- 1School of Oceanography, University of Washington, Seattle, WA, United States
- 2Biology Department, Swarthmore College, Swarthmore, PA, United States
- 3Marine Science Institute, University of California, Santa Barbara, Santa Barbara, CA, United States
- 4Department of Ecology, Evolution, and Marine Biology, University of California, Santa Barbara, Santa Barbara, CA, United States
Marine heatwaves (MHWs) are of increasing concern due to the emerging ecological and socioeconomic impacts on coastal ecosystems. Leveraging the data of the Santa Barbara Coastal Long-Term Ecological Research project, we analyzed the MHW event metrics observed in the kelp forest ecosystem and across Santa Barbara Channel, CA, USA. Not only was there a significant positive trend in the number of MHWs recorded, their duration and intensity were also increasing over time. MHWs were detected year-round, suggesting that marine organisms have exposure risks regardless of their phenology. Exposure at one life history stage could have a legacy effect on the subsequent stages, implying little temporal refuge. In contrast, the coastal mooring data revealed that near-surface and bottom events were not necessarily coupled even at less than 15 m. Such spatial variation in MHWs might provide a temporary refuge for mobile species. These observations also highlight the importance of depth-stratified, long-term coastal monitoring to understand spatio-temporal variation in MHW stress on coastal communities.
1 Introduction
Extremes of warm ocean temperatures – events known as marine heatwaves (MHWs) – have caused ecological disturbance in marine ecosystems, and are expected to increase in frequency and intensity (Oliver, 2019). The biological impacts of MHWs have been significant, causing dramatic mortality events and altering community structure via changes in biodiversity (Leggat et al., 2019; Sanford et al., 2019; Smith et al., 2023; Joyce et al., 2024). While MHWs have been defined as extreme anomalies in sea surface temperature (Hobday et al., 2016, 2018), these events are far from being a surface-only phenomenon. Analyses of bottom or subsurface MHWs (Amaya et al., 2023b; Sun et al., 2023; Wyatt et al., 2023) highlight the needs and urgency to reflect on how MHW events might interact with coastal marine communities using nearshore monitoring data given their spatial and temporal dynamics.
MHWs are particularly impactful in coastal ecosystems. The rapid onset, seasonality, and vertical dynamics of these extreme events interact with a wide array of species, including foundational species such as kelp and coral (Leggat et al., 2019; Rogers-Bennett and Catton, 2019; Smith et al., 2024), and in turn alter key ecological processes such as reproduction (Wild et al., 2019; Shanks et al., 2020; Peruzza et al., 2023). First, MHWs occur quickly relative to ecological time-scales, often leaving little time for species to acclimatize (Stillman, 2019; Guo et al., 2022; Amaya et al., 2023a). Furthermore, the seasonal timing can align with critical life history events (Leach et al., 2021; Harvey et al., 2022), creating phenological mismatches (Laurel et al., 2021; Correia-Martins et al., 2022). Lastly, bottom MHWs can expose vulnerable key sessile species to heat stress (Amaya et al., 2023b). However, there are contrasting reports that demersal fishes lack significant responses to MHWs (Fredston et al., 2023). Such penetrations of warm temperature to the benthos have resulted in mortality (Rogers-Bennett and Catton, 2019; Thomsen et al., 2019; Smale, 2020; Wernberg, 2021), coral bleaching (Couch et al., 2017; Le Nohaïc et al., 2017; Gaspar et al., 2021; Wyatt et al., 2023), changes in biodiversity and ecological interactions (Reed et al., 2016; Sanford et al., 2019; Michaud et al., 2022; Starko et al., 2023), and restructuring of microhabitats and refugia after thermal stress (Woodson et al., 2019; Starko et al., 2022).
Kelp forests are ideal for examining the impacts of MHWs on a coastal ecosystem that is dominated by a single foundation species. The health of kelp forests globally has been threatened by MHWs, with large variations in local level responses (Cavanaugh et al., 2019; Filbee-Dexter et al., 2020; Smale, 2020; McPherson et al., 2021; Tait et al., 2021). The giant kelp (Macrocystis pyrifera) forest community of the Santa Barbara Channel within the California Current System serves as a model system to explore the multidimensional impacts of MHWs on kelp forests. This region is home to the Santa Barbara Coastal Long-Term Ecological Research (SBC LTER) project, which has collected in situ temperature at multiple study sites since 1999. The SBC LTER has collected both temperature data and biological data that has supported important observations regarding the ecological response within the region (Reed et al., 2016; Michaud et al., 2022; Kozal et al., 2024) and has been used to inform ecologically-relevant experiments (Leach et al., 2021; Clare et al., 2022; Lowman et al., 2022; Csik et al., 2023; Leach and Hofmann, 2023).
With MHWs predicted to increase in intensity and frequency (Oliver et al., 2018; Oliver, 2019), understanding their dynamics and vertical structure is critical, especially for near-shore ecosystems such as kelp forests that are characterized by a productive benthos. In this region, physical processes such as upwelling could modify the low-frequency background temperature and stratification and drive the initiation of MHWs (Dalsin et al., 2023). Similarly, interactions between coastal features and internal waves can alter the adjustment of the thermocline and heat transport (Zhang et al., 2023). It is therefore important to consider near-shore dynamics when investigating the future of MHWs.
Using in-situ data from the SBC LTER within the coastal kelp forest, we compared the characteristics and trends of MHW events detected through mooring data with those identified by satellite-based sea surface temperature. We hypothesized that MHWs occur not only at the channel-wide spatial scale but also across the vertical water column, with similar frequency and intensity. Additionally, given the shallow nature of the coastal SBC LTER sites (<15 m), we predicted that near-surface and bottom MHW metrics would be uniform. Alternatively, we considered the possibility that MHWs might exhibit earlier onset and higher intensity near the surface due to solar insolation. The ultimate goal of this work is to enhance our understanding of how MHWs affect shallow coastal ecosystems, providing crucial insights into the threats these events pose to marine biodiversity and resilience.
2 Materials and methods
2.1 SST data sets
Sea surface temperature (SST) from the Santa Barbara Channel area (from -120.625° to -119.375° and 33.875° to 34.625°) was retrieved from the NOAA National Centers for Environmental Information via the ERRDAP Server (https://coastwatch.pfeg.noaa.gov/erddap/griddap/ncdcOisst21Agg.html). This Daily Optimum Interpolation Sea Surface Temperature Analysis (Version 2.1) derived its data from satellite (Advanced Very High Resolution Radiometer) and in situ platforms (i.e., ships and buoys). The analysis provided 18 grid points (hereafter referred to as sites) within the Santa Barbara Channel, with a spatial resolution of 0.25° and temporal coverage spanning from 1982 to 2023 (Kui, 2024). The vertical temperature profile data was retrieved from long-term moorings (2004-2023) managed by the SBC LTER at five coastal sites, namely Alegria Reef (Washburn et al., 2024a), Arroyo Quemada (Washburn et al., 2024b), Naples Reef (Washburn et al., 2024e), Mohawk (Washburn et al., 2024d), and Carpinteria (Washburn et al., 2024c). These data were gathered from one Conductivity, Temperature, and Depth sensor (SBE37CTD) as well as two Onset StowAway Tidbit Temp Loggers at 20-min intervals at each site’s mooring. The SST data were gathered at approximately 2-4 m (“near-surface”) and the bottom temperature data were gathered at 9-15m depth (“deep”, Figure 1). The bottom Tidbits were mounted approximately 1m above the sea floor, while the Acoustic Doppler Current Profiler (ADCP) was positioned about 10 meters away at a similar depth, half a meter above the seafloor. Therefore, the depth at which the sensors were mounted differed between each site and reflects local bathymetry (Supplementary Table 1). All data are publicly available in the Environmental Data Initiative (EDI) repository. The near-surface and bottom temperature data used in the analysis were primarily sourced from the Tidbit, with the temperature readings from the SBE37 CTD and ADCP filling gaps when the Tidbit data for the corresponding depths and sites were unavailable.
