- 1Hawaiʻi Institute of Marine Biology, University of Hawaiʻi at Mānoa, Kāneʻohe, HI, United States
- 2Department of Marine Biology, University of Hawaiʻi at Mānoa, Honolulu, HI, United States
- 3Department of Biological Science, California State University Fullerton, Fullerton, CA, United States
- 4Directorate for Biological Sciences, National Science Foundation, Alexandria, VA, United States
- 5Department of Oceanography, University of Hawaiʻi at Mānoa, Honolulu, HI, United States
- 6Department of Fisheries Science, Virginia Institute of Marine Science, College of William and Mary, Gloucester Point, VA, United States
Scalloped hammerhead sharks (Sphyrna lewini) routinely perform rapid dives to forage on mesopelagic prey. These deep dives consist of intensive swimming followed by recovery periods in the surface mixed layer. Swimming muscle temperature profiles suggest that S. lewini suppresses gill function as a means to reduce convective heat loss during dives into cool water. Such intensive swimming behavior coupled with reduced respiration prompted us to test whether the aerobic and anaerobic metabolic capacities of the white swimming muscle tissue of this species are greater than those of other shark species from the same region. The activities of key enzymes used in aerobic and anaerobic metabolism provide an indirect indicator of the metabolic potential (“poise”) of a tissue. Here we measured the maximal activities [international units (µmol substrate converted to product per min, U) per gram of wet tissue mass at 10°C] of the citric acid cycle enzymes citrate synthase (CS) and malate dehydrogenase (MDH) and glycolytic enzymes pyruvate kinase (PK) and lactate dehydrogenase (LDH) from white swimming muscle of S. lewini. Enzyme activities, and ratios of these enzyme activities that indicate relative indexes of aerobic to anaerobic capacity, were compared to those measured in three sympatric coastal carcharhinid sharks and two deep-dwelling species, Echinorhinus cookei and Hexanchus griseus. This is the first report of swimming-muscle enzyme activity for these deep-dwelling species. In comparison to the other species, S. lewini had significantly higher activities of both LDH and MDH in the white muscle, and a higher MDH/CS ratio. The high LDH activities suggest that the white muscle of S. lewini relies on relatively high rates of anaerobic ATP production, with would result in build up of high lactate levels, during deep foraging dives. High MDH activity in S. lewini white muscle suggests the potential for lactate levels to be rapidly reduced when aerobic conditions are restored while in the surface mixed layer between dives. These biochemical characteristics may enable S. lewini to swim rapidly while suppressing gill function during deep dives and thereby exploit a very different ecological niche from sympatric shark species (e.g., coastal carcharhinids) and hunt more rapidly via faster swimming for deep-water prey compared to species that permanently inhabit deep depths.
Introduction
The scalloped hammerhead shark, Sphyrna lewini, shares overlapping daytime distributions in tropical and warm temperate coastal environments with other sympatric species (coastal carcharhinids), but exploits a very different ecological niche by foraging in deep, cold habitats. Scalloped hammerhead sharks conduct repetitive nocturnal deep dives (over 600m, sometimes exceeding 1000m) into cold water (as low as 5°C), presumably to forage on mesopelagic prey in the oxygen minimum zone (Jorgensen et al., 2009; Bessudo et al., 2011; Hoffmayer et al., 2013; Spaet et al., 2017; Anderson et al., 2022; Hutchinson et al., 2023; Royer et al., 2023). Royer et al. (2023) reported that S. lewini reduces convective heat loss at the gills during these deep dives, and suggested that this strategy of suppressing gill function is broadly similar to a “breath hold” dive. Biologger data of Royer et al. (2023) revealed that these deep dives are characterized by steep descents with swimming bursts when approaching maximum depth, intense activity throughout the deepest phase, with bottom times lasting an average of approximately 4 minutes, and a consistent high-frequency, high-amplitude tailbeat during ascent (Figure 1). During the ascent, at a depth of ~250m, swimming intensity decreases abruptly, sharks level out and ascend more slowly. This part of the ascent is characterized as the “inflection point.” On reaching the surface mixed layer, sharks swim using a lower tailbeat frequency and amplitude until starting the next deep dive. Overall, these dives last an average of 56 minutes (from the start of the slow descent to the end of the slow ascent), with the high-activity phase of the dives (from the start of the fast descent to the end of the fast ascent at the inflection point) lasting an average of 17 minutes. The sharks stayed within the top 50 meters of the water column during their interdive intervals which lasted an average of 43 minutes and ranged from 18 minutes to >3 hours. Temperature measurements of the white swimming muscle show that S. lewini stays warm throughout the deepest portion of the dives and substantive cooling only occurs during the latter stages of the ascent phase and, once initiated, is rapid. These rapid changes in body temperature at different points of the dive cannot be explained by simple thermal inertia. Modeling of heat transfer coefficients (Royer et al., 2023) indicates that convective heat transfer at the gills is suppressed during the high-activity phase of the dives. Although the active mechanism for reducing heat loss at the gills is unknown (e.g., shunting blood away from the gills, reducing blood flow to the gills, reducing ram ventilation by closing the mouth, gill slits or both), any of these will inhibit the shark’s ability to absorb oxygen from the environment. Video of a scalloped hammerhead shark swimming along the seabed at a depth of 1,044m showed its gill slits tightly closed, whereas similar images from surface waters show these sharks swimming with their gill slits wide open, supporting the gill-slit-closing hypothesis (Moore and Gates, 2015; Royer et al., 2023). A sudden cooling in muscle temperature as scalloped hammerhead sharks approach the surface at the end of each dive suggests that they have opened their gill slits to resume gill ventilation while still in relatively cool water.
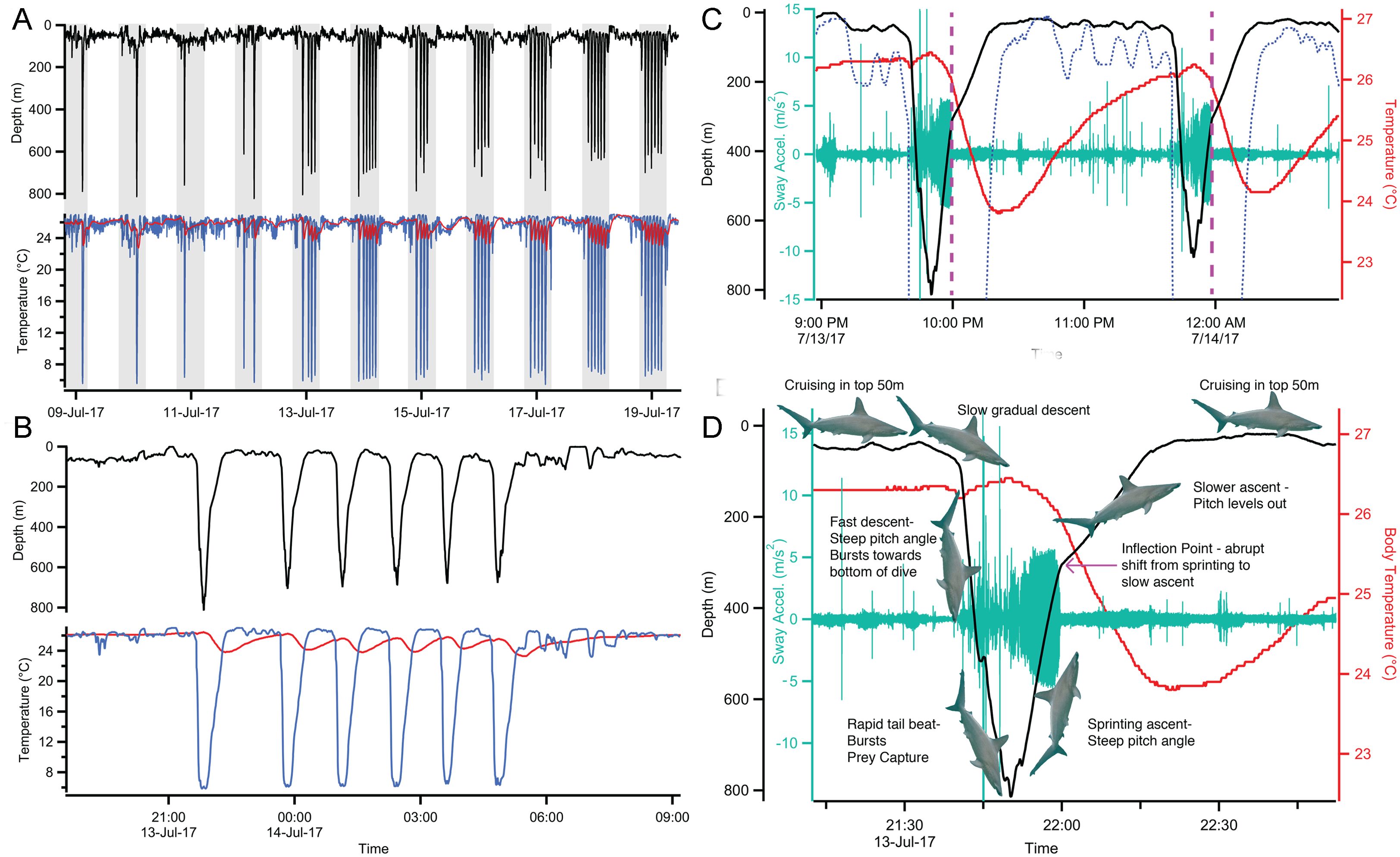
Figure 1. Deep-diving behavior and body temperature of S. lewini, reproduced from Royer et al. (2023). Ambient (blue), and intramuscular (red) temperature profiles from a scalloped hammerhead shark deep-diving during nighttime (A), with repetitive deep-dives shown during a single evening (B). (C) Depth profiles (black), body temperature (red), ambient water temperature (blue dashed), and swimming activity [tailbeat sway acceleration (teal)] during two successive deep dives. Dotted lines indicate the inflection point of the ascents when the swimming activity and pitch angle abruptly decrease. (D) Distinct phases of a deep dive.
