- Ministry of Education Key Laboratory for Ecology of Tropical Islands, Key Laboratory of Tropical Animal and Plant Ecology of Hainan Province, College of Life Sciences, Hainan Normal University, Haikou, China
Butylparaben (BuP) is a ubiquitous preservative in cosmetics, foods, and medicine. As an emerging pollutant, it has gained wide attention in recent years. Because amphibians have both aquatic and terrestrial life stages and high skin permeability, they are sensitive to environmental changes. To assess the toxic effects of BuP on amphibians, the present study was conducted on the spot-legged tree frog (Polypedates megacephalus) tadpoles. In the present study, LC50 of BuP for 96 h exposures was 3509 µg/L. Then, the tadpoles were exposed to 0, 350 and 2100 µg/L BuP (hereafter named as CK, B1 and B2) for 12, 24 and 48 h, the oxidative stress and apoptosis in the liver were analyzed. The results showed that the content of MDA and activity of SOD and GSH-PX were increased with an increase in BuP exposure. BuP stress significantly altered the expression of Nrf2-Keap1 signal pathway genes. An increase in the expression of JAK-STAT signal pathway genes was observed with an increase in the exposure to BuP concentration at 24 and 48 h. In contrast, the expression of negative regulators of JAK-STAT signal pathway were significantly increased at 12 h and decreased at 24 and 48 h. A significant decrease in pro-apoptosis genes expression (Bax and Caspase3) were observed at 12 h but increased at 24 and 48 h, while the expression of anti-apoptosis gene (Bcl-2) decreased at 24 and 48 h. These results provide a valuable reference point for assessing the ecological and health risks associated with BuP in the environment.
1 Introduction
Amphibians stand at the forefront of a global biodiversity crisis (Whitfield et al., 2007). According to the second Global Amphibian Assessment findings, amphibians are the most threatened class of vertebrates (40.7% of species are globally imperiled) (Luedtke et al., 2023). The number of extinct amphibian species was 23 by 1980, and four times more by 2022. Among these factors resulting in the decline of the amphibian population, water pollution is one of the leading causes (Park and Do, 2023). Because of the hypertonicity of the skin, external fertilization and embryonic development, amphibians are sensitive to the pollutants in water, which will affect their physiological activities and growth (Brühl et al., 2011). Some studies have shown that the sharp decline in the population of the northern dusky salamander (Desmognathus fuscus fuscus) population in the Acadia National Park (Maine, USA) has been linked to mercury and aluminum pollution, and the decline of the mountain yellow-legged frog (Rana muscosa) population was caused by airborne pesticide pollutions (Slaby et al., 2019). Therefore, the relationship between water pollution and amphibian population is of great concern.
Parabens are used in a variety of food, pharmaceuticals and personal care products due to their broad spectrum of antimicrobial activity and their preservative properties. They are esters of p-hydroxybenzoic acid with alkyl substituents including methylparaben (MeP), ethylparaben (EtP), propylparaben (PrP), butylparaben (BuP) and pentylparaben (PeP) (Darbre et al., 2004). Traditionally seen as harmless, non-polluting, and non-irritating, parabens have recently been scrutinized for possible toxicity. Recently, more and more research has focusing on the ubiquitous presence of BuP, their environmental metabolites and high levels of parabens in the human body. Among alkyl parabens, BuP is the most powerful and has the most substantial estrogenic effects (Moreno-Marenco et al., 2020). In the meanwhile, toxicity studies including BuP become more common, because BuP is readily absorbed and maintained in bodily tissues. Ingestion, inhalation, or skin absorption of BuP can cause oxidative stress and harm the endocrine system (Belenguer et al., 2014). It can alter enzyme activity and cellular homeostasis inducing organ toxicity and damaging tissues through oxidative imbalance. This leads to damage to lipids, DNA, and proteins, which in turn triggers the development of various diseases (Aydemir et al., 2019). Some studies on BuP-induced oxidative stress mainly focused on mice liver, human spermatozoa, rat testis and turtle liver (Samarasinghe et al., 2018; Schreiber et al., 2019). There is still a lack of available data on the effects of BuP on the early life stages of amphibians.
Amphibians are very susceptible to environmental changes and contaminants in the aquatic ecosystem because of their porous skin, which is essential for gas exchange and osmotic balance (Linder et al., 2010). Compared to other model organisms like zebrafish, amphibians have not been the subject of many ecotoxicological studies, despite being excellent bioindicator species (Burgos-Aceves et al., 2022; Castro-Castellon et al., 2022; Dong et al., 2022). Furthermore, water quality standards developed based on information on fish toxicity do not protect amphibians (Sparling et al., 2010). Numerous studies have demonstrated that the physiological indices associated with oxidative stress in aquatic animals may serve as an invaluable foundation for tracking water pollution levels and assessing the biological safety of contaminants. It can be used as a bioindicator species for assessing environmental risk. In this study, the spot-legged tree frog Polypedates megacephalus tadpole was chosen to study the toxicity of BuP because it widely distributes throughout subtropical and tropical areas such as China, India, northern Vietnam, and so on (Zug, 2022). The study aimed to ascertain the detrimental effects and threshold concentrations of BuP on P. megacephalus tadpoles. Firstly, the LC50 concentration of BuP in the tadpoles was studied. Secondly, according to the LC50 concentration, the responses of the antioxidant system and oxidative damage in the livers of the tadpoles after 12, 24 and 48 h of exposure to BuP were analyzed. The present investigation offers factual support for evaluating the possible ecological hazards associated with BuP in amphibians.
2 Materials and methods
2.1 Animals culture and handling
In July, 2021, P. megacephalus tadpoles were collected from the puddles in the vegetable garden near Dongzhai Harbor (Haikou, Hainan, China). The tadpoles were acclimated in 5 L glass tanks with dechlorinated water for at least 3 d before the experiment at Hainan Normal University, and the individuals at Gosner stage (GS) 25 (Gosner, 1960) were chosen to study. The egg yolk was fed to them daily, and the water was changed. The water temperature was 28 ± 1°C and the photoperiod varied naturally. The pH values of the culture water were maintained at 7.47 ± 0.12, and the dissolved oxygen concentration was 8.32 ± 0.25 mg/L.
BuP [CAS: 94-26-8, Purity: 99%] was acquired from Macklin (Shanghai, China). For complete dissolution, 75% ethanol was added into the BuP solution, ensuring that the ethanol concentration in the water did not exceed 1 μL/L. The preliminary experiment indicated that the ethanol concentration of ethanol < 1 μL/L was safe for the tadpoles.
Approval for this study was obtained from the ethics committee overseeing experimental animal research at the Hainan Ecological and Environmental Education Center (No: HNECEE-2020-003).
2.2 LC50 of BuP to the tadpoles
Tadpoles were exposed to five groups with BuP concentrations of 0, 400, 800, 1600, 3200 and 6400 µg/L, respectively, and the exact concentrations of BuP were 0, 398.21, 789.54, 1602.36, 3195.47 and 6394.62, respectively, which were analyzed by HPLC (Agilent 1260, USA). The live and dead tadpoles were scored after exposure, and the percent mortality was determined. The experiment was repeated thrice independently, using 20 tadpoles of Stage 25 per treatment group. EPA Probit Analysis Program (Version 1.5) was employed to determine LC50.
