- 1State Key Laboratory of Marine Geology, Tongji University, Shanghai, China
- 2Project Management Office of China National Scientific Seafloor Observatory, Tongji University, Shanghai, China
Marine biological nitrogen fixation (BNF) is crucial for introducing “new nitrogen” into the oceans. Over the past 30 years, numerous laboratory and on-board culture experiments have been conducted studying the effects of nutrients such as total dissolved nitrogen (TDN), total dissolved phosphorus (TDP), and dissolved iron (DFe) on marine diazotrophs such as Braarudosphaera bigelowii (B. bigelowii), Trichodesmium, Crocosphaera and noncyanobacterial diazotrophs (NCDs). Most studies concluded that elevated dissolved inorganic nitrogen levels inhibit nitrogen fixation in Trichodesmium, promote its growth, and have minimal effect on B. bigelowii. The impact on NCDs is unclear. Moreover, elevated dissolved inorganic phosphorus (DIP) levels can promote individual growth, population growth, and nitrogen fixation in most diazotrophs in P-limited marine environments. Dissolved organic phosphorus is a potential phosphorous source for diazotrophs in low-DIP environments. Elevated DFe can promote population growth and nitrogen fixation in diazotrophs in Fe-limited marine environments. At present, most diazotrophs have yet to achieve pure culture. Moreover, the effect of nutrients on diazotrophs is mainly limited to the study of a single nutrient, which cannot accurately reflect the actual Marine environment where diazotrophs live. As a result, our understanding of the effect of nutrients on diazotrophs is still insufficient. Future research focusing on the issues above and the development of innovative technologies and methodologies to investigate the impact of marine BNF is highly recommended, which will allow for a more precise assessment of the impact of marine BNF on global primary productivity while providing a scientific foundation for rational evaluation of ocean CO2 uptake and emissions.
1 Introduction
Marine biological nitrogen fixation (BNF) is a microbially-mediated biogeochemical process involving the conversion of atmospheric N2 into bioavailable NH4+ by diazotrophs, thereby providing a continuous supply of “new nitrogen” to producers (Zehr and Capone, 2020; Hutchins and Capone, 2022). This process not only stimulates primary productivity but also enhances the ocean’s capacity to absorb CO2 from the atmosphere (Figure 1). Thus, marine BNF plays a crucial regulatory role in sustaining global primary productivity and driving assimilation and sequestration of carbon in the oceans (Zehr and Capone, 2020).
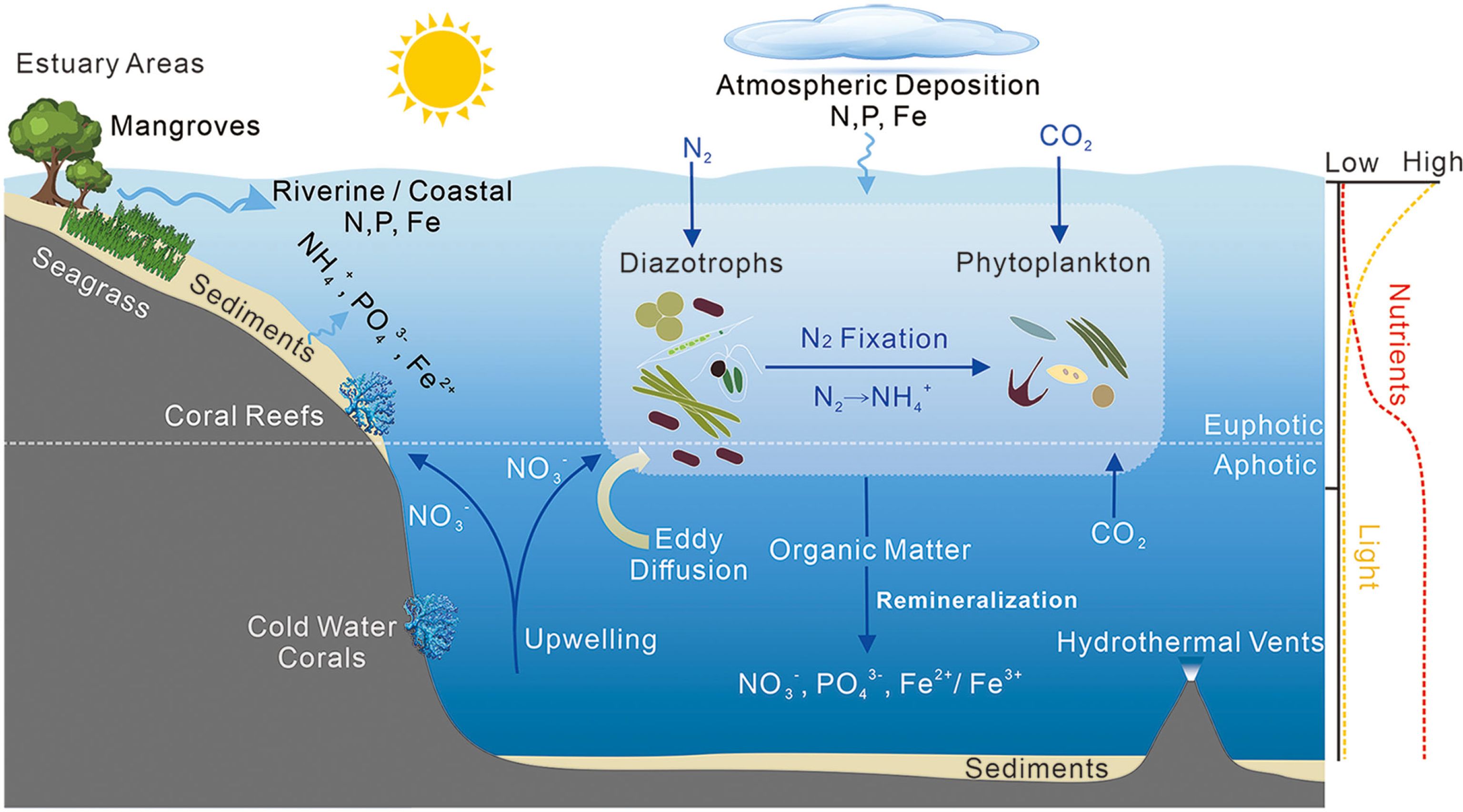
Figure 1. Diagram illustrating marine biological nitrogen fixation (BNF) and BNF-associated microbe habitat distribution. Key habitats include estuary areas, mangroves, sediments, seagrass, coral reefs, and hydrothermal vents (Zehr and Capone, 2020). For BNF, marine diazotrophs first absorb and metabolize atmospheric N2 before converting it to NH4+ via nitrogen fixation, thereby providing new nitrogen for phytoplankton (Sohm et al., 2011b). Several nutrients (including nitrogen [N], phosphorus [P], and iron [Fe]) play important roles in this process. Depth profiles (right) show typical light and nutrient distributions (Bristow et al., 2017). N2, nitrogen gas; CO2, carbon dioxide; NH4+, ammonium; NO3-, nitrate; PO43-, phosphate; Fe2+, ferrous ion; Fe3+, ferri ion.
Marine BNF is influenced by physical and chemical factors (Figure 1). Key physical factors that regulate BNF include temperature, light, and ocean current parameters (Zehr and Capone, 2021b). Ambient temperature impacts the enzyme kinetics and growth rates of diazotrophs, and light affects diazotroph photosynthesis and regulates the ratio of photosynthesis system I (PSI) to photosynthesis system II (PSII). Additionally, ocean currents can induce the mixing of water masses, affecting nutrient structure and diazotroph growth (Zehr and Capone, 2021b). Chemical factors affecting BNF include oxygen, salinity, pH, dissolved organic matter, and various nutrients. Diazotrophs are highly sensitive to oxygen due to the rapid inactivation of nitrogenase enzymes upon oxygen exposure (Zehr and Capone, 2021c). Changes in salinity can alter organismal osmotic pressure, subsequently affecting growth and acclimatization. pH can affect the activities of nitrogenase enzymes in diazotrophs, interfere with their metabolism, and thus affect their community structure (Zehr and Kudela, 2011). The metabolic activity of heterotrophic diazotrophs is closely linked to the presence of dissolved organic matter (Zehr and Capone, 2021b).
Nutrients play a critical role in the growth of marine diazotrophs. Cellular synthesis of various organic compounds, such as amino acids, proteins, cell membranes, nucleic acids, and nitrogenases, requires substantial amounts of specific nutrients or elements, including nitrogen (N), phosphorus (P), and iron (Fe) (Kusano et al., 2011). In the marine environment, N, P, and Fe are often the most essential primary nutrients that affect primary productivity due to their relative availability (Falkowski et al., 1998). However, nutrient distributions exhibit significant variations across different marine environments, and this spatial heterogeneity may contribute to differences in marine BNF (Falkowski et al., 1998; Hutchins and Tagliabue, 2024).
Long-term studies on dinitrogen (N2) fixation have mainly focused on oligotrophic marine regions, such as tropical and subtropical areas with very low dissolved inorganic nitrogen (DIN) concentration in surface water (Zehr and Capone, 2021a), the North Atlantic gyre area with very low dissolved inorganic phosphorus (DIP) content (Moore et al., 2009), and the North Pacific gyre region with low dissolved iron (DFe) (Yuan et al., 2023). However, recent research has also identified N2 fixation occurring in some eutrophic marine environments, including nearshore mangroves, upwelling areas, seagrass habitats, and large estuarine plumes (Zilius et al., 2020; Mohr et al., 2021; Kittu et al., 2023; Louchard et al., 2023) (Figure 1).
By comparing eutrophic and oligotrophic areas, researchers discovered that the nutrient structures and the main diazotroph groups differed (Zehr and Capone, 2024). For example, different diazotrophs respond differently to various nutrients due to their physiological differences, enabling them to fix N in various nutrient environments. Despite the comprehensive summaries of the physiological characteristics and N2 fixation mechanisms of diazotrophs (Zehr and Capone, 2020; 2024) as well as research on cellular interactions among diazotrophs (Thompson and Zehr, 2013; Eichner et al., 2023) and factors controlling BNF (Zehr and Capone, 2020) in current studies, there is still a lack of systematic review on the effects of different nutrients on these microorganisms.
Thus, this study presents a comprehensive review of research conducted over the past 30 years on the relationship between diazotrophs such as Braarudosphaera bigelowii (B. bigelowii), Trichodesmium, Crocosphaera, and noncyanobacterial diazotrophs (NCDs), as well as total dissolved nitrogen (TDN), total dissolved phosphorus (TDP), and DFe. This study aimed to summarize the response mechanisms of different marine diazotrophs to variations in nutrient abundance, providing a foundation for future research on the role of nutrients in marine BNF.