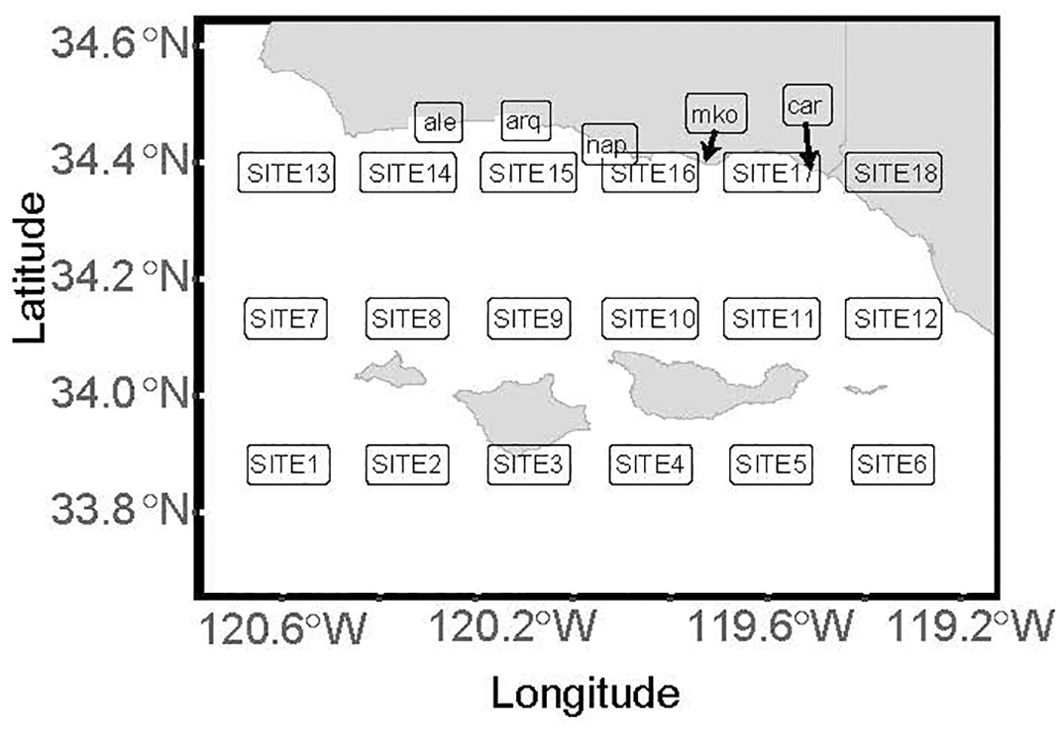
Figure 1. Santa Barbara Channel (SBC), the study area. At a 0.25° resolution, 18 grid points (sites) within the SBC were used for sea surface temperature analysis from 1983-2023. An additional five SBC long-term mooring sites from 2004-2023 were used for the coastal analysis. The coastal sites are Alegria Reef (ale), Arroyo Quemada (arq), Naples Reef (nap), Mohawk (mko) and Carpinteria (car). The four islands in the channel from west to east: Santa Miguel, Santa Rosa, Santa Cruz, Anacapa.
2.2 Statistical analysis
2.2.1 Marine heatwave identification
MHW events were identified separately for each of the sites (satellite grid data and mooring data) and depths (mooring data), following the methodology of Hobday et al. (2016). A discrete MHW event refers to times during which SST was above the threshold for at least five consecutive days. The daily threshold was the 90th percentile of the temperature recorded across all daily temperatures from 1982-2023 within an 11-day window centered on the day, which were then smoothed using a 31-day moving average following Hobday et al. (2016). Consecutive events that were separated by less than two days were combined into one. For each event, metrics described by Hobday et al. (2016) were computed: “duration” is the time between the event’s start and end dates; “maximum intensity” is the maximum temperature anomaly compared to the seasonal-varying mean over the duration of the event, “cumulative intensity” is the cumulated daily temperature anomaly over the duration of the event, and “onset and decline rate” is the rate of temperature increase or decrease at the start and end of the event, respectively. All computations of heatwave metrics were performed using the heatwaveR package (version 0.4.6) (Schlegel and Smit, 2018) in R (version 4.1.2).
2.2.2 Spatial-temporal analysis
To examine the spatial and temporal pattern of the MHWs, an empirical orthogonal function (EOF) was performed on the time series of annual total MHW days for each of the 18 sites across the study period. This metric was chosen after Oliver et al. (2018) and because of its simplicity – more frequent and longer events both increase this metric. The analysis was conducted with the MetR package (version 0.15.0) in R (Campitelli, 2021). To study the temporal trend of MHWs, we compared the trends in total intensity and duration of MHWs observed between 1983-2003 and 2004-2023 with Kendall’s tau based Sen’s slope estimator (Sen, 1968). Mann-Kendall test method in the trend package (version 1.16) in R was used to determine the significance of the detected trends (Pohlert, 2023).
To investigate the vertical structure of MHWs observed, we computed the nearest pairing from a top event to a bottom event and vice versa for the coastal mooring data based on the start date of the event. If the top-and-bottom pairing agreed, the two observations were considered as a single event, unless the difference in initiation dates exceeded five days. All unpaired observations were labeled as top-only or bottom-only event.
3 Results
3.1 Satellite data trends
Based on the 40-year satellite data, there were on average two MHW events annually in the Santa Barbara Channel. The average duration of the event was 11.2 days and the mean average intensity of the events was 2.01°C (Figures 2A-C). MHW events were detected in all months of the year (Figure 3A). There were over 20 events in spring (Mar-May) and summer (Jun – Aug), which was higher than the number recorded in fall (Sept – Nov; 14 events) and winter (Dec – Feb; 10 events). These MHW events lasted the longest in fall (28 days) and had an average intensity of 2.3°C.
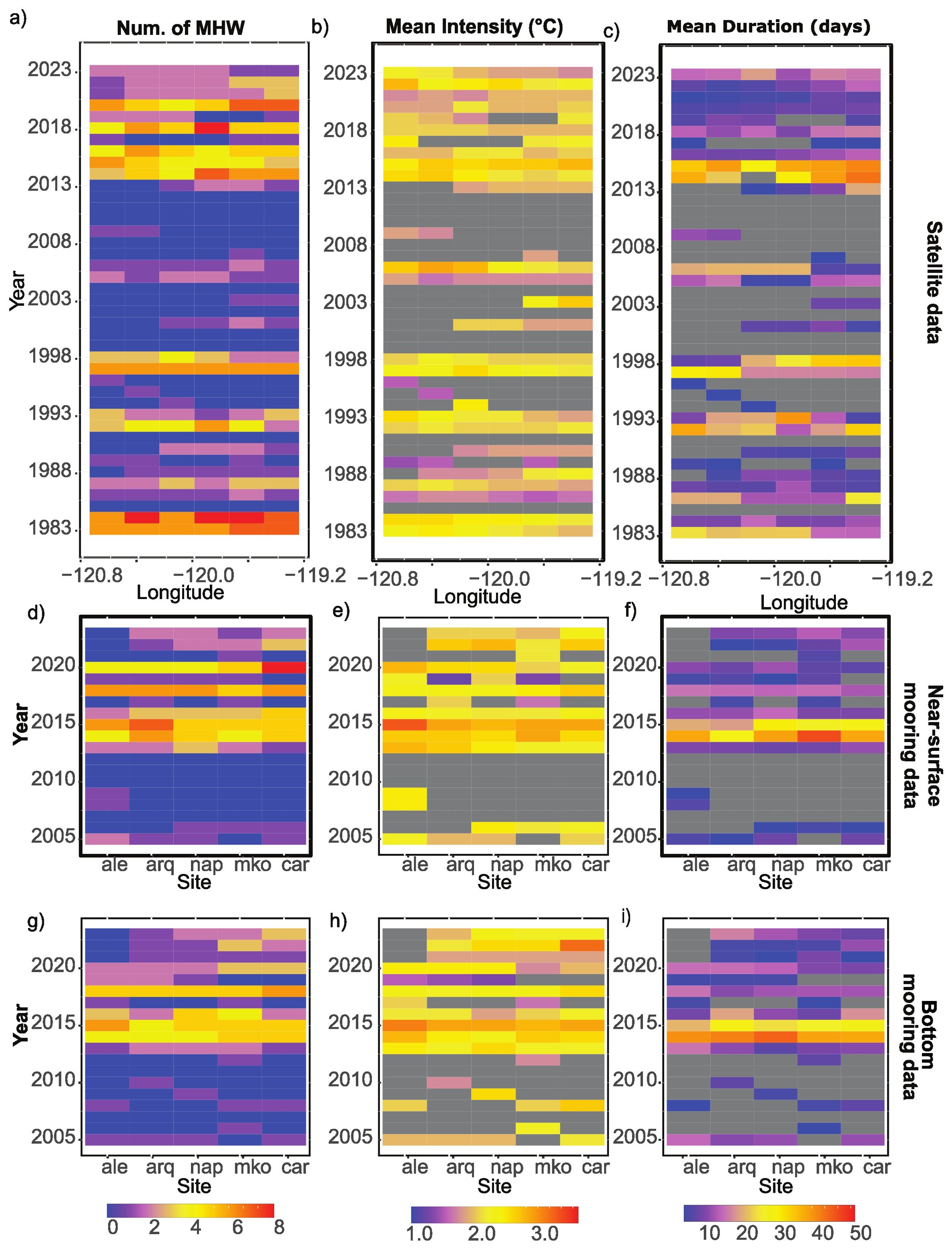
Figure 2. Summary statistics of marine heatwave (MHW) metrics between 1983-2023 of the Santa Barbara Channel (A-C), using sites 7-12; MHW metrics from 2004-2023 near-surface (D-F) and bottom (G-I) mooring from five sites of the SBC LTER project (see Figure 1 for station locations). The gray area indicates no data because there was no MHW during that time at the given site.
