 10-50 µm MPN assay does not discriminate on the basis of size, which leads to the potential of false-positive results: <10 µm or >50 µm sized phytoplankton that also produce chlorophyll-fluorescence. Comparably, false-positives are also possible in the bacteria MPN assays if a non-target species is not inhibited by the incubation specifics for the target organism (Peperzak and van Bleijswijk, 2021).
Cullen and MacIntyre (2016) studied a number of assumptions underlying the MPN method in the enumeration of viable 10-50 µm autotrophs in natural communities and listed six potential problems: 1) failure of viable cells to grow, 2) failure to detect growth, 3) competition, 4) grazing, 5) inclusion of nonregulated organisms (false-positives) and 6) aggregation of phytoplankton cells. In addition, the authors declared that the mathematics of the method were not controversial. For items 2, 3, 4 and 6 Cullen and MacIntyre (2016) convincingly argued these are probably of minor importance. However, their arguments for (1) failure of growth and (5) inclusion of nonregulated organisms or false-positives, appeared less convincing. In addition, false-positives create a problem with the mathematics of the MPN method that have hitherto went unnoticed and that will be addressed later on in this communication.
Based on UVC tests with laboratory cultures MacIntyre et al. (2018) had concluded that MPN is robust and adaptable for use on natural phytoplankton. However, recently Romero-Martinez et al. (2024) found false positive outcomes due to the growth of <10 µm autotrophs, the non-regulated size-class. Of the 80 MPN tubes with UV-treated sample that were examined by microscope, 21% contained <10 µm species (Romero-Martinez et al., 2024). False positive <10 µm organisms may not only lead to non-compliance of treated ballast water. IMO type approval test water for ballast water treatment systems (BWTSs) must have at least of 1,000 c/mL of 10-50 µm organisms. If MPN is used to verify this minimum, false positives will overestimate the true concentration and the system may be tested inappropriately. In addition, IMO allows MPN in the verification of ballast water Compliance Monitoring Devices (CMDs) as a reference method (IMO, 2022, 2023). When such tests employ ambient (multi-species) water, there is a risk of including <10 µm and >50 µm autotrophs which may lead to an aberrant validation.
This communication will focus on failure of species’ growth in the 10-50 µm size fraction and on <10 µm false-positives, potentially leading to respectively under- or overestimation of cell concentrations. In addition, a solution for the MPN calculation of the concentration of specific species or size-constrained species groups in mixed phytoplankton assemblages will be presented.
2 Failure of growth
It is improbable that all phytoplankton species can be cultured and if they will all grow in the standard MPN assay, a fact already recognized by Allen (1919), Knight-Jones (1951) and emphasized by Andersen and Throndsen (2003). This is because a species might be inherently unculturable in vitro or that the laboratory conditions are too different from nature. MPN uses a general culture medium and closed small tubes which probably is not conducive for all species. A positive (Allee) growth effect occurs when multiple cells are placed in a fresh suboptimal culture medium instead of a single one (Courchamp et al., 2008). At high MPN dilutions in fresh culture medium and thus very low cell concentrations the opposite may occur, explaining why single phytoplankton cell isolates incubated in fresh culture medium are not 100% successful (Peperzak, unpublished).
Failure of growth leading to an MPN underestimation of the actual phytoplankton concentration was reported previously (Andersen and Throndsen, 2003; First and Drake, 2014). A counter argument used by Cullen and MacIntyre (2016) is that evidence to support the belief that a majority of phytoplankton in that size class [10-50 µm] cannot be cultured is lacking. However, apart from the inexplicit term “majority”, in standard hypothesis testing the null hypothesis would be: the majority of phytoplankton cannot be cultured. If that hypothesis would be rejected, the opposite is true.
It is unknown exactly how many phytoplankton species exist, and how many have been (non)-successfully cultured. Sournia et al. (1991) estimated there were 5,000 marine phytoplankton species (Sournia et al., 1991), the most widely studied group in the ballast water context. A recent and much higher estimate for extant marine, freshwater and terrestrial algae is 50,000 (Guiry, 2024). Of these, ca. 40% or ca. 20,000 are marine species. The question is how many marine species have been identified in MPN studies?
Unfortunately, many ballast water related MPN validation studies have used only single species from laboratory cultures such as Tetraselmis (Sun and Blatchley, 2017). MacIntyre et al. (2018) used 13 laboratory cultures from 7 phyla. Stephanie Delacroix and August Tobiesen of NIVA identified 46 species in BWTS test water in the 10-50 µm size range growing in MPN tubes. As a pioneer in MPN, Knight-Jones (1951) reported 26 growing species, of which 81% were smaller than 10 µm. Even if 200 species would have been observed growing in MPN tubes, that number would still be 1% of the 20,000 extant species. A comparably low percentage of culturable species was found in bacteria (Kaeberlein et al., 2002). It follows that there is not enough data to reject the null hypothesis that the majority of phytoplankton cannot be cultured. There is a genuine risk that the MPN technique underestimates the true 10-50 µm concentration.
3 False-positives
3.1 Introduction
The goal of the ballast water MPN is to measure the abundance of phytoplankton restricted in and varying by size (10-50 µm). An ecological rule states that small aquatic organisms are more abundant than larger ones (Agustí et al., 1987; Irwin et al., 2006). Autotrophic species ≥ 50 µm, present in a few phyla such as diatoms and dinoflagellates, will thus have a relatively low abundance. On the other hand, the more diverse <10 µm species will be present in relatively higher abundances. The majority of cells growing in one of the first MPN’s performed was <10 µm (Knight-Jones, 1951). In their review of MPN assumptions, Cullen and MacIntyre (2016) recognized the possibility that <10 µm species can lead to an overestimation of the 10-50 µm fraction and suggested that gentle concentration on a 10 µm mesh would largely reduce the problem of these false-positives. That separation of the <10 µm species in MPN is needed was shown by Romero-Martinez et al. (2024) who found both <10 µm and 10-50 µm organisms, and by Casas-Monroy et al. (2016) who found only <10 µm cells, after microscopic examination of the chlorophyl-positive MPN tubes.
A universal method for the separation of plankton constituents and subsequent analysis or cultivation is hard to find (Lewin, 1959; van Ierland and Peperzak, 1984; Waterbury and Willey, 1988; Aguilera et al., 1996; Peperzak and Dyhrman, 2006). When species’ identity or size is required, a per cell analysis using optical methods is still required (Anderson and Cheng, 1988; Yaginuma et al., 2014). Using mesh filters to divide plankton communities into size-fractions appears a simple and straightforward approach to remove too small, or too large, false-positives in MPN assays. Cangelosi et al. (2007) found that plankton removal efficiency by filters was influenced by biotic factors such as organism morphology and structure and that particle size itself was not determinative in phytoplankton removal. This suggests that size-separation by filtration is not that straightforward (Fogg, 1986) although several attempts have been made in ballast water testing.
First and Drake (First and Drake, 2014) used filtration to enhance the concentration of 10-50 µm species for experimental purposes but did not measure the effect of filtration on the removal of <10 µm species. Trindade de Castro and Veldhuis (2019) used a 10 µm mesh sieve for marine samples and measured that 22% of the <10 µm cells were retained on the mesh while 23% of the 10-50 µm cells were in the filtrate. An even larger loss of 10-50 µm freshwater cells due to 10 µm mesh filtration, depending on organism abundance, of 31% to 55% was found by Yardley et al. (2024). A circa 50% loss was also measured in 10-50 µm marine phytoplankton, 24 hours after it had been concentrated with a 10 µm mesh sieve by First and Drake, (2014). Clearly, filtration is less than 100% effective in the removal of <10 µm organisms, and it also substantially reduces the abundance and survival of the 10-50 µm cells. Such a loss of 10-50 µm cells (20 to 50%) is unacceptable in quantitative studies and probably therefore, only one ballast water test facility employed filtration in preparation of the MPN assay. Two other test facilities that used MPN did not filter samples.
In conclusion, filtration or any other method for separating 10-50 µm species from co-occurring plankton is not straightforward and is deemed to introduce a significant bias in the actual concentration of the target organisms. Therefore, the potential impact of specifically the <10 µm autotrophs on the MPN assay results remains and this impact will be examined in the next sections.