2.3 Acute exposure of BuP to the tadpoles
The 96 h-LC50 of BuP to the tadpoles, the safe concentration (SC) = 0.1 × LC50, 96 h = 350 µg/L (Zang et al., 1993), a higher concentration group used 6-fold SC is 2100 µg/L. So, three groups of 0, 350 and 2100 µg/L BuP exposure concentration (for all the other assays listed in this paper as CK, B1 and B2) were set up. To ensure the experimental integrity, an equal amount of alcohol in the group of 2100 µg/L is added into the other two groups (0 and 350 µg/L). The exact concentrations of BuP in the groups of 350 and 2100 µg/L groups were 348.21 and 2103.01, respectively, which were analyzed by HPLC (Agilent 1260, USA). Water parameters were monitored daily and adjusted as necessary. Thirty-six tadpoles were randomly selected in each group. Liver samples were collected after exposure to BuP for 12, 24 and 48 h.
2.4 Biochemical analysis
Following the procedures described by Liang et al. (2020), 0.1 g of liver tissue was homogenized with 1ml ddH2O in a high-throughput tissue grinder (CIENTZ-48, Ningbo, China). The homogenate underwent centrifugation at 3,000 rpm for 10 min at 4°C, and the resulting supernatant was retained for biochemical analysis. The customized ELISA kits produced by Nanjing Jiancheng Bioengineering Institute (Nanjing, China) were used to measure the activities of superoxide dismutase (SOD), malondialdehyde (MDA) and glutathione reductase (GSH-PX) in the liver.
2.5 RNA extraction and qRT-PCR
The liver samples were extracted by TriZol (Invitrogen, Carlsbad, USA) according to the standard manufacturer’s protocol. The RNA was dissolved in 0.1% DEPC water, and its integrity and concentration were measured by 1.2% agarose gel electrophoresis and an ultramicro spectrophotometer (Thermo Fisher, USA). The RNA was kept in an ultra-low temperature refrigerator set at -80°C. The cDNA was synthesized according to the instructions of the manufacturer of the Prime Script Reverse Transcriptase (Takara, Tokyo, Japan). A two-step procedure following the SYBR®PrimeScript™ Kit (Takara, Tokyo, Japan) instructions was used to detect the mRNA expression levels of some genes. The primers were designed using the NCBI online primer design tool and the target genes are listed in Table 1. Relative expression was calculated using the 2-ΔΔCt method with β-actin as the internal control.
2.6 TUNEL assay
Sections of the liver were embedded in paraffin wax, and xylene was used to fix the samples in 4% paraformaldehyde fixative, and ethanol was used to dehydrate them. Proteinase K working solution was applied to the tissue followed by a membrane-breaking working solution. The TUNEL reaction solution was applied according to the Tunel Cell Apoptosis Detection Kit (Servicebio, Wuhan, China) after DAPI re-staining and sealed with a sealer to prevent fluorescence quenching. The images were observed and captured using a Nikon light microscope (Nikon, Eclipse C1 and DS-U3, Japan).
2.7 Data analysis
Experiment results are reported as mean ± S.E., and were analyzed using SPSS 24.0 statistical software. One-way ANOVA was used to evaluate the data after ensuring that the data were homogeneous regarding variances and normality. If one of the non-conforming and was used nonparametric tests. In statistical tests, differences were considered significant when P < 0.05.
3 Results
3.1 LC50 value of BuP for P. megacephalus tadpoles
The cumulative mortality and LC50 of BuP on the tadpoles are shown in Table 2. After 96 h of exposure, the mortality rate of tadpoles in the control group was 0, the cumulative mortality rate of tadpoles in the low-concentration group (BuP 400 µg/L) was 10%, and the mortality rate of tadpoles in the high-concentration group (BuP 6400 µg/L) reached 80%, with the LC50 of 6748, 5183, 4182 and 3509 µg/L, respectively.
3.2 Effect of BuP on MDA content and antioxidant enzyme activity in the liver
The levels of hepatic MDA were shown to be substantially elevated after exposure to BuP at 12 h (p < 0.05). Notably, the B2 group exhibited the highest MDA content after 48 h of exposure (Figure 1A). The SOD activity was shown to be considerably elevated after exposure to BuP at 12, 24 and 48 h. The maximum level of SOD activity was seen after 24 h of stress in the B2 group (Figure 1B). The GSH-PX activity exhibited a considerable increase in the B2 group after 48 h of stress, demonstrating a notable difference when compared to the control group (p < 0.05). However, no significant changes in GSH-PX activity were seen after 12 and 24 h of exposure (Figure 1C).
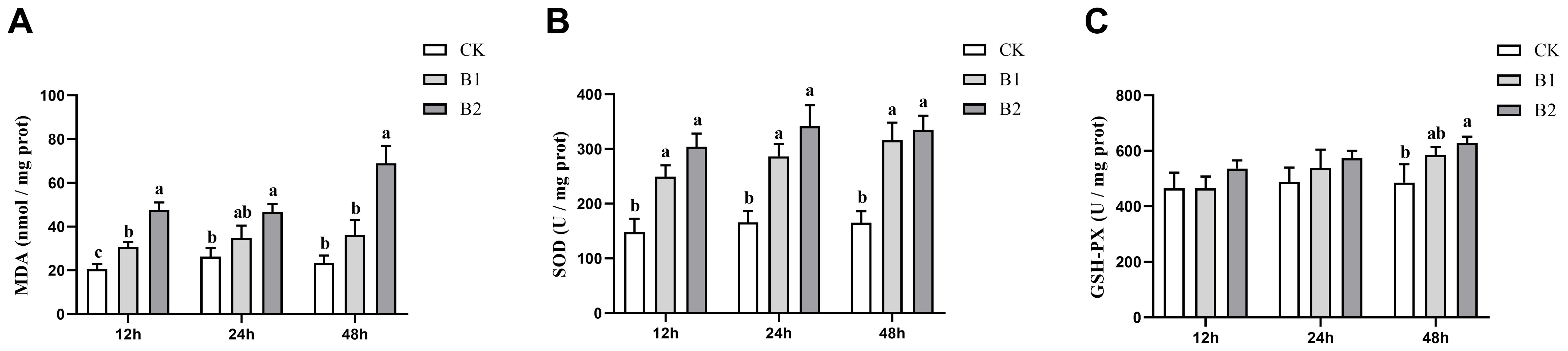
Figure 1. Effect of BuP on the MDA levels and antioxidant enzyme activity in the liver of P. megacephalus tadpoles. (A) MDA content; (B) SOD activity; (C) GSH-PX activity. Different lowercase letters represent significant difference between groups at the same sampling time (p < 0.05).
3.3 Effect of BuP on the mRNA expression of antioxidant genes in the liver
The mRNA expression levels of Nrf2 exhibited a statistically significant increase (p < 0.05) compared to the control group after 12 h exposure to BuP in both the B1 and B2 groups. The B1 group was 1.21-fold and the B2 group was 1.50-fold of the control group. However, after 24 h of exposure, the B1 group was significantly higher 1.32-fold than the control group. Subsequently, after 48 h, no significant differences were observed in the treated groups (Figure 2A). The mRNA expression levels of Keap1 in the B1 and B2 groups exhibited substantial reductions compared to the control group after 12, 24 and 48 h of exposure (Figure 2B). The levels of TOR mRNA expression exhibited a significant elevation in all exposure groups after 12, 24 and 48 h of treatment (p < 0.05). Notably, the B2 group had the highest expression level at the 12 h time point of exposure was 4.32-fold of the control group (Figure 2C). The mRNA expression level of S6K1 exhibited a substantial rise in the B2 group after 24 h exposure was 1.24-fold of the control group. However, in the B1 group, there was a significant increase in S6K1 mRNA expression after 48 h of exposure, which was 1.30-fold of the control group, but in the B2 group, a reduction in expression was seen (Figure 2D). The mRNA expression level of 4E-BP1 exhibited a significant decrease in the B2 group after 12 h of exposure was 0.37-fold of the control group. However, these levels showed recovery after 24 h of exposure. Subsequently, both the B1 and B2 groups demonstrated a significant increase in 4E-BP1 mRNA expression levels after 48 h of exposure (1.22-, 1.33-fold of the control group, respectively), with a notable distinction when compared to the control group (p < 0.05; Figure 2E).
