- 1Zoological Department III, Natural History Museum Vienna, Vienna, Austria
- 2Vienna Doctoral School of Ecology and Evolution, University of Vienna, Vienna, Austria
- 3Faculty of Sciences and Technology, University of Algarve, Faro, Portugal
- 4Department of Molecular Neurosciences, Center for Brain Research, Medical University Vienna, Vienna, Austria
- 5Haus des Meeres, Vienna, Austria
- 6Coral Reef Laboratory, School of Ocean and Earth Science, University of Southampton, Southampton, United Kingdom
- 7Central Research Laboratories, Natural History Museum Vienna, Vienna, Austria
- 8Department of Physiology and Pharmacology, Karolinska Institutet, Stockholm, Sweden
- 9CARMABI Foundation, Piscaderabaai, Willemstad, Curaçao
Reef-building corals have a variety of green fluorescent protein (GFP)-like proteins, also known as fluorescent proteins (FPs). These proteins have broad spectral properties covering most of the visible spectrum, with fluorophores fluorescing from cyan to red. However, the role of FPs is still a topic of debate and requires further investigation, particularly in the direction of mapping these FPs within the coral tissue and describing their cell- and tissue-level distributions. This study applied confocal laser scanning microscopy (CLSM) to investigate species-specific differences in the distribution of FPs in three coral taxa (Stylophora sp., Acropora sp., Echinopora sp.), combined with their photoacclimation response and that of associated symbiotic algae to light gradients in a controlled aquarium experiment. CLSM produced high-resolution images that enabled the identification of different FPs, their tissue distribution and quantification of their fluorescence intensity, as well as quantification of symbiont chlorophyll a (chl-a) fluorescence. Emission scans revealed three emission peaks between 490 - 501 nm (cyan, CFPs), 510 - 515 nm (green, GFPs), and 679 nm (chl-a fluorescence signal; Fchl) shared by all three studied species. The distribution of GFPs in Stylophora was concentrated in the intermesenterial muscle bands of the polyp, whereas CFPs were typically located at the tips of the tentacles. In contrast, Acropora and Echinopora exhibited agglomeration of CFPs and GFPs primarily in the epidermis. In general, species-specific differences in FP distribution remained unaltered during the experiment. However, linear regression models showed a significant negative relationship between CFP fluorescence intensity and light irradiance in Stylophora, whereas Echinopora exhibited a negative relation between chlorophyll fluorescence (Fchl) and light. In summary, the CLSM methodology provided a high-resolution tool to study coral FP patterns and symbiont response to irradiance, revealing ecophysiological differences among coral species at the tissue and cellular levels. CLSM has the potential to elucidate the intricacies of coral photobiology within the natural environment and to discern their adaptive responses in situ.
1 Introduction
Tropical reef-building corals live in a mutualistic symbiotic relationship with photoautotrophic dinoflagellates of the family Symbiodiniaceae, commonly referred to as zooxanthellae (LaJeunesse et al., 2018). These single-celled algae reside within coral tissues and convert sunlight energy into photosynthates, which they provide to the coral host. In return, the host provides shelter and essential nutrients to its photosynthetic partners (Muscatine, 1990). Light is the main energy source in tropical oligotrophic waters, thus the coral’s ability to capture and manage light is essential to its growth and survival, as well as to its reef-building capacity (Cohen et al., 2016; Hoegh-Guldberg, 1999). In order to adapt to varying light conditions such as imposed by the vertical attenuation of light with depth on the reef, the coral host uses a range of mechanisms. For example, larval settlement behavior appears to be directed at choosing a suitable light habitat (Mundy and Babcock, 1998; Price, 2010), and morphological plasticity at the colony level, including changes in skeletal properties and their light scattering capabilities (Enríquez et al., 2005, Enríquez et al., 2017), allows corals to modulate light within their colonies (Kaniewska et al., 2008, Kaniewska et al., 2014; Ow and Todd, 2010).
One fairly less understood mechanism to deal with light is the pigmentation of the coral host itself (Bollati et al., 2020; Iluz and Dubinsky, 2015; Reynolds et al., 2008; Roth and Deheyn, 2013; Warner et al., 2002). Host pigmentation is mainly associated with green fluorescent protein (GFP)-like proteins, which are responsible for producing the typical blue-green-red combination of colors exhibited by corals and additional non-fluorescent colors under specific irradiance conditions (Dove et al., 2001; Oswald et al., 2007; Salih et al., 2000). On the other hand, symbionts can also contribute to photoacclimation via changes in cell densities and their photopigment contents (Frade et al., 2008a). Symbiont photopigments include chlorophylls (a and c2), and carotenoids, with peridinin being the most prominent one contributing to the overall yellow-brownish coloration of the coral colony (Jeffrey and Haxo, 1968).
In reef-building corals, FPs are highly abundant and diverse and can be broadly categorized into two groups based on their absorptance and emission properties: fluorescent proteins (FPs) and chromoproteins (CPs) (Alieva et al., 2008). FPs and CPs both absorb light in the visible spectrum (400-700 nm), however only FPs re-emit it at longer wavelengths (fluorescence) (Alieva et al., 2008). Coral FPs display three basic and common color types: cyan, green, and red, and occasionally there are additional FP colors, including yellow FPs (Alieva et al., 2008). CPs are generally detected as pink, blue, and purple (Alieva et al., 2008). FPs and CPs comprise up to 14% of the soluble cellular proteins in coral tissues (Bollati et al., 2020; Leutenegger et al., 2007; Oswald et al., 2007), thus pointing to their physiological importance.
The high abundance and diversity of FPs observed in corals have led to the proposal of various functions for these proteins in the coral holobiont. FPs have been demonstrated to possess significant hydrogen peroxide scavenging activity (Palmer et al., 2009), facilitate larval settlement success (Kenkel et al., 2011), and serve as a thermal dissipator (Lyndby et al., 2016), but also to promote the attraction of symbionts (Aihara et al., 2019) and the attraction of prey (e.g., zooplankton) in deep habitats (Ben-Zvi et al., 2022). Nevertheless, a well-supported hypothesis suggests that FPs can modulate light use by the coral’s photoautotrophic endosymbionts. This is achieved either by shading them from excessive irradiance in shallow water (Salih et al., 2000; Smith et al., 2013; Gittins et al., 2015; Quick et al., 2018; Bollati et al., 2020) or by re-emitting light at photosynthetically-active wavelengths in deep-water, low-light environments (Schlichter et al., 1986; Salih et al., 2000; Smith et al., 2017; Bollati et al., 2021).
Coral FP expression exhibits variation at the inter- and intra-specific levels (Mazel et al., 2003; Salih et al., 2000; Dove et al., 2001). Across a variety of taxa, corals can be grouped based on their distinct patterns of FPs expression (Dizon et al., 2021; Gruber et al., 2008). On an intraspecific level, different color morphs can be distinguished by different contents of tissue concentrations of specific GFP-like proteins, which can be caused by the environmentally regulated expression of multicopy genes (Quick et al., 2018; Gittins et al., 2015; Kelmanson and Matz, 2003; Satoh et al., 2021). Corals either invest in expensive, high-level FP pigmentation, which offers benefits under light stress, or they rely on low tissue pigment concentrations and use the conserved resources for other purposes, an approach that is preferable in less light-exposed environments (Gittins et al., 2015).
The characterization of the distribution of FPs in coral tissues is still in its infancy, and the ecological function of these proteins is still a matter of debate. To the best of our knowledge, this is the first study to employ CLSM in combination with measurements of symbiont photobiology to investigate the distribution of FPs at tissue and cellular levels. Furthermore, this study aims to elucidate the acclimation response of FPs to irradiance in a controlled aquarium experiment, thereby testing the hypothesis that FPs can modulate light use by the coral’s photoautotrophic endosymbionts, for example by shading them in conditions of high light. Our research presents high-resolution visualizations of FP distributions in coral tissues and a quantitative analysis of their photoacclimation response in three distinct common species used in public aquaria. The technique produces meaningful results and we consider its application to study corals living under natural environmental conditions to be very promising.
2 Material and methods
2.1 Experimental design
Corals cultivated in exhibition tanks of the Haus des Meeres (HdM) aquarium in Vienna, Austria, were used for the present study. Replicate nubbins obtained from various colonies (4 colonies per species and 13 nubbins per colony, single genets) of Acropora sp., Echinopora lamellosa, and Stylophora pistillata (n=52 nubbins per species) were grown and acclimatized for three months in experimental aquaria, under a constant temperature of 26°C, and an irradiance of approximately 100 ± 20 µmol photons m-2 s-1, provided by an LED lamp with a color temperature of 10,000K and luminous flux of 3250 lm (AQUAEL Leddy Slim 36W Marine). This irradiance was similar to that received by corals in the exhibition tanks for 12h daily (100-140 µmol photons m-2 s-1; Quantum Meter QMSS, Apogee Inc., USA). The water is composed of an artificial sea salt premix (Reef Salt, Aqua Forest Inc., Poland) and drinking water, with a salinity of 35 PSU. Flow pumps (Turbelle Stream, Tunze Inc., Germany) provided the required water flow over the corals at a range of 5-10 cm/sec. A thermostatically controlled heater (Jaeger/Eheim Inc., Germany) maintained the water temperature. The inorganic composition of the seawater was analysed using ICP-OES (Oceamo Inc., Austria).