2 Marine diazotrophs
Research on marine diazotrophs commenced in the 1960s, with initial investigations focusing on Trichodesmium due to their microscopic visibility (Dugdale et al., 1961). However, the advent of molecular biology techniques has substantially broadened the exploration of marine nitrogen-fixing biodiversity. Recent research has classified marine diazotrophs into eukaryotic and prokaryotic diazotrophs (Figure 2). B. bigelowii is a eukaryotic diazotroph known for containing a nitrogen-fixing organelle—UCYN-A (Candidatus Atelocyanobacterium thalassa) (Coale et al., 2024). Furthermore, prokaryotic diazotrophs primarily include cyanobacterial and NCDs. The most common cyanobacterial diazotrophs include Trichodesmium, unicellular cyanobacteria, and diatom–diazotroph associations. Typical unicellular cyanobacteria comprise Crocosphaera and Cyanothece (Zehr and Capone, 2020; 2024). Currently, only B. bigelowii (Tschitschko et al., 2024), Trichodesmium (Webb et al., 2022), Crocosphaera (Masuda et al., 2024) have been isolated and purified, and some isolates from NCDs have been enriched and isolated (Farnelid et al., 2014; Rose et al., 2024); studies on the effects of nutrients on diazotrophs mainly focus on the above microorganisms.
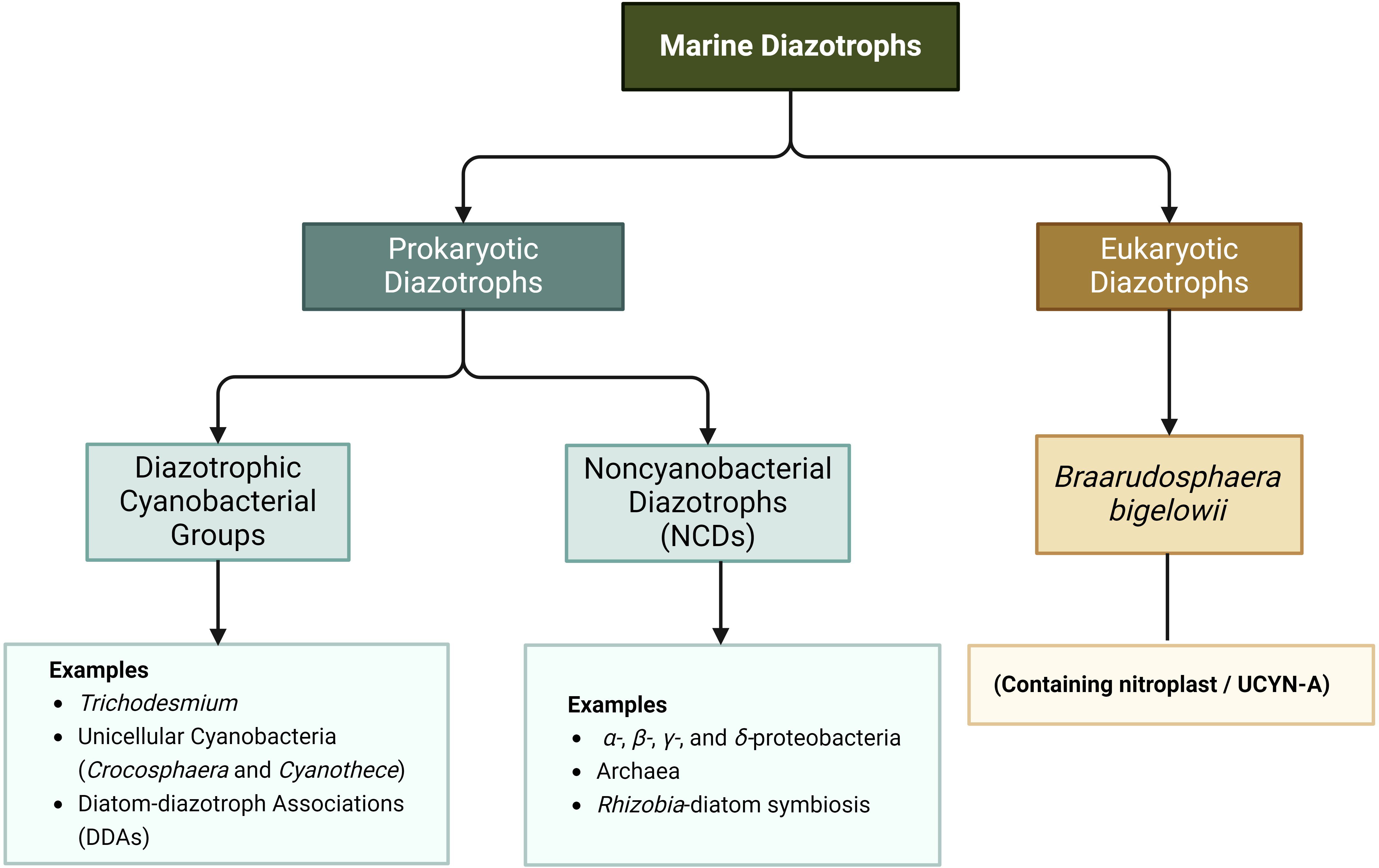
Figure 2. Classification of marine diazotrophs. Created in BioRender. Cao, H. (2024) BioRender.com/r84p523.
B. Bigelow is a eukaryotic diazotroph associated with the nitrogen-fixing organelle UCYN-A. UCYN-A exists in three sublines UCYN-A1, UCYN-A2, and UCYN-A3 (Figure 3A, B). The sublines can exhibit radius differences up to 10 times, and the volume differences can be as large as 1000 times (Thompson et al., 2014; Cornejo-Castillo et al., 2024). Early studies believed that B. Bigelow and UCYN-A exist in “endosymbiosis” because UCYN-A lacks common metabolic pathways such as PSII as well as the entire tricarboxylic acid cycle and remains unaffected by the amount of O2 produced by photosynthesis. Moreover, it primarily fixes nitrogen during the daytime (Thompson and Zehr, 2013; Zehr and Capone, 2020). Recent studies have demonstrated that B. bigelowii controls the division of UCYN-A, and several proteins present in UCYN-A are encoded only in the nucleus of B. bigelowii (Coale et al., 2024; Massana, 2024).
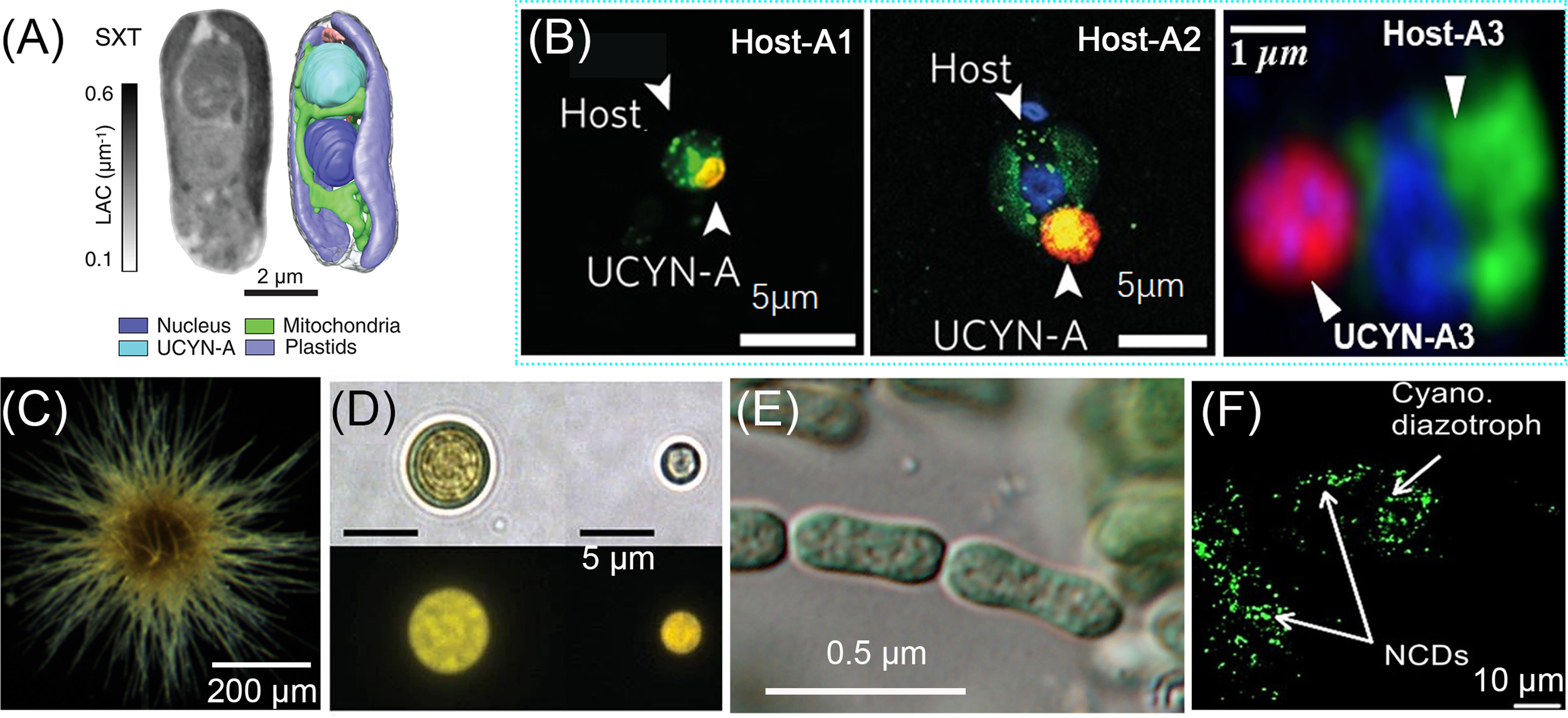
Figure 3. Typical marine diazotrophs. (A) Braarudosphaera bigelowii, orthoslice from SXT data, and colour-coded 3D renderings of subcellular components (Coale et al., 2024), scale bar 2 μm; (B) UCYN-A (including A1–A3) as determined using catalyzed reporter deposition-fluorescence in situ hybridization (CARD-FISH) (Martínez-Pérez et al., 2016; Cornejo-Castillo et al., 2019), scale bar 5 or 1 μm; (C) A Trichodesmium colony photographed in the East China Sea using continuous particle imaging and classification system, scale bar 200 μm; (D) UCYN-B Crocosphaera (Zehr and Capone, 2021d), scale bar 5 μm; (E) Crocosphaera subtropica (Cyanothece sp. ATCC 51142), with cells embedded in diffluent mucilage visible only by staining (Mareš et al., 2019), scale bar 0.5 μm; (F) Enlarged confocal images of NCDs (Riemann et al., 2022), scale bar 10 μm.