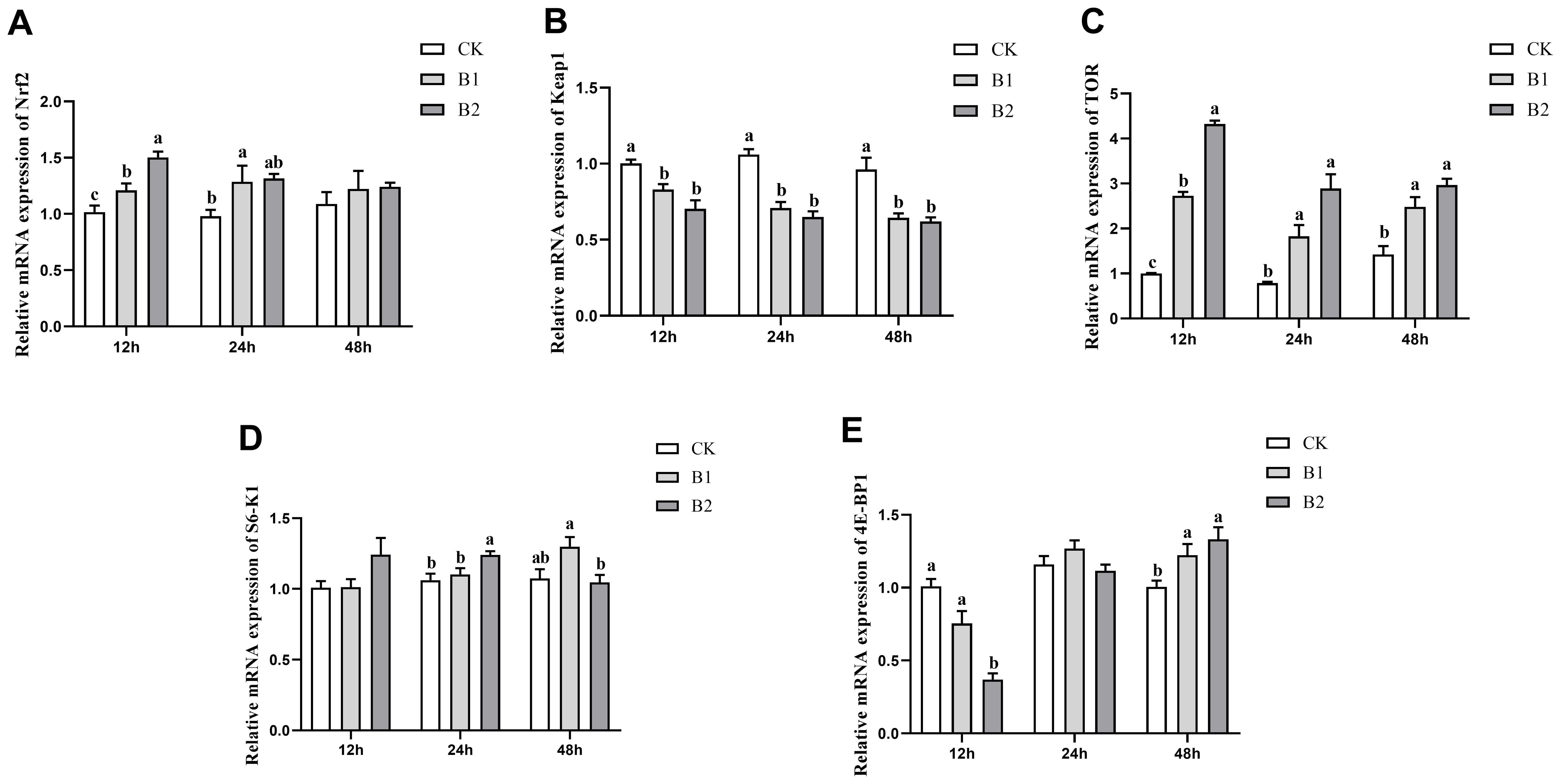
Figure 2. Effect of BuP on the mRNA expression of antioxidant genes in the liver of P. megacephalus tadpoles. (A) relative mRNA expression of Nrf2; (B) relative mRNA expression of Keap1; (C) relative mRNA expression of TOR; (D) relative mRNA expression of S6-K1; (E) relative mRNA expression of 4E-BP1. Different lowercase letters represent significant difference between groups at the same sampling time (p < 0.05).
3.4 Effect of BuP on the mRNA expression of JAK-STAT pathway response
The hepatic JAK1 expression levels in the B1 and B2 treatment groups after 12 h exposure showed no significant difference compared to the control group. However, after 24 h of exposure, the expression level of the B1 group showed a significant increase, whereas the B2 group exhibited a slightly lower expression level than the B1 group. After 48 h of exposure, the hepatic JAK1 expression levels in the B2 group showed a statistically significant increase (1.33-fold) compared to the control group (p < 0.05). The hepatic JAK2 expression levels exhibited a significant increase in the B1 and B2 groups (1.14-fold, 1.13-fold, respectively) compared to the control group during 48 h of stress (p < 0.05). The hepatic JAK3 expression levels exhibited a statistically significant increase at 24 and 48 h of exposure to BuP in the B1 and B2 groups when compared to the control group (p < 0.05). Additionally, the expression levels of STAT3 demonstrated a significant increase in the B2 group after 24 h and 48 h of exposure to BuP.
The mRNA expression levels of hepatic SOCS1 and SOCS2 in the B1 and B2 treatment groups exhibited a significant increase after 12 h, with the B1 group showing the highest expression was 1.33-fold of the control group. Conversely, the mRNA expression levels of hepatic SOCS1 and SOCS2 in the B1 and B2 treatment groups were significantly lower than those in the control group after 24 h of exposure (p < 0.05). However, these levels rebounded after 48 h, with no significant differences observed in comparison with the control group. The hepatic mRNA expression levels of SOCS3 and PIAS1 were significantly elevated after 12 h of exposure in the B1 group (1.41- and 1.45-fold of the control group, respectively). Additionally, PIAS1 expression in the B2 group also showed a significant increase. However, after 24 and 48 h, both SOCS3 and PIAS1 expression levels in the B1 and B2 groups were significantly reduced. The hepatic mRNA expression levels of PIAS2 and PIAS3 were shown to be increased after 12 h of stress in the B1 group, reaching their highest levels (1.45- and 1.25-fold of the control group, respectively), and then reduced after 24 and 48 h of stress (Figure 3).