3.2 Specific species or size-constrained species groups in mixed phytoplankton assemblages in the MPN assay
In order to examine and possibly quantify the risk and effect of <10 µm autotrophs in the MPN assay for enumerating 10-50 µm organisms in mixed autotroph assemblages, a model was constructed on the basis of well-established ecological relationships or allometric equations: between abundance and size, growth rate and size, size and carbon content and the carbon: chlorophyll ratio (Supplementary Table 2). Plankton-Abundance-Size or PAS Model). The PAS model is used to discuss a number of relevant questions: how abundant are <10 µm autotrophs, can <10 µm autotrophs with a small chlorophyll-fluorescence signal be detected by a standard fluorimeter after incubation, and what is the risk of overestimating the 10-50 µm cell concentration by <10 µm autotrophs?
Three major assumptions of the MPN ballast water assay are that 1) Viable autotrophs are selected randomly, and all will grow during the MPN incubation, regardless of taxonomy and incubation circumstances, 2) Viable autotrophs are not prone to predation that will reduce their abundance and ultimately the final fluorescence and 3) the MPN assay detects autotrophic organisms in the 10-50 µm size range, as required by IMO.
Assumptions 1 and 2 are reviewed extensively elsewhere (Cullen and MacIntyre, 2016; Blatchley III et al., 2018) and are considered as correct. Assumption 3 is the one that will be tested. The null hypothesis is therefore that this assumption is not true. Rejection of this hypothesis means that the MPN method correctly detects, and enumerates, cells in the 10-50 µm size range.
3.2.1 PAS model
The PAS model shows that the cell concentration exponentially increases from 1 c/mL at the maximum size of 50 µm towards 400 c/mL at 10 µm and 106 c/mL at 1 µm (Figure 3). Practical experience in ballast water testing and analysis (Lundgreen et al., 2019) (Peperzak, unpublished) agrees that the number of small (near and below 10 µm) organisms is indeed much higher than larger ones (up to 50 µm). In fact, the origin of the MPN assay is to isolate and cultivate small phytoplankton (Knight-Jones, 1951; Throndsen, 1978). Using MPN, Knight-Jones (1951) measured concentrations in the 2 to 10 µm range of 1,000 up to 10,000 c/mL, which for his smallest cells (2 µm) is a factor of 10 lower than the PAS model. More recent data obtained by microscopy suggest that picoplankton (0.2 – 2 µm) concentrations are in the order of 105 to 107 c/mL (Fogg, 1986), which is in agreement with Figure 3. Although a natural water sample will not contain one species in every 1 µm model-increment, the data from Figure 3 will be used as a theoretical example.
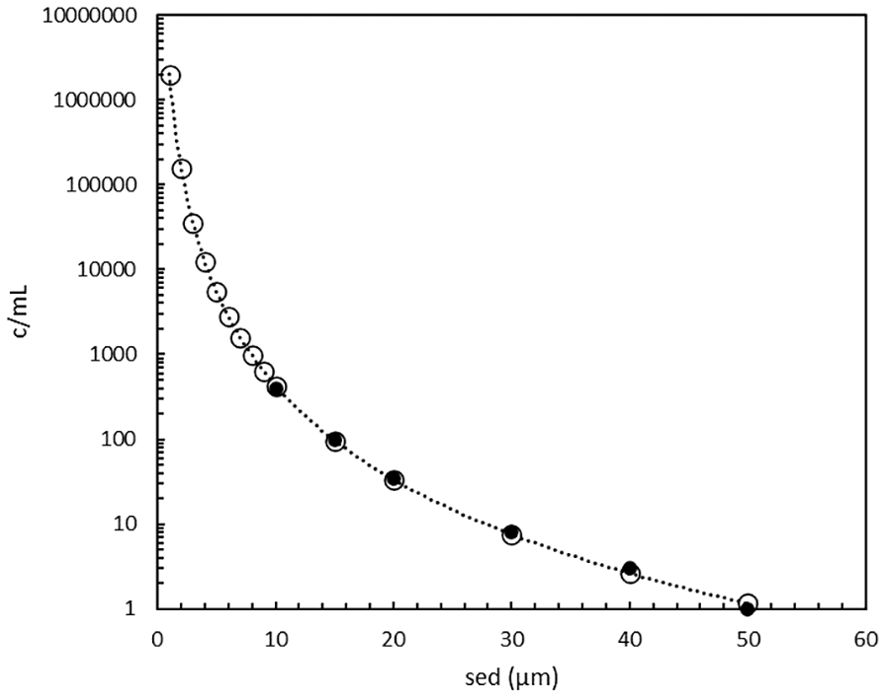
Figure 3. Phytoplankton cell concentrations as function of their size as spherical equivalent diameter (1 to 50 µm) according to the PAS model (o), see Supplementary Table 2, an extension of the original data (●) of Welschmeyer and Kuo (2016).
3.2.2 Cell abundance in <10-50 um range
The theoretical cell abundance in the prescribed ballast water size range (10-50 µm) is 1,800 c/mL (Figure 3, eq.2 in Supplementary Table 2), which is relatively close to the minimum of 1,000 c/mL requirement for BWTS type approval testing. In other words, the abundance calculated with the PAS model pertains to real untreated ballast water values.
In extending the cell abundance below 10 µm, a minimum value of 4 µm was chosen. If the nanoplankton (0.2 to 2 µm) and all the picoplankton (2 to 20 µm) would be included, the abundance of the 1-9 µm organisms would lead to a large relative underestimation of the 10-50 µm cell abundance. Second, ballast water uptake in harbors is often in nutrient-rich coastal waters that may have relatively higher abundances of larger plankton then in off-shore regions. Third, 4 µm is the minimum dimension (width) of the cyanobacteria Phormidium sp., found in MPN tubes containing natural Wadden Sea UV-treated samples (Romero-Martinez et al., 2024). In a freshwater plankton filtration study Cangelosi et al. (2007) found that the minimum dimension of the filamentous cyanobacteria was uniform at 5 µm, close to the width of the marine Phormidium (Cangelosi et al., 2007). Using 4 µm instead of the PAS minimum of 1 µm (Figure 3) means that the potential false-positive effect of the small (<10 µm) species in the MPN assay will not be overestimated.
The result of applying a = 4 µm and b = 50 µm (eq.2 in Supplementary Table 2) resulted in a sum of 25,500 c/mL (including 1,800 c/mL in the 10-50 µm range). This means that of the total (4-50 µm) abundance, 93% has a size below 10 µm. This high percentage emphasizes the potential impact of <10 µm species on MPN analyses. Even if a separation technique could remove the <10 µm cells with an efficiency of 95%, there concentration would still be ca. 1,200 c/mL, in the same order as the 10-50 µm cells. Unfortunately, the <10 µm filtration efficiency as measured by Trindade de Castro and Veldhuis (2019) was less than 80%. Also considering the measured 20-50% loss of 10-50 µm cells by filtration, this technique does not seem suitable for quantitatively separating organisms.
3.2.3 Chance of finding a ≥10 µm cell as determinant of the MPN concentration
The chance of finding a cell of the required ballast water size range (10-50 µm) in the highest MPN dilution where growth will occur is dependent on the MPN matrix, the 4-50 µm cell concentration of 25,500 c/mL and their size distribution (Figure 3). Because the concentration of cells decreases with increasing size, the chance of finding one cell with a specific size ≥10 um is only P ≤ 0.02 (Figure 4). The cumulative chance of finding one cell in the 10 to 50 um size range is also quite small at P = 0.07 (Figure 4). In other words, there is a very high theoretical probability that a cell with size < 10 um is influential in determining the MPN outcome as total c/mL.
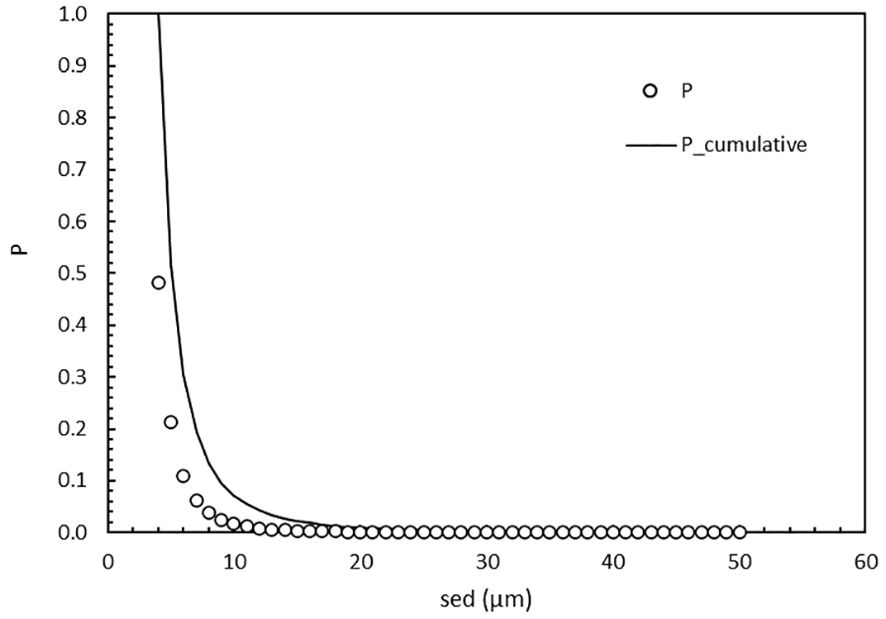
Figure 4. The chance P (o) of finding a cell of a specific size (4 to 50 µm sed) as well as the cumulative chance (line) in the highest MPN dilution. The cumulative chance is the addition of chances of finding a cell at 50 µm and downwards. At sed = 4 µm, P_cumulative = 1.