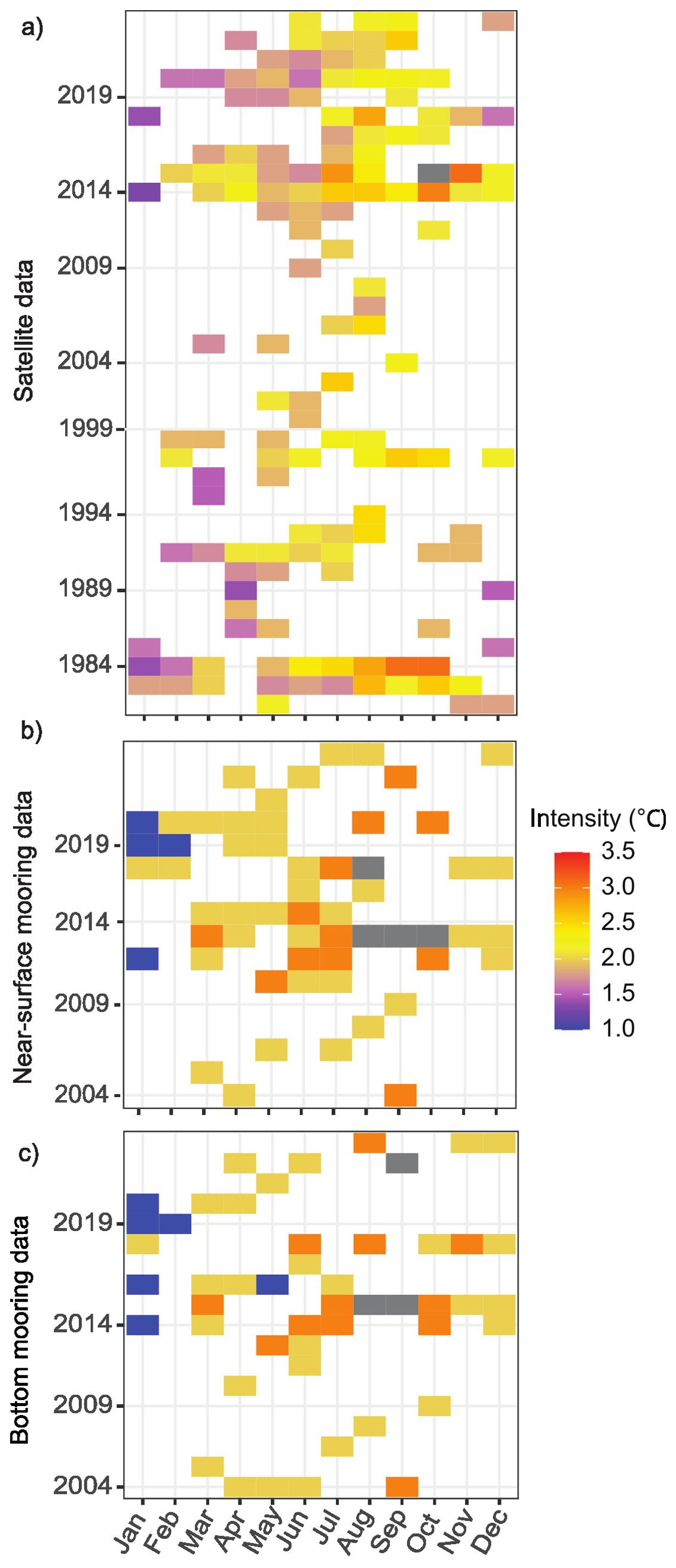
Figure 3. Monthly patterns of mean MHW intensity in Santa Barbara Channel derived from satellite data from 1983-2023 (A) and from coastal moorings at five sites near-surface (B) and at the bottom (C) from 2004 and 2023.
There were significant positive trends in the number of events observed, their duration and cumulative intensity of MHWs between 2004 and 2023 (Sen’s slope = 0.11, 1.08, and 2.08; z ≥ 2.54; p ≤ 0.05 respectively). In contrast, there was no statistically significant trend in all the above-mentioned metrics during the time period between 1984 and 2003. Comparing these two time periods, 2004 to 2023 had more observed MHWs (692 vs. 646) that were on average more intense (mean intensity 2.04°C compared to 1.99°C) and had a slower rate of decline (0.23°C day-1 compared to 0.26°C day-1).
The first mode of the EOF analysis explained 93% of the variance in the total MHW days in this 40-year dataset. The largest peaks corresponded to the historically intense MHW events in 1983, 1999 and 2014-2015 (Figure 4B). 2015 was the most intense event recorded. Recapitulating the pattern of increasing number of events in the past decade, there were more peaks with normalized values over 0.1 since 2012.
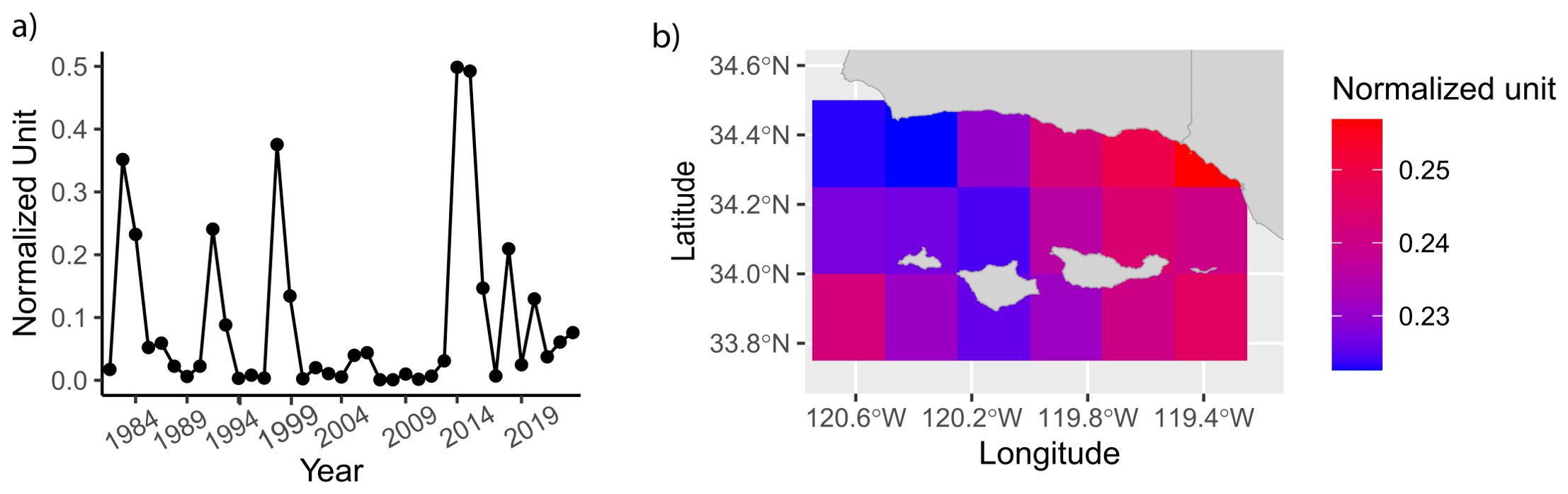
Figure 4. The dominant patterns of the Santa Barbara Channel total MHW days from 1983 to 2023. The spatial second mode (EOF1, A) and its corresponding temporal evolution (PC1, B). Both metrics are shown in normalized variance units.
The observed spatial variation was much smaller than the temporal variation (Figure 4A). The difference in normalized units differed by 0.03 between sites compared to 0.5 between years. There appeared to be an East-West pattern such that the sites receiving more open ocean influence on the West (closer to Point Conception) and the station on the south side of the Santa Rosa Island experienced relatively less MHW days compared to the islands due East.