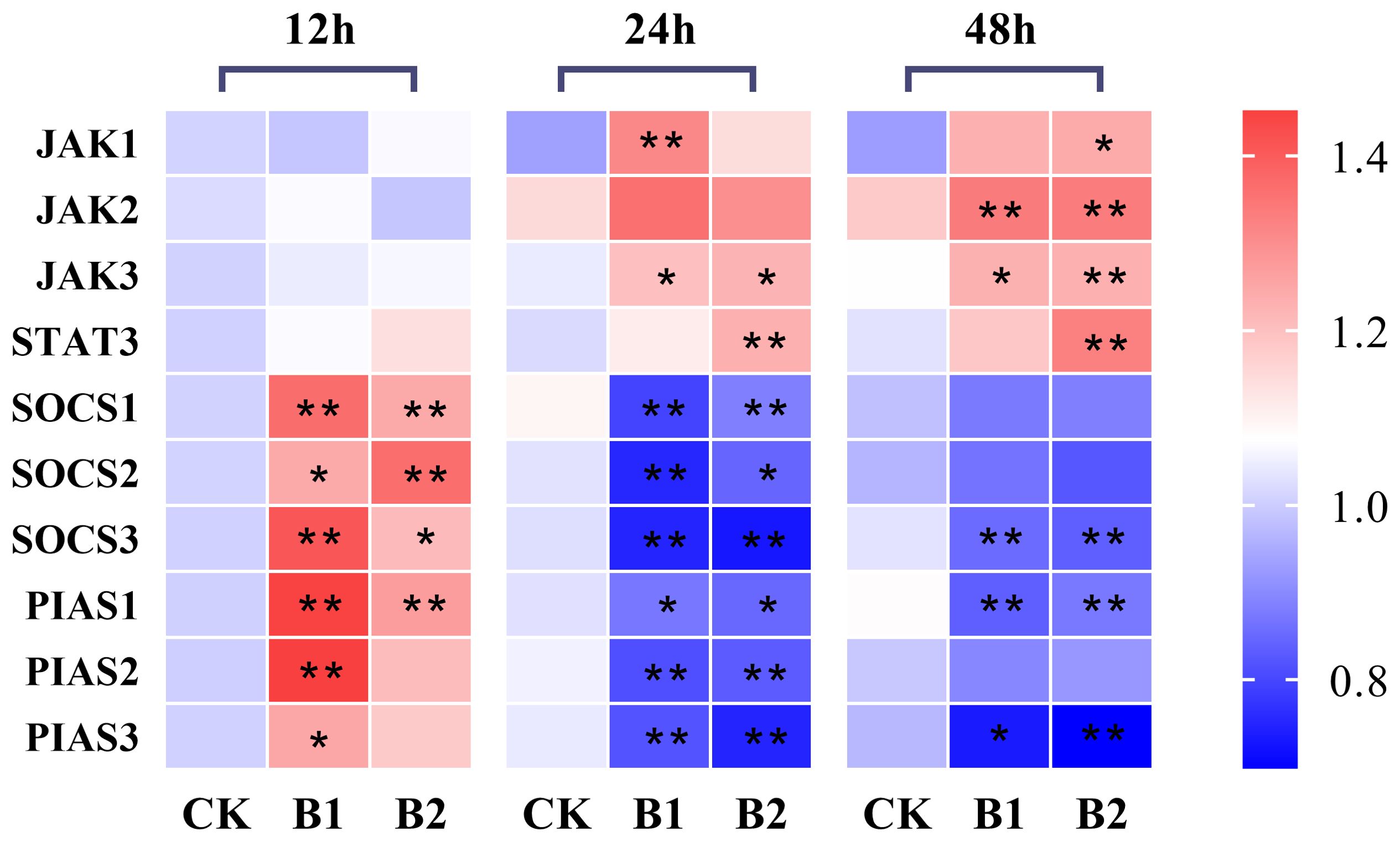
Figure 3. The heatmap of the mRNA expression of JAK-STAT pathway genes under BuP stress at different sampling times in the liver of P. megacephalus tadpoles. The symbol of * means significant difference when p < 0.05, ** highly significant difference when p < 0.01.
3.5 Effect of BuP on cell apoptosis in the liver
The expression levels of the anti-apoptotic gene Bcl-2 showed a substantial drop after 24 and 48 h of exposure in both the B1 and B2 treatment groups. The B2 group exhibited the lowest expression level (0.72-fold of the control group) after 48 h of stress, as seen in Figure 4A. The levels of expression for pro-apoptotic genes Bax and Caspase3 showed a substantial drop after 12 h of stress in the B1 and B2 treatment groups. Conversely, there was a rise in expression levels after 48 h of stress, as seen in Figures 4B, C.
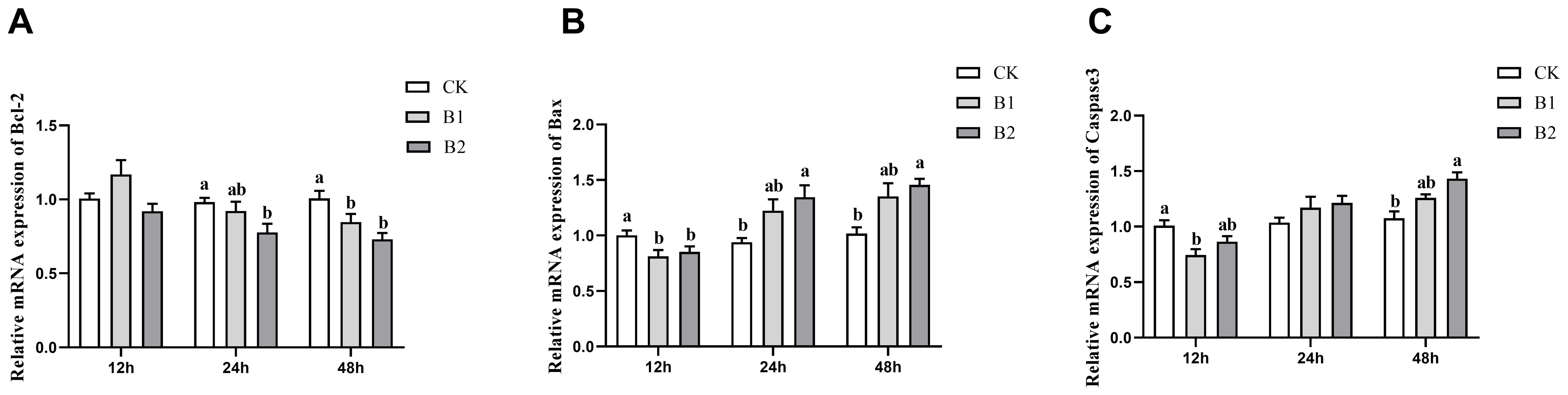
Figure 4. Effect of BuP on the mRNA expression of apoptosis genes in the liver of P. megacephalus tadpoles. (A) relative mRNA expression of Bcl-2; (B) relative mRNA expression of Bax; (C) relative mRNA expression of Caspase3. Different lowercase letters represent significant difference between groups at the same sampling time (p < 0.05).
The TUNEL assay indicated a low presence of apoptotic cells in the liver of the control group. Following 24 and 48 h exposure to BuP, there was an observed rise in the number of positive cells. However, it was noted that the number of apoptotic cells in the B2 group at 24 h was somewhat lower compared to the B1 group at 24 h during the BuP exposure period. The B2 group at 48 h has the highest number of positive cells, as seen in Figure 5.