3.2.4 Can small cells reach sufficient biomass to reach the fluorometer detection limit?
The relation between growth rate and cell size (Supplementary Table 2, eq.3) yields growth rates in the 4 to 50 µm range of 1.89 (4 µm) to 0.26 d-1 (50 µm)(Figure 5). This range is acceptable. For example: the calculated growth rate of 8 µm cells of 1.1 d-1 (eq. 3) is intermediate to values reported of the 8 µm-sized (Peperzak et al., 2000a) prymnesiophyte Phaeocystis globosa: 0.7 d-1 (Peperzak et al., 2000b) to 1.4 d-1 (Peperzak, 2002).
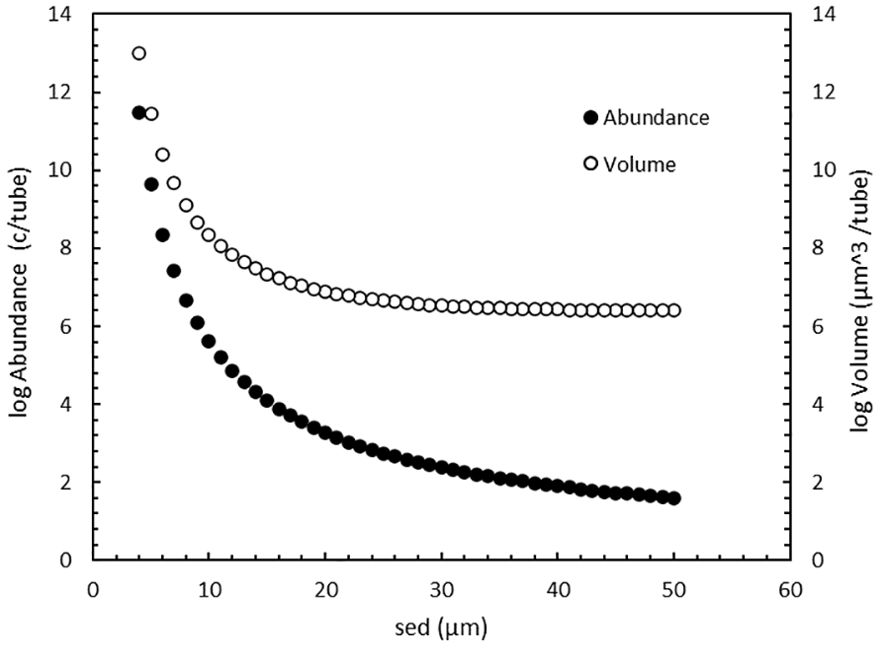
Figure 5. Cell abundance (●) and total cell volume (o) in an MPN tube, starting with 1 cell, after 14 days incubation growing at maximum growth rate as function of cell size.
Using the standard incubation time for MPN assays (14 days) and an optimal incubation scenario with no lag-phase and a constant maximum growth rate, the number of cells (Supplementary Table 2, eq.4) in an MPN tube increases with decreasing cell size (Figure 5). There is a steep increase in both concentration and biovolume (calculated from concentration and the sed) below 20 µm. A check on the plausibility of such high abundances can again be made with the 8 µm sed P. globosa that has a calculated µmax = 1.1 day-1 (Supplementary Table 4, eq. 4). After 14 days of incubation the calculated abundance is 5 x 106 cells (Figure 5) per tube (6 mL medium), i.e. ≈106 per mL, which is the maximum P. globosa concentration found in nature (Cadée and Hegeman, 1986). The increase in biovolume is noteworthy because it determines the chlorophyll content, and the intensity of the chlorophyll-fluorescence will determine if a tube scores negative or positive for growth.
In the next and final step, the plausibility is calculated that cells, especially those <10 µm, can surpass a fluorometer detection limit after 14 days incubation and score a positive tube. To calculate carbon from volume two equations are used, one for protists in general and one specific for diatoms that have relatively low carbon contents (Supplementary Table 2, eq.5a+b). The results for each of the two species groups was then converted to chlorophyll (Supplementary Table 2, eq. 6) using a ratio of 33 for both (Riemann et al., 1989), and the chlorophyll concentration in the MPN tube was calculated. As expected, both the protists and the diatom chlorophyll concentrations increase exponentially with decreasing size (Figure 6).
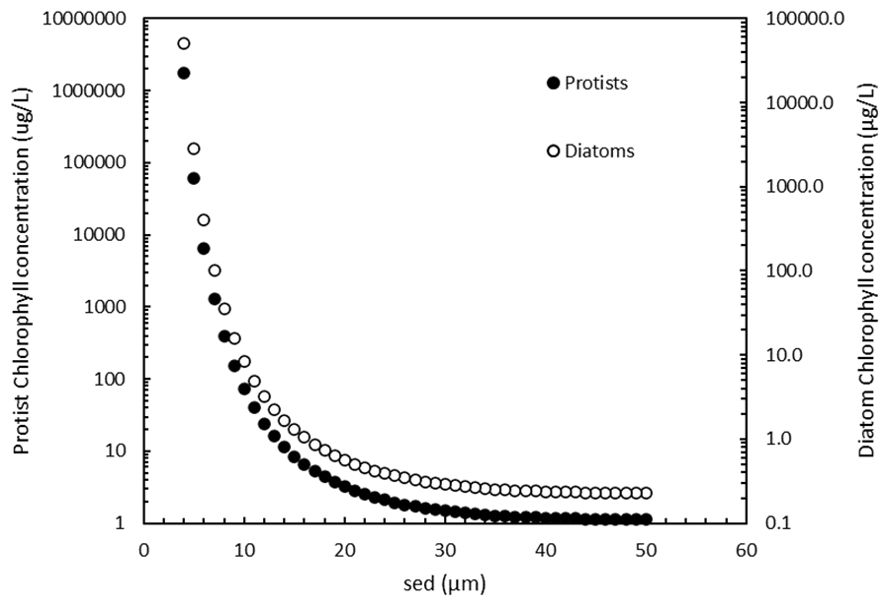
Figure 6. Protist (●) and diatom (o) chlorophyll concentrations in MPN tubes after 14 days incubation growing at maximum growth rate.
The frequently-used Turner 10-AU fluorometer in MPN assays has a detection limit of 0.025 µg/L in the lowest calibration range for extracted chlorophyll a using EPA Method 445.0. (https://www.turnerdesigns.com/10au-field-laboratory-fluorometer, assessed 1-11-2023). Figure 6 shows that the minimum chlorophyll concentrations are 1 µg/L and 0.2 µg/L for the largest protists and diatoms respectively, both above the detection limit. To compare the values in Figure 6 with real world data: in eutrophic coastal Dutch waters the maximum chlorophyll concentrations in diatom-Phaeocystis blooms were between 100 and 200 µg/L (L. Peperzak, unpublished). In Figure 6, values above 200 µg/L are reached by small (<10 µm) cells. Although such high chlorophyll concentrations (>1,000 µg/L, Figure 6) in an MPN tube are unrealistically high it is clear that, according to the model, cells of all sizes can be detected in the standard MPN by a fluorometer.
Figure 6 also makes clear that the smaller cells contribute severely to the chlorophyl concentration compared to the larger organisms. The consequence is that in a mixture of species, the more abundant smaller ones not only have a higher chance of ending up in a tube at higher dilutions, but they are also very likely to be detected. This means that the chance to use false positive (<10 µm) tubes in the MPN calculator or table is not reduced by the cell’s low individual chlorophyll content.