Following the acclimatization period, nubbins were exposed to irradiances of ~200 ± 30 µmol photons m-2 s-1, ~100 ± 20 µmol photons m-2 s-1 and ~50 ± 13 µmol photons m-2 s-1 (Smith et al., 2013), respectively referred as high, medium and low light levels in this study. The high light treatment used in this study does not necessarily correspond to the high irradiances (and certainly not the UV levels) observed in the shallow reef at noon for clear reef waters (Frade et al., 2008b). However, in our aquarium system, Echinopora exhibits optimal growth at light intensities above 50 µmol photons m-2 s-1, Stylophora at intensities around 80 µmol photons m-2 s-1, and Acropora at intensities above 100 µmol photons m-2 s-1. Therefore, 200 µmol photons m-2 s-1 is a notably high light intensity for Echinopora, while 50 µmol photons m-2 s-1 is relatively low for Acropora. 100 µmol photons m-2 s-1 is a medium light intensity to which all three species seem to be acclimatized and under which they demonstrate optimal growth.
The experiment lasted for 29 days with samples taken for physiological assays on day 0 (initial, before light treatment started), days 2, 9, 23, and 29 (see Supplementary Figure 1). At each sampling time, four whole nubbins from each species/light treatment were collected and transported in seawater at a constant temperature and in the dark to the laboratory of the Center of Brain Research (CBR) at the Medical University of Vienna where they were divided into two subsamples within two hours from sampling: 1) for host pigment physiology, subsamples were fixed in a 4% solution of paraformaldehyde (PFA) for 6 hours at 4°C, then moved to phosphate-buffered saline solution (PBS) and stored at 4°C until further processing; and 2) for symbiont photophysiological assays, subsamples were immediately frozen and kept at -80°C until determination of chlorophyll a (chl-a) concentrations and symbiont cell densities.
2.2 Sample preparation
To assess the acclimation response of host FPs to different light levels over time, we applied histological techniques to process all samples for further confocal microscopy observations. This included decalcification, fixation, embedding, and sectioning of coral tissue. PFA-fixed samples were decalcified in a 10% EDTA solution for at least one week until only the tissue remained. Usually, 2-3 weeks were needed, depending on the size of the fragment. Following this, the decalcified samples were treated with a cryoprotective sucrose solution (30%) and frozen at –20°C in OCT blocks until cryosectioning. Using a cryotome at a temperature of -20°C, a series of cross-tissue sections (50 μm-thick) were cut (parallel to the oral-aboral axis) and evenly distributed onto glass slides. Finally, the slides were stored at -20°C until analysis of FPs under a confocal microscope. All working steps were conducted under dim light conditions.
2.3 Image acquisition using confocal microscopy
In this study, coral tissue samples were imaged and processed using Zeiss LSM 780 and Zen Black processing software (2011 v14.0.16.201). Distinct lasers lines were used to excite and image the coral samples: (λ=405nm), (λ=458nm), (λ=488nm), (λ=514nm), (λ=561nm) and (λ=633nm) were used to image the coral samples. The excitation wavelength of each laser allowed for the visualization of fluorescence in the host (animal fluorescent proteins) and Symbiodiniaceae (chlorophyll a fluorescence) at a magnification of 20x and 64x. To ensure consistency during analysis of tissue slices, we selected the brightest focal plane of the specimen tissue slice (Shihan et al., 2021) for all subsequent analyses.
Before acquiring the image, we carefully calibrated several parameters for each species separately. This included fine-tuning the laser power to minimize photobleaching and fluorophore saturation, adjusting the pinhole diameter to reduce out-of-focus light, modifying the master gain to control the detector’s sensitivity, altering the digital offset to manage background noise, and adjusting the digital gain to re-amplify intensities (Shihan et al., 2021). The goal was to implement consistent settings for each species across treatments to facilitate final comparisons. All images were captured in 12-bit format, ensuring a uniform response across a broad range of fluorescent intensities (Shihan et al., 2021). The image frame size (pixel density resolution) was defined at 1024 x 1024 pixels, balancing both image quality (for quantification purposes) and processing time.
We noted that the fluorescence intensity of FPs was stronger and mostly concentrated around the polyp region for all three species, therefore, the focus was on acquiring images in this region. When examining branching corals such as Acropora and Stylophora, we analyzed slices from top to bottom of a nubbin, while for the plating coral Echinopora, we examined across the entire plate. In total, four individual nubbins for each species/treatment were analyzed, including 10 slices for each specimen, obtained homogeneously across the sample.
2.4 Quantification of fluorescence intensities and emission scans
Using the linear unmixing tool of the Zen Black software, individual signals were disentangled to accurately determine and quantify the spatial contribution of each fluorescent structure. Quantitative analysis resulted in several parameters including fluorescence intensity (total, mean and standard deviation), and the area covered by a certain fluorescence signal. To ensure accurate quantification, a threshold of 10% of the maximum intensity was established, effectively removing all pixels with low intensity. To determine the emission spectrum, including the emission peak, we used the lambda scan tool of the Zen Black software. We produced individual images ranging from 412 nm to 693 nm with a 4 nm interval, resulting in the emission spectrum of each fluorescence signal.
2.5 Symbiont photosynthetic activity
Photochemical efficiency was measured by analyzing the changes in chlorophyll fluorescence of photosystem II (PS II) from coral nubbins using a pulse-amplitude modulated (PAM) fluorometer (Diving-PAM; Walz Gmbh, Germany). The parameter that describes the maximum operating efficiency in the light-adapted state is the Fv’/Fm’ ratio, an established indicator of operational PSII efficiency in the light (Murchie and Lawson, 2013), from here onwards referred to as photochemical yield. In this study, the Diving-PAM settings of the “measuring light intensity” and “gain” were 10 and 6, respectively, and kept constant in order to compare photochemical yield across different coral samples. Measurements were performed on all sampling days (days 0, 2, 9, 23, 29), for all nubbins present in the experimental tank at any given time, before nubbins were collected for further analyses.
2.6 Determination of chlorophyll a concentrations and symbiont cell densities
We quantified the densities of zooxanthellae and chl-a concentrations after slowly thawing the respective subsample. The tissue was removed from the skeleton using an airbrush into 10 mL of PBS at room-temperature. The tissue-slurry was then homogenized by vortexing for 1 minute. For chl-a quantification, subsamples were centrifuged at 16,000 rcf for 5 minutes, and pellets frozen for further analysis. Subsamples for zooxanthellae density were fixed in 10% formaline to preserve the symbiont cells for counting.
Pellets for chl-a quantification were thawed on ice and resuspended in 1 mL of 100% acetone and kept in the dark at 4°C for 24 hours to extract chlorophyll. Samples were then centrifuged at 14,000 rcf for 3 minutes to spin down the celullar debris. Absorptance was measured at 630 and 663 nm for chlorophyll, and blank corrected. Samples below detection limit (based on the standards) were not included in the analysis. Chl-a concentrations were calculated based on Jeffrey and Humphrey (1975) equations:
Chl-a concentrations were then normalized to coral surface area (µg cm−2). Surface area of coral samples was measured using the aluminium foil paper wrapping method (Marsh, 1970). To determine zooxanthellae density, formaline-fixed subsamples were homogeneized for 1 minute, and 10 µL of homogenate was loaded onto a Neubauer haemocytometer (0.100 mm depth). Symbiodiniaceae cells were counted at 50x magnification using an Olympus CX31 light microscope for a minimum of 8 independent counts (each for a 0.1 µL volume) and until the coefficient of variation reached 15% to ensure the robustness of density determinations. Cell counts were normalized to coral surface area (cells cm−2).
2.7 Statistical analyses
Statistics were conducted using RStudio (v1.1.463). A series of linear regression models were constructed to evaluate the relationship between experimental irradiance and fluorescence properties (intensity, area) of FPs, as well as symbiont physiological parameters (including photochemical efficiency, areal symbiont cell density, areal chlorophyll concentration, and cellular chlorophyll concentration) in the last day of the experiment (day 29). The irradiance data were initially classified into three groups, designated as “low light”, “medium light”, and “high light.” However, due to relatively high variation in light incidence within a light group (amongst nubbins), the specimen-specific incident light was used as explanatory (continuous) variable. Assumptions of the regression models were evaluated by plotting residuals versus fitted values. Homoscedasticity was checked using the Breusch-Pagan test, while normality of residuals was tested by Q-Q plots. The dimensions of (fluorescent) coral tissue structures, including height, width, and area, were quantified (average ± SD) using the ImageJ software (v.: 2.14.0/1.54f).