Trichodesmium, a nonheteromorphic genus of filamentous cyanobacterium (Figure 3C), comprises six main branches: T. hildebrantti, T. contortum, T. tenue, T. erythraeum, T. thiebautti, and T. spiralis (Webb et al., 2022). Trichodesmium can be found in nature in the form of free filaments or can be organized in bundles, thereby forming colonies of various shapes and structures, including spherical puffs, tufts, rafts, and bow ties (Webb et al., 2022). Notably, these cells can simultaneously fix N2 and photosynthesis during daylight hours through spatial and temporal separation of the two processes to prevent interference of nitrogenase by O2 produced during photosynthesis (Zehr and Capone, 2020; 2024). A recent macro genome-based study has revealed that not all Trichodesmium are capable of N2 fixation, such as T. miru and T. nobis (Delmont, 2021).
Crocosphaera is a unicellular spherical cyanobacterium (Figure 3D, E) and includes C. watsonii, C. subtropica (previously Cyanothece sp. ATCC 51142), C. chwakensis (previously Cyanothece sp. CCY 0110), and C. waterburyi (Masuda et al., 2024). Crocosphaera occurs as single cells, in pairs, or amorphous aggregates (Zehr et al., 2022). It undergoes photosynthesis during the day and N2 fixation at night to prevent exposure to high levels of O2 (Zehr and Capone, 2020). Furthermore, Crocosphaera exists as two distinct cell types: large (≥4 μm) and small (<4 μm); the genomes of these two types exhibit considerable differences, with the larger cell containing more genomes (Bench et al., 2013).
NCDs mainly include α-, β-, γ-, δ-proteobacteria, archaea and non-cyanobacteria (Candidatus Tectiglobus diatomicola) symbiosis with Haslea (Figure 3F), whose nifH gene is widely distributed in oceans. Moreover, the current research mainly focuses on γ-24774A11 (Turk-Kubo et al., 2022; Tschitschko et al., 2024). It is worth noting that α-proteobacterium (Rhodopseudomonas palustris strain BAL398, Rhodobacteraceae Sagittula castanea E-37) and γ-proteobacterium (Pseudomonas stutzeri strain BAL361, Raoultella ornithinolytica strain BAL286, Candidatus Thalassolituus haligoni) have been isolated by enrichment (Farnelid et al., 2014; Bentzon-Tilia et al., 2015; Martínez-Pérez et al., 2018; Rose et al., 2024). However, the mechanism of nitrogen fixation by NCDs has not been determined. Previous studies have shown that NCDs may be chemoheterotrophs, which use organic matter in the environment to maintain the energy demand of nitrogen fixation. It may also be adsorbed on particulate matter, providing low-oxygen microzones to avoid poisoning NCDs by O2 (Bombar et al., 2016; Turk-Kubo et al., 2022).
3 Effect of TDN on marine diazotrophs
TDN in oceans primarily comprises DIN and dissolved organic nitrogen (DON). DIN is predominantly present in the ocean as NO3− (accounting for 99%), NH4+, and NO2− (Katye et al., 2021). Significant components of DON encompass nitrogenous organic compounds such as proteins, amino acids, nucleic acids, amino sugars, and urea (Millero, 2013).
Marine diazotrophs in the ocean can acquire substantial amounts of N from the atmosphere, resulting in minimal constraints on N metabolism (Zehr and Capone, 2021b). Previous research has indicated that elevated DIN levels may partially inhibit N2 fixation, as the energy required to reduce N2 to NH4+ is 25% greater than that needed to reduce NO3− to NH4+ (Falkowski, 1983). Consequently, when DIN content can satisfy the demand for biological N sources, diazotrophs tend to undergo assimilation rather than N2 fixation, thereby limiting N2 fixation (Karl et al., 2002). However, Knapp suggested that elevated DIN content inhibits the rate of N2 fixation but does not entirely halt it; moreover, BNF still occurs in environments with NO3− concentrations up to 30 μmol L−1 or NH4+ concentrations up to 200 μmol L−1 (Knapp, 2012; Knapp et al., 2012). Studies have also discovered that high DIN content’s impact on N2 fixation may be attributed to (1) environments with high DIN content often being accompanied by relatively high P levels and an increase in nitrogen-fixing biomass due to sufficient P can offset the inhibition of DIN on N2 fixation (Knapp et al., 2012); and (2) the fact DIN could inhibit most laboratory culture’s N2 fixation is due to adding excessive amounts of DIN beyond what is found in field environments, which may overestimate high DIN content inhibition on diazotrophs (Knapp, 2012).
In comparison to DIN, the impact of DON on N2 fixation has been relatively understudied. However, few studies report on diverse mechanisms through which DON regulates N2 fixation in marine organisms. For example, (1) DON can directly influence nitrogenase activity by inhibiting the synthesis of the Fe and MoFe proteins of nitrogenase, thereby impeding N2 fixation (Ohki et al., 1991; Mulholland and Capone, 1999; Mulholland et al., 2001). Moreover, (2) DON can serve as a source of N when N2 fixation is inhibited, and its addition can stimulate the expression of nifH genes in diazotrophs while enhancing the rate of N2 fixation (Benavides et al., 2015, 2017; Walworth et al., 2018; Benavides et al., 2020).
3.1 Effect of changes in DIN content on B. bigelowii
UCYN-A, associated with B. bigelowii is widely distributed in nutrient-rich estuaries and upwelling areas (Vieyra-Mexicano et al., 2024). Studies based on the nano-SIMS technology show that unlike most Marine phytoplankton with the ability to absorb NO3− (Simon and Klotz, 2013), B. bigelowii does not assimilate NO3− only absorbs a small amount of NH4+ in the environment, which is highly unusual in eukaryotic algae (Mills et al., 2020). In laboratory culture, under both limited nitrogen conditions (0.025 μmol L−1 NH4+/0.015 μmol L−1 NO3−) as well as relatively nitrogen-sufficient (0.125 μmol L−1 NH4+/0.2 μmol L−1 NO3−) condition, B. bigelowii has been observed to predominantly rely on N2 fixation performed by UCYN-A to meet its nitrogen requirements (Mills et al., 2020). Furthermore, a separate study indicated that even after supplying 2 μmol L−1 NH4NO3 to B. bigelowii, almost no change was observed in the rate of N2 fixation, and no significant alteration was observed in nifH expression (Krupke et al., 2015). The results indicated that the change in DIN content had no significant effect on the nitrogen fixation of B. bigelowii, which might be related to the unique physiological habit of B. bigelowii. Studies have shown that B. bigelowii is a mixed-trophic organism, and photosynthesis and nitrogen fixation alone are insufficient to maintain growth needs. Moreover, B. bigelowii also needs to obtain additional nutrients by swallowing bacteria (Burns et al., 2018; Mak et al., 2024); nitrogen fixation and ingestion processes may have ingested enough DIN to meet the nitrogen requirements of B. bigelowii.
3.2 Effect of changes in DIN content on Trichodesmium
The results of laboratory culture showed that adding high NO3− (i.e., 10–20 μmol L−1) could reduce the nitrogen fixation rate of Trichodesmium by 50%–70% but could not stop the N2 fixation (Mulholland et al., 2001; Holl and Montoya, 2005; Knapp et al., 2012). This is because high NO3− content will inhibit the expression of nitrogenase subunits and thus inhibit nitrogenase synthesis but not nitrogenase activity (Holl and Montoya, 2005; Sandh et al., 2011). However, although the presence of NO3− will affect the nitrogen fixation of Trichodesmium, it can still absorb a small amount of NO3− in the environment for its individual growth (Mulholland et al., 1999; Eichner et al., 2014; Klawonn et al., 2020). For example, Boatman et al. found that adding 100 μmol L−1 NO3− increased the growth rate of Trichodesmium bunchae by 13% (Boatman et al., 2018).
Mulholland et al. found in laboratory studies that there is a tightly coupled relationship between the release and absorption of NH4+ and Trichodesmiu (Mulholland and Capone, 1999; Mulholland et al., 1999, 2004), high NH4+ (i.e., 10–20 μmol L−1) content inhibited the nitrogenase activity, resulting in a decrease in the nitrogen fixation rate of Trichodesmium (Ohki et al., 1991; Mulholland et al., 2001), but can promote the individual growth of Trichodesmium (Knapp, 2012; Post et al., 2012; Boatman et al., 2018). Klawonn et al. ‘s study in the waters near ALOHA Station in the subtropical North Pacific also confirmed that adding about 1 μmol L−1 of 15NH4+ to collected Trichodesmium can rapidly absorb and assimilate NH4+ for its growth (Klawonn et al., 2020).
3.3 Effect of changes in DIN content on Crocosphaera
Moisander reported that the abundance of Crocosphaera exhibited weak or no correlation with NO3− in the southwestern Pacific Ocean (Moisander et al., 2010). Furthermore, laboratory studies have demonstrated that high NO3− concentrations of 800–1000 μmol L−1 did not inhibit Crocosphaera’s N2 fixation; this may be related to the non-expression of the nitrate assimilation gene (nirA) in the cells from Crocosphaera when cultured (Großkopf and LaRoche, 2012; Dekaezemacker et al., 2013; Rabouille et al., 2021). Consequently, it was concluded that NO3− does not impact individual growth, population growth, and N2 fixation in Crocosphaera. However, studies have also shown that increasing NO3− concentration in the environment can induce and activate NO3− transporters on cell membranes from Crocosphaera (Glibert et al., 2016). Thus, small amounts of NO3− can still be absorbed from Crocosphaera (only 2%–4% of total uptake) (Rabouille et al., 2021; Masuda et al., 2022; Umbricht et al., 2024).
However, as NH4+ is a product of N2 fixation, it can suppress the activity of nitrogenase (Thomas et al., 1982; Dekaezemacker and Bonnet, 2011) and hinder N2 fixation in Crocosphaera. For instance, Dekaezemacker and Bonnet (2011) conducted experiments with NH4+ on Crocosphaera. They observed that increasing NH4+ concentration from 0.2 µmol L−1 to 10 µmol L−1 resulted in a reduction of N2 fixation rates by 36% for C. watsonii WH8501 and 83% for C. watsonii WH0003, respectively, and the total nitrogen fixation was significantly reduced (Dekaezemacker and Bonnet, 2011). Additionally, Garcia and Hutchins (2014) found that adding 0.4 µmol L−1 of NH4+ decreased the rate of N2 fixation in C. watsonii WH0003 by up to 90% (Garcia and Hutchins, 2014). It is worth noting that NH4+ is more accessible to transport across membranes due to the low energy requirements of utilizing NH4+ (Ludwig, 1938; Glibert et al., 2016), tends to utilize NH4+ rather than NO3− first, showing a high absorption rate on NH4+ (Masuda et al., 2013, 2022).