3.2 Mooring data trends
Temperature derived from satellite data had a significant and close to 1:1 correlation with near-surface mooring data gathered at the 5 coastal sites (p <0.001, r2 = 0.9; Supplementary Figure 1), indicating compatible results from the two data sources. Every year, there were on average 2.7 MHW events detected by the surface and bottom mooring, respectively. These events were on average 14 days long and the mean average intensity was 2.2°C (Figures 2D-I). Similar to the channel-wide satellite data, MHW events were detected across all months of the year (Figures 3B, C). There were more MHW events recorded during spring and summer (~25 events per site compare to ~16 events in fall and winter). The events in fall were also the longest (35 days).
Given there were only 20 years of mooring data, the trend in intensity and duration of MHW events observed were analyzed as a single group. The Sens’s slope of the number of events, duration, and cumulative intensity had positive slopes for both observations near surface and at the bottom, although these trends were not statistically significant. However, it is important to note that the site-average monthly temperature from 2014 to 2023 appeared to be higher than those recorded for the period 2004 and 2013 (Figures 5A, B).
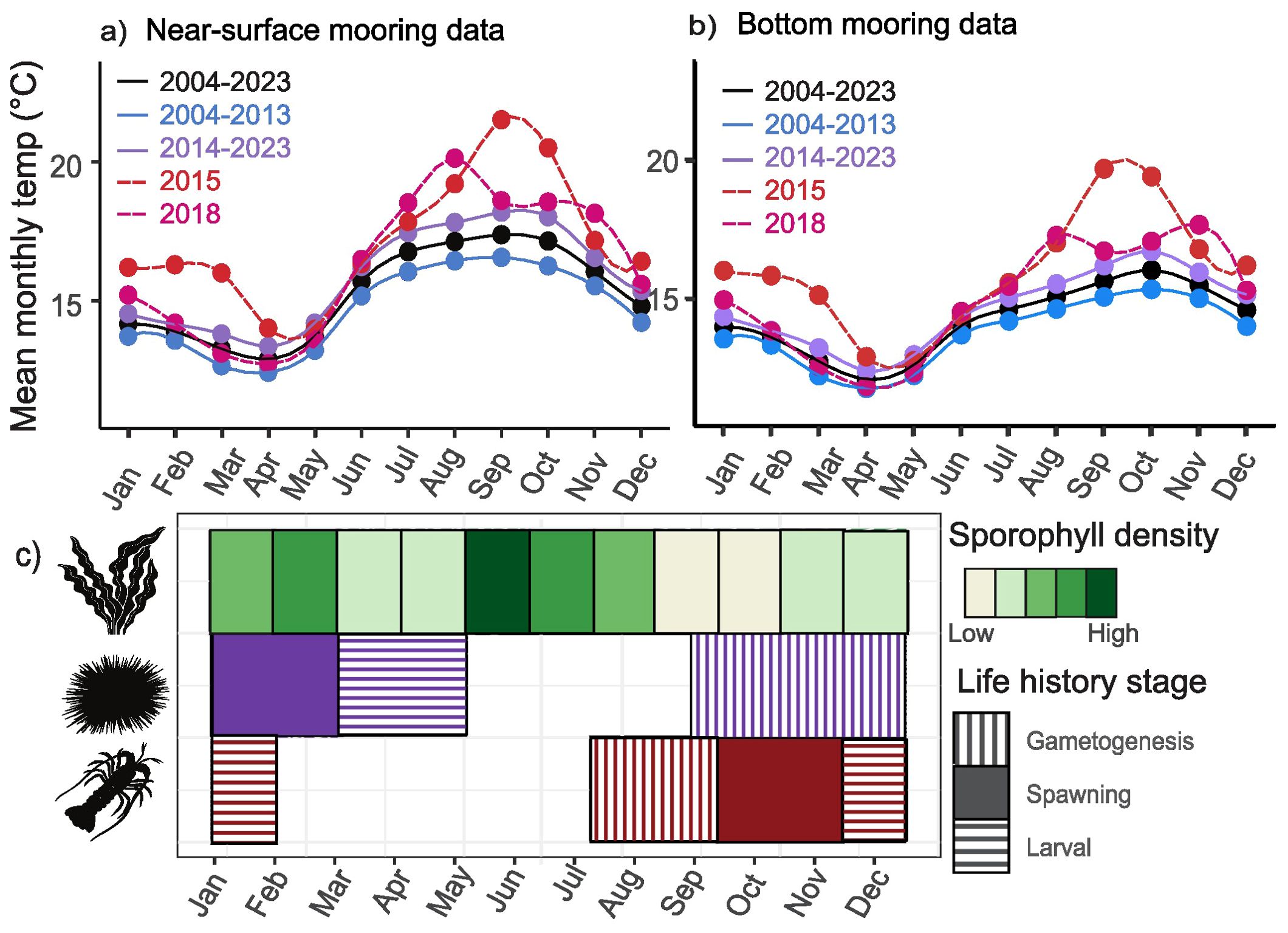
Figure 5. Mean monthly sea surface temperature across from the near-surface (A) and bottom (B) moorings of the SBC LTER coastal sites from 2004 to 2023. Relative abundance of sporophyll (reproductive part) of giant kelp (Macrocystis pyrifera), and key life history events of purple urchins (Strongylocentrotus purpuratus), and California spiny lobsters (Panulirus interruptus) in a year (C). All icons were obtained from theNounProject.com.
Furthermore, there was not complete concurrence when a MHW event occurred near the surface and when seawater temperature was anomalously high on the benthos (Figures 2D-I; Figures 3B, C). On average, approximately one quarter (27%, 62 of 229 events) of the MHW events detected near the surface did not have a corresponding bottom marine heatwave (BMHW) event. In addition, approximately one fifth (21%, 49 of 229 events) of BMHW events did not have a corresponding MHW signal near the surface. When there were paired events, i.e., a MHW was detected both near the surface and at the bottom, the events initiated first at the bottom, leading the surface signal by about 1 day. Of all top- or bottom-only MHW events detected (180 for top, 166 for bottom), the average duration (12.6 and 13.1 days, respectively) and intensity (2.28 and 2.24°C, respectively) were comparable between top and bottom. When compared to top events, bottom events had a faster rate of onset (top = 0.28°C day-1, bottom = 0.36°C day-1, respectively) and a slower rate of decline (top = 0.61°C day-1, bottom = 0.47°C day-1).
There appears to be an East-West pattern along these five coastal sites (Figures 2D-I), such that the easternmost site (car, Carpinteria) had a higher yearly average number of events (3.1 near surface events and 2.6 at bottom events) compared to the westernmost site at Alegria Reef (ale, 2.5 near surface events and 2.6 at bottom events).
4 Discussion
Using satellite and in situ monitoring data, we catalogued the spatial and temporal pattern of marine heatwaves in a biodiverse coastal habitat — the kelp forest community in the Santa Barbara Channel. Consistent with the global pattern, the Channel experienced an increase in frequency and intensity of MHWs over the last four decades. MHW events were recorded in all months of the year such that regardless of phenology, marine organisms in the region’s kelp forests could encounter thermal anomalies consistent with MHW events. Subsurface MHWs were detected; our high frequency coastal monitoring showed that MHW events could be depth stratified such that there was no concordance in anomalously high temperature between the surface and benthos. On a basin or regional scale, vertical structuring in the MHW signals, combined with spatial variation in total MHW days between the northern and southern sides of a large island, suggested the potential for short-lived refuges from MHW-related ocean warming.
4.1 Increasing MHW events
The frequency and intensity of MHWs are rising across the globe (Scannell et al., 2016). This increasing trend has been attributed to anthropogenic ocean warming (Oliver, 2019; Laufkötter et al., 2020) and changes in atmospheric processes (Barkhordarian et al., 2022). While our data sets were not particularly long (ca. 40 years for the satellite and 20 years for coastal in situ monitoring), we observed significant positive trends in number of events, duration, and intensity. Persistent and intense MHWs, like the ones observed in 2014-2016 in the Santa Barbara Channel, strongly correlated with variability in winter SST, namely the Pacific Decadal Oscillation (PDO), and the North Pacific Gyre Oscillation (NPGO; (Joh and Di Lorenzo, 2017). Shorter time scale local dynamics such as wind relaxation and the subsequent weakening of upwelling (Wei et al., 2021) and cloudiness (Myers et al., 2018) also contributed towards intense, long-lasting MHWs. With the predicted increase in NPGO-PDO coupling (Joh and Di Lorenzo, 2017), MHW events are likely to become more frequent, intense, and persistent in the Santa Barbara Channel in the next decade.