3.3 Calculation of species and groups in mixed assemblages
If the purpose of an MPN assay is to measure the concentration of a specific size group, such as the 10-50 µm ballast water autotrophs, there is a considerable chance that <10 µm species are included in the assay. As discussed, there are no 100% effective methods to isolate species or species groups from such assemblages. Even simple mesh filtration reduces the abundance of 10-50 µm species and does not effectively remove the <10 µm ones. The presence of <10 µm phytoplankton adds to the total concentration, of all species, measured in the MPN assay and because the smallest ones are the most abundant, it is likely that they are present in the highest dilution and end up in one or more of the five tubes inoculated from that dilution. This means that by applying the standard IMO protocol (IMO, 2019), with chlorophyll-fluorescence growth detection only, the concentration of the 10-50 µm autotrophic organisms is overestimated if there is no correction for the <10 µm viable phytoplankton.
A simple solution for overestimation seems to check the tubes from the highest dilution with a microscope, and to simply exclude the false-positive tubes with <10 µm species from the MPN calculation. Suppose that in Figure 1, one of the two positive tubes in the 100x dilution (scenario 5-5-2) has only a <10 µm species, and the second tube only a species from the 10-50 µm group. The obvious way to go forward is, for each species, to exclude one tube from the scenario so that it becomes a 5-5-1 calculation for both, not 5-5-1 for one and 5-5-2 for the other because the order is random. However, the 5-5-1 scenario for both is also incorrect because the abundances of all species, in all dilutions and in all MPN tubes, constitute the calculated total phytoplankton concentration. Therefore, it is also incorrect to directly look-up or calculate the concentration of a specific microscopically identified species at any dilution, from the number of species-positive tubes at that dilution. It appears that already Knight-Jones (1951) employed this intuitive but erroneous method because most of his reported concentrations can be found in the MPN tables he used (Anonymous, 1939). Although the most abundant species has the highest concentration, this is not the value from the MPN calculator or MPN table. Some examples will clarify this.
Imagine first that in the MPN assay only chlorophyll-fluorescence is measured (Figure 1). The only information available is the number of positive tubes, five in dilutions 1x and 10x and two in the 100x dilution (scenario 5-5-2), which yields 54 c/mL. Second, suppose that the sample contains only one species, and the scenario remains 5-5-2. It is as if “chlorophyll” is replaced by “one species” and the outcome remains identical: the one-species concentration is also 54 c/mL. Third, as a thought experiment, suppose now that there is not one but that there are 11 species, each at 5 c/mL. According to the MPN table, a single species of 5 c/mL would end up in two tubes in the 10x dilution (scenario 5-2-0). But not all 11 species can end up in two tubes in the 10x dilution and yield 5 c/mL. The species are not individually diluted and pipetted into MPN tubes. The assemblage is diluted and pipetted and its total concentration is still 11 x 5 = 55 c/mL. The MPN dilution, pipetting and tube inoculation method is ‘blind’ for which species are present.
Another way to look at the working of the MPN is to calculate the cell concentrations in the dilutions. If the original sample (1x dilution) has 54 c/mL (27 A/mL and 27 B/mL), the 10x and 100x dilution of that sample have 5.4 c/mL and 0.54 c/mL. If that last dilution has a volume of 10 mL, the number of cells in that volume is rounded to 5. In the standard MPN, five draws of 1 mL give a considerable chance (P = 0.32, Table 1, Supplementary Table 3) that two of the draws contain a cell that will end in the two tubes derived from this 100x dilution, hence scenario 5-5-2. Because the diluting and pipetting work randomly, it does not matter if the cells belong to one, two or more species: the result (5-5-2) will be equal. It is clear that the outcome of scenario 5-5-2 in the MPN calculation or table is the total sample concentration of all growing species dispersed in all the positive tubes.
In the 11 species at 5 c/mL thought experiment above, the consequence of randomness is that there is a considerable chance (P = 0.28, Table 2, Supplementary Table 3) that two species are present in the two tubes at the 100x dilution of the 5-5-2 scenario. Nevertheless, all 11 are still present in the MPN tubes from the 1x and 10x dilution. If the identity of the two species in the two tubes at 100x dilution is known, the concentration of each species would each be calculated from the 5-5-1 scenario (1 positive tube in the 100x dilution = 35 c/mL). Both concentrations added would be 70 c/mL which is already more than the sum (55 c/mL) of all 11 species which cannot be correct. In addition, we know in this thought experiment there are also nine other species at 5 c/mL each, or 45 c/mL summed. However, the grand total of the two and nine species respectively is not 70 + 45 = 115 c/mL, it should be 55 c/mL. Consequently, if the concentration of two species in a mixture cannot be estimated correctly, this must apply to all species in that mixture. There is no recognition in the literature of this complication in calculating specific species or species-groups in mixed assemblages. The question is if it is possible to correct the MPN estimates in mixed assemblages.
The correction of the MPN estimate in mixed assemblages will be examined in a simple example. Suppose that two viable species are present in a test sample in equal concentrations, which means they have the same chance of ending-up in the final tube(s) of the highest dilution showing growth. The chlorophyll-fluorescence MPN example in Figure 1 is used in Figure 7 that now presents the two species scenario. Species A and B, both at 27 c/mL, have a total concentration of 54 c/mL. According to the MPN table there are two tubes at 100x dilution that will be positive after incubation. If the MPN assay is made with the purpose of estimating the concentration of both species, a microscopic examination of all tubes must be made. Suppose that at 100x dilution each species is found in one tube and three tubes are negative (scenario 5-5-2, Figure 7). Note that other combinations A, B and “no cell” are also possible and that in this example the order of A, B and negative tubes in the highest dilution is random: any combination (n = 20) of A, B and the three negative tubes is possible (Supplementary Table 3).
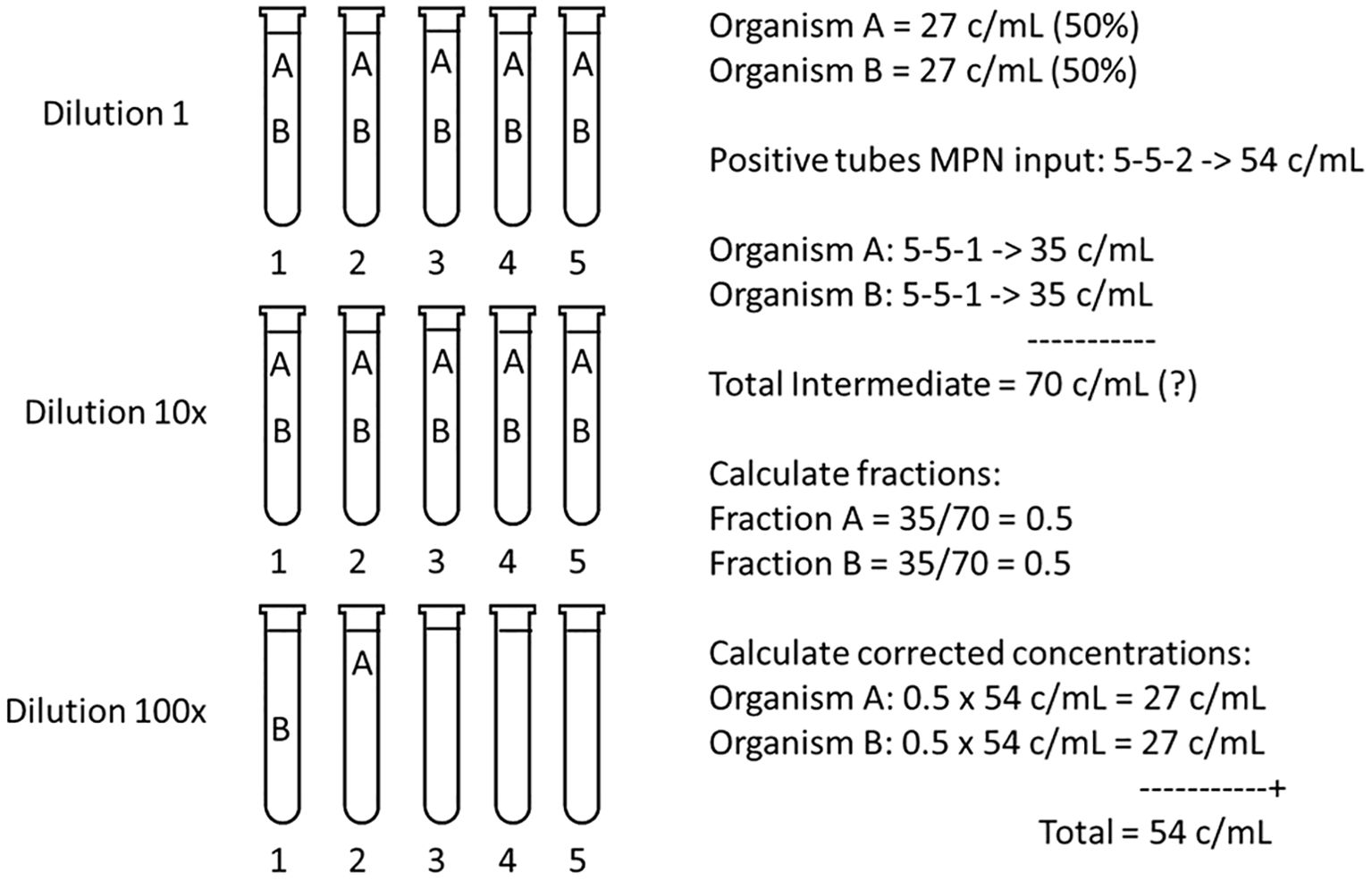
Figure 7. Calculation of the corrected concentrations of species A and B, each at an equal sample concentration of 27 c/mL, hence a Total MPN of 54 c/mL. If only positive growth is monitored, such as chlorophyll fluorescence (Figure 1), the MPN calculator or table input is 5-5-2. Because the species have an equal concentration the odds are that one cell of A and one cell of B ended in two of the five tubes in the 100x dilution. If after incubation microscopic observation reveals one tube with A and one with B, and the MPN calculation is repeated for both A and B with 5-5-1, then the Total Intermediate (A+B) concentration = 2 x 35 = 70 c/mL, which contradicts 54 c/mL. By calculating the fractions of species present from the Total Intermediate, and by multiplying with the Total MPN concentration, corrected concentrations for both A and B are found.