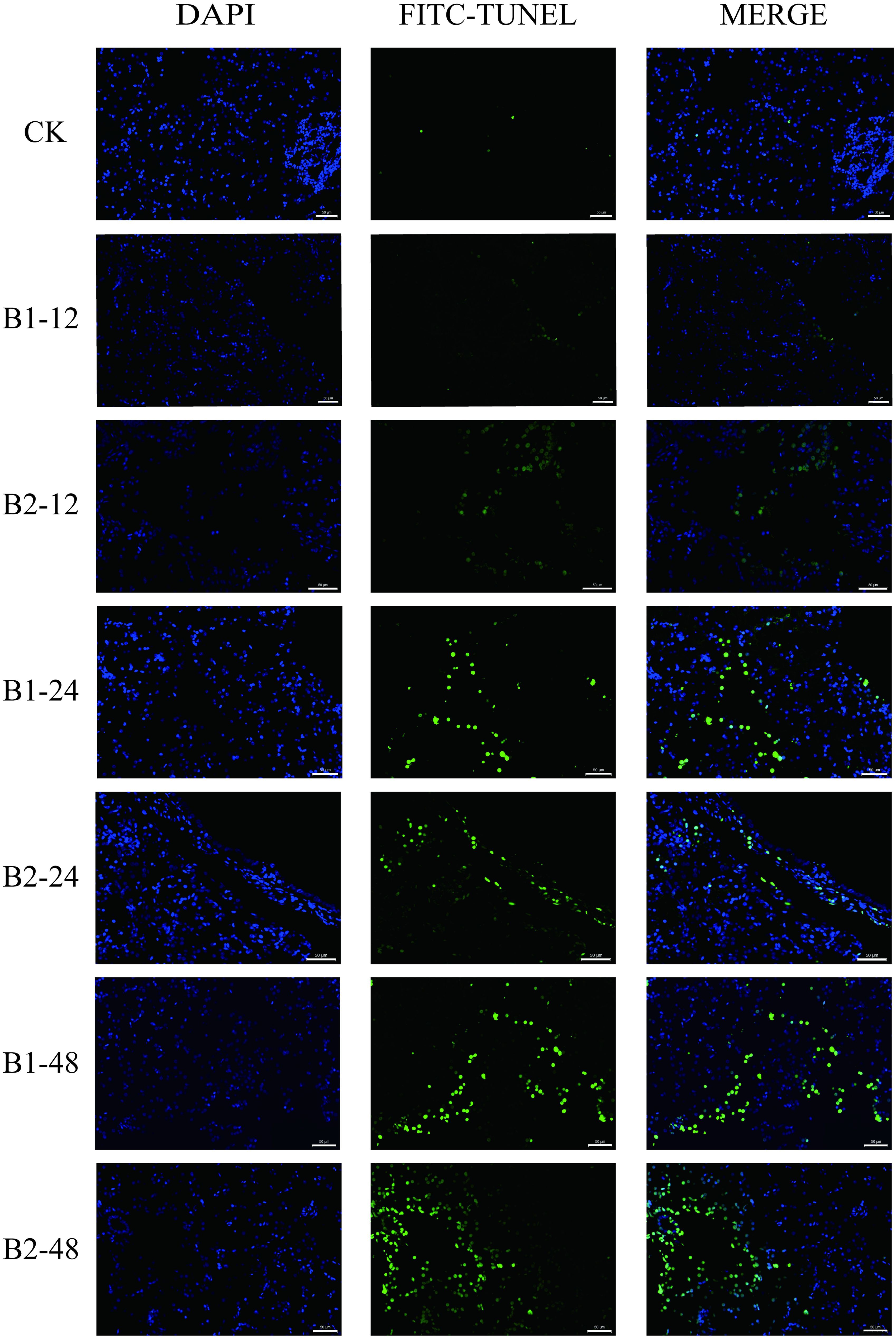
Figure 5. Apoptosis of liver cells in P. megacephalus tadpoles. CK, control group; B1-12, B1-24 and B1-48: B1 group sampling at 12, 24 and 48h; B2-12, B2-24 and B2-48: B2 group sampling at 12, 24 and 48h. Note: The first column showed the nucleus (blue); the second column showed the positive cell of apoptosis (green); the third column showed the merge; bar = 50 μm.
4 Discussion
Some studies on the toxicity of BuP using fish as animal models indicated that the 96 h-LC50 BuP was 2.9 mg/L in the early life stages of medaka (Oryzias latipes), 4.2 mg/L in fathead minnow (Pimephales promelas), 1.359 mg/L and 2.34 mg/L in embryos and early-life stages of zebrafish (Danio rerio), respectively (Yamamoto et al., 2007; Dobbins et al., 2009; Merola et al., 2020; Li et al., 2023a). The present investigation showed that 96 h-LC50 BuP in P. megacephalus tadpoles was 3.5 mg/L, which is lower than those reported in fathead minnow (Pimephales promelas), higher than that in the early life stages of medaka and zebrafish. Compared with other parabens, MeP was most toxic while BuP was least toxic to Dugesia jaonica, for example, 96 h-LC50 of MeP, EtP, PrP and BuP exposures were found to be 77, 31, 12.3, and 7.3 mg/L, respectively (Li, 2012). However, parabens concentrations in surface water ranged from 3.31 to 55.2 ng/L in the Yellow River (China) and from 15.0 to 164 ng/L in the Huai River (China) (Feng et al., 2019), which was much lower than 96 h-LC50 BuP to the tadpoles. However, higher BuP concentrations can be detected in the tissues of animals or human beings, such as 70 ng/g in fish (Ramaswamy et al., 2011), and 137 µg/L in women’s urine (Smith et al., 2013), which indicated its bioaccumulation and biomagnifications. As endocrine-disrupting chemicals, parabens would exert severe effects on the body even at sublethal exposure. The study on adult zebrafish (Danio rerio) showed the hepatotoxicity of MeP pollutants even at environmentally realistic concentrations (Hu et al., 2022). Exposure to 1 mg/L of BuP had a detrimental impact on the growth of fathead minnow (Pimephales promelas) (Dobbins et al., 2009). So, it is necessary to discuss the effect of higher BuP exposure on aquatic animals although the ambient concentration of BuP is not so high.
Numerous studies have shown that the body’s antioxidant enzyme system can adapt to environmental stress by altering the activity of antioxidant enzymes during oxidative stress when aquatic animals are stressed by pollution (Silva et al., 2018; Yang et al., 2018; Schreiber et al., 2019). The promotion of antioxidant systems that consist of enzymatic antioxidants such as superoxide dismutase (SOD), catalase (CAT), and glutathione peroxidases (GSH-PXs) as well as the non-enzymatic antioxidants which collectively reduce oxidative state. Primarily, SOD functions as a catalyst for the dismutation of superoxide anions (O2-) into O2 and H2O2, acting as the main protective mechanism against free radicals. CAT and GSH-PX play complementary roles in the removal of H2O2, and GSH acts as a primary electron donor in the reduction of H2O2 and organic peroxides, thereby scavenging O2- and other reactive oxygen species and safeguarding the organisms from oxidative stress (Hermes-Lima, 2004; Pacoń and Pacoń, 2021). BuP has potential cytotoxic and genotoxic impacts in a clinical application and it enhances the production of mitochondrial ROS and triggers oxidative DNA damage. The concentration of BuP in our study was positively correlated with the activities of SOD and GSH-PX, indicating activation of the antioxidant defense mechanism. The activities of SOD exhibited a statistically significant increase compared to the control group after 12, 24 and 48 h exposure to BuP in both the B1 and B2 groups (p < 0.05). The activities of GSH-PX were significantly increased in the B2 group than the control group after 48 h of stress (p < 0.05). This increased activity of antioxidant enzymes in tadpoles exposed to BuP demonstrated that there was an increase in ROS in the liver, and the tadpoles showed signs of lipid peroxidation in the liver, indicating that antioxidant defenses and neutralized ROS were weakened in prolonged and highly concentrated environments. We infer that antioxidant defenses are upregulated in the initial phases of exposure as a result of oxidative stress, including superoxide dismutase (SOD) and glutathione peroxidase (GSH-PX). As a result of the BuP exposure, cells attempt to neutralize reactive oxygen species (ROS). Nevertheless, prolonged exposure can overwhelm these antioxidant systems. Due to persistent ROS generation, key antioxidants like GSH-PX may be depleted and antioxidant enzymes may become less active, which leads to a weakening of defenses. A compromised antioxidant defense system can result in oxidative damage over time. A similar phenomenon was observed in Nile tilapia (Oreochromis niloticus), with liver SOD and GSH-PX activities increased after 6 d and 12 d BuP exposure respectively. Furthermore, having strong antioxidant defenses at all times might quickly prevent, or prior research has shown that when ROS are not promptly removed (Pacoń et al., 2021), they may induce lipid peroxidation and generate a substantial quantity of peroxides, such as MDA. In our study, the MDA contents were significantly increased in the B2 group compared to the control group.