4.2 MHW coinciding with multiple key life history events
We observed more MHW events in spring and summer, and more persistent and intense events were observed in fall. It is, however, important to note that there were MHW events in winter as well. Similar seasonal variation in MHW occurrence were reported in other ocean basins (Thoral et al., 2022; Wang et al., 2024). For giant kelp that release zoospores year round (Reed et al., 1997), their reproductive success can be influenced by MHWs throughout the year. Furthermore, this seasonal variation in MHW events could coincide with key life history events of kelp forest organisms, including, purple urchin (Chatlynne, 1969; Pearse, 1980), and spiny lobsters (Barsky, 2008). This phenological alignment is illustrated in Figure 5C, where life history data were gleaned from the literature. For example, planktonic larval stages are often considered the most vulnerable phase of the multi-phasic life histories of marine organisms. Various economically and ecologically important species in the Southern California Bight spawn or perform gametogenesis during the spring and summer, including anchovies (Picquelle and Hewitt, 1983), sardines (Alheit, 1993), market squids (Zeidberg et al., 2012), spiny lobsters (Engle, 1979) and sea urchins (Ebert et al., 1994; Teck et al., 2018). Indeed, during the persistent 2014-2016 MHW event, the catch of pollock larvae was the lowest of the 30-year record along the Californian coast (Rogers et al., 2021). Recruitment failure after MHWs were also reported in rabbitfish (Siganus sp.) along the west coast of Australia (Lenanton et al., 2017) and oysters (Crassostrea gigas) in Mediterranean coastal lagoons (Correia-Martins et al., 2022).
Given MHWs occur year round, even if the most sensitive stage is not directly exposed to the thermal stress of a MHW, there is a potential for a legacy effect. Legacy effect refers to the experience in one life history stage affecting the subsequent stage (Padilla and Miner, 2006). Leach et al. (2021) showed that for purple sea urchins (Strongylocentrotus purpuratus), exposure to prolonged warming (28 days) in a simulated MHW during gametogenesis reduced male gamete performance such that fewer of their offspring developed successfully. Maternal effects on progeny traits have also been observed in purple urchins (Chamorro et al., 2023). Further experiments demonstrated that matching parental and larval experiences confer a higher thermal tolerance (Leach and Hofmann, 2023). Similarly, larval exposure to a simulated MHW was demonstrated to reduce juvenile performance by narrowing their thermal tolerance in an Australian urchin (Gall et al., 2021). These lab-based observations appeared to match field observations, such that during the 2013-2016 persistent MHW events, purple urchin recruitment remained high in the Santa Barbara Channel only to decline in the following year (Okamoto et al., 2020). This field observation highlights that the impact of MHWs may not be immediate. One reasonable inference is that adults exposed to a MHW produced lower quality gametes, resulting in lower performing larvae that have higher mortality and lower recruitment, i.e., legacy effects. Therefore, in addition to thermal niche and physiology (Harvey et al., 2022), the phenology of a given species relative to the timing of MHWs, which can be inferred from coastal monitoring similar to that presented here, plays vital roles in shaping its response to thermal stress.
4.3 Spatial variations as potential refuge for mobile species
While others have demonstrated the presence of subsurface MHWs at the continental slope (Amaya et al., 2023b; Zhang et al., 2023), we showed that the lack of concordance between surface and bottom could occur even in relatively shallow waters (<15 m). Such vertical dynamics in MHWs could be associated with several physical drivers including wind and cloud cover, coastal trapped waves and suppression of upwelling due to reduced mixing (Chen et al., 2021; Amaya et al., 2023b). Mobile species may be able to navigate the water column to avoid warmer waters when the MHWs do not extend throughout the water column. Fishes and even marine invertebrate larvae can respond to temperature by alternating their swimming behaviors (Matern et al., 2000; Gray and Kingsford, 2003; Chan and Grünbaum, 2010). Indeed, Fredston et al. (2023) showed that demersal fish were little affected by MHWs. However, subsurface MHWs could exert significant, previously underestimated pressure on benthic marine ecosystems (Amaya et al., 2023b), particularly on species with sedentary or sessile lifestyles such as kelp (Cavanaugh et al., 2019). Differential impacts of subsurface MHWs on sedentary and mobile species may lead to shifts in ecological interactions and community structures and warrant further analysis (Correia-Martins et al., 2022; Michaud et al., 2022; Panzeri et al., 2024).
The East-West difference in current velocity in the Santa Barbara Channel coincided with the spatial variations of the EOF of total MHW days and the enticing trend along the coast. A cold plume extended westward from Pt. Conception towards the Channel Islands, resulting in the relatively cold water towards the south of the islands. This pattern might also explain the relatively lower variance in EOF of total MHW days observed near Santa Rosa Island. This observed East-West spatial variation in MHW days suggests potential between-sites differences in stress exposure, including temperature anomalies and the associated change in nutrient availability (Landry et al., 2024). Starko et al. (2022) showed that warm microclimates increased the risk of habitat loss (kelp mortality) during MHWs. Interestingly, the area associated with stronger total MHW days in our record coincided with the area of slower recovery observed through Landsat imagery (Bell et al., 2023). Recent satellite-based analysis of bull kelp cover in Northern California (Cavanaugh et al., 2023) and airborne surveys of coral reef in Hawaii (Asner et al., 2022) identified pockets of resilience to MHWs. The elevated resilience to thermal stress could be a result of local genetic variations (Becheler et al., 2022) and other mediating factors, including other stressors and hydrodynamics. Identifying these refuges and their effectiveness as potential sources to reseed kelp communities in the future requires consistent long-term, community monitoring (Weitzman et al., 2021; Michaud et al., 2022).
5 Conclusion
In situ mooring data revealed vertical dynamics in MHWs, suggesting SST alone is not sufficient for estimating the risk of MHWs. Instead, long-term monitoring throughout the whole water column combined with ecological surveys is essential for understanding how coastal communities would persist in the future ocean, and how further ocean warming will impact giant kelp as a foundation species (Smith et al., 2024). Our analysis of satellite records and long-term coastal monitoring data showed that the sentinel kelp forest ecosystem in the Santa Barbara Channel, like other marine ecosystems, are experiencing an increasing frequency, intensity, and duration of MHWs. Such an increase in MHW events poses a significant threat to marine biodiversity and the socioeconomic resources from coastal environments (Smith et al., 2021, 2023).
Data availability statement
Publicly available datasets were analyzed in this study. This data can be found here: 1.doi.org/10.6073/pasta/e930954949b2635928b8be6824630f84 2.doi.org/10.6073/pasta/71ba36812add71bee887e24e23dcbbc1 3.doi.org/10.6073/pasta/440705c4b1bc634f6d08c130294748b0 4.doi.org/10.6073/pasta/7bd8c3dd431424d79bd47118f708b462 5.doi.org/10.6073/pasta/8e322b55d75ea55e8a89b94cb3cb6a05 6.doi.org/10.6073/pasta/98bacce98ca7299f6e3d0420d46980a6.
Author contributions
KC: Conceptualization, Formal Analysis, Funding acquisition, Investigation, Methodology, Visualization, Writing – original draft, Writing – review & editing. LK: Data curation, Formal Analysis, Investigation, Methodology, Resources, Software, Visualization, Writing – review & editing. AM: Formal Analysis, Visualization, Writing – review & editing. AR: Conceptualization, Data curation, Methodology, Visualization, Writing – review & editing. GH: Conceptualization, Funding acquisition, Methodology, Project administration, Resources, Supervision, Writing – original draft, Writing – review & editing.
Funding
The author(s) declare that financial support was received for the research, authorship, and/or publication of this article. This work was supported by awards from the U.S. National Science Foundation (NSF): an award to the SBC-LTER project (OCE-1831937) and NSF award 2131283 to GH. KC was supported by an ROA supplemental award to NSF 2131283.
Acknowledgments
We thank the SBC LTER team for maintaining the oceanographic sensors and moorings that make the collection of these time series data possible.
Conflict of interest
The authors declare that the research was conducted in the absence of any commercial or financial relationships that could be construed as a potential conflict of interest.
Publisher’s note
All claims expressed in this article are solely those of the authors and do not necessarily represent those of their affiliated organizations, or those of the publisher, the editors and the reviewers. Any product that may be evaluated in this article, or claim that may be made by its manufacturer, is not guaranteed or endorsed by the publisher.