3 Results
3.1 Confocal microscopy
CLSM was used to idenfity different FPs in the coral tissue, (together with the presence of chl-a in zooxanthellae), characterize their tissue distribution, and quantify their intensities and area of occurrence. FPs were characterized in all species, Acropora, Echinopora, and Stylophora (Figure 1). Emission scans revealed three emission peaks between 490-501, 510-515, and 679 nm shared by all three species, with optimum excitation at 405 and 488 nm. CFPs and GFPs are terms used to refer to emission peaks between 490-501 nm and 510-515 nm, respectively. The third emission peak at 679 nm corresponded to the fluorescence of chl-a (Fchl) present in the zooxanthellae. In addition, a peak at 581 nm was observed in Echinopora samples (referred as red, RFP), excited with 561 nm laser line. This emission peak was rare among samples, present only in three out of the 12 Echinopora samples analyzed. There was no variation in the emission peaks with light intensity, and no other fluorescent signals appeared or disappeared during the photoacclimation experiment.
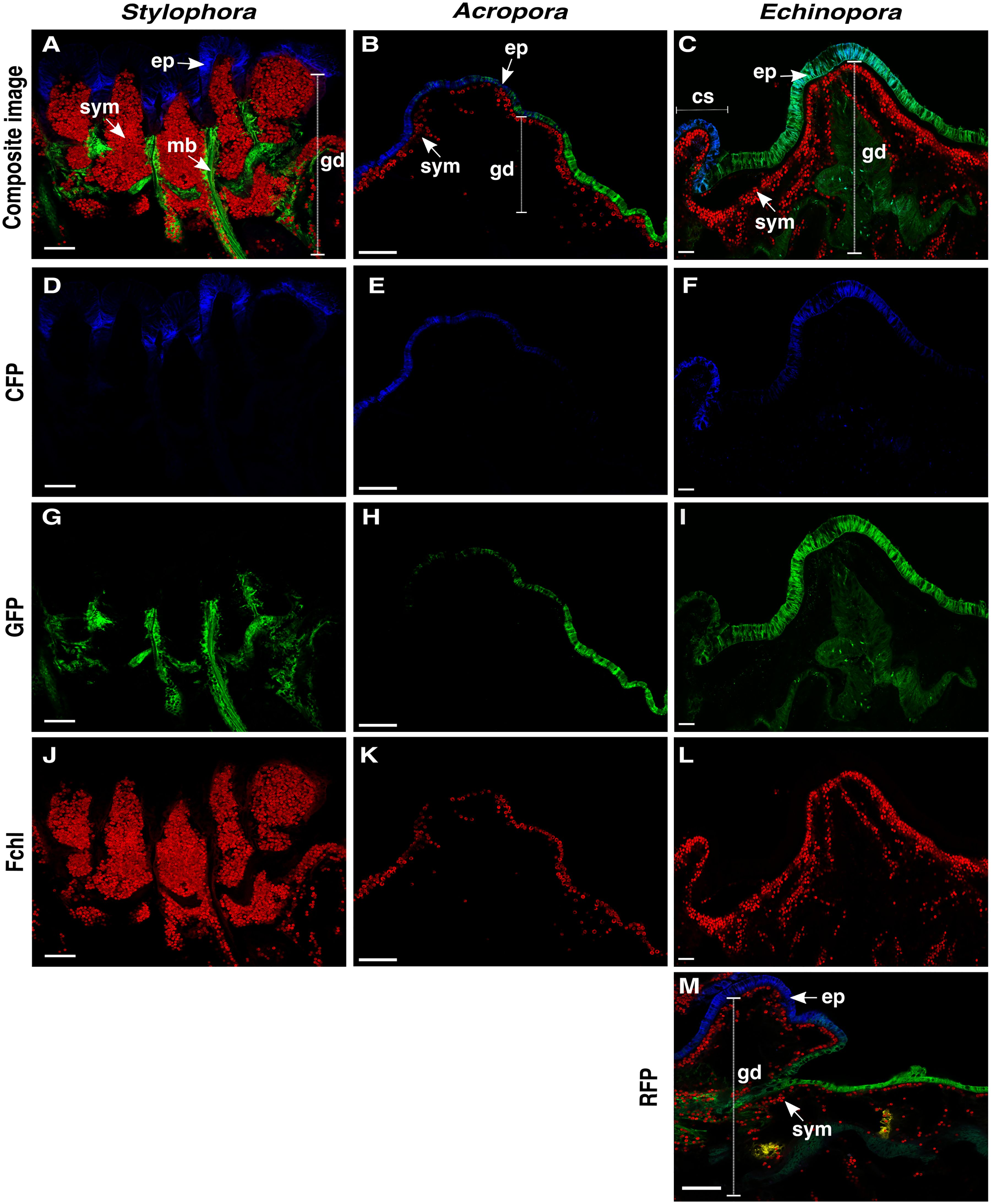
Figure 1. Tissue-level distribution of FPs in Stylophora, Acropora and Echinopora. Confocal microscopy images of 50-μm-thick coral tissue cross-sections containing a representation of a polyp, including epidermis (ep), gastrodermis (gd) and distribution of fluorescent proteins and the symbionts (sym) (N slices = 360). (A-C) Composite image of each species showing all fluorescent signals combined. (D-F) 405 nm excitation, 490-501 nm emission peak: CFPs (in blue) in the epidermis of the tips of the tentacles of Stylophora and in the epidermis of Acropora and Echinopora. In Echinopora, CFPs were concentrated towards the coenosarc (cs). (G-I) 488 nm excitation, 510 – 515 nm emission peak: GFPs (in green) in the intermesenterial muscle bands (mb) of the polyp in Stylophora, and in the epidermis of Acropora and Echinopora, and gastrodermis of Echinopora. (J-L) 405 nm excitation, 679 nm emission peak: Fluorescence signal from the chl-a within the symbiont cells (Fchl, in red). (M) 561 nm excitation, 581 nm emission peak: Composite image of Echinopora tissue showing a rare fluorescent signal (RFP, in yellow) concentrated in small parts of the gastrodermis. Scale bar for (A, C, D, F, G, I, J, L) = 300 μm; and for (B, E, H, K) = 100 μm.
The localization of FPs in relation to the different tissue layers was found to be consistent and stereotypical within species, yet exhibited variability across coral species (Figure 1). Stylophora (Figure 1A) displayed remarkable differences in comparison to Acropora (Figure 1B) and Echinopora (Figure 1C). In Stylophora, CFPs were typically detected in the epidermis on the tips of the tentacles (Figure 1D), while the GFPs were concentrated in the longitudinal muscle bands along the mesentery of the polyp (Figure 1G). This latter observation suggests that GFPs may be associated with the mesenterial ectoderm. The longitudinal muscle bands of GFPs in Stylophora (Figures 1A, G) exhibited an average length of 370 ± 86 μm and width of 38 ± 5 μm, and extended across more than half of the polyp’s height. Longitudinally across the sections the mean percentage of the epidermis covered by CFP (Figure 1D) was observed to be approximately 20%, and on the oral-aboral axis the epidermis had an average thickness of 80 ± 20 μm, which was either fully covered or completely depleted of CFP. In contrast, in Acropora (Figure 1B) and Echinopora (Figure 1C), both CFPs (Figures 1E, F) and GFPs (Figures 1H, I) were typically concentrated in the coral epidermis only, covering the entire cell layer, which had a thickness of 27 ± 15 μm in Acropora and 43 ± 15 μm in Echinopora. The distribution of RFP in Echinopora was concentrated in small areas of the gastrodermis (Figure 1M), covering approximately 1% of the total gastrodermis area in the confocal images.
Fluorescence intensity in general was stronger in the polyp region of both the Echinopora and Stylophora species. However, in Echinopora, GFPs were closer to the polyp mouth and CFPs were more concentrated towards the coenosarc. In Acropora, the fluorescence was observed to be present throughout the entire epidermis, with no evident differences between the polyp and coenosarc regions. Interestingly, a high concentration of symbionts was observed near CFPs and GFPs in all three species (Figures 1A–C). For instance, in Echinopora, the concentration of symbiont cells was notably higher (ca. 2,600 cells/mm²) in regions of gastrodermis contiguous to the oral epidermis in comparison to the concentration of symbiont cells (ca. 700 cells/mm²) towards the gastrovascular cavity (Figure 1C). In Stylophora, GFP was observed in the gastrodermis to line the mesenteries (Figure 1A), with the symbionts themselves consistently situated near the GFPs. In contrast, RFPs in Echinopora were typically concentrated in parts of the gastrodermis not packed with symbionts (Figure 1M).