The Keap1-Nrf2 system plays a vital role in the transcriptional activation of an array of antioxidant and detoxification genes (Wang et al., 2018). In response to oxidative stress, Keap1 is a sensor for the stress signals that lose their activity to interact with transcription factor Nrf2. Subsequently, Nrf2 translocates into the nucleus, which initiates the activation of genes encoding antioxidant enzymes (Taguchi et al., 2011). The mRNA levels of Nrf2 in the large yellow croaker (Pseudosciaena crocea) were increased sharply when exposed to 8 mg/L Zn and 32, 64 μg/L Hg (Zheng et al., 2016). The activity of Nrf2 is primarily controlled by Keap1 (Itoh et al., 2010). In this study, the relative expression of Keap1 was inversely linked with the concentration of BuP in all groups, but the mRNA expression level of Nrf2 rose and peaked in the B2 group. The mRNA expression level of Nrf2 was significantly increased in the three different concentrations of BuP groups after 12 h of exposure, while only the B1 group was significantly increased than the control group after 24 h of exposure (p < 0.05). The relative expression of Keap1 in the B1 and B2 groups exhibited a significant decrease compared to the control group after 12, 24 and 48 h of exposure (p < 0.05). Therefore, we speculated that increasing Nrf2 mRNA expression related to a decrease of Keap1 could elevate the antioxidant ability to BuP stress. On the other hand, by activating S6K1 and suppressing 4E-BP1, the TOR signaling pathway may promote the production of anti-inflammatory cytokines (Weichhart et al., 2011). In a sensitive manner, cytokines, growth factors, amino acids, or insulin activate mTOR and dramatically increase the phosphorylation status of 4E-BP1 and S6K1 (Hay and Sonenberg, 2004). A loss of mTOR function causes a cell cycle arrest as well as a severe reduction in protein synthesis. Our findings demonstrated that exposure to BuP significantly increased TOR transcription in the liver of P. megacephalus tadpoles. After exposure for 12 h, the transcriptional levels of 4E-BP1 dramatically dropped in the B2 group, and after 48 h, it significantly increased in both the B1 and B2 groups. A substantial increase in S6K1 transcriptional levels was seen in the B1 group, whereas a reduction in activity was observed in the B2 group. Gonzalez-Rodriguez et al. examined the impact of S6K1 on the vulnerability of hepatocytes to apoptosis triggered by death receptor activation within a hepatic cellular model (González-Rodriguez et al., 2009). The results of our study are consistent with the findings of Pardo et al. which indicate that the expression of S6K1 in hepatocytes altered following 16 h treatment with palmitic acid, as compared to the control group (Pardo et al., 2015).
The present investigation revealed that exposure to BuP produced oxidative stress resulting in an up-regulation of JAK1, JAK2, JAK3, and STAT3, hence initiating the JAK-STAT3 signaling pathway. Our findings are consistent with Li et al. (2023b) findings that, compared to the controls, grass carp liver cells subjected to TBBA and Pb showed substantial oxidative stress as well as increased expression levels of the hepatic JAK2-STAT3 signaling pathways. On the other hand, preventing oxidative stress from activating the JAK-STAT3 signaling pathway may be a more efficient strategy to shield the body’s tissues and cells from harmful external stimuli. SOCS, PIAS, and protein tyrosine phosphatases (PTPs) are regulators that adversely regulate the JAK-STAT signaling pathway in addition to its constituent parts (Wormald and Hilton, 2004). The initial elevation and subsequent downregulation of the genes SOCS1, SOCS2, SOCS3, PIAS1, PIAS2, and PIAS3 were seen in this research after 24 h of exposure to BuP. It is suggested that these findings, namely the initially elevated SOCS genes and PIAS proteins, may represent a kind of adaptive cellular reaction to the JAK-STAT signaling system, which is necessary to control or modify the BuP-induced JAK-STAT pathway activation. For example, Adeyi et al. showed that rats exposed to 2-methoxyethanol first showed an elevation of SOCS genes and PIAS proteins, and then later on, a downregulation was seen (Adeyi et al., 2023).
Oxidative stress may trigger programmed cell death, such as apoptosis, a protective process that controls the number of cells in the body and eliminates undesirable and perhaps hazardous ones (Estaquier et al., 2012). Pro- and anti-apoptotic Bcl protein family members and Caspase inhibitor proteins regulate apoptosis (Logan and Storey, 2016). Bax is a pro-apoptotic protein that translocates from the cytoplasm to the mitochondria upon cellular injury or stress, promoting an increase in permeability of the outer mitochondrial membrane, leading to the release of cytochrome c, which in turn activates the downstream apoptotic cascade response. Like Bax, the B-cell lymphocytic leukemia proto-oncogene (Bcl-2) family is an anti-death protein that prevents cell apoptosis (Yin et al., 2023). Bcl-2 family proteins move from the cytoplasm to the mitochondrial membrane when the cells receive an apoptotic signal. Caspases may readily identify stress-induced apoptosis. The intrinsic pathway is initiated by the release of cytochrome c from the mitochondria, which then activates Caspase3 and Caspase9. Numerous proteins, such as fodrin and nuclear laminae, are cleaved by Caspase3 and Caspase9, which ultimately results in cell death (Liang et al., 2016). The results of this experiment show that increasing the concentration of BuP can cause a gradual decrease in the mRNA expression level of Bcl-2 in the liver, while Bax and Caspase3 levels first decrease and then increase, suggesting that BuP can cause apoptosis in P. megacephalus tadpoles’ hepatocytes through the accumulation of toxicity over time. The mRNA expression level of Bcl-2 was significantly decreased in the B2 group after 24 and 48 h of exposure to BuP (p < 0.05). The Bax and Caspase3 levels were significantly decreased in the B1 group after 12 h of stress and then significant increased in the B2 group after 48 h of stress (p < 0.05). The TUNEL assay revealed a significant increase in the quantity of apoptotic cells inside the liver. The findings of this study suggest that exposure to BuP stress may potentially trigger apoptosis in P. megacephalus tadpoles.
5 Conclusions
Following exposure to BuP, the amount of MDA in the liver increased as exposure duration and concentration increased. The antioxidant defense response to BuP stress included the activation of the antioxidant enzymes (SOD and GSH-PX), and the Nrf2-Keap1 signaling pathway contributes to tadpoles’ antioxidant defense response. The JAK-STAT pathway inhibited toxic stress after 24 h, but with prolonged exposure, BuP stress induced apoptosis in tadpole hepatic cells.
Data availability statement
The original contributions presented in the study are included in the article/supplementary material. Further inquiries can be directed to the corresponding authors.
Ethics statement
All animal protocols described herein were performed according to the guiding principles of the Hainan Provincial Ecological Environment Education Center Experimental Animal Ethics Committee (No: HNECEE-2020-003).
Author contributions
XA: Data curation, Software, Writing – original draft, Writing – review & editing. MI: Formal analysis, Writing – review & editing. RL: Methodology, Writing – review & editing. XW: Methodology,Writing – review & editing. LW: Funding acquisition, Resources, Supervision, Visualization, Writing – review & editing. MH: Writing – review & editing, Funding acquisition, Resources.