Supplementary material
The Supplementary Material for this article can be found online at: https://www.frontiersin.org/articles/10.3389/fmars.2024.1476542/full#supplementary-material
References
Alheit J. (1993). Use of the daily egg production method for estimating biomass of clupeoid fishes: a review and evaluation. Bull. Mar. Sci. 53, 750–767. doi: 10.1126/sciadv.adf0954
Amaya D., Jacox M. G., Fewings M. R., Saba V. S., Stuecker M. F., Rykaczewski R. R., et al. (2023a). Marine heatwaves need clear definitions so coastal communities can adapt. Nature 616, 29–32. doi: 10.1038/d41586-023-00924-2
Amaya D. J., Jacox M. G., Alexander M. A., Scott J. D., Deser C., Capotondi A., et al. (2023b). Bottom marine heatwaves along the continental shelves of North America. Nat. Commun. 14, 1038. doi: 10.1038/s41467-023-36567-0
Asner G. P., Vaughn N. R., Martin R. E., Foo S. A., Heckler J., Neilson B. J., et al. (2022). Mapped coral mortality and refugia in an archipelago-scale marine heat wave. Proc. Natl. Acad. Sci. 119, e2123331119. doi: 10.1073/pnas.2123331119
Barkhordarian A., Nielsen D. M., Baehr J. (2022). Recent marine heatwaves in the North Pacific warming pool can be attributed to rising atmospheric levels of greenhouse gases. Commun. Earth Environ. 3, 131. doi: 10.1038/s43247-022-00461-2
Barsky K. (2008). Status of the fisheries report-an update through 2006. California: Department of Fish and Game Marine Region.
Becheler R., Haverbeck D., Clerc C., Montecinos G., Valero M., Mansilla A., et al. (2022). Variation in thermal tolerance of the giant kelp’s gametophytes: Suitability of habitat, population quality or local adaptation? Front. Mar. Sci. 9, 802535. doi: 10.3389/fmars.2022.802535
Bell T. W., Cavanaugh K. C., Saccomanno V. R., Cavanaugh K. C., Houskeeper H. F., Eddy N., et al. (2023). Kelpwatch: A new visualization and analysis tool to explore kelp canopy dynamics reveals variable response to and recovery from marine heatwaves. PloS One 18, e0271477. doi: 10.1371/journal.pone.0271477
Cavanaugh K. C., Cavanaugh K. C., Pawlak C. C., Bell T. W., Saccomanno V. R. (2023). CubeSats show persistence of bull kelp refugia amidst a regional collapse in California. Remote Sens. Environ. 290, 113521. doi: 10.1016/j.rse.2023.113521
Cavanaugh K. C., Reed D. C., Bell T. W., Castorani M. C., Beas-Luna R. (2019). Spatial variability in the resistance and resilience of giant kelp in southern and Baja California to a multiyear heatwave. Front. Mar. Sci. 6, 413. doi: 10.3389/fmars.2019.00413
Chamorro J. D., McDonald A. M., Hofmann G. E. (2023). Transgenerational plasticity as a mechanism of response to marine heatwaves in the purple sea urchin, Strongylocentrotus purpuratus. Front. Mar. Sci. 10, 1212781. doi: 10.3389/fmars.2023.1212781
Chan K. Y. K., Grünbaum D. (2010). Temperature and diet modified swimming behaviors of larval sand dollar. Mar. Ecol. Prog. Ser. 415, 49–59. doi: 10.3354/meps08744
Chatlynne L. G. (1969). A histochemical study of oogenesis in the sea urchin, Strongylocentrotus purpuratus. Biol. Bull. 136, 167–184. doi: 10.2307/1539811
Chen Z., Shi J., Liu Q., Chen H., Li C. (2021). A persistent and intense marine heatwave in the Northeast Pacific during 2019–2020. Geophysical Res. Lett. 48, e2021GL093239. doi: 10.1029/2021GL093239
Clare X. S., Kui L., Hofmann G. E. (2022). Larval thermal tolerance of Kellet’s whelk (Kelletia kelletii) as a window into the resilience of a wild shellfishery to marine heatwaves. J. Shellfish Res. 41, 283–290, 288. doi: 10.2983/035.041.0214
Correia-Martins A., Tremblay R., Bec B., Roques C., Atteia A., Gobet A., et al. (2022). Failure of bivalve foundation species recruitment related to trophic changes during an extreme heatwave event. Mar. Ecol. Prog. Ser. 691, 69–82. doi: 10.3354/meps14060
Couch C. S., Burns J. H., Liu G., Steward K., Gutlay T. N., Kenyon J., et al. (2017). Mass coral bleaching due to unprecedented marine heatwave in Papahānaumokuākea Marine National Monument (Northwestern Hawaiian Islands). PloS One 12, e0185121. doi: 10.1371/journal.pone.0185121
Csik S. R., DiFiore B. P., Kraskura K., Hardison E. A., Curtis J. S., Eliason E. J., et al. (2023). The metabolic underpinnings of temperature-dependent predation in a key marine predator. Front. Mar. Sci. 10. doi: 10.3389/fmars.2023.1072807
Dalsin M., Walter R. K., Mazzini P. L. (2023). Effects of basin-scale climate modes and upwelling on nearshore marine heatwaves and cold spells in the California Current. Sci. Rep. 13, 12389. doi: 10.1038/s41598-023-39193-4
Ebert T. A., Schroeter S. C., Dixon J. D., Kalvass P. (1994). Settlement patterns of red and purple sea urchins (Strongylocentrotus franciscanus and S. purpuratus) in California, USA. Mar. Ecol. Prog. Ser. 111, 41–52. doi: 10.3354/meps111041
Engle J. M. (1979). Ecology and growth of juvenile California spiny lobster, Panulirus interruptus (Randall) (Los Angeles, California: University of Southern California).
Filbee-Dexter K., Wernberg T., Grace S. P., Thormar J., Fredriksen S., Narvaez C. N., et al. (2020). Marine heatwaves and the collapse of marginal North Atlantic kelp forests. Sci. Rep. 10, 13388. doi: 10.1038/s41598-020-70273-x
Fredston A. L., Cheung W. W., Frölicher T. L., Kitchel Z. J., Maureaud A. A., Thorson J. T., et al. (2023). Marine heatwaves are not a dominant driver of change in demersal fishes. Nature 621, 324–329. doi: 10.1038/s41586-023-06449-y
Gall M. L., Holmes S. P., Campbell H., Byrne M. (2021). Effects of marine heatwave conditions across the metamorphic transition to the juvenile sea urchin (Heliocidaris erythrogramma). Mar. pollut. Bull. 163, 111914. doi: 10.1016/j.marpolbul.2020.111914
Gaspar T. L., Quimbayo J. P., Ozekoski R., Nunes L. T., Aued A. W., Mendes T. C., et al. (2021). Severe coral bleaching of Siderastrea stellata at the only atoll in the South Atlantic driven by sequential Marine Heatwaves. Biota Neotropica 21, e20201131. doi: 10.1590/1676-0611-bn-2020-1131
Gray C. A., Kingsford M. J. (2003). Variability in thermocline depth and strength, and relationships with vertical distributions of fish larvae and mesozooplankton in dynamic coastal waters. Mar. Ecol. Prog. Ser. 247, 211–224. doi: 10.3354/meps247211
Guo X., Gao Y., Zhang S., Wu L., Chang P., Cai W., et al. (2022). Threat by marine heatwaves to adaptive large marine ecosystems in an eddy-resolving model. Nat. Climate Change 12, 179–186. doi: 10.1038/s41558-021-01266-5