In general, FPs in Echinopora, Acropora, and Stylophora were distributed diffusely (Figure 2), and no evident granules were observed in this study. At a cellular level, all epidermal cells of Echinopora and Acropora contained high GFP concentration (Figures 2H, I). In contrast, Stylophora exhibited high concentration of GFPs in the epidermis along the mesenteries adjacent to the symbionts (Figure 2G), and absence of GFP on the epidermis above the symbionts (unlike the pattern observed in Acropora and Echinopora). In Echinopora, a limited number of gastrodermal cells also exhibited GFPs (Figures 1I, 2I), albeit at a concentration that was lower than that observed in the epidermis.
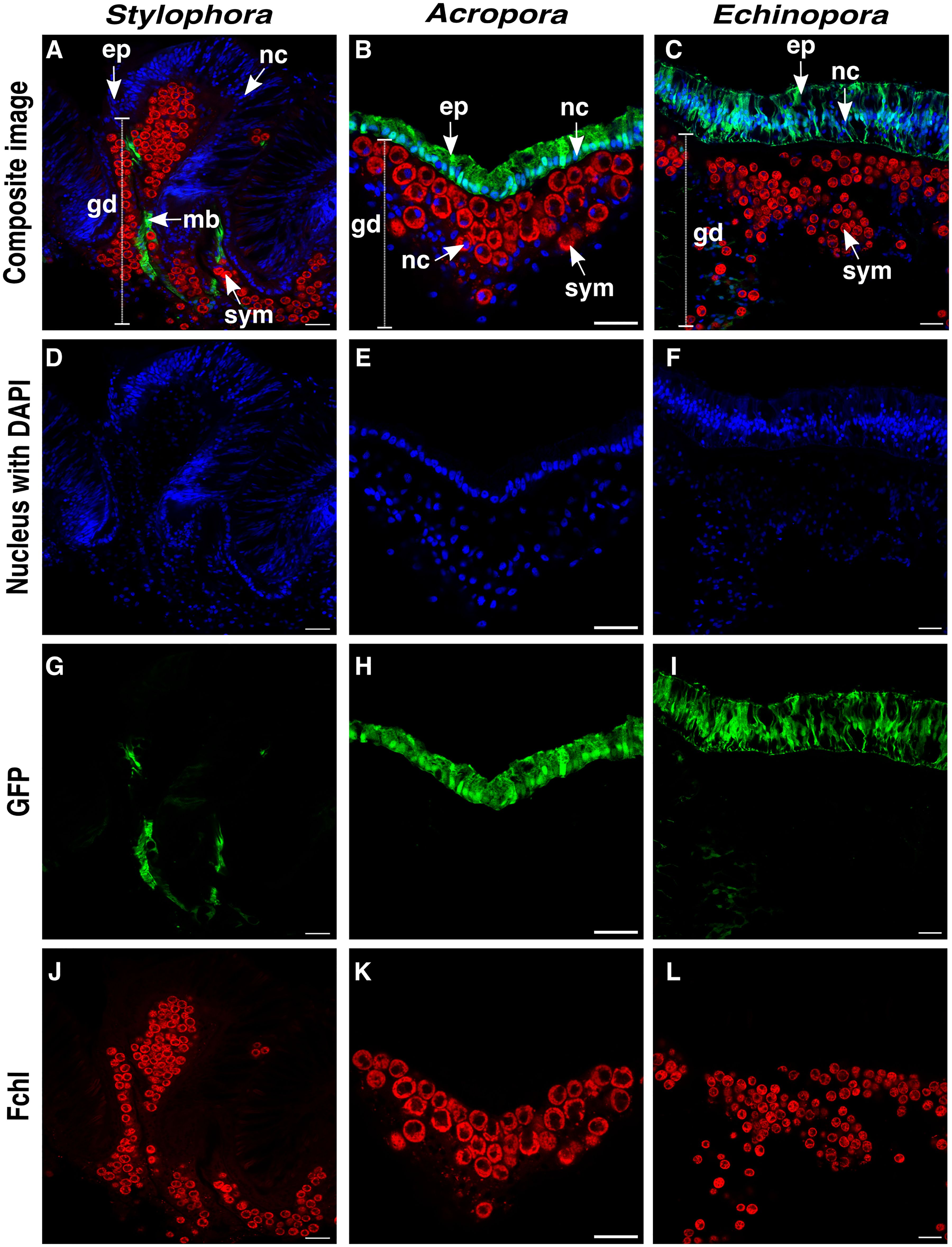
Figure 2. Cellular-level distribution of FPs in Stylophora, Acropora and Echinopora. (A-C) Composite image of cell nuclei (stained with DAPI), GFPs and chlorophyll fluorescence within the cells of Stylophora, Acropora and Echinopora, respectively. (D-F) Nuclei (nc) of epidermis (ep) and gastrodermis (gd) cells of each coral species. Cells in the epidermis are more compacted as compared to the gastrodemis cells. (G-I) Diffuse arrangement of GFPs within the epidermis cells. (J-L) Chlorophyll signal within the gastrodermis cells. Scale bar = 20 μm.
The abovementioned species-specific general appearance of FP distribution at the cellular and tissue level was maintained throughout the experiment (Figure 3). However, when fluorescence intensity was quantified, Stylophora exhibited significant variation in CFP intensity with light. Linear regression demonstrated a significant negative relationship between CFP intensity present in the tip of tentacles (epidermal cells) and experimental light conditions (y=1106.26−0.918×x; F(1,9)= 25.84, p< 0.05). In contrast, only Echinopora exhibited a negative relationship of average intensity of chlorophyll fluorescence (Fchl) and light intensity (y=1465.44−1.616×x; F(1,10)= 5.65, p< 0.05). No other FP differences were found in intensity or area of fluorescence (Figure 3) throughout the experiment.
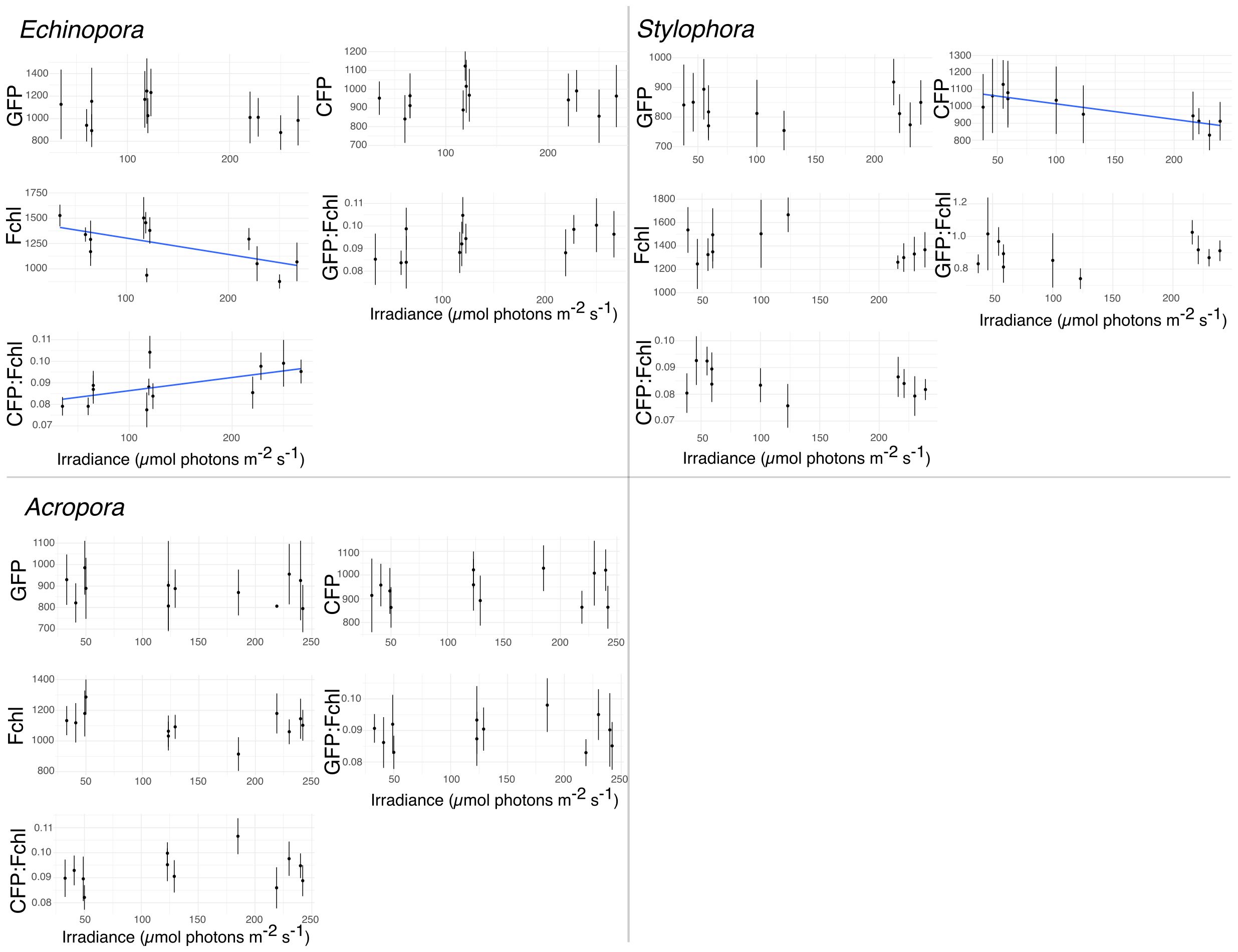
Figure 3. Variation in (mean) fluorescence intensity for different FPs and chl a (plus ratios) with irradiance intensity in Acropora, Echinopora and Stylophora. Linear regression modeled the response of Stylophora, Acropora, and Echinopora to light variation. Stylophora exhibited negative relation between CFPs and light intensity, while Echinopora exhibited negative correlation with chl-a fluorescence signal (Fchl) and light intensity. Acropora did not show any significant correlation.