How to calculate concentration of B if this is the species of interest? What lies in reason, and has been common practice, is to discard one tube (here with species A) and to perform a new MPN calculation for species B using scenario 5-5-1, which yields 35 c/mL. Naturally, if the calculation is repeated for A (also with 5-5-1) this also yields 35 c/mL. This seems correct because both species were present in equal amounts (each one tube in the final dilution). However, the total of A and B then would be 70 c/mL, which cannot be true because the total MPN is 54 c/mL.
The solution to arrive at the corrected concentrations of A and B is to calculate the fractions of the species present in the Total Intermediate (adding 2x scenario 5-5-1), and to multiply these fractions with the first, Total MPN concentration of 54 c/mL (Figure 7). This will result in the correct 27 c/mL for both A and B. In this example the calculations seem trivial because it is clear that A and B, each in one tube, should make up 50% of the MPN calculated concentration. However, there are more common and variable combinations of species possible where a more elaborate calculation, based on the Intermediate Total, is needed.
Because uncorrected MPN outcomes cannot be added (Intermediate A c/mL + Intermediate B c/mL ≠ Total MPN c/mL, Figure 7), A or B can also not be subtracted from the total. This means that leaving out unwanted (non-target) positive tubes from the MPN scenario and to do the calculation with only the wanted (target) positive tubes, can lead to serious aberrations. The subtraction must take place after the correction.
Subtraction is important in the case of a three species mixture, when the concentration of a specific species (say C) needs to be estimated. All positive MPN tubes should then be examined for species composition and the straightforward approach and common practice would be to input all positive tubes with species C in the MPN calculator or table (Knight-Jones, 1951). For instance, if in the mix with species A and B (scenario 5-5-2, 54 c/mL, Figure 7), species C would be present in three tubes of dilution 10x (input 5-3-0), the MPN calculator yields C = 9 c/mL. The Intermediate Total is A+B+C = 35 + 35 + 9 = 79 c/mL. The correction would be that C is present as 9/79 of the Total MPN concentration (54 c/mL) = 6 c/mL instead of 9 c/mL. Note that A and B now also need to be corrected to 35/79 x 54 c/mL = 24 c/mL each. Although the correction for C is -33%, it seems trivial considering it is only -3 c/mL and given that the MPN confidence intervals are large (Figure 2). However, if increasing numbers of species are present, the fractions of the total concentration they occupy makes the downward correction more relevant. In ballast water testing a correction of 3 c/mL can make the difference between compliance (<10 c/mL) and non-compliance (≥ 10 c/mL).
The calculation of separate species concentrations with the fractions of the Intermediate Total does not always give exact outcomes in all mixtures. In simulations with uneven and different fractions of two species (A and B), the Total MPN outcome results in slight under- and overestimations of A and B respectively (Supplementary Table 4). This effect is inherent to the stepwise and non-linear increase in the MPN-calculator concentrations.
In the case of more than three species, such as the 11 species at 5 c/mL example, it is improbable that all 11 are present in the final two tubes of the 100x dilution. In fact, it is probable that only two species are present in the 100x dilution and all of them in the 10x and 1x dilutions. The two random species in the 100x dilution will yield the highest concentrations, while the other nine will yield 10x lower estimate, which would be incorrect. If all 11 species are present in all five tubes in the penultimate dilution, they cannot be treated as the maximum of the 5-5-0 scenario, which is 24 c/mL, because that would lead to 24/11 = 2 c/mL per species. The consequence seems to be that the suggested correction, using the fractions of species abundances (Figure 7) is possible in simple mixtures such as two or three species, but that it becomes increasingly difficult or even impossible in more complex mixtures. If an attempt is going to be made to correct the concentrations of species or species-groups in mixtures, at least information on the relative abundance of all species in the tubes of at least the (pen)-ultimate dilutions (10x, 100x) should be obtained. The contribution of species present only in tubes with less diluted sample (1x) to the total concentration would be negligible.
Another method to achieve the correct species concentrations from the Total MPN outcome is to measure the relative abundances or fractions of all species on day 0 of the MPN assay. This means detailed microscopic counting of all viable species. This information cannot be obtained on day 14 from the 1x dilution of the MPN assay because the organisms present at the start of the incubation, with their various growth rates, will have changed the original relative species composition. To establish the relative abundances of all species beforehand seems a formidable task. It would involve detailed microscopic counting of all presumed viable species which would be time-consuming, prone to errors, and is counter to the essence of the MPN assay itself. In practice, absolute or relative species abundances have never been measured beforehand which means that published species-specific MPN concentrations cannot be corrected. The semi-quantitative approach suggested by Throndsen (Throndsen, 1982), to report MPN-derived cell concentrations in factor of 10 steps, appears to be the highest accuracy feasible.
4 MPN and microscopy
The difference between significantly compliant or non-compliant ballast water seems trivial, but it is not: is the a priori presumption of the analyst that the water is compliant or non-compliant? The null-hypothesis chosen here, laying the burden of proof on the BWTS that is tested (Miller et al., 2011), is that the test water is non-compliant (≥10 c/mL). This is also the approach of the Dutch Port State Control (PSC). When this hypothesis is rejected, the opposite is true, i.e. the test water is compliant (<10 c/mL). In the case of the standard MPN assay, the lowest concentration that is compliant is 9 c/mL (Figure 2), but because the +95% c.i. is 30 c/mL the null-hypothesis cannot be rejected at this concentration. The +95% c.i. should be <10 c/mL, and this is reached in the standard matrix in scenario 5-0, at 2 c/mL (+95% c.i. = 7 c/mL). This appears to make the MPN detection limit for non-compliance much lower than for microscopic counts, that can achieve 9 c/mL.
However, microscopic enumerations also have an error and associated 95% c.i. around the calculated concentration. For a single count yielding n cells, assuming a Poisson distribution and the standard deviation s = √n and the 95% c.i.’s approximated by 2*s, the concentration at which the null-hypothesis can be rejected is 5 c/mL (+95% c.i. = 4 c/mL). For multiple counts this will depend on their number (m), the standard deviation between them (s) and the mean concentration. The 95% c.i. of multiple counts is calculated as: s * t/√ m, where t is the t-value for m-1 counts at α = 0.05. For three counts for instance, the +95% c.i. = s * 4.3/1.7 ≈ 2.5 * s. Because s = 1 if two counts are -1 and +1 c/mL of the middle value, +95% c.i. ≈ 3, which means the concentration at which the null-hypothesis can be rejected is 6 c/mL.
In reality, and when the treatment is not 100% effective, the counts are expected to vary more than ±1 c/mL. An extreme case is when 0, 9 and 18 c/mL yield a mean of 9 c/mL, s = 9 and the +95% c.i. = 22 c/mL. Test facilities are not required to report the calculated confidence limits because neither IMO nor USCG have offered guidance in that respect (Miller et al., 2011). In case of doubt, such as in this extreme example, a test facility will immediately choose to make more microscopic counts in that particular sample to gain more certainty. Therefore, the actual confidence limits of microscopic counts will vary per sample depending on cell abundance, counting variability and the number of counts, and they remain unknown if not reported. Although the MPN assay appears to have an a priori low rejection limit (2 c/mL), in practice the a posteriori microscopic limit (≤6 c/mL) may not fair much better.