3.4 Effect of changes in DIN content on NCDs
Limited studies have shown that the effects of changes in DIN content on NCDs’ growth and nitrogen fixation are unclear (Moisander et al., 2017). Rose et al. isolated, enriched and cultured γ-proteobacterial, and their genome study showed that γ-proteobacterial contained genes that absorbed and assimilated NH4+ and NO3− (Rose et al., 2024). Therefore, when DIN is deficient, the growth rate of NCDs will be significantly reduced (Bentzon-Tilia et al., 2015). Some reports have also found higher abundances of NCDs in DIN-rich environments (Shiozaki et al., 2014). For example, In the Arab Sea with NO3− content up to 15 μmol L−1 (Bird and Wyman, 2013), in the western Yellow Sea of China with DIN content of 7.29 μmol L−1 (Zhang et al., 2015), and in the Benguela upwelling zone with NO3− content between 5 and 27 μmol L−1 (Sohm et al., 2011a), all detected significant levels of γ-proteobacterial. In addition, through generalized addition model operation on global qPCR copy data of the nifH gene of γ-24774A11, it is also found that the abundance of γ-24774A11 rises significantly when NO3− is greater than 10 μmol L−1 (Shao and Luo, 2022). However, there are few studies on the nitrogen fixation rate of NCDs with the change of NO3− content. Limited studies have shown that the nitrogen fixation rate of NCDs will be significantly inhibited when NO3− content increases (Bentzon-Tilia et al., 2015).
4 Effect of TDP on marine diazotrophs
TDP in the ocean consists of DIP and dissolved organic phosphorus (DOP). In seawater, DIP is predominantly composed of H2PO4− (1%), HPO42− (87%), and PO43− (12%) ions (Ruttenberg, 2014). DOP primarily comprises phosphate monomer (C–O–P), phosphate polymers (C–O–P), and phosphonates (C–P) compounds (Karl and Björkman, 2015).
DIP is acknowledged as a potential limiting factor for BNF in most oceans (Deutsch et al., 2007). For example, in the west-central tropical North Atlantic, substantial amounts of DFe are introduced by winds carrying dust from the Sahara Desert. This stimulates N2 fixation but also results in significant consumption of DIP, leading to P limitation (e.g., 0.2–1.0 nmol L−1) on BNF processes (Sañudo-Wilhelmy et al., 2001; Moore et al., 2009; Martiny et al., 2019). Other studies conducted in the northern part of the subtropical northwest Pacific Gyre have confirmed that atmospheric Fe deposition enhances N2 fixation and subsequently depletes DIP levels, ultimately resulting in a phosphorous deficit (~20 nmol L−1) (Yuan et al., 2023).
Although diazotrophs primarily utilize DIP, DOP may serve as a potential source of phosphorus for the growth of diazotrophs in environments where DOP dominates TDP components (Karl, 2014; Karl and Björkman, 2015; Duhamel et al., 2021; Adams et al., 2022). Several studies have indicated that alkaline phosphatase genes (i.e., phoA, phoD, and phoX) present in diazotrophs play a role in regulating the microbial uptake and metabolization of DOP (Orchard et al., 2009; Frischkorn et al., 2019). Therefore, diazotrophs can utilize DOP to alleviate the P limitation of growth and N2 fixation rates in low-DIP environments (Dyhrman et al., 2006; Karl, 2014; Rouco et al., 2018).
4.1 Effects of changes in TDP content on B. bigelowii
Elevated DIP content has been observed to contribute to population growth and N2 fixation in B. bigelowii. In a study conducted by Langlois, based on on-board culture experiments using samples collected from the northeastern part of the tropical Atlantic Ocean, the addition of 0.2 μmol L−1 DIP was reported to cause an increase in the rate of N2 fixation and abundance of UCYN-A (Langlois et al., 2012). Similarly, a comparable phenomenon was observed in on-board culture experiments by adding 1 μmol L−1 DIP in samples from the southwestern Pacific Ocean (Benavides et al., 2022).
Early studies indicated that UCYN-A lacked genes that are essential for the hydrolysis of the C–O–P phosphate monomer, such as alkaline phosphatase phoA, and for the utilization of the phosphonate C–P, such as phosphonate lyase phnC–P. Hence, UCYN-A is not likely to utilize DOP directly (Tripp et al., 2010; Thompson et al., 2012). However, experiments conducted by Benavides demonstrated that adding 1 μmol L−1 DOP to samples from the southwest Pacific stimulated UCYN-A1 growth (Benavides et al., 2022). This could be attributed to the demand for phosphorus being satisfied by B. bigelowii through a unique utilization strategy (Turk-Kubo et al., 2012; Thompson and Zehr, 2013); however, the underlying mechanism for this utilization remains unclear.
4.2 Effect of changes in TDP content on Trichodesmium
4.2.1 Effect of changes in DIP content on Trichodesmium
Elevated DIP levels in the ocean can stimulate individual growth, population growth and nitrogen fixation of Trichodesmium. Laboratory culture showed that under the condition of 26°C, the growth rate and nitrogen fixation rate of Trichodesmium with a high content of DIP (10 μmol L−1) were three times and two times higher than those with a low content of DIP (0.2 μmol L−1) (Qu et al., 2019), respectively. Under the pH value 7.69–7.76, a high content of DIP (20μmol L−1) was added to Trichodesmium. The results showed that the nitrogen fixation rate and carbon sequestration rate of Trichodesmium increased by 35%–120% and 15%–100%, respectively, compared with the addition of low-content DIP (0.2μmol L−1) (Hutchins et al., 2007). In the field culture experiment (MEGA3) of samples collected in the tropical North Atlantic Ocean, 0.2 μmol L−1 DIP was added, and the nifH gene copy number of Trichodesmium was observed to increase by ~2 times (Turk-Kubo et al., 2012). Webb et al. added DIP of 30 μmol L−1 to samples collected in the tropical South Atlantic and found that nitrogen fixation was significantly stimulated (Webb et al., 2007). It is important to note that if DIP is added to non-phosphorus-restricted areas, it may not stimulate individual growth and population growth of Trichodesmium. In the South China Sea, according to a study, adding 0.1 μmol L−1 was not observed after the DIP of Trichodesmium cells abundance increased because the environment here DIP concentration is relatively high (5–20 nmol L−1) (Wu et al., 2003).
Several studies have attempted to explain the mechanism of DIP utilization by Trichodesmium from the perspectives of DIP absorption kinetics and molecular biology. In the southwestern Pacific Trichodesmium DI33P absorption kinetics experiments, the results show that when the DIP > 9 nmol L−1, surface waters in Trichodesmium absorption DIP alone would maintain cell growth (Moutin et al., 2005). Further found that Trichodesmium had a higher rate of DIP uptake under P-limited (0.2 μmol L−1) (Fu et al., 2005); this is because, under the condition of phosphorus limitation, Trichodesmium can increase intracellular phosphate transporter sphX expression of the genes encoding to improve the ability of from extracellular DIP, At the same time, the expression of nifH gene was down-regulated, showing a lower rate of nitrogen fixation (Orchard et al., 2009; Held et al., 2020). In addition, the results of DI33P absorption kinetics also showed that the total absorption rate of DIP in Trichodesmium was lower than that of environmental microorganisms, indicating that the ability of Trichodesmium to compete for DIP was worse than that of other microorganisms in the environment (Sohm and Capone, 2006; Sohm et al., 2008; Orchard et al., 2010a; Glibert, 2024), so Trichodesmium need to adopt more strategies to maintain their growth in low-P environments.
Due to the low DIP levels in most surface oceans, Trichodesmium has evolved strategies to cope with low concentrations of DIP. In laboratory culture, it was found that under low DIP (0.5 μmol L−1), Trichodesmium can increase the DIP absorption area of cells by increasing the individual length (Zhu et al., 2020). Low DIP can also stimulate Trichodesmium mobile and induce colony formation (Tzubari et al., 2018), and Trichodesmium colonies can sense and the choice of particles that are rich in P to maximize absorption of the DIP from the ocean (Held et al., 2020; Wang et al., 2022a); Trichodesmium can also change the demand for P in different cellular processes by reducing the transcription and translation process of ribosomal proteins (with a high demand for P), adjusting ATP concentration, and replacing phospholipids with sulfur lipids (Van Mooy et al., 2009; Spungin et al., 2014; Grosse et al., 2017). In addition, some studies also found that when the Trichodesmium is in a DIP-restricted environment, DIP is stored in the cell surface by Trichodesmium’s surface adsorption (Sañudo-Wilhelmy et al., 2004), and Trichodesmium can also store limited DIP in the form of polyP to cells (Orchard, 2010; Orchard et al., 2010b), and directly or indirectly alleviate the effects of DIP restriction on cells (Björkman, 2014; Sanz-Luque et al., 2020). Moreover, Trichodesmium can also migrate to the phosphocline and store phosphorus by adjusting its buoyancy and then return to the surface ocean for photosynthesis and nitrogen fixation (Karl et al., 1992; White et al., 2006).
4.2.2 Effect of changes in DOP content on Trichodesmium
The change in DOP content can stimulate Trichodesmium’s individual growth and nitrogen fixation. Orchard et al. added 0.25–1 μCi DO33P to Trichodesmium collected in the North Atlantic Ocean. They found that more than 25% of the P absorbed by Trichodesmium at most stations was DOP (Orchard et al., 2010a), indicating that DOP was an important P source for Trichodesmium (Fu et al., 2005; Dyhrman et al., 2006). This is because when DIP is restricted, Trichodesmium can up-regulate the expression of alkaline phosphatase genes (phoA, phoD, and phoX) (Stihl et al., 2001; Orchard et al., 2009), thereby changing the intracellular protein abundance and enzyme activity of DOP hydrolysis to promote the absorption and utilization of DOP by cells (Frischkorn et al., 2019). It is worth noting that Mulholland et al. ‘s culture experiment suggested that adding 1–50 μmol L−1 DOP could stimulate the growth of Trichodesmium. However, the individual growth caused by the addition of high DOP content was not as high as that caused by the addition of low DOP content (Mulholland et al., 2002). This may be due to the inhibition of alkaline phosphatase synthesis by DIP produced by DOP hydrolysis, which leads to the limitation of DOP that Trichodesmium can absorb and utilize and small changes in individual growth (Stihl et al., 2001).
Trichodesmium has developed many strategies to fully absorb and utilize DOP under DIP constraints. Epibiotic bacteria within Trichodesmium aggregates can sense the P source or, by use of quorum sensing, perceive and choose other alternative metal enzymes to regulate the activity of alkaline phosphatase (Van Mooy et al., 2012; Lee et al., 2017) to promote the absorption and utilization of DOP by Trichodesmium under phosphorus restriction. For example, Rouco et al. found that the genes regulating DOP in the North Atlantic and North Pacific are dominated by phnX (Ca and Fe as cofactors) and phoA (Zn and Mg as cofactors), respectively, and that Trichodesmium can switch the relative transcriptional abundance of the required metalloenzymes and change the PhoA/PhoX ratio for DOP hydrolysis. This stimulates the expression of the alkaline phosphatase gene (Rouco et al., 2018; Wang et al., 2022b).