3.2 Symbiont physiology assays
Among the symbiont parameters measured (areal symbiont cell density, areal chlorophyll concentration, and cellular chlorophyll concentration) there was a substantially stronger response to light irradiance than among the animal FP parameters (Supplementary Figure 2). Significant differences were observed in both Echinopora and Acropora in response to changes in incident light. Both species demonstrated a statistically significant reduction in chlorophyll per cell in response to increasing irradiance (Echinopora: y=0.1435−0.0001563×x; F(1,8) = 7.74, p< 0.05); Acropora: y=0.1568−0.0002395×x; F(1,9) = 9.33, p< 0.05). In contrast, Echinopora showed an increase in symbiont cell density with light (y=14.18 + 0.002766×x; F(1,8) = 11, p< 0.05), whereas Acropora exhibited a decrease in chlorophyll per area with increasing light (y=0.1649−0.0003051×x; F(1,9) = 9.33, p< 0.05). Stylophora did not yield any significant results with regards to changes in symbiont photophysiology with light intensity.
As expected, at the end of the experiment (day 29) all three species exhibited a statistically significant negative correlation between photochemical efficiency (photochemical yield, Fv’/Fm’) and experimental light intensity (Supplementary Figure 3), reflecting photoacclimation. Acropora exhibited (photochemical) yield values exceeding Y=0.600 in low-light conditions (~ 50 µmol photons m-2 s-1), while in light intensities exceeding 200 µmol photons m-2 s-1, yield significantly decreased to a minimum of ~ Y=0.400 (F(1,13) = 23.52 p< 0.05). In the case of Stylophora, the yield values observed in lower light conditions ranged between Y=0.470 and Y=0.600, exhibiting a notable decline in high light conditions, where they fell to around Y=0.400 (F(1,12) = 10.35 p< 0.05). In comparison, Echinopora displayed yield values around Y=0.600 in lower light conditions, while in high light conditions, these values decreased significantly, reaching Y=0.300 (F(1,8) = 15.09 p< 0.05).
4 Discussion
Understanding how FPs are implicated in coral photobiology is critical to resolve the controversies surrounding their ecological function. Previous studies have used CLSM as a tool to study the distribution of coral FPs (Ben-Zvi et al., 2015; Caldwell et al., 2017; Cox et al., 2007; Huffmyer et al., 2020; Salih, 2012; Salih et al., 2000), but few of them conducted experiments under controlled light levels to evaluate the response of coral FPs (and photosymbionts alike) and to analyze their distribution within the coral tissue (Ben-Zvi et al., 2015; Cox et al., 2007; Salih et al., 2000). In this study, we present a methodology to study acclimation of coral FPs to light variation using high resolution images captured by CLSM in order to infer the distribution of FPs within coral tissues at cellular resolution, and to retrieve quantitative data on their responses to external light.
4.1 Identification of FPs in coral tissues
In the present study, confocal microscopy was employed to localize and measure fluorescence and distinguish FPs through their emission curves, including their emission peaks. Based on spectral properties, coral FPs can be distinguished as follows: CFPs with an emission peak between 485 - 495 nm, GFPs between 500 – 525 nm, permanently RFPs (DsRED-type red) and photoconvertible RFPs (Kaede-type RED) between 570 - 595 nm (Alieva et al., 2008). In this study, all three species – Acropora sp., Echinopora sp., and Stylophora sp. - were found to have emission peaks between 490 - 501 and 510 - 515 nm, identified as CFPs and GFPs (Alieva et al, 2008), 581 nm identified as RFP (Alieva et al., 2008), and 679 nm identified as symbiont chl-a fluorescence, as expected (Figure 1; Mazel and Fuchs, 2003). Fluorescent corals, including Acropora and Stylophora, usually contain more than one type of FP, with the most abundant belonging to the cyan and green FPs groups (Alieva et al., 2008; D’Angelo et al., 2008; D’Angelo and Wiedenmann, 2012; Palmer et al., 2009; Roth et al., 2015; Satoh et al., 2021; Bollati et al., 2020; Cox et al., 2007; Salih et al., 2000). The different coral species analyzed in this study did not show substantial variation in the emission peaks derived from FPs. CFPs with an emission peak between 490 - 501 nm could be considered a borderline case. In this case, the proteins have a narrow “green-like” emission peak at 502 nm but a rather wide excitation peak, which is most common among cyan proteins (Alieva et al., 2008). However, as confocal microscopy does not provide the excitation curve, we could not verify this identification as either CFP or GFP.
The specific laser lines used for excitation and the obtained emission curves were satisfactory (except in the borderline CFP-GFP case) for the protein identification of each species based on previous research. It is worth mentioning that the optimal laser lines at 405 and 488 nm used in this study coincides with the maximum excitation peak for CFPs and GFPs, which falls within the range of 404 - 477 for CFPs and 478-508 nm for GFPs (Alieva et al., 2008; Ferreira et al., 2023). The most suitable laser to excite each fluorescence signal detected was determined by performing lambda scans at 4 nm intervals with all six available lasers ((λ=405nm), (λ=458nm), (λ=488nm), (λ=514nm), (λ=561nm) and (λ=633nm)), which improved accuracy of emission peaks for each fluorescence signal.
4.2 Distribution and quantification of FPs
FPs can be characterized according to their distribution across the colony, their localization (above, embedding the symbionts, or underneath the symbionts), and their arrangement (in granules or diffuse) within the coral tissue (Salih et al., 2000). Each of these features is critical to the understanding of the modulation of light in the coral tissue by FPs, including their interaction with symbionts.
The occurrence of multiple FPs with distinct distributions is suggestive of different roles in coral photophysiology (Alieva et al., 2008; D’Angelo et al., 2008). The localization and arrangement of coral FPs directly affect the optical properties inside the coral tissue and have been suggested to play a role in coral photophysiology, such as photoprotection when located above the symbionts, or enhancement of photosynthesis when located below and within the symbiont layer (Salih et al., 2000). In the present study, the localization of CFPs and GFPs in Acropora and Echinopora show them to be co-ocurrent in the epidermis cells and mostly concentrated above and close to the symbionts, suggesting that they may act synergistically to create a barrier against excessive light reaching the symbiont layer. In contrast, in Stylophora CFPs are concentrated in the tentacle tips, while GFPs next to the symbionts in the gastrodermis cells, which might suggest distinct ecological functions (see below).
In Echinopora and Stylophora, the FP fluorescence was concentrated mostly in the polyp region rather than in the coenosarc. This finding is supported by previous studies (Caldwell et al., 2017; Salih et al., 1998). In contrast, in Acropora, the fluorescence was observed to be present throughout the entire epidermis, with no discernible differences between the polyp and coenosarc regions. Furthermore, typically, in branching coral species, FP fluorescence has been shown to be more concentrated in areas of the colony most exposed to light (Salih et al., 2000; Salih et al., 1998). However, in this study, the fluorescence was distributed uniformly across single nubbin in the branching Stylophora and Acropora species. This distribution may be attributed to the relatively small size of the nubbins utilized in the present study. Additionally, no differences in fluorescent intensity were observed across the colony in the plate coral Echinopora.
The arrangement of coral FPs can be either granular or diffuse (Eyal et al., 2015; Lyndby et al., 2016; Salih et al., 2004). Host pigments with diffuse distribution are primarily situated in ectodermal cells and are frequently observed in corals across all depths (Eyal et al., 2015; Salih et al., 2004). While confocal imaging provides high-resolution images with good contrast, distinguishing between host pigments in granular versus diffuse distribution can be challenging. In our study we did not identify granular distribution of FPs for the studied species. Although optical coherence tomography (OCT) is often considered the optimal technique for detecting granular versus diffuse GFP-like protein distributions due to its high contrast (Wangpraseurt et al., 2017), confocal imaging provides high-resolution images with good contrast, where distinguishing between host pigments in granular versus diffuse forms is possible (pers. obs. Giulia Marchioro, for other coral species).