Powering swimming during these repetitive, intense, deep dives necessitates a high energetic output by the locomotor muscles. It is possible that S. lewini relies on anaerobic energy production during these deep dives due to the combination of intense swimming activity and suppression of normal respiration. This highly active swimming combined with apparent “breath holding” should be reflected in the activities of key muscle enzymes involved with locomotion and energy mobilization.
Anaerobic metabolism becomes an essential process when aerobic pathways of ATP production cannot sustain cellular energetic demands (Pörtner, 2002). Burst swimming typically requires anaerobic metabolism and a greater reliance on muscle energy stores (Williams et al., 1997). In fishes, fast-twitch glycolytic white (type II) muscle is the largest tissue mass and is specialized for anaerobic high-intensity swimming when power output is needed beyond what the slow-twitch red muscle (type I) is capable of producing (Bernal et al., 2003; Seamone and Syme, 2016). High-intensity swimming events, such as the chasing of prey, typically require anaerobic pathways independent of oxygen availability and deplete muscle creatine-phosphate and glycogen stores, leading to a concomitant buildup of lactate and H+ byproducts (Guppy and Hochachka, 1978; Bernal et al., 2003; Kane, 2014).
Generally, elasmobranchs found in warm shallow waters (above mesophotic depths) have higher metabolic rates and stronger burst-locomotor capabilities relative to deep-dwelling elasmobranchs (Treberg et al., 2003; Condon et al., 2012). This is supported by electronic tagging data, with slower vertical velocities, slower swimming speeds, and slower tail beats for H. griseus and E. cookei than for epipelagic shark species (Nakamura et al., 2011, 2015; Comfort and Weng, 2015; Andrzejaczek et al., 2018; Coffey et al., 2020; Royer et al., 2023). Capacity of tissues for aerobic and anaerobic metabolism can be estimated by measuring the maximal activity of key enzymes involved in the production of ATP. The activities of the enzymes citrate synthase (CS) and malate dehydrogenase (MDH) have been widely used as indicators of tissue aerobic capacity. CS is the first catalyst in the citric acid cycle (Childress and Somero, 1979) and its activity correlates with tissue mitochondrial density (Moyes et al., 1992; Dickson et al., 1993; Dickson, 1995; Duong et al., 2006) and whole animal metabolic rate (Torres and Somero, 1988; Hochachka and Somero, 2002). MDH is used in the citric acid cycle in the shuttling of reducing equivalents between the mitochondria and cytosol. MDH is also involved in gluconeogenesis, the synthesis of glucose from smaller molecules such as pyruvate formed by oxidation of lactate accumulated during anaerobic function (Kane, 2014; Rogatzki et al., 2015). Activities of the enzymes pyruvate kinase (PK) and lactate dehydrogenase (LDH) have been used as indicators of anaerobic capacity. PK is a good indicator of the capacity for glycolysis, as it catalyzes pyruvate formation during glycolysis (Somero and Childress, 1980). LDH catalyzes the reversible conversion of pyruvate and NADH to lactate and NAD+ to maintain redox balance, allowing anaerobic production of ATP to continue in the cytosol; therefore, LDH activity is considered a strong indicator of tissue anaerobic capacity (Hochachka et al., 1982). Measurements of the maximal activities of all four of these enzymes have been widely used in previous studies to assess the aerobic and anaerobic capacity of muscle in fishes (Childress and Somero, 1979; Sullivan and Somero, 1980; Somero, 1992; Dickson et al., 1993; Vetter and Lynn, 1997; Bernal et al., 2003; Treberg et al., 2003; Ombres et al., 2011; Condon et al., 2012; Drazen et al., 2015; Saavedra et al., 2016; Pinte et al., 2021).
The combination of intensive swimming and likely suppressed gill function suggests that S. lewini relies heavily on anaerobic metabolism during deep dives (Meekan and Gleiss, 2023). These characteristics may enable S. lewini to swim rapidly while suppressing gill function during dives and thereby exploit a very different ecological niche from sympatric shark species (e.g., coastal carcharhinids) and hunt more rapidly for deep-water prey compared to slow-moving shark species that permanently inhabit deep depths. Therefore, we predicted that the white muscle tissue of S. lewini possesses enzyme characteristics that facilitate anaerobic metabolism during deep dives, and the necessary aerobic metabolism to allow for rapid recovery (i.e., the breakdown of anaerobic end products) in well-oxygenated surface waters during intervals between dives. Furthermore, we predicted that the white muscle enzyme characteristics of S. lewini can be distinguished from those of both sympatric shallow-water shark species (e.g., coastal carcharhinids) that share similar daytime distributions and deep-water shark species, by having a greater capacity for both anaerobic and aerobic metabolism in the white swimming muscle. To test this hypothesis, the maximal activities of the enzymes CS, MDH, PK, and LDH were measured to assess the aerobic and anaerobic poise of the white muscle of adult S. lewini. Activities of these enzymes were compared to those measured in other coastal tropical/temperate shark species [sandbar shark (Carcharhinus plumbeus), blacktip shark (Carcharhinus limbatus), and tiger shark (Galeocerdo cuvier)] and in two deep-water sharks [bluntnose sixgill (Hexanchus griseus) and prickly shark (Echinorhinus cookei)].
Materials and methods
Tissue sampling
White muscle tissue samples were collected from sharks caught on demersal long-lines set in waters off the island of O’ahu (Hawai’i, USA). Shallow species (S. lewini, G. cuvier, C. plumbeus, C. limbatus) were caught at depths of 5-50 meters and deep species (H. griseus and E. cookei) were caught between depths of 250–300 meters. Muscle samples were collected from a 2-cm incision below the base of the first dorsal fin using a 5mm or 8mm biopsy punch. Samples were placed in a cryovial and immediately dropped into a Dewar flask containing liquid nitrogen. Samples were later transferred to and stored in a -80°C freezer for 3-24 months. This storage time falls within the allowable timeframe between tissue sampling and assay running without compromising (decreasing) tissue enzyme activity (Dickson et al., 1993; Condon et al., 2012).
Enzyme assays
The maximal activities of the enzymes CS, PK, LDH, and MDH in each white muscle tissue sample were measured using enzyme assay protocols based on those established by previous studies (Childress and Somero, 1979; Treberg et al., 2003; Condon et al., 2012; Friedman et al., 2012). Frozen muscle samples were weighed and homogenized in a Kontes Duall ground glass tissue grinder with ice-cold 10 mM Tris-HCL buffer (pH 7.55 at 10°C) at a ratio of 0.1 g of tissue to 1 ml of buffer. Duplicate homogenates were prepared for each sample unless the total sample was less than 0.05 g, in which case a single homogenate was prepared. CS assays were performed before centrifugation for the other enzyme assays. Homogenates were then centrifuged at 5000g for 5 minutes, and the supernatants were used in the assays.