Funding
The author(s) declare financial support was received for the research, authorship, and/or publication of this article. This work was supported by the Natural Science Foundation of Hainan Province (320MS039); The National Natural Science Foundation of China (40901261).
Conflict of interest
The authors declare that the research was conducted in the absence of any commercial or financial relationships that could be construed as a potential conflict of interest.
Publisher’s note
All claims expressed in this article are solely those of the authors and do not necessarily represent those of their affiliated organizations, or those of the publisher, the editors and the reviewers. Any product that may be evaluated in this article, or claim that may be made by its manufacturer, is not guaranteed or endorsed by the publisher.
References
Adeyi O. E., Somade O. T., James A. S., Adeyi A. O., Ogbonna-Eze S. N., Salako O. Q., et al. (2023). Ferulic acid mitigates 2-methoxyethanol-induced testicular oxidative stress via combined downregulation of foxo1, pten, and modulation of nrf2-hmox1-nqo1 signaling pathway in rats. Pharmacol. Res. - Modern Chin. Med. 7, 100257. doi: 10.1016/j.prmcm.2023.100257
Aydemir D., Öztaşcı B., Barlas N., Ulusu N. N. (2019). Effects of butylparaben on antioxidant enzyme activities and histopathological changes in rat tissues. Arch. Ind. Hygiene Toxicol. 70, 315–324. doi: 10.2478/aiht-2019-70-3342
Belenguer V., Martinez-Capel F., Masiá A., Picó Y. (2014). Patterns of presence and concentration of pesticides in fish and waters of the júcar river (eastern Spain). J. Hazard. Mater. 265, 271–279. doi: 10.1016/j.jhazmat.2013.11.016
Brühl C. A., Pieper S., Weber B. (2011). Amphibians at risk? Susceptibility of terrestrial amphibian life stages to pesticides. Environ. Toxicol. Chem. 30, 2465–2472. doi: 10.1002/etc.650
Burgos-Aceves M. A., Faggio C., Betancourt-Lozano M., González-Mille D. J., Ilizaliturri-Hernández C. A. (2022). Ecotoxicological perspectives of microplastic pollution in amphibians. J. Toxicol. Environ. Health Part B. 25, 405–421. doi: 10.1080/10937404.2022.2140372
Castro-Castellon A. T., Horton A. A., Hughes J. M. R., Rampley C., Jeffers E. S., Bussi G., et al. (2022). Ecotoxicity of microplastics to freshwater biota: Considering exposure and hazard across trophic levels. Sci. Total Environ. 816, 151638. doi: 10.1016/j.scitotenv.2021.151638
Darbre P. D., Aljarrah A., Miller W. R., Coldham N. G., Sauer M. J., Pope G. S. (2004). Concentrations of parabens in human breast tumours. J. Appl. Toxicol. 24, 5–13. doi: 10.1002/jat.958
Dobbins L. L., Usenko S., Brain R. A., Brooks B. W. (2009). Probabilistic ecological hazard assessment of parabens using Daphnia magna and Pimephales promelas. Environ. Toxicol. Chem. 28, 2744–2753. doi: 10.1897/08-523.1
Dong L., Zhang L., Peng Z., Guo J., Zhang X., Zhou L., et al. (2022). Monitoring and ecological risk assessment of contaminants in freshwater bodies by bioindicators in China: A proposed framework. Environ. Sci. pollut. Res. 29, 82098–82109. doi: 10.1007/s11356-022-21223-9
Estaquier J., Vallette F., Vayssiere J. L., Mignotte B. (2012). The mitochondrial pathways of apoptosis. Adv. Exp. Med. Biol. 942, 157–183. doi: 10.1007/978-94-007-2869-1_7
Feng J., Zhao J., Xi N., Guo W., Sun J. (2019). Parabens and their metabolite in surface water and sediment from the yellow river and the Huai river in Henan province: Spatial distribution, seasonal variation and risk assessment. Ecotoxicol. Environ. Safe. 172, 480–487. doi: 10.1016/j.ecoenv.2019.01.102
González-Rodriguez Á., Alba J., Zimmerman V., Kozma S. C., Valverde Á. M. (2009). S6K1 deficiency protects against apoptosis in hepatocytes. Hepatology 50, 216–229. doi: 10.1002/hep.22915
Gosner K. L. (1960). A simplified table for staging anuran embryos and larvae with notes on identification. Herpetologica 16, 183–190.
Hay N., Sonenberg N. (2004). Upstream and downstream of mTOR. Genes Dev. 18, 1926–1945. doi: 10.1101/gad.1212704
Hermes-Lima M. (2004). Oxygen in biology and biochemistry: Role of free radicals. Funct. metabol.: Regul. adapt. 1, 319–366. doi: 10.1002/047167558X.ch12
Hu C., Sun B., Tang L., Liu M., Huang Z., Zhou X., et al. (2022). Hepatotoxicity caused by methylparaben in adult zebrafish. Aquat. Toxicol. 250, 106255. doi: 10.1016/j.aquatox.2022.106255
Itoh K., Mimura J., Yamamoto M. (2010). Discovery of the negative regulator of Nrf2, Keap1: A historical overview. Antioxid. Redox Signal. 13, 1665–1678. doi: 10.1089/ars.2010.3222
Li M. H. (2012). Acute toxicity of benzophenone-type UV filters and paraben preservatives to freshwater planarian, Dugesia japonica. Toxicol. Environ. Chem. 94, 566–573. doi: 10.1080/02772248.2012.655695
Li Z., Jia K., Chen X., Guo J., Zheng Z., Chen W., et al. (2023a). Exposure to butylparaben induces craniofacial bone developmental toxicity in zebrafish (Danio rerio) embryos. Ecotoxicol. Environ. Saf. 265, 115523. doi: 10.1016/j.ecoenv.2023.115523
Li L., Li W., Liu Y., Jin X., Yu Y., Lin H. (2023b). Tbbpa and lead co-exposure induces grass carp liver cells apoptosis via ROS/JAK2/STAT3 signaling axis. Fish Shellf. Immunol. 142, 109100. doi: 10.1016/j.fsi.2023.109100
Liang L., Huang Z., Li N., Wang D., Ding L., Shi H., et al. (2020). Effects of ammonia exposure on antioxidant function, immune response and NF-κB pathway in Chinese Strip-necked Turtle (Mauremys sinensis). Aquat Toxicol. 229, 105621. doi: 10.1016/j.aquatox.2020.105621
Liang S., Sun K., Wang Y., Dong S., Wang C., Liu L., et al. (2016). Role of cyt-c/caspases-9,3, bax/bcl-2 and the fas death receptor pathway in apoptosis induced by zinc oxide nanoparticles in human aortic endothelial cells and the protective effect by alpha-lipoic acid. Chemico-Biol. Interact. 258, 40–51. doi: 10.1016/j.cbi.2016.08.013
Linder G., Palmer B. D., Little E. E., Rowe C. L., Henry P. (2010). Physiological ecology of amphibians and reptiles. Pensacola: CRC Press 105–166.