Quantitative analysis was also performed to better understand the response of coral FPs to light variation. The expression of GFPs and RFPs is typically observed under high irradiance conditions (>400 μmol photons m-2 s-1) and their tissue content increases proportionally to photon flux increases up to 700 μmol photons m-2 s-1, thus distinguishing a high-threshold FP group (Bollati et al., 2020; D’Angelo et al., 2008). In contrast, CFP expression is triggered under low irradiance conditions (~ 80-100 μmol photons m-2 s-1) and becomes saturated at high light exposure levels (>400 μmol photons m-2 s-1). This is thus referred to as the low-threshold group. In Stylophora, the mean fluorescence intensity of CFPs was higher at 50 than at 200 μmol photons m-2 s-1, suggesting that it can belong to the low-threshold protein group. In contrast, no significant increase in GFPs was observed in any of the species included in this study at 200 µmol photons m-2 s-1. This result could be attributed to insufficient light irradiance exposure of the coral samples subjected in the current study.
We found no significant differences in fluorescence intensity of FPs with light for Acropora and Echinopora. It is likely that both species initially invest in other mechanisms (e.g., symbiont photobiology) to adjust/acclimatize to light.
4.3 Coral light use strategies
The main objective of this work was initially to optimize the CLSM methodology to analyze FP identification and tissue distribution. On a positive note, further insights into the photoacclimation and adaptation strategies of each species can be infered from our results, demonstrating the utility of the method to answer broader eco-evolutionary questions. Photoacclimation mechanisms in corals include changes in host pigmentation, such as mediated by FPs as mentioned above, as well as changes by the symbionts (Falkowski and Dubinsky, 1981; Kaniewska and Sampayo, 2022). Symbiont photoacclimation can include changes in cell density and chl-a concentration, variation in symbiont type (which may have different environmental tolerances; Frade et al., 2008a; Iglesias-Prieto’ and Trench, 1994; Reynolds et al., 2008), and production of symbiont-derived mycosporine-like amino acids (MAAs) (which may confer protection against UV radiation; Rosic and Dove, 2011). Based on the combined analyses of host and symbiont physiology, we could identify different light use strategies among Acropora, Echinopora and Stylophora, which mainly differ in the component (host versus symbiont) responsible for the response.
Acropora did not exhibit host-regulated variation in intensity of FPs. Instead, it demonstrated decreased chl-a per cell and chl-a per surface area with increased light intensity. This aligns well with the negative correlation between chlorophyll concentration and light availability generally reported for aquatic photosynthetic organisms (Krause-Jensen and Sand-Jensen, 1998), and for coral reef systems (Vogel et al., 2015). Symbiont cell densities in Acropora did not change with increasing light intensity (200 ± 30 μmol photons m-2 s-1), which is concurrent with prior research for the species (Izumi et al., 2023). Acropora’s capability to tolerate high irradiance levels and dominate shallow-water habitats (Veron, 1995) is likely achieved by initially regulating cellular chlorophyll concentration in its symbionts. Prior research has demonstrated that Acropora yongei exhibits an increase in green fluorescence for high-light adapted corals (900 µmol photons m-2 s-1) and a decrease in low-light corals (30 µmol photons m-2 s-1), as compared to medium-light corals (300 µmol photons m-2 s-1). The current study employed high-light values of approximately 200-250 µmol photons m-2 s-1, which may have been insufficient to upregulate, for instance, the green FPs as compared to the other study.
In contrast, Stylophora pistillata is a depth-generalist species that can adapt to a wide range of light intensities (Kaniewska and Sampayo, 2022). Stylophora responded to light through changes in FPs under ca. 50 µmol photons m-2 s-1. CFPs fluorescence intensity increased at the tip of the polyp’s tentacles. Increased fluorescence intensity in low light in Stylophora could be associated with prey attraction, a phenomenon by which zooplankton has been shown to be actively attracted by (green) fluorescence (Ben-Zvi et al., 2022). Stylophora pistillata is known to increase the ingestion of microheterotrophs, including bacteria and oligotrich ciliates, at dim light compared to high irradiance, thus suggesting that plankton feeding complements autotrophic nutrition in S. pistillata (Ferrier-Pagès et al., 1998; Titlyanovl et al., 2000). In contrast to CFPs, GFP mean intensity did not vary with light and was mainly concentrated on the muscle bands along the mesenteries next to the symbiont-harboring gastrodermis. This suggests that CFPs and GFPs may play different physiological roles for the host in the present study. One possibility is that GFP, more concentrated than CFP in the tissue and characteristically surrounding the symbionts, could have a photoprotective function by absorbing excessive light and re-emitting it at wavelenghts (500-525 nm) not preferentially absorbed by the symbiont’s chlorophyll a.
In Echinopora, there was a decrease in the fluorescence intensity of chl-a and in the concentration of chl-a per cell with increasing light as evidenced by confocal analysis and symbiont physiological assays, respectively. Importantly, this demonstrates that the fluorescence intensity of chl-a, as measured in our protocol by confocal analysis, has the potential to serve as a proxy for cellular chlorophyll concentration in corals. Echinopora exhibited the lowest efficiency range in photochemical activity under high-light treatment conditions. Moreover, one sample of Echinopora under ca. 200 µmol photons m-2 s-1 showed mortality, while the other two exhibited bleaching. This is in line with reports of Echinopora being a shade-tolerant coral and having the capacity to acclimate to extremely low light conditions, such as 0.1% PAR of surface irradiance (Titlyanovl et al., 2000). It is likely that the response of Echinopora to light relates to the response of its symbionts, similarly to what has been observed for Acropora, rather than through FP mechanisms.
4.4 Final remarks
In conclusion, under current and future ecosystem perturbations caused by climate change, there is an urgent need to understand acclimation and adaptation of different coral species (Pörtner et al., 2010). The application of CLSM methodology provides a high-resolution tool for studying acclimation of coral FPs and symbiont response to light variation. The ability of the CLSM method to detect ecophysiological differences between coral species, even under small variations in experimental light, suggests that it may be useful in detecting early indicators of physiological stress. One of the major advantages of this method for this study is the ability to provide cell and tissue level resolution of the distribution of coral FPs. Numerous studies have focused on the study of coral FPs, but few of them have provided high-resolution visualization of the distribution of FPs within coral tissues during acclimatization experiments, which can decisively contribute to infering light-use strategies of different species. In future work of our research team, CLSM and associated methods will be used to characterize shifts in coral photobiology as response to environmental gradients in-situ.
Data availability statement
The original contributions presented in the study are included in the article/Supplementary Material. Further inquiries can be directed to the corresponding authors.
Author contributions
GMM: Writing – review & editing, Methodology, Conceptualization, Formal analysis, Writing – original draft. DC: Writing – review & editing, Formal analysis. TB: Conceptualization, Methodology, Writing – review & editing. DA-N: Writing – review & editing, Methodology, Conceptualization. MS: Writing – review & editing. CD’A: Writing – review & editing. LK: Writing – review & editing. IA: Writing – review & editing, Methodology, Conceptualization. PRF: Writing – review & editing, Methodology, Conceptualization.
Funding
The author(s) declare financial support was received for the research, authorship, and/or publication of this article. Giulia M. Marchioro is funded by the Portuguese Science and Technology Foundation (FCT) through fellowship 10.54499/2021.06934.BD (https://doi.org/10.54499/2021.06934.BD).
Conflict of interest
The authors declare that the research was conducted in the absence of any commercial or financial relationships that could be construed as a potential conflict of interest.
Publisher’s note
All claims expressed in this article are solely those of the authors and do not necessarily represent those of their affiliated organizations, or those of the publisher, the editors and the reviewers. Any product that may be evaluated in this article, or claim that may be made by its manufacturer, is not guaranteed or endorsed by the publisher.
Supplementary material
The Supplementary Material for this article can be found online at: https://www.frontiersin.org/articles/10.3389/fmars.2024.1483206/full#supplementary-material
References
Aihara Y., Maruyama S., Baird A. H., Iguchi A., Takahashi S., Minagawa J. (2019). Green fluorescence from cnidarian hosts attracts symbiotic algae. Proc. Natl. Acad. Sci. 116, 2118–2123. doi: 10.1073/pnas.1812257116
Alieva N. O., Konzen K. A., Field S. F., Meleshkevitch E. A., Hunt M. E., Beltran-Ramirez V., et al. (2008). Diversity and evolution of coral fluorescent proteins. PloS One 3, e2680. doi: 10.1371/journal.pone.0002680
Ben-Zvi O., Eyal G., Loya Y. (2015). Light-dependent fluorescence in the coral Galaxea fascicularis. Hydrobiologia 759, 15–26. doi: 10.1007/s10750-014-2063-6
Ben-Zvi O., Lindemann Y., Eyal G., Loya Y. (2022). Coral fluorescence: a prey-lure in deep habitats. Commun. Biol. 5, 537. doi: 10.1038/s42003-022-03460-3
Bollati E., D’Angelo C., Alderdice R., Pratchett M., Ziegler M., Wiedenmann J. (2020). Optical feedback loop involving dinoflagellate symbiont and scleractinian host drives colorful coral bleaching. Curr. Biol. 30, 2433–2445.e3. doi: 10.1016/j.cub.2020.04.055
Bollati E., Lyndby N. H., D’angelo C., Kühl M., Wiedenmann J., Wangpraseurt D. (2022). Green fluorescent protein-like pigments optimise the internal light environment in symbiotic reef-building corals. Elife 11, e73521.