All assays were run in a volume of 2 mL at 10°C in a Shimadzu UV 1601 spectrophotometer with a water-jacketed 12-cell cuvette holder attached to a water chiller (Condon et al., 2012). Activities were measured as change in absorbance over time and reported in international units (U; µmol substrate converted to product per min) per gram of wet tissue mass. PK, LDH, and MDH assays were run at 340 nm for 40 seconds and CS was run at 412 nm for 3 minutes. Enzyme assays were run under the following saturating substrate conditions: citrate synthase: 0.1 mM 5,5-dithiobis-nitrobenzoic acid (DTNB), 50 mM imidazole HCl (pH 8.0 at 10°C), 2 mM MgCl2, 0.1 mM acetyl-CoA, 0.5 mM oxaloacetate. After recording background change in absorbance over time with the tissue homogenate present, the CS reaction was initiated by adding oxaloacetate substrate. Pyruvate kinase: 80 mM Tris HCl (pH 7.8 at 10°C), 100 mM KCl, 10 mM MgSO4, 10 U/ml lactate dehydrogenase, 0.1 mM fructose 1-6 bisphosphate, 5.0 mM adenosine diphosphate, 150 µM NADH. The PK reaction was initiated by the addition of 1.0 mM phosphoenol pyruvate. Lactate dehydrogenase: 80 mM imidazole (pH 7.8 at 10°C), 100 mM KCl, 150 µM NADH, 2 mM sodium pyruvate. Malate dehydrogenase: 100 mM Tris HCl (pH 8.1 at 10°C), 20 mM MgCl2, 150 µM NADH, 0.5 mM oxaloacetic acid. LDH and MDH reactions were initiated with the addition of the supernatant.
Data analysis
The body mass for each shark was estimated from measured lengths using length-weight relationships from Kohler et al. (1996) for S. lewini, G. cuvier, C. plumbeus, Ebert (1986) for H. griseus, and Pollack et al. (2019) for C. limbatus. No length-weight relationships were available for E. cookei. Regression analysis was used to evaluate whether muscle enzyme activity scaled with shark body size for each species. Normality of enzyme activity data was assessed by examining distribution histograms for each species and enzyme, and using Levene’s test to assess homogeneity of variance. Due to the non-normal (based on histograms and NQQ plots) and heteroscedastic (Levene’s test, all P < 0.05) nature of the data and the unequal sample sizes among species, non-parametric Welch’s ANOVAs with post-hoc Games-Howell tests were used to assess interspecific differences in muscle enzyme activity. High activity of MDH observed in S. lewini prompted a post-hoc interspecific comparison of the ratio of the aerobic enzymes (MDH and CS). The ratio of MDH to CS activity was calculated for each individual shark, and then averages and standard deviations were calculated for each species. Based on similar data characteristics mentioned above, non-parametric Welch’s ANOVA with a post-hoc Games-Howell test was used to assess interspecific differences in the MDH: CS ratio between species.
Results
Body size scaling effects on enzyme activity
All S. lewini (n = 10) and E. cookei (n = 5) sampled were mature adult males. All G. cuvier (n = 22) were immature sub-adults or juveniles. One C. limbatus (n = 5) and 12 C. plumbeus (n = 24) were mature adults. Body length measurements were missing for 2 G. cuvier and 3 C. plumbeus. Two of the male H. griseus (n = 9) were mature adults, 2 of the other males were large sub-adults, and all 5 of the females were large sub-adults, based on clasper calcification (for males) and estimates of age at maturity from (Ebert, 2002). Regression analyses showed no significant scaling of enzyme activity with body size for the species studied (all P values > 0.05), with the exception of LDH and MDH for S. lewini (P = 0.02, P = 0.01) and H. griseus (P = 0.03, P = 0.02). The largest individual of both species had unusually low LDH and MDH values. When those two individuals were removed as outliers, no significant body size scaling was observed (all P values > 0.05). As a result, mean enzyme activities, unadjusted for body size but with the outlier data included, are reported in Table 1 and used for interspecific comparisons.
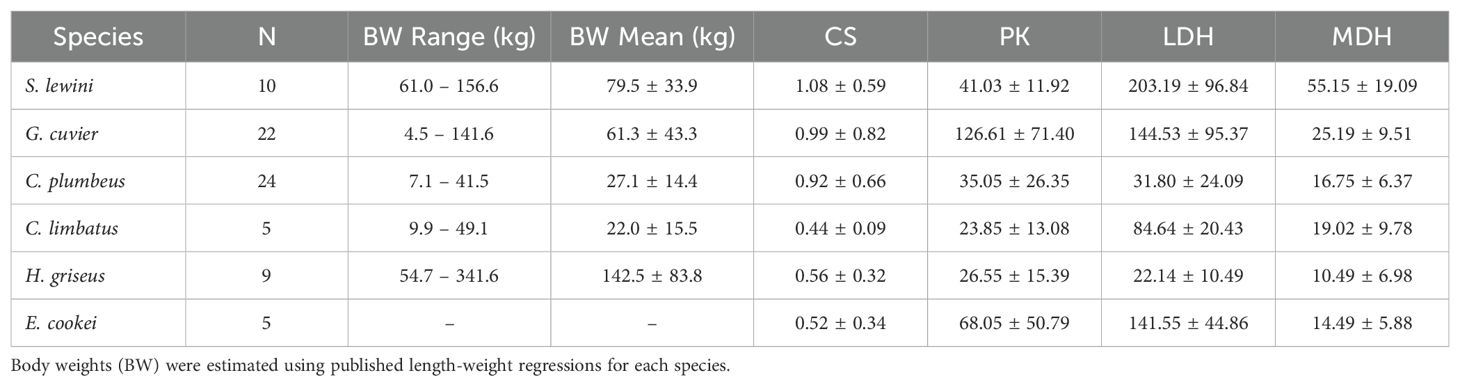
Table 1. White muscle enzyme activity (mean ± standard deviation) reported in U/g wet mass at 10°C for each species.
White muscle enzyme activities
Sphyrna lewini white muscle exhibited high activities of LDH, CS, and MDH (Table 1), with the activities of LDH and MDH of S. lewini standing out as much higher in comparison to the sympatric coastal (C. plumbeus, C. limbatus, G. cuvier) and deep-water (H. griseus, E. cookei) species (Figures 2, 3A). MDH activity in S. lewini was significantly greater than that in all of the coastal and deep-water species (all P ≤ 0.007, Games-Howell tests; Figure 1). LDH activity was significantly greater in S. lewini than in the coastal sharks C. limbatus (P = 0.02) and C. plumbeus (P = 0.003) and the deep-water shark H. griseus (P = 0.006). Average CS activity in S. lewini was the highest amongst the species studied, but not significantly so (all P > 0.05) (Figure 2). PK activity in S. lewini did not differ significantly from that measured in the coastal sharks C. limbatus and C. plumbeus and in both deep water sharks, but was significantly lower than that of G. cuvier (P < 0.001). MDH activity relative to CS activity was highest in S. lewini compared to the other coastal carcharhinids and bathyal shark species, followed by C. plumbeus and G. cuvier (Table 2, Figure 3B). Though the statistical comparisons of MDH: CS ratios between S. lewini and all other species except E. cookei were not significant (all P>0.05), it is noticeable in Figure 3B that G. cuvier and C. plumbeus also have high CS activity but not the correspondingly higher MDH activity observed in S. lewini.