4.3 Effect of changes in TDP content on Crocosphaera
4.3.1 Effect of changes in DIP content on Crocosphaera
Laboratory culture studies have demonstrated that increased DIP content helps stimulate individual growth and N2 fixation in Crocosphaera (Zhu et al., 2020). In these cultures, the supplementation of 10 μmol L−1 DIP led to a three- to fourfold enhancement in the respective growth and N2 fixation rates of Crocosphaera compared to the addition of 0.1 μmol L−1 DIP (Liu et al., 2020). Pereira et al. also found that adding sufficient DIP (60 nmol L−1) can significantly reduce the transcription level of the high-affinity phosphate-binding protein pstS from Crocosphaera and increase cell abundance by 12% within three days (Pereira et al., 2016; 2019). However, when DIP concentration is in the threshold of 20–60 nmol L−1, the transcription level of pstS in cells is up-regulated, which increases the nitrogen fixation rate (Pereira et al., 2019; Marki et al., 2020; Rahav et al., 2022). Higher temperatures can mitigate the effects of DIP limits on growth and nitrogen fixation (Fu et al., 2014). The gene expressions of pstS and sphX on Crocosphaera are up-regulated to increase DIP uptake under P-limited conditions (0.2 μmol L−1) and high-temperature conditions, so the growth and nitrogen fixation rate of Crocosphaera will increase with increasing temperature (Deng et al., 2022).
However, some onboard culture experiments have yielded inconsistent results from observations from DIP additions to laboratory cultures. For example, Turk-Kubo et al. conducted cultures of collected samples in the tropical North Atlantic Ocean following the addition of 0.2 μmol L−1 DIP and observed that higher levels of DIP in areas affected by the Amazon River plume already met the P requirements of Crocosphaera (Turk-Kubo et al., 2012). Consequently, exogenous DIP did not significantly increase the copy number of the Crocosphaera nifH gene in this region. Similar findings were reported by Benavides from an on-board culture study conducted in the tropical southwest Pacific Ocean (Benavides et al., 2022). Notably, a recent study revealed that adding 0.1 μmol L−1 DIP to samples collected from P-limited regions such as the eastern Mediterranean resulted in a decrease rather than an increase in Crocosphaera’s nitrogen fixation rate, presumably due to non-nitrogen-fixing heterotrophs responding more rapidly to DIP additions. The non-nitrogen-fixing heterotrophs have a faster rate of DIP turnover than Crocosphaera, so they consume a large amount of DIP (Rahav et al., 2022).
4.3.2 Effect of changes in DOP content on Crocosphaera
As Crocosphaera lacks a homologue of the phosphonate-specific transfer protein phnCDE in its genome and is unable to utilize phosphonate C–P for growth, fluctuations in the phosphonate C–P content of DOP have minimal impact on Crocosphaera growth (Dyhrman and Haley, 2006). Interestingly, Filella et al. observed an enhancement in the rate of N2 fixation by Crocosphaera following the addition of 20 μmol L−1 DOP (adenosine monophosphate: AMP, belonging to the phosphate ester monomers C–O–P) to samples collected near the Tonga volcano (Filella et al., 2022), indicating that Crocosphaera can utilize the phosphate ester monomer C–O–P in DOP as a source of organophosphorus, consistent with findings from several recent studies (Pereira et al., 2019; Yamaguchi et al., 2020). The differences for Crocosphaera in the cracking efficiency of different components in DOP may explain the above differences (Rabouille et al., 2022).
4.4 Effect of changes in TDP content on NCDs
The effect of DIP content change on NCDs’ growth and nitrogen fixation is still controversial. Studies have shown that NCDs can increase their ability to obtain DIP from the outside world by regulating gene expression in the cell under DIP restriction. The analysis of the γ-4 genome showed that the γ-4 genome contains phoBR, pstSCAP, phnCDE and ppK-ppX genes related to phosphorus clearance, and the PhoB-PhoR two-component system in γ-proteobacterial can sense DIP limitation. This activating gene expression encodes high-affinity phosphate transporter (PstSCAP) (Chakraborty et al., 2011; Cheung et al., 2021). However, no evidence of the sensitivity of NCDs to DIP has been found in field studies. For example, Zehr et al. added 0.5 μmol L−1 DIP to samples collected in the North Pacific subtropical gyre. They found that γ-a nitrogen fixation rate and nifH gene transcription were not significantly stimulated (Zehr et al., 2007); Moisander et al. also found little change in the expression of γ-24774A11 nifH gene after adding 2 μmol L−1 DIP to samples collected in the waters off eastern Australia (Moisander et al., 2012).
5 Effect of DFe on marine diazotrophs
Iron from atmospheric deposition, surface runoff, seafloor hydrothermal fluids, sediment inputs, and glacial melting are key nutrient elements that influence the primary productivity of the global oceans (Tagliabue et al., 2017; Jiang et al., 2024). Fe in the ocean exists in granular (>0.4 μm), colloidal (0.02–0.4 μm), and dissolved (<0.02 μm) forms. DFe is an essential form of iron that is available to diazotrophs. Diazotroph nitrogenase consists of a Fe-protein and a FeMo protein encoded by nifHDK (Zhang et al., 2020), with diazotrophs requiring fivefold more Fe for N2 fixation than for ammonia assimilation and tenfold more than other phytoplankton (Kustka et al., 2003; Sohm et al., 2011b). However, DFe content is relatively low in most marine environments, leading to its long-standing recognition as a limiting factor for marine BNF.
In laboratory and on-board culture experiments, higher DFe content is frequently associated with elevated rates of N2 fixation. An onboard DFe addition experiment conducted at the ALOHA station revealed a 128% increase in the rate of N2 fixation in response to the addition of 2 nmol L−1 of DFe (Grabowski et al., 2008). Studies in the Atlantic affected by Fe inputs from Saharan desert wind and dust have demonstrated a positive correlation between DFe content and N2 fixation rates (Orcutt et al., 2001; Tyrrell et al., 2003; Moore et al., 2009). Similarly, regions with higher N2 fixation rates in the northwestern Pacific Ocean and the North Pacific gyre correspond to those with higher atmospheric dust deposition fluxes (Shiozaki et al., 2009, 2010; Yuan et al., 2023). These correlations suggest that atmospheric Fe deposition may influence N2 fixation. Furthermore, recent studies have also indicated that DFe (i.e., up to 66 nmol L−1) released by shallow hydrothermal fluids has a significant impact on N2 fixation, establishing the southwest tropical Pacific as a new N2 fixation hotspot (Bonnet et al., 2023; Mériguet et al., 2023).
5.1 Effects of changes in DFe content on B. bigelowii
An elevated DFe content is moderately beneficial for population growth and N2 fixation in B. bigelowii. Despite the absence of PSII, UCYN-A retains genes that regulate Fe3+ transporter proteins (afuABC), which may play a crucial role in oligotrophic ocean areas with limited DFe content (Zehr et al., 2016). In an on-board culture experiment, adding 400 nmol L−1 DFe to samples collected from eastern Australian waters significantly increased nifH gene copy numbers in UCYN-A (Moisander et al., 2012). Another experiment conducted with samples from the temperate northeast Atlantic Ocean showed that adding 200 nmol L−1 DFe led to a twofold increase in the rate of N2 fixation by UCYN-A1 (Li et al., 2018). Furthermore, a recent study near the Bering Strait demonstrated a notable increase in UCYN-A abundance in response to elevated content of Fe sourced from riverine inputs (Cheung et al., 2022).
Notably, adding DFe to collected samples did not increase nifH expression in UCYN-A sampled from DFe-rich marine areas. For instance, Turk-Kubo et al. conducted a DFe addition experiment at sampling locations across the tropical North Atlantic; following the addition of 2 nmol L−1 DFe, the authors observed an increase in the nifH copy number of UCYN-A only in samples obtained from the Amazon River plume, and no significant increase was observed at any other station. This could be attributed to the fact that the ambient DFe levels in other regions (0.74 ± 0.01 nmol L−1) already meet UCYN-A’s Fe requirements (Turk-Kubo et al., 2012).
5.2 Effects of changes in DFe content on Trichodesmium
The addition of 1 μmol L−1 of DFe in laboratory culture studies has resulted in a nearly tenfold increase in the rates of N2 fixation in Trichodesmium (Rueter, 1988; Paerl et al., 1994). In an on-board culture experiment conducted in Fe-limited waters, a significant increase in the rate of N2 fixation was observed with the addition of 50 nmol L−1 DFe to samples of Trichodesmium collected from the western part of the southern equatorial Atlantic (Webb et al., 2007). Additionally, outbreaks of Trichodesmium blooms have also been documented on the continental shelf off the west coast of Florida, which are known as areas with high DFe levels (16 nmol kg−1) (Lenes et al., 2001). Trichodesmium can absorb and utilize DFe because it can produce a reducing agent (superoxide) and release it into vitro (Roe and Barbeau, 2014), reducing Fe3+ to Fe2+ in vitro. Fe2+ is transported into the Trichodesmium by the high-affinity Fe2+ transporters FeoA and FeoB on the plasma membrane of Trichodesmium (Chappell and Webb, 2010).
Some studies have reported the possible adaptation mechanism of Trichodesmium in the absence of DFe content. Under the condition of limited DFe content, the abundance of proteins involved in the absorption of DFe increased, while the abundance of iron-binding proteins involved in photosynthesis and the activity of nitrogenases decreased rapidly, indicating that Trichodesmium can rapidly redistribute limited DFe resources and transfer iron from the photosynthetic system, respiratory transport chain and other cellular pathways. It is used to increase the synthesis of iron-rich nitrogenase to cope with the decrease in nitrogen fixation efficiency caused by iron restriction (Küpper et al., 2008; Snow et al., 2015; Zhang et al., 2019). Trichodesmium colonies can also directly use particulate iron or sand dust to maintain the iron demand in the body under the condition of DFe content limitation (Rubin et al., 2011; Polyviou et al., 2018; Eichner et al., 2023). This process may include the following steps: (1) Trichodesmium can migrate and aggregate and form puff-like colonies under Fe restriction (Roe et al., 2012; Tzubari et al., 2018) because puff-like colonies have higher efficiency of iron uptake (Achilles et al., 2003; Roe et al., 2012); (2) The particulate iron and sand are attached to the filaments of the Trichodesmium colony and transferred to the centre of the colony along the filaments (Rubin et al., 2011; Basu and Shaked, 2018); (3) Iron complex molecules produced by bacteria parasitizing the centre of their colonies can promote the dissolution of particulate iron (Frischkorn et al., 2017; Lee et al., 2017; Basu et al., 2019). Individual studies have also found that H2 may provide a source of electrons for mineral iron reduction in Trichodesmium cells, thereby promoting Fe uptake by Trichodesmium colonies (Eichner et al., 2019).