Caldwell J. M., Ushijima B., Couch C. S., Gates R. D. (2017). Intra-colony disease progression induces fragmentation of coral fluorescent pigments. Sci. Rep. 7, 1–9. doi: 10.1038/s41598-017-15084-3
Cohen I., Dubinsky Z., Erez J. (2016). Light enhanced calcification in hermatypic corals: New insights from light spectral responses. Front. Mar. Sci. 2. doi: 10.3389/fmars.2015.00122
Cox G., Matz M., Salih A. (2007). Fluorescence lifetime imaging of coral fluorescent proteins. Microscopy Res. Technique 70, 243–251. doi: 10.1002/jemt.20410
D’Angelo C., Denzel A., Vogt A., Matz M. V., Oswald F., Salih A., et al. (2008). Blue light regulation of host pigment in reef-building corals. Mar. Ecol. Prog. Ser. 364, 97–106. doi: 10.3354/meps07588
D’Angelo C., Wiedenmann J. (2012). An experimental mesocosm for long-term studies of reef corals. J. Mar. Biol. Assoc. United Kingdom 92, 769–775. doi: 10.1017/S0025315411001883
Dizon E. G. S., Da-Anoy J. P., Roth M. S., Conaco C. (2021). Fluorescent protein expression in temperature tolerant and susceptible reef-building corals. J. Mar. Biol. Assoc. United Kingdom 101, 71–80. doi: 10.1017/S0025315421000059
Dove S. G., Hoegh-Guldberg O., Ranganathan S. (2001). Major colour patterns of reef-building corals are due to a family of GFP-like proteins. Coral Reefs 19, 197–204. doi: 10.1007/PL00006956
Enríquez S., Méndez E. R., Hoegh-Guldberg O., Iglesias-Prieto R. (2017). Key functional role of the optical properties of coral skeletons in coral ecology and evolution. Proc. R. Soc. B: Biol. Sci. 284, 20161667. doi: 10.1098/rspb.2016.1667
Enríquez S., Méndez E. R., Iglesias-Prieto R. (2005). Multiple scattering on coral skeletons enhances light absorption by symbiotic algae. In Limnol. Oceanogr 50, 4. doi: 10.4319/lo.2005.50.4.1025
Eyal G., Wiedenmann J., Grinblat M., D’Angelo C., Kramarsky-Winter E., Treibitz T., et al. (2015). Spectral diversity and regulation of coral fluorescence in a mesophotic reef habitat in the Red Sea. PloS One 10, e0128697. doi: 10.1371/journal.pone.0128697
Falkowski P. G., Dubinsky Z. (1981). Light-shade adaptation of Stylophora pistillata, a hermatypic coral from the Gulf of Eilat. Nature 289, 172–174. doi: 10.1038/289172a0
Ferreira G., Bollati E., Kühl M. (2023). The role of host pigments in coral photobiology. In Frontiers in Marine Science. Front. Media SA Vol. 10). doi: 10.3389/fmars.2023.1204843
Ferrier-Pagès C., Allemand D., Gattuso J. P., Jaubert J., Rassoulzadegan F. (1998). Microheterotrophy in the zooxanthellate coral Stylophora pistillata: Effects of light and ciliate density. Limnology Oceanography 43, 1639–1648. doi: 10.4319/lo.1998.43.7.1639
Frade P. R., De Jongh F., Vermeulen F., Van Bleijswijk J., Bak R. P. M. (2008b). Variation in symbiont distribution between closely related coral species over large depth ranges. Mol. Ecol. 17, 691–703. doi: 10.1111/j.1365-294X.2007.03612.x
Frade P. R., Englebert N., Faria J., Visser P. M., Bak R. P. M. (2008a). Distribution and photobiology of Symbiodinium types in different light environments for three colour morphs of the coral Madracis pharensis: Is there more to it than total irradiance? Coral Reefs 27, 913–925. doi: 10.1007/s00338-008-0406-3
Gittins J. R., D’Angelo C., Oswald F., Edwards R. J., Wiedenmann J. (2015). Fluorescent protein-mediated colour polymorphism in reef corals: Multicopy genes extend the adaptation/acclimatization potential to variable light environments. Mol. Ecol. 24, 453–465. doi: 10.1111/mec.13041
Gruber D. F., Kao H.-T., Janoschka S., Tsai J., Pieribone V. A. (2008). Patterns of fluorescent protein expression in scleractinian corals. Biol. Bull. 215, 143–154.
Hoegh-Guldberg O., Jones R. J. (1999). Photoinhibition and photoprotection in symbiotic dinoflagellates from reef-building corals. Mar. Ecol. Prog. Ser. 183, 73–86. doi: 10.1071/MF99078
Huffmyer A. S., Matsuda S. B., Eggers A. R., Lemus J. D., Gates R. D. (2020). Evaluation of laser scanning confocal microscopy as a method for characterizing reef-building coral tissue thickness and Symbiodiniaceae fluorescence. J. Exp. Biol. 223, jeb220335. doi: 10.1242/jeb.220335
Iglesias-Prieto’ R., Trench R. K. (1994). Acclimation and adaptation to irradiance in symbiotic dinoflagellates. I. Responses of the photosynthetic unit to changes in photon flux density. Mar. Ecol. Prog. Ser. 113, 163–175.
Iluz D., Dubinsky Z. (2015). Coral photobiology: new light on old views. Zoology 118, 71–78. doi: 10.1016/j.zool.2014.08.003
Izumi R., Tan E. S., Higa H., Shi Z., Takeuchi Y., Isomura N., et al. (2023). Effects of light intensity and spectral composition on the growth and physiological adaptation of Acroporid corals. Coral Reefs 42, 385–398. doi: 10.1007/s00338-023-02348-w
Jeffrey S. W., Haxo F. T. (1968). Photosynthetic pigments of symbiotic dinoflagellates (Zooxanthellae) from corals and clams. Biol Bull. 135, 149–165.
Jeffrey S. W., Humphrey G. F. (1975). New spectrophotometric equations for determining chlorophylls a, b, c1 and c2 in higher plants, algae and natural phytoplankton. Biochem. Physiol. Pflanzen (BPP) 167, 191–194. doi: 10.1016/S0015-3796(17)30778-3
Kaniewska P., Anthony K. R. N., Hoegh-Guldberg O. (2008). Variation in colony geometry modulates internal light levels in branching corals, Acropora humilis and Stylophora pistillata. Mar. Biol. 155, 649–660. doi: 10.1007/s00227-008-1061-5
Kaniewska P., Anthony K. R. N., Sampayo E. M., Campbell P. R., Hoegh-Guldberg O. (2014). Implications of geometric plasticity for maximizing photosynthesis in branching corals. Mar. Biol. 161, 313–328. doi: 10.1007/s00227-013-2336-z
Kaniewska P., Sampayo E. M. (2022). Macro- and micro-scale adaptations allow distinct Stylophora pistillata-symbiodiniaceae holobionts to optimize performance across a broad light habitat. J. Phycology 58, 55–70. doi: 10.1111/jpy.13215
Kelmanson I. V., Matz M. V. (2003). Molecular basis and evolutionary origins of color diversity in great star coral Montastraea cavernosa (Scleractinia: Faviida). Mol. Biol. Evol. 20, 1125–1133. doi: 10.1093/molbev/msg130
Kenkel C. D., Traylor M. R., Wiedenmann J., Salih A., Matz M. V. (2011). Fluorescence of coral larvae predicts their settlement response to crustose coralline algae and reflects stress. Proc. R. Soc. Lond. B. Biol. Sci. 278, 2691–2697.