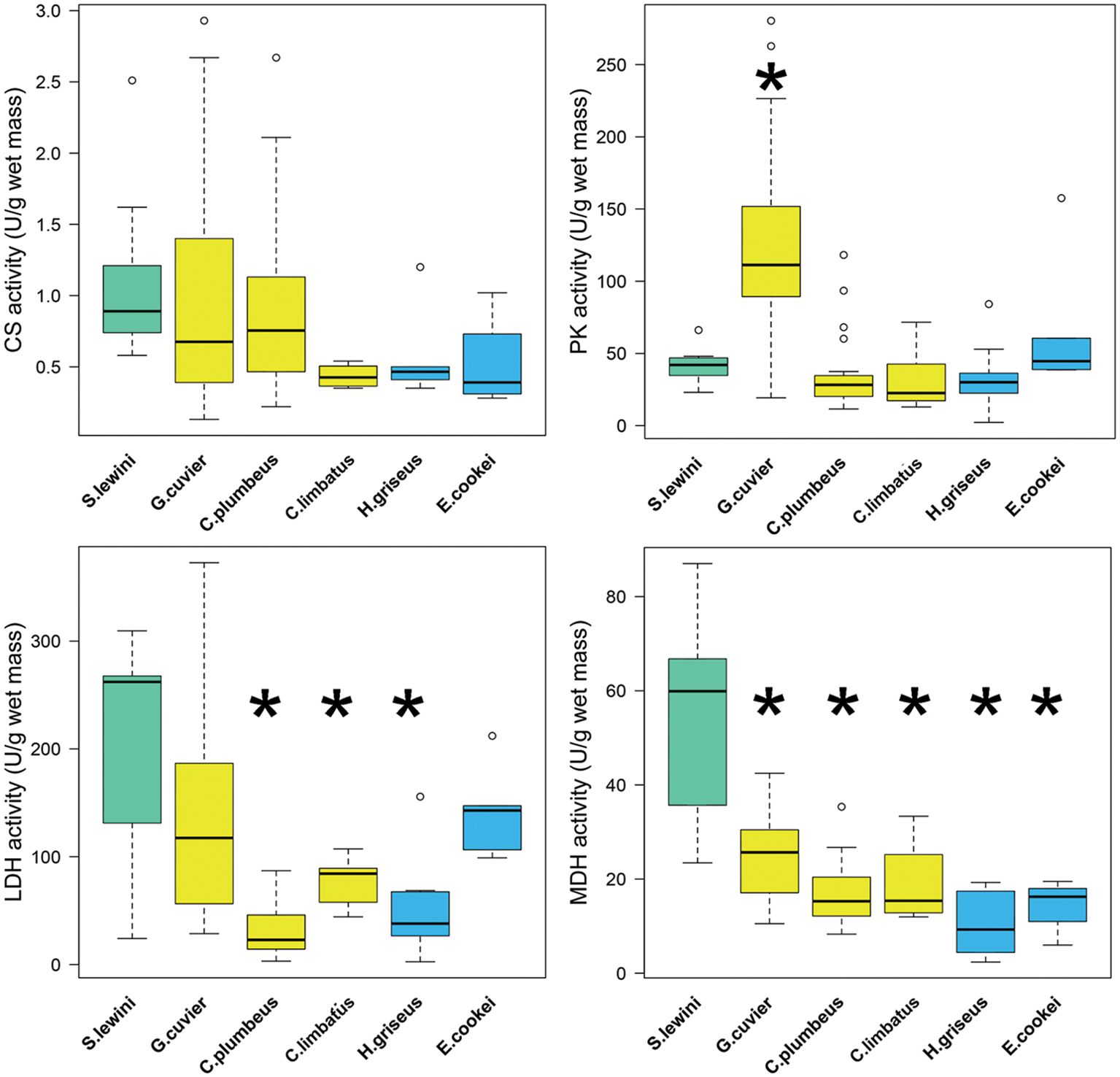
Figure 2. Boxplots of white muscle CS, PK, LDH, and MDH activities (U g-1 wet mass at 10°C) from (left to right) Sphyrna lewini (teal), three coastal species (yellow) (Galeocerdo cuvier, Carcharhinus plumbeus, Carcharhinus limbatus), and two deep-water species (blue) (Hexanchus griseus, Echinorhinus cookei). Thick line in each represents the mean, box ends are the 25th and 75th quantiles, dashed lines are min and max values, circles are outliers. Asterisks (*) indicate significant differences in species means in comparison to S. lewini (P-values < 0.05, Welsh’s ANOVA post-hoc Games-Howell test).
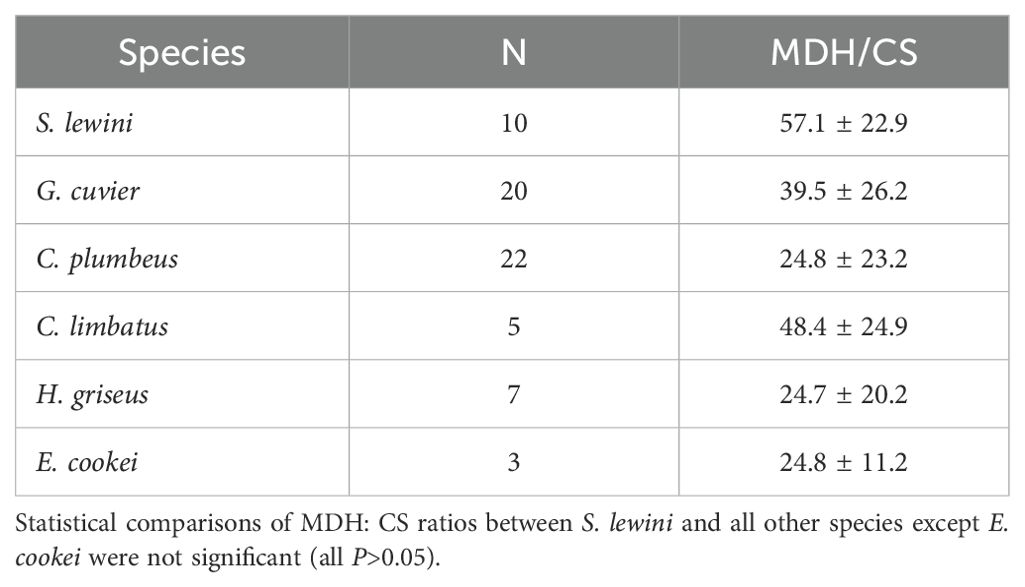
Table 2. Ratios (mean ± standard deviation) of MDH to CS enzyme activity in white muscle of each species.
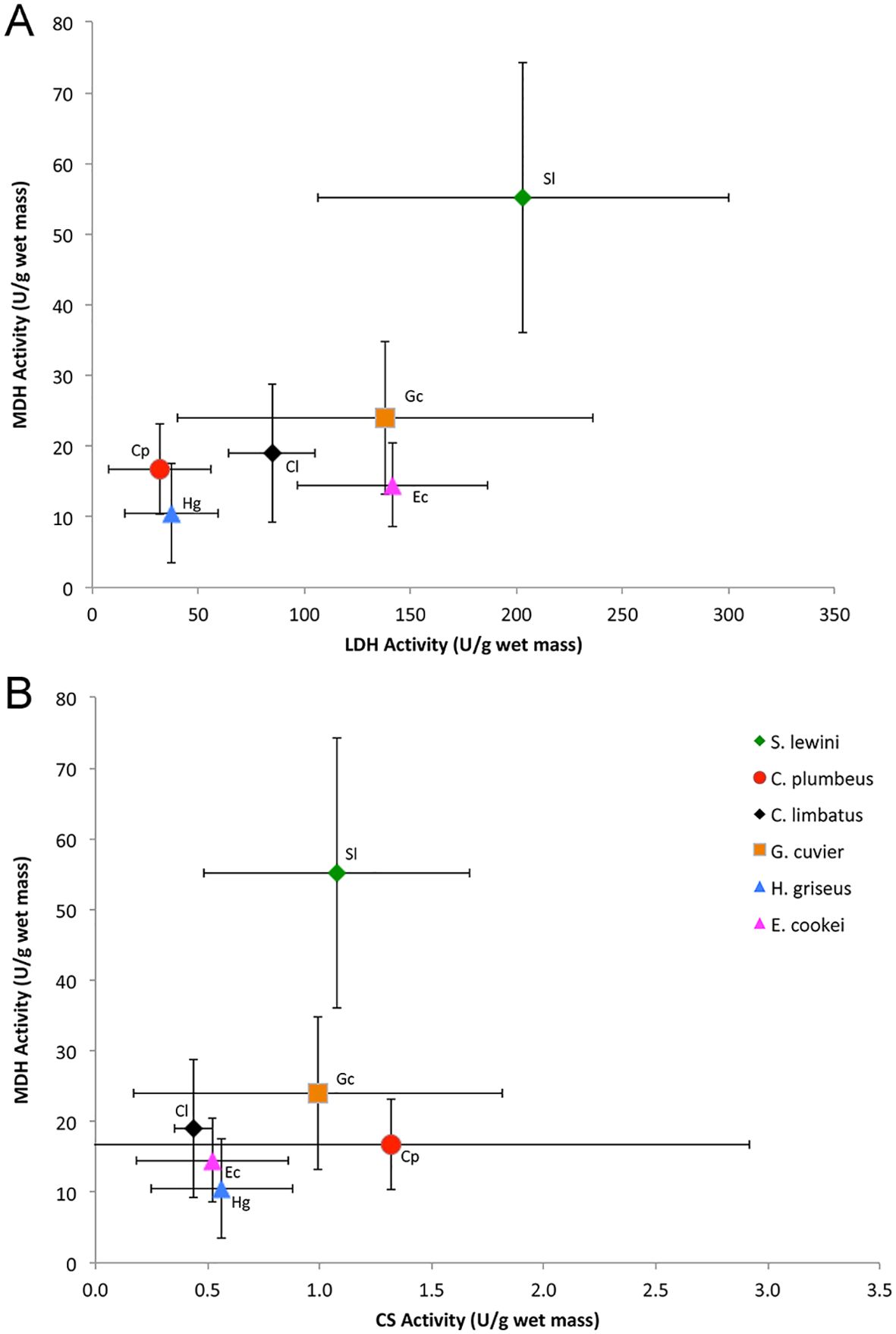
Figure 3. Comparison of mean white muscle activities (U g-1 wet mass at 10°C) of (A) LDH versus MDH and (B) CS versus MDH in the six shark species studied. Error bars indicate standard deviations. Though the statistical comparisons of MHD: CS ratios between S. lewini and all other species except E. cookei were not significant (all P>0.05), it is noticeable that G. cuvier and C. plumbeus also have high CS activity but not the correspondingly higher MDH activity observed in S. lewini.