It is worth noting that adding sufficient DFe (925 pmol L−1) or limited DFe (35 pmol L−1) to Trichodesmium at pH 7.81 showed lower growth and nitrogen fixation rates compared with pH 8.02 (Zhang et al., 2019). It is suggested that pH change may affect the effect of DFe on Trichodesmium. It has been shown that under acidification conditions, Trichodesmium cells mitigate the acidification-induced reduction in nitrogen fixation rate by up-regulating the expression of nitrogenase genes. However, the processes of nitrogenase synthesis and energy production are iron-demanding so that iron limitation (as low as 0.04 nmol L−1) exacerbates the suppression of Trichodesmium nitrogen fixation rate by ocean acidification (Shi et al., 2012; Hong et al., 2017). Therefore, when Trichodesmium faces the effects of both iron limitation and ocean acidification, it needs to divert iron from cellular pathways, such as the photosynthetic system and respiratory transport chain, and use it for nitrogenase synthesis in response to decreased nitrogen-fixing efficiency due to acidification (Zhang et al., 2019).
5.3 Effects of changes in DFe content on Crocosphaera
Elevated DFe content typically enhances the expression of the nifH gene and N2 fixation in Crocosphaera. In laboratory cultures, Crocosphaera can efficiently utilize added DFe (i.e., 250–450 nmol L−1), resulting in a substantial increase in N2 fixation rates of up to 17-fold (Fu et al., 2008; Jacq et al., 2014; Garcia et al., 2015; Yang et al., 2021). Lory et al. found that high DFe content (~1.6 nmol L−1) near the volcano of Tonga in the western tropical South Pacific significantly stimulates nitrogen fixation on Crocosphaera and added 0.2 nmol L−1 D55Fe to the collected samples (Lory et al., 2022). The results showed that Fe: C from Crocosphaera is higher than that from other non-nitrogen-fixing organisms, and the cell-specific iron uptake rate (0.46–1.21 amol Fe cell−1 d−1) is more than two times higher than that from other non-nitrogen-fixing organisms. It suggests that DFe can be actively absorbed from Crocosphaera to maintain cell growth (Tuit et al., 2004; Lory et al., 2022).
However, since large Crocosphaera cells (≥4 μm) have relatively higher copy numbers of the iron-related genes isiA and feoB than small cells (<4 μm), the effect of adding DFe to samples sourced from different regions with different Crocosphaera cell’s size can vary (Bench et al., 2013; Masuda et al., 2022; Zehr et al., 2022). For instance, the addition of DFe at a concentration of 10 nmol L−1 resulted in nearly unchanged N2 fixation rates and cellular abundance in small Crocosphaera cells (<4 μm) during culture experiments conducted in the Southern Ocean (Benavides et al., 2022). Conversely, adding 400 nmol L−1 of DFe to samples collected from eastern Australian waters led to a significant increase in the nifH gene-copy number in large Crocosphaera cells (≥4 μm) (Moisander et al., 2012; Bench et al., 2013).
In addition, strategies have evolved from Crocosphaera to adapt to Fe-limiting environments, such as re-using Fe or reducing cell size (Saito et al., 2011; Jacq et al., 2014). Saito et al. found that Fe demand from cells can be reduced by efficient diurnal cycling of nitrogen fixation and photosynthetic-related Ferri proteins, and this strategy reduces the total iron requirement of cells by about 40% (Saito et al., 2011; Masuda et al., 2022); Crocosphaera can also keep enough energy on cells by reducing cell size under Fe constraints (1–3.3 nmol L−1) (Jacq et al., 2014; Marki et al., 2020), and the reduction of cell size can ease the absorption rate limit and diffusion limit caused by Fe element (Garcia et al., 2015), to maintain the optimal nitrogen fixation rate and carbon sequestration rate from Crocosphaera.
5.4 Effect of changes in DFe content on NCDs
Studies have shown that the genes afuABC and fhuDCB, which regulate the Fe3+ABC transporter, were found in γ-24774A11 cells (Cheung et al., 2021), indicating that NCDs have the potential to utilize DFe to meet its own growth and nitrogenase synthesis needs. For example, Moisander et al. observed a significant increase in the nifH gene copy number of γ-24774A11 after adding 400 nmol L−1 DFe to the samples collected in the eastern waters of Australia (Moisander et al., 2012). A similar phenomenon was observed by Langlois et al. after the addition of 2 nmol L−1 DFe along the subtropical South Atlantic coast (Langlois et al., 2012). However, a generalized additive model study on the qPCR copy data of the nifH gene of γ-24774A11 in the world showed that the abundance of γ-A decreased with the increase of DFe content, which may be because γ-A has a competitive advantage compared with autotrophic nitrogen-fixing cyanobacteria in low-iron environments (Shao and Luo, 2022).
6 Conclusions and perspectives
We have observed that most researchers support several general patterns by analysing the impacts of nutrients such as TDN, TDP, and DFe on B. bigelowii, Trichodesmium, Crocosphaera, and NCDs. First, it was observed that changes in DIN levels had little effect on B. bigelowii; increased DIN levels had a negative effect on nitrogen fixation by Trichodesmium, although it did not wholly inhibit N2 fixation. Additionally, elevated NO3− levels did not impact Crocosphaera, but elevated NH4+ significantly hindered its ability to fix N; the effect on NCDs is unclear. Under phosphorus-limited conditions, high DIP content can enhance individual growth, population growth, and N2 fixation in diazotrophs; however, in nonphosphorus-limited areas, increased DIP content may have limited effects on diazotrophs. The influence of changes in DOP content on most diazotrophs remains unclear; nevertheless, DOP could still serve as a potential source of P under DIP-constrained conditions. Elevated DFe content is often linked to higher rates of N2 fixation due to the correlation between the structural composition of nitrogenase and Fe demand. However, if ambient DFe levels already meet the Fe requirements of diazotrophs, further increases in DFe content may not lead to significant increases in N2 fixation rates.
Following years of research, we have developed a basic understanding of how nutrients affect the growth of diazotrophs and their BNF. However, due to the complexity of the marine environment and limited knowledge regarding diazotrophs, our understanding of this mechanism is limited and requires future elucidation.
6.1 Advance the cultivation of as-yet uncultivated marine diazotrophs
The application of molecular biology techniques to the study of marine diazotrophs has contributed significantly to the understanding of the role of marine BNF, especially in recent years when the development of high-throughput sequencing technologies such as macro genomes and macro transcriptomes (Delmont et al., 2022; Tschitschko et al., 2024) has broadened the understanding of uncultured marine diazotrophs. For example, Delmont et al. found from the TARA Oceans macro genome that NCDs express the nifH gene in situ and that their macroeconomic abundance exceeds that of most open-ocean cyanobacterial diazotrophs (Delmont et al., 2018, 2022).
However, due to the low abundance of most NCDs, no direct evidence has been obtained to date that NCDs can perform nitrogen fixation in the in situ ocean (Turk-Kubo et al., 2022). To address the challenge that it is difficult to achieve the detection of low-abundance cells with the current level of detection (Bombar et al., 2016), scholars have begun to carry out research on the metabolic pathways and physiological and ecological characteristics of NCDs by integrating the application of enrichment and isolation with molecular techniques. For example, Martínez-Pérez et al. enriched S. castanea among α-proteobacteria from an active nitrogen-fixing region in the Peruvian upwelling zone and detected its active metabolic processes (Martínez-Pérez et al., 2018); Rose et al. enriched γ- proteobacterial diazotrophs Candidatus Thalassolituus haligoni from Halifax Harbour, and the cell-specific nitrogen-fixing rate of the γ-proteobacterium was obtained from measurements made by 15N tracer technology (Rose et al., 2024). Although they enriched cultures of several isolates, the rate of nitrogen fixation by NCDs in the in situ ocean is still unavailable. For this reason, there is an urgent need to accelerate the realisation of pure cultures of these enriched isolates and to select optimal model organisms for physiological and ecological studies (Lewis et al., 2021) to confirm the ability of NCDs to fix nitrogen in the in situ environment aggressively.
Isolation to obtain pure strains is the best method to study microorganisms’ metabolic activity and physiological properties (Liu et al., 2022), but it comes with significant challenges. New technologies may accelerate the realisation of pure cultures of diazotrophs such as NCDs. For example, the reverse genomics approach is essential for targeting the culture of marine microorganisms, which provides technical support for the targeted isolation and culture of functional microorganisms (Cross et al., 2019); automated Raman-based sorting techniques allow for the functional classification of stable isotope-labelled living cells, which can generate living cells suitable for subsequent single-cell genomics, micro-macro-genomics, and pure culture (Lee et al., 2019).
6.2 Strengthen the investigation into the synergistic effects of multiple nutrients on marine BNF
Current research on the role of nutrients in marine BNF often focuses on a single nutrient, and studies of single-nutrient limitation may fail to capture the complexity of the in situ physiological ecology of diazotrophs (Held et al., 2020). Indeed, in the surface layer of the open ocean, where multiple nutrients are often simultaneously deficient (Saito et al., 2008; Moore et al., 2013), co-limitation of nutrients often occurs (Garcia et al., 2015; Held et al., 2020; Cerdan-Garcia et al., 2022; Yang et al., 2022; Browning and Moore, 2023). In addition, global warming and ocean acidification and nutrients can synergistically affect marine BNF (Deutsch et al., 2024; Hutchins and Tagliabue, 2024). For example, DFe limitation exacerbates ocean acidification’s adverse effects on Trichodesmium’s growth and nitrogen fixation (Hong et al., 2017; Zhang et al., 2019). Thus, it is necessary and urgent to research the synergistic effects of nutrients on marine BNF.
Studies have reported the effects of global warming and ocean acidification on nitrogen fixation in synergy with nutrients through in-laboratory simulations and ecological modelling studies (Deutsch et al., 2024; Hutchins and Tagliabue, 2024). However, these synergistic effects are difficult to confirm through field culture studies due to the relatively large timescales of global warming and ocean acidification and small culture timescales (Gruber, 2016), as well as the complexity and variability of marine environments and the multiple resistances to obtaining high-resolution sampling data (Benavides and Robidart, 2020). The recent broadening of the understanding of marine nitrogen-fixing biodiversity has further increased the complexity and difficulty of studies (Hutchins and Capone, 2022).