Harvey B. P., Marshall K. E., Harley C. D., Russell B. D. (2022). Predicting responses to marine heatwaves using functional traits. Trends Ecol. Evol. 37, 20–29. doi: 10.1016/j.tree.2021.09.003
Hobday A. J., Alexander L. V., Perkins S. E., Smale D. A., Straub S. C., Oliver E. C., et al. (2016). A hierarchical approach to defining marine heatwaves. Prog. Oceanography 141, 227–238. doi: 10.1016/j.pocean.2015.12.014
Hobday A. J., Oliver E. C., Gupta A. S., Benthuysen J. A., Burrows M. T., Donat M. G., et al. (2018). Categorizing and naming marine heatwaves. Oceanography 31, 162–173. doi: 10.5670/oceanog.2018.205
Joh Y., Di Lorenzo E. (2017). Increasing coupling between NPGO and PDO leads to prolonged marine heatwaves in the Northeast Pacific. Geophysical Res. Lett. 44, 11,663–611,671. doi: 10.1002/2017GL075930
Joyce P. W., Tong C. B., Yip Y. L., Falkenberg L. J. (2024). Marine heatwaves as drivers of biological and ecological change: implications of current research patterns and future opportunities. Mar. Biol. 171, 20. doi: 10.1007/s00227-023-04340-y
Kozal L. C., Nelson J. C., Hofmann G. E. (2024). Kelp-associated variability in seawater chemistry during a marine heatwave event connects to transgenerational effects in the purple urchin Strongylocentrotus purpuratus. Mar. Ecol. Prog. Ser. 733, 59–77. doi: 10.3354/meps14523
Kui L. (2024). Daily sea surface temperature in Santa Barbara channel between 1982 and 2023 ver 3. Environmental Data Initiative. doi: 10.6073/pasta/e930954949b2635928b8be6824630f84
Landry M. R., Freibott A. L., Beatty J. L., Selph K. E. (2024). Phytoplankton biomass responses to a marine heat wave align with altered nitracline depth. Limnology Oceanography 69, 1683–1694. doi: 10.1002/lno.12624
Laufkötter C., Zscheischler J., Frölicher T. L. (2020). High-impact marine heatwaves attributable to human-induced global warming. Science 369, 1621–1625. doi: 10.1126/science.aba0690
Laurel B. J., Hunsicker M. E., Ciannelli L., Hurst T. P., Duffy-Anderson J., O’Malley R., et al. (2021). Regional warming exacerbates match/mismatch vulnerability for cod larvae in Alaska. Prog. Oceanography 193, 102555. doi: 10.1016/j.pocean.2021.102555
Leach T. S., Hofmann G. E. (2023). Marine heatwave temperatures enhance larval performance but are meditated by paternal thermal history and inter-individual differences in the purple sea urchin, Strongylocentrotus purpuratus. Front. Physiol. 14. doi: 10.3389/fphys.2023.1230590
Leach T. S., BuyanUrt B., Hofmann G. E. (2021). Exploring impacts of marine heatwaves: paternal heat exposure diminishes fertilization success in the purple sea urchin (Strongylocentrotus purpuratus). Mar. Biol. 168, 103. doi: 10.1007/s00227-021-03915-x
Leggat W. P., Camp E. F., Suggett D. J., Heron S. F., Fordyce A. J., Gardner S., et al. (2019). Rapid coral decay is associated with marine heatwave mortality events on reefs. Curr. Biol. 29, 2723–2730.e2724. doi: 10.1016/j.cub.2019.06.077
Lenanton R. C. J., Dowling C. E., Smith K. A., Fairclough D. V., Jackson G. (2017). Potential influence of a marine heatwave on range extensions of tropical fishes in the eastern Indian Ocean—Invaluable contributions from amateur observers. Regional Stud. Mar. Sci. 13, 19–31. doi: 10.1016/j.rsma.2017.03.005
Le Nohaïc M., Ross C. L., Cornwall C. E., Comeau S., Lowe R., McCulloch M. T., et al. (2017). Marine heatwave causes unprecedented regional mass bleaching of thermally resistant corals in northwestern Australia. Sci. Rep. 7, 1–11. doi: 10.1038/s41598-017-14794-y
Lowman H. E., Emery K. A., Dugan J. E., Miller R. J. (2022). Nutritional quality of giant kelp declines due to warming ocean temperatures. Oikos 2022, e08619. doi: 10.1111/oik.08619
Matern S. A., Cech J. J., Hopkins T. E. (2000). Diel movements of bat rays, Myliobatis californica, in Tomales Bay, California: evidence for behavioral thermoregulation? Environ. Biol. Fishes 58, 173–182. doi: 10.1023/A:1007625212099
McPherson M. L., Finger D. J. I., Houskeeper H. F., Bell T. W., Carr M. H., Rogers-Bennett L., et al. (2021). Large-scale shift in the structure of a kelp forest ecosystem co-occurs with an epizootic and marine heatwave. Commun. Biol. 4, 298. doi: 10.1038/s42003-021-01827-6
Michaud K. M., Reed D. C., Miller R. J. (2022). The Blob marine heatwave transforms California kelp forest ecosystems. Commun. Biol. 5, 1143. doi: 10.1038/s42003-022-04107-z
Myers T. A., Mechoso C. R., Cesana G. V., DeFlorio M. J., Waliser D. E. (2018). Cloud feedback key to marine heatwave off Baja California. Geophysical Res. Lett. 45, 4345–4352. doi: 10.1029/2018GL078242
Okamoto D. K., Schroeter S. C., Reed D. C. (2020). Effects of ocean climate on spatiotemporal variation in sea urchin settlement and recruitment. Limnology Oceanography 65, 2076–2091. doi: 10.1002/lno.11440
Oliver E. C. (2019). Mean warming not variability drives marine heatwave trends. Climate Dynamics 53, 1653–1659. doi: 10.1007/s00382-019-04707-2
Oliver E. C., Donat M. G., Burrows M. T., Moore P. J., Smale D. A., Alexander L. V., et al. (2018). Longer and more frequent marine heatwaves over the past century. Nat. Commun. 9, 1–12. doi: 10.1038/s41467-018-03732-9
Padilla D. K., Miner B. G. (2006). Legacies in life histories. Integr. Comp. Biol. 46, 217–223. doi: 10.1093/icb/icj029
Panzeri D., Reale M., Cossarini G., Salon S., Carlucci R., Spedicato M. T., et al. (2024). Future distribution of demersal species in a warming Mediterranean sub-basin. Front. Mar. Sci. 11, 1308325. doi: 10.3389/fmars.2024.1308325
Pearse J. S. (1981). Synchronization of gametogenesis in the sea urchins Strongylocentrotus purpuratus and S. franciscanus. In: Adams TS, Clark WH Jr (eds) Recent advances in invertebrate reproduction. North Holland, Amsterdam: Elsevier, p 53−68.
Peruzza L., Tucci C. F., Frizzo R., Riello T., Quagliariello A., Martino M. E., et al. (2023). Impaired reproduction, energy reserves and dysbiosis: The overlooked consequences of heatwaves in a bivalve mollusc. Mar. pollut. Bull. 193, 115192. doi: 10.1016/j.marpolbul.2023.115192
Picquelle S. J., Hewitt R. P. (1983). “The northern anchovy spawning biomass for the 1982-83 California fishing season,” in California Cooperative Oceanic Fisheries Investigations Reports.