Discussion
Based on its higher LDH and MDH activities (Figure 3A), and to a lesser extent the relatively high CS activity (Table 2, Figure 3B), S. lewini white muscle has higher anaerobic and aerobic capacities than other coastal carcharhinids and bathyal shark species that do not exhibit the repetitive nocturnal dives observed in S. lewini. Analyses of white muscle metabolic poise suggest that S. lewini possesses enzyme characteristics that facilitate high anaerobic metabolic activity during deep dives and the necessary aerobic metabolism to allow for rapid recovery, through the breakdown of anaerobic end products, when near the surface between dives. These physiological characteristics are proposed to enable the observed high activity levels of S. lewini even while they are suppressing gill function during dives into cold habitats. These same features would allow them to recover quickly in well-oxygenated surface waters through rapid processing of the lactate built up during anaerobic activity (Meekan and Gleiss, 2023). The intense swimming activity exhibited by S. lewini during deep dives requires ATP production at a high rate (Meekan and Gleiss, 2023). It is unknown to what extent anaerobic ATP production in the white muscle is required during dives, but the high intensity swimming, including frequent bursts, recorded by Royer et al. (2023) suggests that the white fast-twitch muscle is active at depth, making it likely that anaerobic metabolism and lactate production occur. This would then require the lactate to be removed from the white muscle or possibly processed within it after a dive. It is possible that red slow-twitch muscle is also recruited during the rapid but sustained swimming phases of each deep dive, reducing to some extent the amount of lactate produced in the white muscle. Regardless of the extent to which deep-diving behavior relies on aerobic versus anaerobic capacity, these behavioral and physiological characteristics likely enable S. lewini to exploit a very different ecological niche than other sympatric shark species (e.g., coastal carcharhinids with daytime distributions overlapping that of S. lewini).
The white muscle enzyme profile of S. lewini suggests that the deep-water hunting capacity of this species may be facilitated by rapid recovery in surface waters between dives. Higher levels of LDH allow for higher rates of glycolysis to power white muscle contraction during bursts and high-speed swimming, but can also cause a high rate of lactate buildup in the cytosol. The amount and fate of lactate produced in the white muscle of S. lewini during intensive swimming during dives is unknown. Previous studies indicate that teleosts and the dogfish shark, Squalus acanthias, retain lactate produced from strenuous exercise in their white muscle (for up to 4 hours in S. acanthias) (Milligan and Wood, 1986; Girard and Milligan, 1992; Richards et al., 2003), but little evidence for gluconeogenesis within fish white muscle has been found. Lactate can be converted to pyruvate and then used as a substrate for ATP synthesis or in gluconeogenesis under aerobic conditions. MDH plays a key role in the malate-aspartate cycle, to shuttle electrons across the inner mitochondrial membrane after the conversion of lactate to pyruvate (Ombres et al., 2011). The high MDH activity measured in S. lewini, in comparison to all other species examined, suggests a higher potential for this process in the white muscle under aerobic conditions (Suarez et al., 1985; Ombres et al., 2011). This is further supported by the high MDH: CS ratio observed in S. lewini (Table 2, Figure 3B). CS activity often scales with whole-body metabolic rate (Childress and Somero, 1979; Dahlhoff, 2004; Drazen and Seibel, 2007). Tiger (G. cuvier) and sandbar (C. plumbeus) sharks also have high CS activities, but not nearly the correspondingly high MDH activity measured in S. lewini, as reflected in the MDH: CS ratios (Figure 3B). MDH and CS are part of the citric acid cycle, and their activities in a given tissue should co-vary with mitochondrial content if their only role is as catalysts in the primary aerobic metabolic pathway (Dalziel et al., 2017). Thus, finding that MDH and the MDH: CS ratio are higher in S. lewini than in the other species studied suggests a more important role for MDH in S. lewini. The predominant role is likely to facilitate lactate clearance for post-exercise recovery after dives, but it may also be involved in redox balance during high-intensity activity. Although challenging, future studies measuring the production of lactate during dives and its subsequent fate during recovery from intensive activity, would be needed to distinguish among these alternatives and fully understand which biochemical processes underly the diving and foraging behavior of S. lewini.
Lactate processing may be initiated when S. lewini reaches the “inflection point” of the dive ascent at ~250 m, which marks reduced swimming activity and a slower rate of ascent, associated with rapidly cooling muscle temperatures (Meekan and Gleiss, 2023; Royer et al., 2023). Available evidence suggests that this is the moment when S. lewini resumes ram-ventilation in adequately oxygenated waters, which would allow rapid repayment of any oxygen debt incurred and to remove lactate accumulated during the dive (Meekan and Gleiss, 2023; Royer et al., 2023). Converting lactate into glycogen via gluconeogenesis in situ under aerobic conditions during the inter-dive period would allow S. lewini to reduce lactate concentration and replenish white muscle glycogen stores for subsequent deep dives (Iosilevskii et al., 2022). Gluconeogenesis could also occur in other tissues such as the liver and kidney (Suarez et al., 1985). Future studies should investigate the activities of key enzymes in the gluconeogenesis pathway in multiple tissues of S. lewini and other sharks. Although evidence for gluconeogenesis in white muscle has been observed in several teleosts (Milligan and Wood, 1986; Girard and Milligan, 1992), a study of activities of several key gluconeogenic enzymes in the white and red locomotor muscle, liver, and heart in mako, blue, and leopard sharks found no evidence for gluconeogenic capacity (Backey, 2007). Nevertheless, rapid removal of lactate by conversion to pyruvate and oxidation of pyruvate in the citric acid cycle would be beneficial for repeated dives by S. lewini.