5 Discussion and conclusions
The estimation of planktonic cell concentrations has a long history and was approached with many techniques such as microscopy, serial dilution culture, sensing-zone counters, flow cytometry and holography (Knight-Jones, 1951; Utermohl, 1958; Sournia, 1978; Throndsen, 1978; Hofstraat et al., 1990; Nir et al., 1990; Wietzorrek et al., 1994; Veldhuis and Kraay, 2000; Stehouwer et al., 2013; Romero-Martinez et al., 2017; Peperzak et al., 2020). The quest for the correct concentration becomes even more daunting when the enumeration involves a specific size class, such as the IMO 10-50 µm “minimum dimension”, and when the species need to be categorized as live or dead, viable or non-viable. Regardless of the enumeration technique, a statistical evaluation of the final cell concentration is needed to ascertain how reliable the end result is.
In comparison with other techniques, the MPN assay is peculiar because the calculated concentrations and their 95% confidence intervals, the 95% chance that the true sample concentration lies within that interval, go up stepwise. For every MPN matrix and for every concentration estimate, these intervals are known a priori. A disadvantage in microscopy and other counting techniques, is that the confidence intervals are calculated a posteriori. In the case of ballast water testing, there is a time limit of eight hours to microscopically count the live organisms in fresh samples. As in other biological tests, counting cannot be extended for days until a statistically satisfying result is obtained. Here, another advantage of the MPN assay is that, although the end result will take two weeks at minimum, diluting, pipetting and the start of the incubation can be performed relatively easy and fast, even at sea. Regardless of which counting technique is used in ballast water testing, the confidence interval of the calculated mean cell concentration is rarely used to infer if the tested ballast water was significantly compliant or significantly non-compliant.
Viably reproducing planktonic autotrophs lay at the heart of the MPN assay and it has been used to estimate cell concentrations of previously unknown and often small species and to bring them into cultivation (Knight-Jones, 1951; Throndsen, 1978, 1982; Andersen and Throndsen, 2003). However, when the MPN technique is to be applied to estimate the concentration of species, singly or in a specific category as size (10-50 µm), a number of shortcomings emerge.
First, only 1% of the extant phytoplankton species has been shown to grow in MPN assays. No growth can mean a cell is non-viable but also that it is unable to grow under the culture conditions offered (Allen, 1919; Andersen and Throndsen, 2003). Second, the more abundant <10 µm species are included in the total MPN cell count (Casas-Monroy et al., 2018; Romero-Martinez et al., 2024). The standard method to prove growth in the MPN assay is by chlorophyll fluorescence and that signal can emanate from both target and non-target species. Without optical confirmation that only the target species are growing in the positive MPN tubes, the MPN result will only indicate the total concentration of all viable and culturable species in the sample. Third, the MPN results have a large 95% confidence interval leading Throndsen (1982) to interpret the assay as a semiquantitative method with results to be presented as orders of magnitude (100 c/mL, 1,000 c/mL, etc.). Unless the assay is extended enormously with smaller (<10x) dilutions steps and more tubes at each dilution, the MPN assay seems inadequate for IMO regulations that prescribe 1 c/mL accuracy: 9 c/mL is compliant, 10 c/mL is non-compliant (IMO, 2018). Fourth and practical, PSC officers who monitor discharged ballast water for compliance with IMO regulations, need analysis methods that turn out results within hours, not after 14 days. The MPN assay is not practical for routine shipboard ballast water testing.
Several studies have shown that after UV-treatment, the concentration of MPN cell concentrations are <10 c/mL, regardless of size, i.e. numerically compliant with IMO’s requirement (Casas-Monroy et al., 2016; IMO, 2018; Romero-Martinez et al., 2024). Such outcomes have been interpreted as a successful treatment. This might well be the case but what if a large number of species in the sample did not grow because they are unculturable? One can argue that such non-growing species are so sensitive or need such specific growth requirements that, after deballasting, it might be unlikely that they will multiply and pose a threat to their new environment. The problem is that there is no exact answer to these questions. It is improbable that all extant phytoplankton species will be tested to prove they can grow in MPN assays. And it appears impossible to prove that unculturable species cannot be transferred successfully into a new habitat by ballast water discharges. The MPN assay in IMO ballast water testing (IMO, 2019) is a technique that is and probably will remain unvalidated.
One could interpret compliant, <10 c/mL MPN outcomes, as an indication that ballast water treatment has been successful. But authorities in type approval of BWTS or CMDs are not satisfied with an indication. In addition, when the MPN technique has been used to test the minimum 10-50 µm organism concentration in the test water that was used in approving BWTS or CMDs, there is a chance that this water did not meet that requirement. The approval might therefore be based on incorrect data. In this light, the stand of the USCG, that only the microscopic count of vital (FDA/CMFDA) organisms is allowed in ballast water testing, is a safer choice than the IMO MPN viability assay. Even if the FDA/CMFDA vitality method means that higher UV-doses need to be applied at ballast water treatment to kill vital but non-vital cells, the reliability of discharging compliant ballast water is higher.
The co-occurrence of multiple species in MPN sample tubes has been recognized but has not lead to an adaptation of the calculation of the target species only (Throndsen, 1978; Andersen and Throndsen, 2003). Here, the multiple species examples made clear that a correction is necessary to avoid erroneous concentrations of specific species or species-groups. By calculating intermediate concentrations for each species and to use these to obtain the fractions of each species, better estimates of their concentrations can be calculated from the total MPN count. The downside is that more work is involved due to microscopy and calculations, especially when large numbers of tubes are used, or when several species are present. In addition, as the number of species increases, the correction of the concentrations by using their fractions of the total MPN concentration becomes uncertain or even impossible. In cases with multiple autotrophs present in a ballast water sample, and when only chlorophyll fluorescence is measured, the MPN assay is not a detailed quantitative method.
In conclusion, the advantages of the MPN assay are that it is relatively easy and fast to start and that for every concentration estimate the 95% confidence intervals are known a priori. On the other hand, it takes weeks of incubation before an estimate can be made, estimates are non-linear stepwise concentrations, the 95% confidence intervals are large, only 1% of the extant phytoplankton species has been shown to grow in MPN assays and without optical confirmation that only the target species are growing, the MPN result will only indicate the total concentration of the subset of all autotrophs present in the original sample: the viable and culturable species. In addition, the mathematics underlying the MPN method are more intricate than hitherto realized and to avoid calculating erroneous concentrations of specific species in that subset a correction is necessary. However, this correction becomes uncertain or even impossible with increasing numbers of species. The MPN assay should not be used as a quantitative method in ecological studies or in applications such as ballast water testing.
Author contributions
LP: Conceptualization, Formal analysis, Investigation, Methodology, Visualization, Writing – original draft, Writing – review & editing.
Funding
The author(s) declare that no financial support was received for the research, authorship, and/or publication of this article.
Acknowledgments
For discussions and literature on the relation between cell size and abundance I thank dr. N. Welschmeyer (Golden Bear, USA) and dr. D. van Oevelen (NIOZ, The Netherlands). Questions on MPN analyses were kindly answered by drs. O. Casas-Monroy and S. Bailey (Great Lakes Laboratory for Fisheries and Aquatic Sciences, Canada), G.I. Petersen and G. Hansen (DHI, Denmark), N. Welschmeyer (Golden Bear, USA) and L. Romero-Martinez (Spain). Dr. M.D.R. Guiry presented data on algal species abundance. Dr. P. Wilrich (Berlin, Germany) answered questions on the MPN calculation. Dr. J. Throndsen (Norway) helped to clarify aspects of the MPN assay. Dr. A.U.D. Tobiesen and Dr. S. Delacroix (NIVA, Norway) communicated the number of culturable species in their MPN assays. Mr. K. Hak (Port State Control, The Netherlands) was helpful in discussing (non)-compliance in ballast water analysis. Prof. Dr. J. Cullen (em. Dalhousie University) was helpful in discussing several aspects of the MPN assay.
Conflict of interest
The author declares that the research was conducted in the absence of any commercial or financial relationships that could be construed as a potential conflict of interest.
Publisher’s note
All claims expressed in this article are solely those of the authors and do not necessarily represent those of their affiliated organizations, or those of the publisher, the editors and the reviewers. Any product that may be evaluated in this article, or claim that may be made by its manufacturer, is not guaranteed or endorsed by the publisher.