Reed D. C., Anderson T. W., Ebeling A. W., Anghera M. (1997). The role of reproductive synchrony in the colonization potential of kelp. Ecology 78, 2443–2457. doi: 10.1890/0012-9658(1997)078[2443:TRORSI]2.0.CO;2
Reed D., Washburn L., Rassweiler A., Miller R., Bell T., Harrer S. (2016). Extreme warming challenges sentinel status of kelp forests as indicators of climate change. Nat. Commun. 7, 13757. doi: 10.1038/ncomms13757
Rogers L. A., Wilson M. T., Duffy-Anderson J. T., Kimmel D. G., Lamb J. F. (2021). Pollock and “the Blob”: Impacts of a marine heatwave on walleye pollock early life stages. Fisheries Oceanography 30, 142–158. doi: 10.1111/fog.12508
Rogers-Bennett L., Catton C. A. (2019). Marine heat wave and multiple stressors tip bull kelp forest to sea urchin barrens. Sci. Rep. 9, 15050. doi: 10.1038/s41598-019-51114-y
Sanford E., Sones J. L., García-Reyes M., Goddard J. H. R., Largier J. L. (2019). Widespread shifts in the coastal biota of northern California during the 2014–2016 marine heatwaves. Sci. Rep. 9, 4216. doi: 10.1038/s41598-019-40784-3
Scannell H. A., Pershing A. J., Alexander M. A., Thomas A. C., Mills K. E. (2016). Frequency of marine heatwaves in the North Atlantic and North Pacific since 1950. Geophysical Res. Lett. 43, 2069–2076. doi: 10.1002/2015GL067308
Schlegel R. W., Smit A. J. (2018). heatwaveR: A central algorithm for the detection of heatwaves and cold-spells. J. Open Source Software 3, 821. doi: 10.21105/joss.00821
Sen P. K. (1968). Estimates of the regression coefficient based on Kendall’s tau. J. Am. Stat. Assoc. 63, 1379–1389. doi: 10.1080/01621459.1968.10480934
Shanks A. L., Rasmuson L. K., Valley J. R., Jarvis M. A., Salant C., Sutherland D. A., et al. (2020). Marine heat waves, climate change, and failed spawning by coastal invertebrates. Limnology Oceanography 65, 627–636. doi: 10.1002/lno.11331
Smale D. A. (2020). Impacts of ocean warming on kelp forest ecosystems. New Phytol. 225, 1447–1454. doi: 10.1111/nph.16107
Smith K. E., Aubin M., Burrows M. T., Filbee-Dexter K., Hobday A. J., Holbrook N. J., et al. (2024). Global impacts of marine heatwaves on coastal foundation species. Nat. Commun. 15, 5052. doi: 10.1038/s41467-024-49307-9
Smith K. E., Burrows M. T., Hobday A. J., King N. G., Moore P. J., Sen Gupta A., et al. (2023). Biological impacts of marine heatwaves. Annu. Rev. Mar. Sci. 15, 119–145. doi: 10.1146/annurev-marine-032122-121437
Smith K. E., Burrows M. T., Hobday A. J., Sen Gupta A., Moore P. J., Thomsen M., et al. (2021). Socioeconomic impacts of marine heatwaves: Global issues and opportunities. Science 374, eabj3593. doi: 10.1126/science.abj3593
Starko S., Fifer J. E., Claar D. C., Davies S. W., Cunning R., Baker A. C., et al. (2023). Marine heatwaves threaten cryptic coral diversity and erode associations among coevolving partners. Sci. Adv. 9, e, adf0954. doi: 10.1126/sciadv.adf0954
Starko S., Neufeld C. J., Gendall L., Timmer B., Campbell L., Yakimishyn J., et al. (2022). Microclimate predicts kelp forest extinction in the face of direct and indirect marine heatwave effects. Ecol. Appl. 32, e2673. doi: 10.1002/eap.2673
Stillman J. H. (2019). Heat waves, the new normal: Summertime temperature extremes will impact animals, ecosystems, and human communities. Physiology 34, 86–100. doi: 10.1152/physiol.00040.2018
Sun D., Li F., Jing Z., Hu S., Zhang B. (2023). Frequent marine heatwaves hidden below the surface of the global ocean. Nat. Geosci. 16, 1099–1104. doi: 10.1038/s41561-023-01325-w
Tait L. W., Thoral F., Pinkerton M. H., Thomsen M. S., Schiel D. R. (2021). Loss of giant kelp, Macrocystis pyrifera, driven by marine heatwaves and exacerbated by poor water clarity in New Zealand. Front. Mar. Sci. 8. doi: 10.3389/fmars.2021.721087
Teck S. J., Lorda J., Shears N. T., Ben-Horin T., Toseland R. E., Rathbone S. T., et al. (2018). Quality of a fished resource: Assessing spatial and temporal dynamics. PloS One 13, e0196864. doi: 10.1371/journal.pone.0196864
Thomsen M. S., Mondardini L., Alestra T., Gerrity S., Tait L., South P. M., et al. (2019). Local extinction of bull kelp (Durvillaea spp.) due to a marine heatwave. Front. Mar. Sci. 6. doi: 10.3389/fmars.2019.00084
Thoral F., Montie S., Thomsen M. S., Tait L. W., Pinkerton M. H., Schiel D. R. (2022). Unravelling seasonal trends in coastal marine heatwave metrics across global biogeographical realms. Sci. Rep. 12, 7740. doi: 10.1038/s41598-022-11908-z
Wang H., Lu Y., Zhai L., Chen X., Liu S. (2024). Variations of surface marine heatwaves in the Northwest Pacific during 1993–2019. Front. Mar. Sci. 11, 1323702. doi: 10.3389/fmars.2024.1323702
Washburn L., Gotschalk C., Salazar D. (2024a). SBC LTER: Ocean: Currents and Biogeochemistry: Moored CTD and ADCP data from Alegria Reef Mooring (ALE), 1999-2021 ver 15. Environmental Data Initiative. doi: 10.6073/pasta/71ba36812add71bee887e24e23dcbbc1
Washburn L., Gotschalk C., Salazar D. (2024b). SBC LTER: Ocean: Currents and Biogeochemistry: Moored CTD and ADCP data from Arroyo Quemada Reef Mooring (ARQ), ongoing since 2004 ver 27. Environmental Data Initiative. doi: 10.6073/pasta/440705c4b1bc634f6d08c130294748b0
Washburn L., Gotschalk C., Salazar D. (2024c). SBC LTER: Ocean: Currents and Biogeochemistry: Moored CTD and ADCP data from Carpinteria Reef Mooring (CAR, ongoing since 2001 ver 29. Environmental Data Initiative. doi: 10.6073/pasta/98bacce98ca7299f6e3d0420d46980a6
Washburn L., Gotschalk C., Salazar D. (2024d). SBC LTER: Ocean: Currents and Biogeochemistry: Moored CTD and ADCP data from Mohawk Outside Spar (MKO), ongoing since 2005 ver 19. Environmental Data Initiative. doi: 10.6073/pasta/8e322b55d75ea55e8a89b94cb3cb6a05
Washburn L., Gotschalk C., Salazar D. (2024e). SBC LTER: Ocean: Currents and Biogeochemistry: Moored CTD and ADCP data from Naples Reef Mooring (NAP), ongoing since 2001 ver 33. Environmental Data Initiative. doi: 10.6073/pasta/7bd8c3dd431424d79bd47118f708b462
Wei X., Li K. Y., Kilpatrick T., Wang M., Xie S. P. (2021). Large-scale conditions for the record-setting Southern California marine heatwave of August 2018. Geophysical Res. Lett. 48, e2020GL091803. doi: 10.1029/2020GL091803
Weitzman B., Konar B., Iken K., Coletti H., Monson D., Suryan R., et al. (2021). Changes in rocky intertidal community structure during a marine heatwave in the northern Gulf of Alaska. Front. Mar. Sci. 8, 556820. doi: 10.3389/fmars.2021.556820
Wernberg T. (2021). “Marine heatwave drives collapse of kelp fsorests in Western Australia,” in Ecosystem Collapse and Climate Change. Eds. Canadell J. G., Jackson R. B. (Springer International Publishing, Cham), 325–343.
Wild S., Krützen M., Rankin R. W., Hoppitt W. J., Gerber L., Allen S. J. (2019). Long-term decline in survival and reproduction of dolphins following a marine heatwave. Curr. Biol. 29, R239–R240. doi: 10.1016/j.cub.2019.02.047
Woodson C. B., Micheli F., Boch C., Al-Najjar M., Espinoza A., Hernandez A., et al. (2019). Harnessing marine microclimates for climate change adaptation and marine conservation. Conserv. Lett. 12, e12609. doi: 10.1111/conl.12609
Wyatt A. S., Leichter J. J., Washburn L., Kui L., Edmunds P. J., Burgess S. C. (2023). Hidden heatwaves and severe coral bleaching linked to mesoscale eddies and thermocline dynamics. Nat. Commun. 14, 25. doi: 10.1038/s41467-022-35550-5
Zeidberg L. D., Butler J. L., Ramon D., Cossio A., Stierhoff K. L., Henry A. (2012). Estimation of spawning habitats of market squid (Doryteuthis opalescens) from field surveys of eggs off Central and Southern California. Mar. Ecol. 33, 326–336. doi: 10.1111/j.1439-0485.2011.00498.x
Keywords: extreme events, climate change, LTER, climate variability, marine heatwave, kelp forest, ocean warming
Citation: Chan KYK, Kui L, McDonald AM, Ritger AL and Hofmann GE (2024) Coastal marine heatwaves in the Santa Barbara Channel: decadal trends and ecological implications. Front. Mar. Sci. 11:1476542. doi: 10.3389/fmars.2024.1476542
Received: 06 August 2024; Accepted: 11 November 2024;
Published: 16 December 2024.
Edited by:
Pauline Mary Ross, The University of Sydney, AustraliaReviewed by:
Jinlin Liu, Tongji University, ChinaChristopher Edward Cornwall, Victoria University of Wellington, New Zealand
Katie Fenton, Victoria University of Wellington, New Zealand in collaboration with reviewer CEC
Copyright © 2024 Chan, Kui, McDonald, Ritger and Hofmann. This is an open-access article distributed under the terms of the Creative Commons Attribution License (CC BY). The use, distribution or reproduction in other forums is permitted, provided the original author(s) and the copyright owner(s) are credited and that the original publication in this journal is cited, in accordance with accepted academic practice. No use, distribution or reproduction is permitted which does not comply with these terms.
*Correspondence: Kit Yu Karen Chan, a3ljaGFuQHV3LmVkdQ==