In comparison to sympatric coastal and deep-water shark species, the higher capacity of S. lewini for aerobic and anaerobic metabolism and hence high activity during deep dives may confer a competitive advantage in actively foraging for deep-dwelling prey which may be relatively sluggish due to low body temperatures and metabolic rates (Childress, 1995; Seibel et al., 2000; Seibel and Drazen, 2007). Previous studies revealed a significant decline in tissue anaerobic capacities of the white muscle of deep-dwelling teleosts and cephalopods with increasing minimum depths of occurrence (Childress and Somero, 1979; Sullivan and Somero, 1980; Drazen and Seibel, 2007). For the sympatric coastal species that are adapted to warm-temperate waters, the deleterious physiological effects of moving into colder water make these deep prey resources generally inaccessible. Deep-water sharks (e.g. H. griseus and E. cookei) on the other hand are able to permanently inhabit these cold depths with access to deep prey patches, with the trade-off of living with reduced metabolic capacity. In contrast, by maintaining a high capacity for burst swimming and briefly maintaining a warm body temperature (Royer et al., 2023), S. lewini are able to conduct their intensive deep-dives to exploit an ecological niche that is inaccessible to sympatric coastal species (e.g., coastal carcharhinids), and have a more competitive, albeit transient, hunting capacity than the shark species that permanently inhabit these deep depths. The tradeoff for S. lewini is that they must have the energy reserves needed to power these brief and intensive deep dives (Meekan and Gleiss, 2023). Another potential trade-off is the increased vulnerability to predation during the inter-dive recovery period, similarly to beaked whales after their deep dives (Aguilar de Soto et al., 2020; Siegal et al., 2022).
Important caveats must be considered when interpreting the results of this study. Although it is known that enzyme activity scales with body size in many fishes, we did not detect any scaling effects in our study, most likely due to low sample sizes and limited size ranges for each species. Enzymatic activity can change based on a number of factors such as sampling period, time of day, recent feeding or mating events, and fish activity level (Yang and Somero, 1993; Dahlhoff, 2004; Li et al., 2012) - none of which are known for any of the sharks that were sampled for this study, but could explain some of the variability in the data. However, the same factors affect other published studies of fish samples from wild populations. Tissue samples were collected from live sharks under challenging field conditions and with rapid handling times to ensure proper animal welfare upon release, especially for species that are more sensitive to capture stress, particularly S. lewini (Hutchinson et al., 2023).
Conclusions
The scalloped hammerhead shark possesses enzyme characteristics that probably facilitate anaerobic metabolism during deep dives and rapid recovery during inter-dive intervals through the rapid aerobic breakdown of anaerobic end products. The high aerobic and anaerobic metabolic capacities measured in the white muscle of S. lewini are likely crucial for conducting repeated high-activity, deep dives while the shark “holds its breath” by suppressing gill function. High levels of MDH likely facilitate clearance of lactate and possibly restoration of glycogen stores in the white muscle when the sharks are in well-oxygenated surface waters. The results also raise further questions about the oxygen storage capacity of S. lewini, and whether hypoxia occurs during deep foraging dives (Meekan and Gleiss, 2023). A greater understanding of the metabolic pathways and oxygen demands during dives and post-dive recovery may explain the paradox of how this species is capable of routinely exerting itself to conduct intense deep dives while suppressing gill function, yet is also highly vulnerable to rapid stress-induced mortality from fishing capture. This ability to forage actively in deep waters and to rapidly recover from periods powered by anaerobic metabolism may be key to the ecological success of S. lewini, which has historically been circumglobally abundant in coastal and offshore waters (Rigby et al., 2019).
Data availability statement
The raw data supporting the conclusions of this article will be made available by the authors, without undue reservation.
Ethics statement
The animal study was approved by Shark capture and sampling activities were carried out in accordance with the animal use protocols of the University of Hawai'i Institutional Animal Care and Use Committee (IACUC) and were approved under IACUC protocols #05-053 and #11-1242. The study was conducted in accordance with the local legislation and institutional requirements.
Author contributions
MR: Conceptualization, Data curation, Formal Analysis, Funding acquisition, Investigation, Methodology, Project administration, Resources, Validation, Visualization, Writing – original draft, Writing – review & editing. DG: Conceptualization, Data curation, Formal Analysis, Funding acquisition, Investigation, Methodology, Project administration, Resources, Validation, Writing – review & editing. KD: Conceptualization, Data curation, Formal Analysis, Methodology, Resources, Software, Supervision, Validation, Writing – review & editing. KW: Conceptualization, Data curation, Funding acquisition, Investigation, Methodology, Project administration, Resources, Supervision, Validation, Writing – review & editing. CM: Funding acquisition, Project administration, Resources, Supervision, Validation, Writing – review & editing. KH: Funding acquisition, Resources, Supervision, Writing – review & editing, Validation. JD: Conceptualization, Data curation, Formal Analysis, Funding acquisition, Investigation, Methodology, Project administration, Resources, Supervision, Validation, Visualization, Writing – review & editing.
Funding
The author(s) declare financial support was received for the research, authorship, and/or publication of this article. This work was supported by the Jessie D. Kay Memorial Research Grant (UH Mānoa Department of Biology, now Department of Life Sciences), Hawaiʻi Institute of Marine Biology Lord Endowed Scholarship, Robinson Family Ocean Studies Assistantship, and the Pelagic Fisheries Research Program, under Cooperative Agreements NA17RJ1230 and NA09OAR4320075 between the Joint Institute for Marine and Atmospheric Research (JIMAR) and the National Oceanic and Atmospheric Administration (NOAA). Additional support was provided by the U.S. Department of Energy’s Wind and Water Power Program under Award DE-FG36-08GO18180 to the Hawaii Natural Energy Institute (HNEI). K Dickson’s work on the manuscript was supported by the U.S. National Science Foundation (NSF). Any opinion, findings, and conclusions or recommendations expressed in this material are those of the authors and do not necessarily reflect the views of the NSF.
Acknowledgments
We thank J. Muir, T. Swenarton, C. Comfort, A. Gray, G. Del Raye, G. Perez-Andujar, J. Anderson, J. Clark, M. Hutchinson, C. Ambrosino, D. Lau, J. Friedman, K. Carlson, K. Haiat, N. Hu, B. Rackliffe, J. Lazor, P. Mino, T. Clegg, M. Maxwell, S. Rucker, K. Scidmore-Rossing, K. Maloney, E. Cardona, C. Blandino, G. Silva, K. Whittingham, D. Coffey, and D. Itano. The lead author thanks B. Bowen, A. Seale, and M. Yoshizawa for providing feedback on parts of this study as dissertation committee members, of which K. Holland was chair. Bait was generously donated by the United Fishing Agency and we thank Brooks Tanaka and Nelson Aberilla. The Hawaiʻi Institute of Marine Biology and University of Hawaiʻi Marine Center provided logistical support and we thank S. Shor, R. Barnes and B. Hunt. This is contribution no. 1975 from the Hawaiʻi Institute of Marine Biology and no. 11867 from the School of Ocean and Earth Science and Technology at the University of Hawaiʻi.
Conflict of interest
The authors declare that the research was conducted in the absence of any commercial or financial relationships that could be construed as a potential conflict of interest.
Publisher’s note
All claims expressed in this article are solely those of the authors and do not necessarily represent those of their affiliated organizations, or those of the publisher, the editors and the reviewers. Any product that may be evaluated in this article, or claim that may be made by its manufacturer, is not guaranteed or endorsed by the publisher.
Author disclaimer
The views expressed herein are those of the authors and do not necessarily reflect the views of NOAA or any of its subdivisions.