The integrated application of laboratory simulations, genomics techniques, in situ cultures and long-term observations, combined with artificial intelligence techniques such as machine learning (Tang et al., 2019) and the construction of Earth system models (Yao et al., 2022), ecological models, can help to comprehensively and integratively assess the synergistic effects of nutrients as well as the impacts of global changes on marine BNF, respectively (Zehr and Capone, 2024).
6.3 Enhance the advancement of innovative technologies and methodologies for investigating marine BNF
With the application of technologies such as flow cytometry (Geisler et al., 2023), macro genomics and macro transcriptomics (Delmont, 2021), nano-SIMS (Mills et al., 2020), and soft X-ray tomography (SXT) (Coale et al., 2024), the physiological and ecological study of marine nitrogen-fixing organisms has refreshed our understanding of marine nitrogen-fixing biodiversity. For example, UCYN-A, long thought to be ‘endosymbiotic’, is an organelle-nitroplast within the host, and marine diazotrophs have been corrected from containing only prokaryotes to both prokaryotes and eukaryotes (Coale et al., 2024; Massana, 2024).
Around the determination of nitrogen fixation rates, Großkopf et al. proposed a 15N2 enriched water incubation method in 2012 (Großkopf et al., 2012). Comparative experiments have revealed that because 15N2 bubbles do not dissolve sufficiently in incubation bottles (Mohr et al., 2010), the 15N2 bubble injection method, which has been traditionally used for the past 20 years, potentially underestimates the nitrogen fixation rate of 15N2-dominated marine waters dominated by Trichodesmium (Großkopf et al., 2012). Although the 15N2 enriched water method can introduce potential nutrient and trace metal contamination due to its operational complexity (Klawonn et al., 2015), it is still the most accurate and direct estimate of nitrogen fixation rates available (White et al., 2020). Recently, new sensitive methods such as stable-isotope probing (Kapili et al., 2020) and isotopic acetylene reduction assay (Haynes et al., 2022) have begun to be applied to the determination of oceanic nitrogen fixation rates, which are expected to obtain rates closer to the real ones.
In addition, almost all the studies on marine BNF are based on shipboard culture after water harvesting or laboratory culture, which makes it challenging to fully reflect the actual marine environment and limits our comprehensive understanding of the role of marine diazotrophs. Researchers have begun to develop marine in situ culture techniques. For example, the Microbial Sampler-Submersible Incubation Device (MS-SID) applies the 15N2 enriched water incubation method to achieve in situ culture of BNF as well as collection and preservation of in situ diazotrophs, but it is limited to in situ deployment of short-term time series due to the limited sample preservation time (Bombar et al., 2015; Edgcomb et al., 2016); the Environmental Sample Processor (ESP) enables in situ genetic analyses of diazotrophs and in situ assessment of nitrogen-fixing biodiversity, an abundance of specific genes and metabolites through DNA probes, protein arrays and qPCR techniques, but its large size and deployment and recovery require significant logistical assistance (Robidart et al., 2014; Scholin et al., 2017). Due to the complexity of the marine environment, the integrated technology of in situ culture and biodiversity analysis for marine nitrogen fixation still faces many technical challenges that need to be broken through. Nevertheless, advances in in situ culture and analysis techniques are expected to advance our understanding of marine BNF.
Author contributions
WC: Writing – review & editing, Writing – original draft, Visualization, Investigation. QY: Writing – review & editing, Writing – original draft, Visualization, Project administration, Funding acquisition, Conceptualization. FJ: Writing – review & editing, Supervision. CL: Writing – review & editing.
Funding
The author(s) declare that financial support was received for the research, authorship, and/or publication of this article. This research was sponsored by the National Natural Science Foundation of China (40976025), the Natural Science Foundation of Shanghai (21ZR1466700), the China National Scientific Seafloor Observatory (2017-000030-73-01-002437), and the interdisciplinary project of Tongji University (2023-1-ZD-03, 2022-2-YB-05).
Acknowledgments
We thank all those who helped write this article and the editors and reviewers of this paper for their constructive feedback.
Conflict of interest
The authors declare that the research was conducted in the absence of any commercial or financial relationships that could be construed as a potential conflict of interest.
Publisher’s note
All claims expressed in this article are solely those of the authors and do not necessarily represent those of their affiliated organizations, or those of the publisher, the editors and the reviewers. Any product that may be evaluated in this article, or claim that may be made by its manufacturer, is not guaranteed or endorsed by the publisher.
References
Achilles K. M., Church T. M., Wilhelm S. W., Luther G. I. W., Hutchins D. A. (2003). Bioavailability of iron to Trichodesmium colonies in the western subtropical Atlantic Ocean. Limnol. Oceanogr. 48, 2250–2255. doi: 10.4319/lo.2003.48.6.2250
Adams J. C., Steffen R., Chou C.-W., Duhamel S., Diaz J. M. (2022). Dissolved organic phosphorus utilization by the marine bacterium Ruegeria pomeroyi DSS-3 reveals chain length-dependent polyphosphate degradation. Environ. Microbiol. 24, 2259–2269. doi: 10.1111/1462-2920.15877
Basu S., Gledhill M., de Beer D., Prabhu Matondkar S. G., Shaked Y. (2019). Colonies of marine cyanobacteria Trichodesmium interact with associated bacteria to acquire iron from dust. Commun. Biol. 2, 284. doi: 10.1038/s42003-019-0534-z
Basu S., Shaked Y. (2018). Mineral iron utilization by natural and cultured Trichodesmium and associated bacteria. Limnol. Oceanogr. 63, 2307–2320. doi: 10.1002/lno.10939
Benavides M., Berthelot H., Duhamel S., Raimbault P., Bonnet S. (2017). Dissolved organic matter uptake by Trichodesmium in the Southwest Pacific. Sci. Rep. 7, 41315. doi: 10.1038/srep41315
Benavides M., Caffin M., Duhamel S., Foster R. A., Grosso O., Guieu C., et al. (2022). Anomalously high abundance of Crocosphaera in the South Pacific Gyre. FEMS Microbiol. Lett. 369, fnac039. doi: 10.1093/femsle/fnac039
Benavides M., Duhamel S., Van Wambeke F., Shoemaker K. M., Moisander P. H., Salamon E. R., et al. (2020). Dissolved organic matter stimulates N2 fixation and nifH gene expression in Trichodesmium. FEMS Microbiol. Lett. 367, fnaa034. doi: 10.1093/femsle/fnaa034
Benavides M., Moisander P. H., Berthelot H., Dittmar T., Grosso O., Bonnet S. (2015). Mesopelagic N2 fixation related to organic matter composition in the solomon and bismarck seas (Southwest pacific). PloS One 10, e0143775. doi: 10.1371/journal.pone.0143775
Benavides M., Robidart J. (2020). Bridging the spatiotemporal gap in diazotroph activity and diversity with high-resolution measurements. Front. Mar. Sci. 7. doi: 10.3389/fmars.2020.568876
Bench S. R., Heller P., Frank I., Arciniega M., Shilova I. N., Zehr J. P. (2013). Whole genome comparison of six Crocosphaera watsonii strains with differing phenotypes. J. Phycol. 49, 786–801. doi: 10.1111/jpy.12090
Bentzon-Tilia M., Severin I., Hansen L. H., Riemann L. (2015). Genomics and ecophysiology of heterotrophic nitrogen-fixing bacteria isolated from estuarine surface water. mBio 6, e00929. doi: 10.1128/mBio.00929-15
Bird C., Wyman M. (2013). Transcriptionally active heterotrophic diazotrophs are widespread in the upper water column of the Arabian Sea. FEMS Microbiol. Ecol. 84, 189–200. doi: 10.1111/1574-6941.12049
Björkman K. M. (2014). Polyphosphate goes from pedestrian to prominent in the marine P-cycle. Proc. Natl. Acad. Sci. U. S. A. 111, 7890–7891. doi: 10.1073/pnas.1407195111
Boatman T. G., Davey P. A., Lawson T., Geider R. J. (2018). The physiological cost of diazotrophy for Trichodesmium erythraeum IMS101. PloS One 13, e0195638. doi: 10.1371/journal.pone.0195638
Bombar D., Paerl R. W., Riemann L. (2016). Marine non-cyanobacterial diazotrophs: moving beyond molecular detection. Trends Microbiol. 24, 916–927. doi: 10.1016/j.tim.2016.07.002
Bombar D., Taylor C. D., Wilson S. T., Robidart J. C., Rabines A., Turk-Kubo K. A., et al. (2015). Measurements of nitrogen fixation in the oligotrophic North Pacific Subtropical Gyre using a free-drifting submersible incubation device. J. Plankton Res. 37, 727–739. doi: 10.1093/plankt/fbv049
Bonnet S., Guieu C., Taillandier V., Boulart C., Bouruet-Aubertot P., Gazeau F., et al. (2023). Natural iron fertilization by shallow hydrothermal sources fuels diazotroph blooms in the ocean. Science 380, 812–817. doi: 10.1126/science.abq4654
Bristow L. A., Mohr W., Ahmerkamp S., Kuypers M. M. M. (2017). Nutrients that limit growth in the ocean. Curr. Biol. 27, R474–R478. doi: 10.1016/j.cub.2017.03.030
Browning T. J., Moore C. M. (2023). Global analysis of ocean phytoplankton nutrient limitation reveals high prevalence of co-limitation. Nat. Commun. 14, 5014. doi: 10.1038/s41467-023-40774-0
Burns J. A., Pittis A. A., Kim E. (2018). Gene-based predictive models of trophic modes suggest Asgard archaea are not phagocytotic. Nat. Ecol. Evol. 2, 697–704. doi: 10.1038/s41559-018-0477-7