Logan S. M., Storey K. B. (2016). Avoiding apoptosis during mammalian hibernation. Temperature 4, 15–17. doi: 10.1080/23328940.2016.1211071
Luedtke J. A., Chanson J., Neam K., Hobin L., Maciel A. O., Catenazzi A., et al. (2023). Ongoing declines for the world’s amphibians in the face of emerging threats. Nature 622, 308–314. doi: 10.1038/s41586-023-06578-4
Merola C., Lai O., Conte A., Crescenzo G., Torelli T., Alloro M., et al. (2020). Toxicological assessment and developmental abnormalities induced by butylparaben and ethylparaben exposure in zebrafish early-life stages. Environ. Toxicol. Pharmacol. 80, 103504. doi: 10.1016/j.etap.2020.103504
Moreno-Marenco A. R., Giraldo L., Moreno-Piraján J. C. (2020). Adsorption of n-butylparaben from aqueous solution on surface of modified granular activated carbons prepared from african palm shell. Thermodynamic study of interactions. J. Environ. Chem. Eng. 8, 103969. doi: 10.1016/j.jece.2020.103969
Pacoń J., Pacoń J. (2021). The influence of environmental factors on the behavior and mortality risk of polypedates megacephalus tadpoles. Asian Herpetological Research 12, 100-109. doi: 10.16373/j.cnki.ahr.200015
Pardo V., González-Rodríguez Á., Muntané J., Kozma S. C., Valverde Á. M. (2015). Role of hepatocyte S6K1 in palmitic acid-induced endoplasmic reticulum stress, lipotoxicity, insulin resistance and in oleic acid-induced protection. Food Chem. Toxicol. 80, 298–309. doi: 10.1016/j.fct.2015.03.029
Park J. K., Do Y. (2023). Current state of conservation physiology for amphibians: Major research topics and physiological parameters. Animals 13, 3162. doi: 10.3390/ani13203162
Ramaswamy B. R., Kim J. W., Isobe T., Chang K. H., Amano A., Miller T. W., et al. (2011). Determination of preservative and antimicrobial compounds in fish from manila bay, Philippines using ultra high performance liquid chromatography tandem mass spectrometry, and assessment of human dietary exposure. J. Hazard. Mater. 192, 1739–1745. doi: 10.1016/j.jhazmat.2011.07.006
Samarasinghe S., Krishnan K., Naidu R., Megharaj M., Miller K., Fraser B., et al. (2018). Parabens generate reactive oxygen species in human spermatozoa. Andrology 6, 532–541. doi: 10.1111/andr.12499
Schreiber E., Garcia T., Sharma R. P., Torrente M., Domingo J. L., Gómez M. (2019). Oxidative stress in testes of rats exposed to n-butylparaben. Food Chem. Toxicol. 131, 110573. doi: 10.1016/j.fct.2019.110573
Silva D. C., Serrano L., Oliveira T. M. A., Mansano A. S., Almeida E. A., Vieira E. M. (2018). Effects of parabens on antioxidant system and oxidative damages in nile tilapia (Oreochromis niloticus). Ecotoxicol. Environ. Safe. 162, 85–91. doi: 10.1016/j.ecoenv.2018.06.076
Slaby S., Marin M., Marchand G., Lemiere S. (2019). Exposures to chemical contaminants:What can we learn from reproduction and development endpoints in the amphibian toxicology literature? Environ. Pollut. 248, 478–495. doi: 10.1016/j.envpol.2019.02.014
Smith K. W., Souter I., Dimitriadis I., Ehrlich S., Williams P. L., Calafat A. M., et al. (2013). Urinary paraben concentrations and ovarian aging among women from a fertility center. Environ. Health Perspect. 121, 1299–1305. doi: 10.1289/ehp.1205350
Sparling D. W., Linder G., Bishop C. A., Krest S. K. (2010). Ecotoxicology of amphibians and reptiles (Boca Raton, FL: CRC Press), 261–288.
Taguchi K., Motohashi H., Yamamoto M. (2011). Molecular mechanisms of the keap1-nrf2 pathway in stress response and cancer evolution. Genes to Cells 16, 123–140. doi: 10.1111/j.1365-2443.2010.01473.x
Wang H., Pan L., Si L., Miao J. (2018). The role of nrf2-keap1 signaling pathway in the antioxidant defense response induced by pahs in the calm ruditapes philippinarum. Fish Shellf. Immunol. 80, 325–334. doi: 10.1016/j.fsi.2018.06.030
Weichhart T., Haidinger M., Katholnig K., Kopecky C., Poglitsch M., Lassnig C., et al. (2011). Inhibition of mtor blocks the anti-inflammatory effects of glucocorticoids in myeloid immune cells. Blood 117, 4273–4283. doi: 10.1182/blood-2010-09-310888
Whitfield S. M., Bell K. E., Philippi T., Sasa M., Bolaños F., Chaves G., et al. (2007). Amphibian and reptile declines over 35 years at la selva, Costa Rica. Proc. Natl. Acad. Sci. United States America, 104, 8352–8356. doi: 10.1073/pnas.0611256104
Wormald S., Hilton D. J. (2004). Inhibitors of cytokine signal transduction. J. Biol. Chem. 279, 821–824. doi: 10.1074/jbc.R300030200
Yamamoto H., Watanabe M., Hirata Y., Nakamura Y., Nakamura Y., Kitani C., et al. (2007). Preliminary ecological risk assessment of butylparaben and benzylparaben -1. Removal efficiency in wastewater treatment, acutechronic toxicity for aquatic organisms, and effects on medaka gene expression. Environ. sci.: an Int. J. Environ. Physiol. Toxicol. 14, 73–87.
Yang C., Lim W., Bazer F. W., Song G. (2018). Butyl paraben promotes apoptosis in human trophoblast cells through increased oxidative stress-induced endoplasmic reticulum stress. Environ. Toxicol. 33, 436–445. doi: 10.1002/tox.22529
Yin Y., Xie Z., Sun X., Wu X., Zhang J., Shi H., et al. (2023). Effect of butyl paraben on oxidative stress in the liver of mauremys sinensis. Toxics 11, 915. doi: 10.3390/toxics11110915
Zang W. L., Xu X. C., Dai X. L., Zhu Z. G. (1993). Toxic effects of Zn2+, Cu2+, Cd2+ and NH3 on Chinese prawn. Chin. J. Oceanol. Limnol. 11, 254–259. doi: 10.1007/BF02850858
Zheng J. L., Zeng L., Shen B., Xu M. Y., Zhu A. Y., Wu C. W. (2016). Antioxidant defenses at transcriptional and enzymatic levels and gene expression of nrf2-keap1 signaling molecules in response to acute zinc exposure in the spleen of the large yellow croaker pseudosciaena crocea. Fish Shellf. Immunol. 52, 1–8. doi: 10.1016/j.fsi.2016.02.031
Keywords: butylparaben, Polypedates megacephalus tadpoles, antioxidant enzymes, apoptosis, JAK-STAT signal pathway
Citation: Ai X, Iqbal MS, Lin R, Wu X, Wang L and Hong M (2024) Exposure to butylparaben induces oxidative stress and apoptosis in the liver of Polypedates megacephalus tadpole. Front. Mar. Sci. 11:1480097. doi: 10.3389/fmars.2024.1480097
Received: 13 August 2024; Accepted: 23 September 2024;
Published: 17 October 2024.
Edited by:
Yafei Duan, South China Sea Fisheries Research Institute, ChinaReviewed by:
Basanta Kumar Das, Central Inland Fisheries Research Institute (ICAR), IndiaMohamed Hamed, Louisiana State University, United States
Copyright © 2024 Ai, Iqbal, Lin, Wu, Wang and Hong. This is an open-access article distributed under the terms of the Creative Commons Attribution License (CC BY). The use, distribution or reproduction in other forums is permitted, provided the original author(s) and the copyright owner(s) are credited and that the original publication in this journal is cited, in accordance with accepted academic practice. No use, distribution or reproduction is permitted which does not comply with these terms.
*Correspondence: Lijun Wang, wanglijun_haikou@hainnu.edu.cn; Meiling Hong, mlhong@hainnu.edu.cn
†These authors have contributed equally to this work