References
Aguilar de Soto N., Visser F., Tyack P. L., Alcazar J., Ruxton G., Arranz P., et al. (2020). Fear of killer whales drives extreme synchrony in deep diving beaked whales. Sci. Rep. 10, 13. doi: 10.1038/s41598-019-55911-3
Anderson J. M., Rex P. T., Maloney K., Johnston M., Verbeck D., Allen N., et al. (2022). Observations of a species-record deep dive by a central Pacific female scalloped hammerhead shark (Sphyrna lewini). J. Fish Biol. 101, 323–327. doi: 10.1111/jfb.15115
Andrzejaczek S., Gleiss A. C., Pattiaratchi C. B., Meekan M. G. (2018). First insights into the fine-scale movements of the sandbar shark, carcharhinus plumbeus. Front. Mar. Sci. 5. doi: 10.3389/fmars.2018.00483
Backey J. M. (2007). The Potential for Lactate Processing in Shortfin Mako, Leopard, and Blue Sharks (Fullerton: California State University). doi: 10.1016/j.jaci.2012.05.050
Bernal D., Smith D., Lopez G., Weitz D., Grimminger T., Dickson K., et al. (2003). Comparative studies of high performance swimming in sharks II. Metabolic biochemistry of locomotor and myocardial muscle in endothermic and ectothermic sharks. J. Exp. Biol. 206, 2845–2857. doi: 10.1242/jeb.00504
Bessudo S., Soler G. A., Klimley P. A., Ketchum J., Arauz R. (2011). Vertical and horizontal movements of the scalloped hammerhead shark (Sphyrna lewini) around malpelo and Cocos Islands (Tropical Eastern Pacific) using satellite telemetry. Boletín Investig. Mar. Costeras 40, 91–106. doi: 10.25268/bimc.invemar.2011.40.0.133
Childress J. J. (1995). Are there physiological and biochemical adaptations of metabolism in deep-sea animals? Trends Ecol. Evol. 10, 30–36. doi: 10.1016/S0169-5347(00)88957-0
Childress J. J., Somero G. N. (1979). Depth-related enzymic activities in muscle, brain and heart of deep-living pelagic marine teleosts. Mar. Biol. 52, 273–283. doi: 10.1007/BF00398141
Coffey D. M., Royer M. A., Meyer C. G., Holland K. N. (2020). Diel patterns in swimming behavior of a vertically migrating deepwater shark, the bluntnose sixgill (Hexanchus griseus). PloS One 15, e0228253. doi: 10.1371/journal.pone.0228253
Comfort C. M., Weng K. C. (2015). Vertical habitat and behaviour of the bluntnose sixgill shark in Hawaii. Deep Sea Res. Part II Top. Stud. Oceanogr. 115, 116–126. doi: 10.1016/j.dsr2.2014.04.005
Condon N. E., Friedman J. R., Drazen J. C. (2012). Metabolic enzyme activities in shallow- and deep-water chondrichthyans: implications for metabolic and locomotor capacity. Mar. Biol. 159, 1713–1731. doi: 10.1007/s00227-012-1960-3
Dahlhoff E. P. (2004). Biochemical indicators of stress and metabolism: Applications for marine ecological studies. Annu. Rev. Physiol. 66, 183–207. doi: 10.1146/annurev.physiol.66.032102.114509
Dalziel A. C., Laporte M., Rougeux C., Guderley H., Bernatchez L. (2017). Convergence in organ size but not energy metabolism enzyme activities among wild Lake Whitefish (Coregonus clupeaformis) species pairs. Mol. Ecol. 26, 225–244. doi: 10.1111/mec.13847
Dickson K. A. (1995). Unique adaptations of the metabolic biochemistry of tunas and billfishes for life in the pelagic environment. Environ. Biol. Fishes 42, 65–97. doi: 10.1007/BF00002352
Dickson K. A., Gregorio M. O., Gruber S. J., Loefler K. L., Tran M., Terrell C. (1993). Biochemical indices of aerobic and anaerobic capacity in muscle tissues of California elasmobranch fishes differing in typical activity level. Mar. Biol. 117, 185–193. doi: 10.1007/BF00345662
Drazen J. C., Friedman J. R., Condon N. E., Aus E. J., Gerringer M. E., Keller A. A., et al. (2015). Enzyme activities of demersal fishes from the shelf to the abyssal plain. Deep. Res. Part I Oceanogr. Res. Pap. 100, 117–126. doi: 10.1016/j.dsr.2015.02.013
Drazen J. C., Seibel B. A. (2007). Depth-related trends in metabolism of benthic and benthopelagic deep-sea fishes. Limnol. Ocean. 52, 2306–2316. doi: 10.4319/lo.2007.52.5.2306
Duong C. A., Sepulveda C. A., Graham J. B., Dickson K. A. (2006). Mitochondrial proton leak rates in the slow, oxidative myotomal muscle and liver of the endothermic shortfin mako shark (Isurus oxyrinchus) and the ectothermic blue shark (Prionace glauca) and leopard shark (Triakis semifasciata). J. Exp. Biol. 209, 2678–2685. doi: 10.1242/jeb.02317
Ebert D. A. (2002). Some observations on the reproductive biology of The sixgill shark Hexanchus griseus (Bonnaterre 1778) from southern African waters. South Afr. J. Mar. Sci. 24, 359–363. doi: 10.2989/025776102784528439
Friedman J. R., Condon N. E., Drazen J. C. (2012). Gill surface area and metabolic enzyme activities of demersal fishes associated with the oxygen minimum zone off California. Limnol. Oceanogr. 57, 1701–1710. doi: 10.4319/lo.2012.57.6.1701
Girard S. S., Milligan C. L. (1992). The Metabolic Fate of Blood-Borne Lactate in Winter Flounder (Pseudopleuronectes americanus) during Recovery from Strenuous Exercise. Physiol. Zool. 65, 1114–1134. doi: 10.1086/physzool.65.6.30158271
Guppy M., Hochachka P. W. (1978). Controlling the highest lactate dehydrogenase activity known in nature. Am. J. Physiol. - Regul. Integr. Comp. Physiol. 3, 136-140. doi: 10.1152/ajpregu.1978.234.3.r136
Hochachka P. W., Somero G. N. (2002). Biochemical adaptation: mechanism and process in physiological evolution (Oxford, UK: Oxford University Press).
Hochachka P. W., Stanley C., Merkt J., Sumar-Kalinowski J. (1982). Metabolic meaning of elevated levels of oxidative enzymes in high altitude adapted animals: an interpretive hypothesis. Respir. Physiol. 52, 303–313. doi: 10.1016/0034-5687(83)90087-7
Hoffmayer E. R., Franks J. S., Driggers W. B., Howey P. W. (2013). Diel vertical movements of a scalloped hammerhead, Sphyrna lewini, in the northern Gulf of Mexico. Bull. Mar. Sci. 89, 551–557. doi: 10.5343/bms.2012.1048
Hutchinson M., Scott M., Bauer R., Anderson J., Coffey D., Holland K., et al. (2023). Habitat use and movement patterns of adult male and juvenile scalloped hammerhead sharks, Sphyrna lewini, throughout the Hawaiian archipelago. Endanger. Species Res. 52, 41–64. doi: 10.3354/esr01267
Iosilevskii G., Kong J. D., Meyer C. G., Watanabe Y. Y., Papastamatiou Y. P., Royer M. A., et al. (2022). A general swimming response in exhausted obligate swimming fish. R. Soc Open Sci. 9, 211869. doi: 10.1098/rsos.211869
Jorgensen S. J., Klimley A. P., Muhlia-Melo A. F. (2009). Scalloped hammerhead shark sphyrna lewini, utilizes deep-water, hypoxic zone in the gulf of California. J. Fish Biol. 74, 1682–1687. doi: 10.1111/j.1095-8649.2009.02230.x
Kane D. A. (2014). Lactate oxidation at the mitochondria: A lactate-malate-aspartate shuttle at work. Front. Neurosci. 8. doi: 10.3389/fnins.2014.00366
Kohler N. E., Casey J. G., Turner P. A. (1996). Length-weight relationships for 13 species of sharks from the western North Atlantic. Fish. Bull. 93, 412–418.