Krause-Jensen D., Sand-Jensen K. (1998). Light attenuation and photosynthesis of aquatic plant communities. Limnology Oceanography 43, 396–407. doi: 10.4319/lo.1998.43.3.0396
LaJeunesse T. C., Parkinson J. E., Gabrielson P. W., Jeong H. J., Reimer J. D., Voolstra C. R., et al. (2018). Systematic revision of symbiodiniaceae highlights the antiquity and diversity of coral endosymbionts. Curr. Biol. 28, 2570–2580.e6. doi: 10.1016/j.cub.2018.07.008
Leutenegger A., D’Angelo C., Matz M. V., Denzel A., Oswald F., Salih A., et al. (2007). It’s cheap to be colorful: Anthozoans show a slow turnover of GFP-like proteins. FEBS J. 274, 2496–2505. doi: 10.1111/j.1742-4658.2007.05785.x
Lyndby N. H., Kuhl M., Wangpraseurt D. (2016). Heat generation and light scattering of green fluorescent protein-like pigments in coral tissue. Sci. Rep. 6, 26599. doi: 10.1038/srep26599
Mazel C. H., Fuchs E. (2003). Contribution of fluorescence to the spectral signature and perceived color of corals. Limnology Oceanography 48, 390–401. doi: 10.4319/lo.2003.48.1_part_2.0390
Mazel C. H., Lesser M. P., Gorbunov M. Y., Barry T. M., Farrell J. H., Wyman K. D., et al. (2003). Green-fluorescent proteins in Caribbean corals. Limnol. Oceanogr 48, 402–411.
Mundy C. N., Babcock R. C. (1998). Role of light intensity and spectral quality in coral 1 settlement: Implications for depth-dependent settlement? J. Exp. Mar. Biol. Ecol. 223, 235–255. doi: 10.1016/S0022-0981(97)00167-6
Murchie E. H., Lawson T. (2013). Chlorophyll fluorescence analysis: a guide to good practice and understanding some new applications. J. Exp. Bot. 64, 3983–3998. doi: 10.1093/jxb/ert208
Muscatine L. (1990). The role of symbiotic algae in carbon and energy flux in reef corals Ecosystems of the world. Coral Reefs Ecosyst. World 25, 75–87.
Oswald F., Schmitt F., Leutenegger A., Ivanchenko S., D’Angelo C., Salih A., et al. (2007). Contributions of host and symbiont pigments to the coloration of reef corals. FEBS J. 274, 1102–1122. doi: 10.1111/j.1742-4658.2007.05661.x
Ow Y. X., Todd P. A. (2010). Light-induced morphological plasticity in the scleractinian coral Goniastrea pectinata and its functional significance. Coral Reefs 29, 797–808. doi: 10.1007/s00338-010-0631-4
Palmer C. V., Modi C. K., Mydlarz L. D. (2009). Coral fluorescent proteins as antioxidants. PloS One 4, e7298. doi: 10.1371/journal.pone.0007298
Price N. (2010). Habitat selection, facilitation, and biotic settlement cues affect distribution and performance of coral recruits in French Polynesia. Oecologia 163, 747–758. doi: 10.1007/s00442-010-1578-4
Pörtner H. O. (2010). Oxygen-and capacity-limitation of thermal tolerance: a matrix for integrating climate-related stressor effects in marine ecosystems. J. Exp. Biol. 213, 881–893.
Quick C., D’Angelo C., Wiedenmann J. (2018). Trade-offs associated with photoprotective green fluorescent protein expression as potential drivers of balancing selection for color polymorphism in reef corals. Front. Mar. Sci. 5, 11. doi: 10.3389/fmars.2018.00011
Reynolds J. M., Bruns B. U., Fitt W. K., Schmidt G. W. (2008).Enhanced photoprotection pathways in symbiotic dinoflagellates of shallow-water corals and other cnidarians. Proc. Natl. Acad. Sci. 105, 13674–13678.
Rosic N. N., Dove S. (2011). Mycosporine-like amino acids from coral dinoflagellates. Appl. Environ. Microbiol. 77, 8478–8486. doi: 10.1128/AEM.05870-11
Roth M. S., Deheyn D. D. (2013). Effects of cold stress and heat stress on coral fluorescence in reef-building corals. Sci. Rep. 3, 1421. doi: 10.1038/srep01421
Roth M. S., Padilla-Gamiño J. L., Pochon X., Bidigare R. R., Gates R. D., Smith C. M., et al. (2015). Fluorescent proteins in dominant mesophotic reef-building corals. Mar. Ecol. Prog. Ser. 521, 63–79. doi: 10.3354/meps11108
Salih A. (2012). Screening reef corals for novel GFP-type fluorescent proteins by confocal imaging. Methods Mol. Biol. 872, 217–233. doi: 10.1007/978-1-61779-797-2_15
Salih A., Hoegh-Guldberg O., Cox G. (1998). “Photoprotection of symbiotic dinoflagellates by fluorescent pigments in reef corals.” in Proceedings of the Australian Coral Reef Society 75th Anniversary Conference. (Queensland, Australia: Ed. School of Marine Science, University of Queensland Brisbane), pp. 217–230.
Salih A., Larkum A., Cox G., Kühl M., Hoegh-Guldberg O. (2000). Fluorescent pigments in corals are photoprotective. Nature 408, 850–853. doi: 10.1038/35048564
Salih A., Larkum A. W., Cronin T. W., Wiedenmann J., Szymczak R., Cox G. C. (2004). Biological properties of coral GFP-type proteins provide clues for engineering novel optical probes and biosensors. Genetically Engineered Optical Probes Biomed. Appl. II 5329, 61. doi: 10.1117/12.548926
Satoh N., Kinjo K., Shintaku K., Kezuka D., Ishimori H., Yokokura A., et al. (2021). Color morphs of the coral, Acropora tenuis, show different responses to environmental stress and different expression profiles of fluorescent-protein genes. G3 11, jkab018. doi: 10.1093/g3journal/jkab018
Schlichter D., Fricke H. W., Weber W. (1986). Light harvesting by wavelength transformation in a symbiotic coral of the Red Sea twilight zone. Mar. Biol. 91, 403–407. doi: 10.1007/BF00428634
Shihan M. H., Novo S. G., Le Marchand S. J., Wang Y., Duncan M. K. (2021). A simple method for quantitating confocal fluorescent images. Biochem. Biophysics Rep. 25, 100916. doi: 10.1016/j.bbrep.2021.100916
Smith E. G., D’Angelo C., Salih A., Wiedenmann J. (2013). Screening by coral green fluorescent protein (GFP)-like chromoproteins supports a role in photoprotection of zooxanthellae. Coral Reefs 32, 463–474. doi: 10.1007/s00338-012-0994-9
Smith E. G., D’Angelo C., Sharon Y., Tchernov D., Wiedenmann J. (2017). Acclimatization of symbiotic corals to mesophotic light environments through wavelength transformation by fluorescent protein pigments. Proc. R. Soc. B: Biol. Sci. 284, 20170320. doi: 10.1098/rspb.2017.0320
Titlyanovl E. A., Leletkinl V. A., Dubinsky2 Z. (2000). Autotrophy and predation in the hermatypic coral stylophora pistillata in different light habitats. Symbiosis.
Veron J. E. N. (1995). Corals in space and time: the biogeography and evolution of the Scleractinia. Cornell University Press.
Vogel N., Meyer F. W., Wild C., Uthicke S. (2015). Decreased light availability can amplify negative impacts of ocean acidification on calcifying coral reef organisms. Mar. Ecol. Prog. Ser. 521, 49–61. doi: 10.3354/meps11088
Wangpraseurt D., Wentzel C., Jacques S. L., Wagner M., Kühl M. (2017). In vivo imaging of coral tissue and skeleton with optical coherence tomography. J. R. Soc. Interface 14, 20161003. doi: 10.1098/rsif.2016.1003
Keywords: photoacclimation, confocal microscopy, fluorescence, GFP-like protein, photosynthesis
Citation: Marchioro GM, Coelho D, Bouderlique T, Abed-Navandi D, Schagerl M, D’Angelo C, Kruckenhauser L, Adameyko I and Frade PR (2024) Confocal laser scanning microscopy reveals species-specific differences in distribution of fluorescent proteins in coral tissues. Front. Mar. Sci. 11:1483206. doi: 10.3389/fmars.2024.1483206
Received: 19 August 2024; Accepted: 28 October 2024;
Published: 21 November 2024.
Edited by:
Cliff Ross, University of North Florida, United StatesReviewed by:
Frank Smith, University of North Florida, United StatesChristine Ferrier-Pagès, Centre Scientifique de Monaco, Monaco
Copyright © 2024 Marchioro, Coelho, Bouderlique, Abed-Navandi, Schagerl, D’Angelo, Kruckenhauser, Adameyko and Frade. This is an open-access article distributed under the terms of the Creative Commons Attribution License (CC BY). The use, distribution or reproduction in other forums is permitted, provided the original author(s) and the copyright owner(s) are credited and that the original publication in this journal is cited, in accordance with accepted academic practice. No use, distribution or reproduction is permitted which does not comply with these terms.
*Correspondence: Giulia M. Marchioro, YTEyMTM5NjkyQHVuZXQudW5pdmllLmFjLmF0