Supplementary material
The Supplementary Material for this article can be found online at: https://www.frontiersin.org/articles/10.3389/fmars.2024.1494598/full#supplementary-material
References
Aguilera A., Gonzales-Gil S., Keafer B. A., Anderson D. M. (1996). Immunomagnetic separation of cells of the toxic dinoflagellate Alexandrium fundyense from natural plankton samples. Mar. Ecol. Prog. Ser. 143, 255–269. doi: 10.3354/meps143255
Agustí S., Duarte C. M., Kalff J. (1987). Algal cell size and the maximum density and biomass of phytoplankton. Limnol. Oceanogr. 32, 983–986. doi: 10.4319/lo.1987.32.4.0983
Allen E. (1919). A contribution to the quantitative study of plankton. J. Mar. Biol. Assoc. UK (New Series) 12, 1–8. doi: 10.1017/S0025315400059889
Andersen P., Throndsen J. (2003). “Estimating cell numbers,” in Manual on harmful marine microalgae, Book 11 (UNESCO, Paris).
Anderson D. M., Cheng T. P. (1988). Intracellular localization of saxitoxins in the dinoflagellate Gonyaulax tamarensis. J. Phycol. 24, 17–22. doi: 10.1111/j.1529-8817.1988.tb04451.x
Anonymous (1939). “The bacteriological examination of water supplies,” in Reports on Public Health and Medical Subjects., Book 71 (revised edition) (Plymouth, UK: Ministery of Health).
Blatchley E. R. III, Cullen J. J., Petri B., Bircher K., Welschmeyer N. (2018). The biological basis for ballast water performance standards:”viable/non-viable” or “live/dead”? Environ. Sci. Technol. 52, 8075–8086.
Brand L. E., Guillard R. L., Murphy L. S. (1981). A method for the rapid and precise determination of acclimated phytoplankton reproduction rates. J. Plankton Res. 3, 193–201. doi: 10.1093/plankt/3.2.193
Cadée G. C., Hegeman J. (1986). Seasonal and annual variation in Phaeocystis pouchetii (Haptophyceae) in the westernmost inlet of the Wadden Sea during the 1973 to 1985 period. Netherlands J. Sea Res. 20, 29–36. doi: 10.1016/0077-7579(86)90058-X
Cangelosi A. A., Mays N. L., Balcer M. D., Reavie E. D., Reid D. M., Sturtevant R., et al. (2007). The response of zooplankton and phytoplankton from the North American Great Lakes to filtration. Harmful Algae 6, 547–566. doi: 10.1016/j.hal.2006.11.005
Casas-Monroy O., Chan P.-S., Linley R. D., Vanden Byllaardt J., Kydd J., Bailey S. A. (2016). Comparison of three techniques to evaluate the number of viable phytoplankton cells in ballast water after ultraviolet irradiation treatment. J. Appl. Phycol. 28, 2821–2830. doi: 10.1007/s10811-016-0798-3
Casas-Monroy O., Linley R. D., Chan P.-S., Kydd J., Byllaardt J. V., Bailey S. (2018). Evaluating efficacy of filtration+ UV-C radiation for ballast water treatment at different temperatures. J. Sea Res. 133, 20–28. doi: 10.1016/j.seares.2017.02.001
Courchamp F., Berec L., Gascoigne J., Berec L. (2008). “Mechanisms for Allee effects,” in Allee Effects in Ecology and Conservation (Oxford, UK: Oxford University Press).
Cullen J. J., MacIntyre H. L. (2016). On the use of the serial dilution culture method to enumerate viable phytoplankton in natural communities of plankton subjected to ballast water treatment. J. Appl. Phycol. 28, 279–298. doi: 10.1007/s10811-015-0601-x
First M. R., Drake L. A. (2014). Life after treatment: detecting living microorganisms following exposure to UV light and chlorine dioxide. J. Appl. Phycol. 26, 227–235. doi: 10.1007/s10811-013-0049-9
Gollasch S., Cangelosi A., Peperzak L. (2012). Testing of ballast water treatment systems performance regarding organisms below 10 micron in minimum dimension. Final report Prepared for Interreg IVB North Sea Ballast Water Opportunity project, Vol. 17. 1–25 (Texel, The Netherlands: Interreg IVB North Sea Ballast Water Opportunity project).
Gollasch S., David M., Voigt M., Dragsund E., Hewitt C., Fukuyo Y. (2007). Critical review of the IMO international convention on the management of ships’ ballast water and sediments. Harmful Algae 6, 585–600. doi: 10.1016/j.hal.2006.12.009
Guiry M. (2024). How many species of algae are there? A reprise. Four kingdoms, 14 phyla, 63 classes and still growing. J. Phycol. 60, 214–228. doi: 10.1111/jpy.13431
Helbling E. W., Buma A. G., de Boer M. K., Villafañe V. E. (2001). In situ impact of solar ultraviolet radiation on photosynthesis and DNA in temperate marine phytoplankton. Mar. Ecol. Prog. Ser. 211, 43–49. doi: 10.3354/meps211043
Hofstraat J. W., van Zeijl W. J. M., Peeters J. C. H., Peperzak L. (1990). “Flow cytometry and other optical methods for characterization and quantification of phytoplankton in seawater,” in Environment and pollution measurement sensors and systems, Book 1269 (Society of Photo Optical Engineers, Washington).
IMO (2018). “Code for approval of ballast water management systems (BWMS Code),” in IMO (ed) MEPC300(72), Book 300 (IMO, London).
IMO (2019). Description of the MPN serial dilution culture method + Motility method (London: Book PPR 7/INF.10).
IMO (2022). 2022 Guidance on Methodologies that May be Used for Enumerating Viable Organisms for Type Approval of Ballast Water Management Systems. Book BWM.2/Circ.61/Rev.1. 2022.
IMO (2023). Protocol for the verification of ballast water compliance monitoring devices (London, UK: IMO).
Irwin A. J., Finkel Z. V., Schofield O. M. E., Falkowski P. G. (2006). Scaling-up from nutrient physiology to the size-structure of phytoplankton communities. J. Plankton Res. 28, 459–471. doi: 10.1093/plankt/fbi148
Jarvis B., Wilrich C., Wilrich P. T. (2010). Reconsideration of the derivation of Most Probable Numbers, their standard deviations, confidence bounds and rarity values. J. Appl. Microbiol. 109, 1660–1667. doi: 10.1111/j.1365-2672.2010.04792.x
Kaeberlein T., Lewis K., Epstein S. S. (2002). Isolating “uncultivable” microorganisms in pure culture in a simulated natural environment. Science 296, 1127–1129. doi: 10.1126/science.1070633
Knight-Jones E. W. (1951). Preliminary studies of nanoplankton and ultraplankton systematics and abundance by a quantitative culture method. ICES J. Mar. Sci. 17, 140–155. doi: 10.1093/icesjms/17.2.140
Lundgreen K., Eckford-Soper L., Pedersen K. L., Holbech H. (2019). Development of a novel automated analytical method for viability assessment of phytoplankton used for validation of ballast water treatment systems. J. Appl. Phycol. 31, 2941–2955. doi: 10.1007/s10811-019-01817-6
MacIntyre H. L., Cullen J. J. (2016). Classification of phytoplankton cells as live or dead using the vital stains fluorescein diacetate and 5-chloromethylfluorescein diacetate. J. Phycol. 52, 572–589. doi: 10.1111/jpy.2016.52.issue-4
MacIntyre H. L., Cullen J. J., Whitsitt T. J., Petri B. (2018). Enumerating viable phytoplankton using a culture-based Most Probable Number assay following ultraviolet-C treatment. J. Appl. Phycol. 30, 1073–1094. doi: 10.1007/s10811-017-1254-8
Miller A. W., Frazier M., Smith G. E., Perry E. S., Ruiz G. M., Tamburri M. N. (2011). Enumerating sparse organisms in ships’ ballast water: why counting to 10 is not so easy. Environ. Sci. Technol. 45, 3539–3546. doi: 10.1021/es102790d
Nir R., YIsraeli Y., Lamed R., Sahar E. (1990). Flow cytometry sorting of viable bacteria and yeasts according to á-Galactosidase activity. Appl. Environ. Microbiol. 56, 3861–3866. doi: 10.1128/aem.56.12.3861-3866.1990
Peeters J. C. H., Peperzak L. (1990). Nutrient limitation in the North Sea: A bioassay approach. Netherlands J. Sea Res. 26, 61–73. doi: 10.1016/0077-7579(90)90056-M
Peperzak L. (2002). The wax and wane of Phaeocystis globosa blooms. Ph.D. Thesis. Rijksuniversiteit Groningen, The Netherlands, Groningen.