Li G., Lee P., Mori N., Yamamoto I., Arai T. (2012). Long term intensive exercise training leads to a higher plasma malate/lactate dehydrogenase (M/L) ratio and increased level of lipid mobilization in horses. Vet. Res. Commun. 36, 149–155. doi: 10.1007/s11259-012-9515-0
Milligan C. L., Wood C. M. (1986). Intracellular and extracellular acid-base status and H+ exchange with the environment after exhaustive exercise in the rainbow trout. J. Exp. Biol. VOL. 123, 93–121. doi: 10.1242/jeb.123.1.93
Moore A. B. M., Gates A. R. (2015). Deep-water observation of scalloped hammerhead Sphyrna lewini in the western Indian Ocean off Tanzania. Mar. Biodivers. Rec. 8, 1–4. doi: 10.1017/S1755267215000627
Moyes C. D., Mathieu-Costello O. A., Brill R. W., Hochachka P. W. (1992). Mitochondrial metabolism of cardiac and skeletal muscles from a fast (Katsuwonus pelamis) and a slow (Cyprinus carpio) fish. Can. J. Zool. 70, 1246–1253. doi: 10.1139/z92-172
Nakamura I., Meyer C. G., Sato K. (2015). Unexpected Positive Buoyancy in Deep Sea Sharks, Hexanchus griseus, and a Echinorhinus cookei. PloS One 10, e0127667. doi: 10.1371/journal.pone.0127667
Nakamura I., Watanabe Y., Papastamatiou Y., Sato K., Meyer C. (2011). Yo-yo vertical movements suggest a foraging strategy for tiger sharks Galeocerdo cuvier. Mar. Ecol. Prog. Ser. 424, 237–246. doi: 10.3354/meps08980
Ombres E. H., Donnelly J., Clarke M. E., Harms J. H., Torres J. J. (2011). Aerobic and anaerobic enzyme assays in Southern California Rockfish: Proxies for physiological and ecological data. J. Exp. Mar. Bio. Ecol. 399, 201–207. doi: 10.1016/j.jembe.2010.11.007
Pinte N., Coubris C., Jones E., Mallefet J. (2021). Red and white muscle proportions and enzyme activities in mesopelagic sharks. Comp. Biochem. Physiol. Part - B Biochem. Mol. Biol. 256, 110649. doi: 10.1016/j.cbpb.2021.110649
Pollack A. G., Iii W. B. D., Hanisko D. S., Ingram G. W. (2019). Distribution and Length Data for Blacktip Sharks Captured on the NOAA / NMFS / SEFSC / MSLABS Bottom Longline Survey in the Western North Atlantic Ocean (North Charleston, SC).
Pörtner H. O. (2002). Climate variations and the physiological basis of temperature dependent biogeography: Systemic to molecular hierarchy of thermal tolerance in animals. Comp. Biochem. Physiol. - A Mol. Integr. Physiol. 132, 739–761. doi: 10.1016/S1095-6433(02)00045-4
Richards J. G., Heigenhauser G. J. F., Wood C. M. (2003). Exercise and recovery metabolism in the pacific spiny dogfish (Squalus acanthias). J. Comp. Physiol. B Biochem. Syst. Environ. Physiol. 173, 463–474. doi: 10.1007/s00360-003-0354-8
Rigby C. L., Dulvy N. K., Barreto R., Carlson J., Fernando D., Fordham S., et al. (2019). Sphyrna lewini. IUCN Red List Threat. Species. e.T39385A2918526.
Rogatzki M. J., Ferguson B. S., Goodwin M. L., Gladden L. B. (2015). Lactate is always the end product of glycolysis. Front. Neurosci. 9. doi: 10.3389/fnins.2015.00022
Royer M., Meyer C., Royer J., Maloney K., Cardona E., Blandino C., et al. (2023). Breath holding“ as a thermoregulation strategy in the deep-diving scalloped hammerhead shark. Science. 380, 651–655. doi: 10.1126/science.add4445
Saavedra L. M., Quiñones R. A., Gonzalez-Saldía R. R., Niklitschek E. J. (2016). Aerobic and anaerobic enzymatic activity of orange roughy (Hoplostethus atlanticus) and alfonsino (Beryx splendens) from the Juan Fernandez seamounts area. Fish Physiol. Biochem. 42, 869–882. doi: 10.1007/s10695-015-0181-3
Seamone S. G., Syme D. A. (2016). Elasmobranch muscle structure and mechanical properties, In: Physiology of Elasmobranch Fishes: Structure and Interaction with Environment. 189-218. doi: 10.1016/B978-0-12-801289-5.00005-5
Seibel B. A., Drazen J. C. (2007). The rate of metabolism in marine animals: Environmental constraints, ecological demands and energetic opportunities. Philos. Trans. R. Soc B Biol. Sci. 362, 2061–2078. doi: 10.1098/rstb.2007.2101
Seibel B. A., Thuesen E. V., Childress J. J. (2000). Light-limitation on predator-prey interactions : consequences for metabolism and locomotion of deep-sea cephalopods. Biol. Bull. 198, 284–298. doi: 10.2307/1542531
Siegal E., Hooker S. K., Isojunno S., Miller P. J. O. (2022). Beaked whales and state-dependent decision-making: how does body condition affect the trade-off between foraging and predator avoidance? Proc. R. Soc B Biol. Sci. 289, 20212539. doi: 10.1098/rspb.2021.2539
Somero G. N. (1992). Biochemical ecology of deep-sea animals. Experientia 48, 537–543. doi: 10.1007/BF01920236
Somero G. N., Childress J. J. (1980). A violation of the metabolism-size scaling paradigm: activities of glycolytic enzymes in muscle increase in larger-size fish. Physiol. Zool. 53, 322–337. doi: 10.1086/physzool.53.3.30155794
Spaet J. L. Y., Lam C. H., Braun C. D., Berumen M. L. (2017). Extensive use of mesopelagic waters by a scalloped hammerhead shark (Sphyrna lewini) in the Red Sea. Anim. Biotelemetry. doi: 10.1186/s40317-017-0135-x
Suarez R. K., Mallet M. D., Daxboeck C., Hochachka P. W. (1985). Enzymes of energy metabolism and gluconeogenesis in the Pacific blue marlin, Makaira nigricans. Can. J. Zool. 64, 694–697. doi: 10.1139/z86-102
Sullivan K. M., Somero G. N. (1980). Enzyme activities of fish skeletal muscle and brain as influenced by depth of occurrence and habits of feeding and locomotion. Mar. Biol. 60, 91–99. doi: 10.1007/BF00389152
Torres J. J., Somero G. N. (1988). Metabolism, enzymic activities and cold adaptation in Antarctic mesopelagic fishes. Mar. Biol. 98, 169–180. doi: 10.1007/BF00391192
Treberg J. R., Martin R. A., Driedzic W. R. (2003). Muscle Enzyme Activities in a Deep-Sea Squaloid Shark, Centroscyllium fabricii, Compared With Its Shallow-Living Relative, Squalus acanthias. J. Exp. Zool 300A, 133–139. doi: 10.1002/jez.a.10318.134
Vetter R. D., Lynn E. A. (1997). Bathymetric demography, enzyme activity patterns, and bioenergetics of deep-living scorpaenid fishes (genera Sebastes and Sebastolobus): Paradigms revisited. Mar. Ecol. Prog. Ser. 155, 173–188. doi: 10.3354/meps155173
Williams T. M., Dobson G. P., Mathieu-Costello O., Morsbach D., Worley M. B., Phillips J. A. (1997). Skeletal muscle histology and biochemistry of an elite sprinter, the African cheetah. J. Comp. Physiol. - B Biochem. Syst. Environ. Physiol. 167, 527–535. doi: 10.1007/s003600050105
Yang T., Somero G. N. (1993). Effects of feeding and food deprivation on oxygen consumption, muscle protein concentration and activities of energy metabolism enzymes in muscle and brain of shallow-living (Scorpaena guttata) and deep-living (Sebastolobus alascanus) Scorpaenid fishes. J. Exp. Biol. 181, 213–232. doi: 10.1242/jeb.181.1.213
Keywords: enzyme activity, lactate dehydrogenase, malate dehydrogenase, white muscle, Sphyrna lewini, Hexanchus griseus, deep-diving
Citation: Royer M, Garcia D, Dickson K, Weng KC, Meyer C, Holland KN and Drazen JC (2024) Aerobic and anaerobic poise of white swimming muscles of the deep-diving scalloped hammerhead shark: comparison to sympatric coastal and deep-water species. Front. Mar. Sci. 11:1477553. doi: 10.3389/fmars.2024.1477553
Received: 08 August 2024; Accepted: 22 October 2024;
Published: 11 November 2024.
Edited by:
Emily Lester, Australian Institute of Marine Science (AIMS), AustraliaReviewed by:
Adrian C Gleiss, Murdoch University, AustraliaStephen T. Kinsey, University of North Carolina Wilmington, United States
Copyright © 2024 Royer, Garcia, Dickson, Weng, Meyer, Holland and Drazen. This is an open-access article distributed under the terms of the Creative Commons Attribution License (CC BY). The use, distribution or reproduction in other forums is permitted, provided the original author(s) and the copyright owner(s) are credited and that the original publication in this journal is cited, in accordance with accepted academic practice. No use, distribution or reproduction is permitted which does not comply with these terms.
*Correspondence: Mark Royer, cm95ZXJtQGhhd2FpaS5lZHU=