Peperzak L., Brussaard C. (2011). Flow cytometric applicability of fluorescent vitality probes on phytoplankton. J. Phycol. 47, 692–702. doi: 10.1111/j.1529-8817.2011.00991.x
Peperzak L., Colijn F., Vrieling E. G., Gieskes W. W. C., Peeters J. C. H. (2000a). Observations of flagellates and stars in colonies of Phaeocystis globosa (Prymnesiophyceae); a hypothesis for their position in the life cycle. J. Plankton Res. 22, 2181–2203. doi: 10.1093/plankt/22.12.2181
Peperzak L., Duin R. N. M., Colijn F., Gieskes W. W. C. (2000b). Growth and mortality of flagellates and non-flagellated cells of Phaeocystis globosa (Prymnesiophyceae). J. Plankton Res. 22, 107–119. doi: 10.1093/plankt/22.1.107
Peperzak L., Dyhrman S. T. (2006). “From microscope to magnet: probing phytoplankton population structure and physiology using mammalian antibodies,” in Algal Cultures, Analogues of Blooms and Applications. Ed. Rao D. V. S. (Science Publishers, Enfield USA).
Peperzak L., van Bleijswijk J. (2021). False-positive enterococci counts in seawater with the IDEXX Enterolert-E most probable number technique caused by Bacillus licheniformis. Environ. Sci. pollut. Res. 28, 10654–10660. doi: 10.1007/s11356-020-11342-6
Peperzak L., Zetsche E.-M., Gollasch S., Artigas L. F., Bonato S., Creach V., et al. (2020). Comparing flow cytometry and microscopy in the quantification of vital aquatic organisms in ballast water. J. Mar. Eng. Technol. 19, 68–77. doi: 10.1080/20464177.2018.1525806
Riemann B., Simonsen P., Stensgaard L. (1989). The carbon and chlorophyll content of phytoplankton from various nutrient regimes. J. Plankton Res. 11, 1037–1045. doi: 10.1093/plankt/11.5.1037
Romero-Martinez L., van Slooten C., Nebot E., Acevedo-Merino A., Peperzak L. (2017). Assessment of imaging-in-flow system (FlowCAM) for systematic ballast water management. Sci. Total Environ. 603, 550–561. doi: 10.1016/j.scitotenv.2017.06.070
Romero-Martinez L., van Slooten C., Nebot E., van Harten M., Peperzak L. (2024). Validation of ballast water indicative measuring devices with natural UV-treated water. Mar. Pollut. Bull. 209 (Part B), 117193. doi: 10.1016/j.marpolbul.2024.117193
Sinha R. P., Häder D.-P. (2002). UV-induced DNA damage and repair: a review. Photochem. Photobiol. Sci. 1, 225–236. doi: 10.1039/b201230h
Sournia A. (1978). Phytoplankton manual. Monographs on Oceanographic Methodology. 6, 337 (Paris: Unesco).
Sournia A., Chrétiennot-Dinet M.-J., Ricard M. (1991). Marine phytoplankton: how many species in the world ocean? J. Plankton Res. 13, 1093–1099. doi: 10.1093/plankt/13.5.1093
Stehouwer P., Buma A., Peperzak L. (2015). A comparison of six different ballast water treatment systems based on UV radiation, electrochlorination and chlorine dioxide. Environ. Technol. 36, 2094–2104. doi: 10.1080/09593330.2015.1021858
Stehouwer P., Liebich V., Peperzak L. (2013). Flow cytometry, microscopy, and DNA analysis as complementary phytoplankton screening methods in ballast water treatment studies. J. Appl. Phycol. 25, 1047–1053. doi: 10.1007/s10811-012-9944-8
Steinberg M. K., First M. R., Lemieux E. J., Drake L. A., Nelson B. N., Kulis D. M., et al. (2012). Comparison of techniques used to count single-celled viable phytoplankton. J. Appl. Phycol. 24, 751–758. doi: 10.1007/s10811-011-9694-z
Sun Z., Blatchley E. R. III (2017). Tetraselmis as a challenge organism for validation of ballast water UV systems. Water Res. 121, 311–319. doi: 10.1016/j.watres.2017.05.052
Tang Y. Z., Shang L., Dobbs F. C. (2022). Measuring viability of dinoflagellate cysts and diatoms with stains to test the efficiency of facsimile treatments possibly applicable to ships’ ballast water and sediment. Harmful Algae 114, 102220. doi: 10.1016/j.hal.2022.102220
Throndsen J. (1978). “The dilution-culture method,” in Phytoplankton manual. Ed. Sournia A. (UNESCO, Paris).
Throndsen J. (1982). “Oil pollution and plankton dynamics: III,” in Effects on flagellate communities in controlled ecosystem experiments in Lindåspollene, Norway, June 1980 and 1981, vol. 67. (Paris, France: Sarsia), 163–169.
Trindade de Castro M. C., Veldhuis M. J. W. (2019). Temporal changes in phytoplankton biomass and cellular properties; implications for the IMO ballast water convention. Environ. Technol. 40, 1455–1466. doi: 10.1080/09593330.2017.1423117
USCG (2012). “Standards for living organisms in ships’ Ballast water discharged in U.S. Waters (Final rule),” in (DHS) DoHS (ed) RIN 1625–AA32, Book 33 CFR 151 (Federal Register, Washington, DC).
Utermohl H. (1958). Limnologie: Mit 1 Tabelle und 15 abbildungen im Text und auf 1 Tafel. Internationale Vereinigung Für Theoretische Und Angewandte Limnologie: Mitteilungen. 9, 1–38. doi: 10.1080/05384680.1958.11904091
van Ierland E. T., Peperzak L. (1984). Separation of marine seston and density determination of marine diatoms by density gradient centrifugation. J. Plankton Res. 6, 29–44. doi: 10.1093/plankt/6.1.29
Veldhuis M. J. W., Kraay G. W. (2000). Application of flow cytometry in marine phytoplankton research: current applications and future perspectives. Scientia Marina 64, 121–134. doi: 10.3989/scimar.2000.64n2121
Veldhuis M. J. W., Kraay G. W., Timmermans K. R. (2001). Cell death in phytoplankton: correlation between changes in membrane permeability, photosynthetic activity, pigmentation and growth. Eur. J. Phycol. 36, 167–177. doi: 10.1080/09670260110001735318
Waterbury J. B., Willey J. M. (1988). “Isolation and growth of marine planktonic cyanobacteria,” in Methods in Enzymology, Volume 167. (Academic Press). doi: 10.1016/0076-6879(88)67009-1
Welschmeyer N., Kuo J. (2016). Analysis of Adenosine Triphosphate (ATP) as a rapid, quantitative compliance test for ships’ ballast water. (Moss Landing Marine Laboratories).
Wietzorrek J., Stadler M., Kachel V. (1994). Flow cytometric imaging - a novel tool for identification of marine organisms. Proceedings of OCEANS’94 (Brest, France: IEEE).
Yaginuma H., Kawai S., Tabata K. V., Tomiyama K., Kakizuka A., Komatsuzaki T., et al. (2014). Diversity in ATP concentrations in a single bacterial cell population revealed by quantitative single-cell imaging. Sci. Rep. 4, 6522. doi: 10.1038/srep06522
Keywords: serial dilution culture-most probable number, SDC-MPN, calculation correction, false-positives, false-negatives, phytoplankton, model, ballast water
Citation: Peperzak L (2024) The serial dilution culture-most probable number assay to estimate phytoplankton concentrations in ballast water: comments and improvements. Front. Mar. Sci. 11:1494598. doi: 10.3389/fmars.2024.1494598
Received: 11 September 2024; Accepted: 11 November 2024;
Published: 12 December 2024.
Edited by:
Wei Wang, Ocean University of China, ChinaReviewed by:
Matthew Wayne Parrow, University of North Carolina at Charlotte, United StatesRoberta Congestri, University of Rome Tor Vergata, Italy
Copyright © 2024 Peperzak. This is an open-access article distributed under the terms of the Creative Commons Attribution License (CC BY). The use, distribution or reproduction in other forums is permitted, provided the original author(s) and the copyright owner(s) are credited and that the original publication in this journal is cited, in accordance with accepted academic practice. No use, distribution or reproduction is permitted which does not comply with these terms.
*Correspondence: Louis Peperzak, bG91aXMucGVwZXJ6YWtAbmlvei5ubA==
†ORCID: Louis Peperzak, orcid.org/0000-0003-0691-2521