Cerdan-Garcia E., Baylay A., Polyviou D., Woodward E. M. S., Wrightson L., Mahaffey C., et al. (2022). Transcriptional responses of Trichodesmium to natural inverse gradients of Fe and P availability. ISME J. 16, 1055–1064. doi: 10.1038/s41396-021-01151-1
Chakraborty S., Sivaraman J., Leung K., Mok Y. (2011). Two-component phoB-phoR regulatory system and ferric uptake regulator sense phosphate and iron to control virulence genes in type III and VI secretion systems of edwardsiella tarda*. J. Biol. Chem. 286, 39417–39430. doi: 10.1074/jbc.M111.295188
Chappell P. D., Webb E. A. (2010). A molecular assessment of the iron stress response in the two phylogenetic clades of Trichodesmium. Environ. Microbiol. 12, 13–27. doi: 10.1111/j.1462-2920.2009.02026.x
Cheung S., Liu K., Turk-Kubo K. A., Nishioka J., Suzuki K., Landry M. R., et al. (2022). High biomass turnover rates of endosymbiotic nitrogen-fixing cyanobacteria in the western Bering Sea. Limnol. Oceanogr. Lett. 7, 501–509. doi: 10.1002/lol2.10267
Cheung S., Zehr J. P., Xia X., Tsurumoto C., Endo H., Nakaoka S. I., et al. (2021). Gamma4: a genetically versatile Gammaproteobacterial nifH phylotype that is widely distributed in the North Pacific Ocean. Environ. Microbiol. 23, 4246–4259. doi: 10.1111/1462-2920.15604
Coale T. H., Loconte V., Turk-Kubo K. A., Vanslembrouck B., Mak W. K. E., Cheung S., et al. (2024). Nitrogen-fixing organelle in a marine alga. Science 384, 217–222. doi: 10.1126/science.adk1075
Cornejo-Castillo F. M., Inomura K., Zehr J. P., Follows M. J. (2024). Metabolic trade-offs constrain the cell size ratio in a nitrogen-fixing symbiosis. Cell 187, 1762–1768. doi: 10.1016/j.cell.2024.02.016
Cornejo-Castillo F. M., Muñoz-Marín M. D. C., Turk-Kubo K. A., Royo-Llonch M., Farnelid H., Acinas S. G., et al. (2019). UCYN-A3, a newly characterized open ocean sublineage of the symbiotic N2-fixing cyanobacterium Candidatus Atelocyanobacterium thalassa. Environ. Microbiol. 21, 111–124. doi: 10.1111/1462-2920.14429
Cross K. L., Campbell J. H., Balachandran M., Campbell A. G., Cooper C. J., Griffen A., et al. (2019). Targeted isolation and cultivation of uncultivated bacteria by reverse genomics. Nat. Biotechnol. 37, 1314–1321. doi: 10.1038/s41587-019-0260-6
Dekaezemacker J., Bonnet S. (2011). Sensitivity of N2 fixation to combined nitrogen forms (NO3- and NH4+) in two strains of the marine diazotroph Crocosphaera watsonii (Cyanobacteria). Mar. Ecol.-Prog. Ser. 438, 33–46. doi: 10.3354/meps09297
Dekaezemacker J., Bonnet S., Grosso O., Moutin T., Bressac M., Capone D. G. (2013). Evidence of active dinitrogen fixation in surface waters of the eastern tropical South Pacific during El Niño and La Niña events and evaluation of its potential nutrient controls. Glob. Biogeochem. Cycle 27, 768–779. doi: 10.1002/gbc.20063
Delmont T. O. (2021). Discovery of nondiazotrophic Trichodesmium species abundant and widespread in the open ocean. Proc. Natl. Acad. Sci. U. S. A. 118, e2112355118. doi: 10.1073/pnas.2112355118
Delmont T. O., Pierella Karlusich J. J., Veseli I., Fuessel J., Eren A. M., Foster R. A., et al. (2022). Heterotrophic bacterial diazotrophs are more abundant than their cyanobacterial counterparts in metagenomes covering most of the sunlit ocean. ISME J. 16, 927–936. doi: 10.1038/s41396-021-01135-1
Delmont T. O., Quince C., Shaiber A., Esen Ö.C., Lee S. T. M., Rappé M. S., et al. (2018). Nitrogen-fixing populations of Planctomycetes and Proteobacteria are abundant in surface ocean metagenomes. Nat. Microbiol. 3, 804–813. doi: 10.1038/s41564-018-0176-9
Deng L., Cheung S., Kang C., Liu K., Xia X., Liu H. (2022). Elevated temperature relieves phosphorus limitation of marine unicellular diazotrophic cyanobacteria. Limnol. Oceanogr. 67, 122–134. doi: 10.1002/lno.11980
Deutsch C., Inomura K., Luo Y., Wang Y. (2024). Projecting global biological N2 fixation under climate warming across land and ocean. Trends Microbiol. 32 (6), 546–553. doi: 10.1016/j.tim.2023.12.007
Deutsch C., Sarmiento J. L., Sigman D. M., Gruber N., Dunne J. P. (2007). Spatial coupling of nitrogen inputs and losses in the ocean. Nature 445, 163–167. doi: 10.1038/nature05392
Dugdale R. C., Menzel D. W., Ryther J. H. (1961). Nitrogen fixation in the sargasso sea. Deep-Sea Res. 7, 297–300. doi: 10.1016/0146-6313(61)90051-X
Duhamel S., Diaz J. M., Adams J. C., Djaoudi K., Steck V., Waggoner E. M. (2021). Phosphorus as an integral component of global marine biogeochemistry. Nat. Geosci. 14, 359–368. doi: 10.1038/s41561-021-00755-8
Dyhrman S. T., Chappell P. D., Haley S. T., Moffett J. W., Orchard E. D., Waterbury J. B., et al. (2006). Phosphonate utilization by the globally important marine diazotroph Trichodesmium. Nature 439, 68–71. doi: 10.1038/nature04203
Dyhrman S. T., Haley S. T. (2006). Phosphorus scavenging in the unicellular marine diazotroph crocosphaera watsonii. Appl. Environ. Microbiol. 72, 1452–1458. doi: 10.1128/AEM.72.2.1452-1458.2006
Edgcomb V. P., Taylor C., Pachiadaki M. G., Honjo S., Engstrom I., Yakimov M. (2016). Comparison of Niskin vs. in situ approaches for analysis of gene expression in deep Mediterranean Sea water samples. Deep Sea Res. Part II: Topical Stud. Oceanogr 129, 213–222. doi: 10.1016/j.dsr2.2014.10.020
Eichner M., Basu S., Gledhill M., de Beer D., Shaked Y. (2019). Hydrogen dynamics in trichodesmium colonies and their potential role in mineral iron acquisition. Front. Microbiol. 10. doi: 10.3389/fmicb.2019.01565
Eichner M., Inomura K., Pierella Karlusich J. J., Shaked Y. (2023). Better together? Lessons on sociality from Trichodesmium. Trends Microbiol. 31, 1072–1084. doi: 10.1016/j.tim.2023.05.001
Eichner M., Kranz S. A., Rost B. (2014). Combined effects of different CO2 levels and N sources on the diazotrophic cyanobacterium Trichodesmium. Physiologia Plantarum 152, 316–330. doi: 10.1111/ppl.12172
Falkowski P. G. (1983). “Enzymology of nitrogen assimilation,” in Nitrogen in the Marine Environment. Ed. Capone E. (Academic Press, New York: NY), 839–868.
Falkowski P. G., Barber R. T., Smetacek V. (1998). Biogeochemical controls and feedbacks on ocean primary production. Science 281, 200–206. doi: 10.1126/science.281.5374.200
Farnelid H., Harder J., Bentzon-Tilia M., Riemann L. (2014). Isolation of heterotrophic diazotrophic bacteria from estuarine surface waters. Environ. Microbiol. 16, 3072–3082. doi: 10.1111/1462-2920.12335
Filella A., Riemann L., Van Wambeke F., Pulido-Villena E., Vogts A., Bonnet S., et al. (2022). Contrasting roles of DOP as a source of phosphorus and energy for marine diazotrophs. Front. Mar. Sci. 9. doi: 10.3389/fmars.2022.923765
Frischkorn K. R., Haley S. T., Dyhrman S. T. (2019). Transcriptional and proteomic choreography under phosphorus deficiency and re-supply in the N2 fixing cyanobacterium Trichodesmium erythraeum. Front. Microbiol. 10. doi: 10.3389/fmicb.2019.00330
Frischkorn K. R., Rouco M., Van Mooy B. A. S., Dyhrman S. T. (2017). Epibionts dominate metabolic functional potential of Trichodesmium colonies from the oligotrophic ocean. ISME J. 11, 2090–2101. doi: 10.1038/ismej.2017.74
Fu F.-X., Mulholland M. R., Garcia N. S., Beck A., Bernhardt P. W., Warner M. E., et al. (2008). Interactions between changing pCO2, N2 fixation, and Fe limitation in the marine unicellular cyanobacterium Crocosphaera. Limnol. Oceanogr. 53, 2472–2484. doi: 10.4319/lo.2008.53.6.2472
Fu F.-X., Yu E., Garcia N. S., Gale J., Luo Y., Webb E. A., et al. (2014). Differing responses of marine N2 fixers to warming and consequences for future diazotroph community structure. Aquat. Microb. Ecol. 72, 33–46. doi: 10.3354/ame01683
Fu F.-X., Zhang Y., Bell P. R. F., Hutchins D. A. (2005). Phosphate uptake and growth kinetics of Trichodesmium (cyanobacteria) isolates from the North Atlantic Ocean and the Great Barrier Reef, Australia. J. Phycol. 41, 62–73. doi: 10.1111/j.1529-8817.2005.04063.x
Garcia N. S., Fu F.-X., Sedwick P. N., Hutchins D. A. (2015). Iron deficiency increases growth and nitrogen-fixation rates of phosphorus-deficient marine cyanobacteria. ISME J. 9, 238–245. doi: 10.1038/ismej.2014.104
Garcia N. S., Hutchins D. A. (2014). Light-limited growth rate modulates nitrate inhibition of dinitrogen fixation in the marine unicellular cyanobacterium Crocosphaera watsonii. PloS One 9, e0114465. doi: 10.1371/journal.pone.0114465
Geisler E., Siebner H., Rahav E., Bar-Zeev E. (2023). Quantification of aquatic unicellular diazotrophs by immunolabeled flow cytometry. Biogeochemistry 164, 509–520. doi: 10.1007/s10533-023-01025-y
Glibert P. M. (2024). “Phosphorus Uptake, Assimilation, and Metabolism: Making Energy and Genetic Material,” in Phytoplankton Whispering: An Introduction to the Physiology and Ecology of Microalgae (Springer International Publishing, Cham), 297–311.
Glibert P. M., Wilkerson F. P., Dugdale R. C., Raven J. A., Dupont C. L., Leavitt P. R., et al. (2016). Pluses and minuses of ammonium and nitrate uptake and assimilation by phytoplankton and implications for productivity and community composition, with emphasis on nitrogen-enriched conditions. Limnol. Oceanogr. 61, 165–197. doi: 10.1002/lno.10203
Grabowski M. N. W., Church M. J., Karl D. M. (2008). Nitrogen fixation rates and controls at Stn ALOHA. Aquat. Microb. Ecol. 52, 175–183. doi: 10.3354/ame01209
Großkopf T., LaRoche J. (2012). Direct and indirect costs of dinitrogen fixation in Crocosphaera watsonii WH8501 and possible implications for the nitrogen cycle. Front. Microbiol. 3. doi: 10.3389/fmicb.2012.00236
Großkopf T., Mohr W., Baustian T., Schunck H., Gill D., Kuypers M. M. M., et al. (2012). Doubling of marine dinitrogen-fixation rates based on direct measurements. Nature 488, 361–364. doi: 10.1038/nature11338
Grosse J., van Breugel P., Brussaard C. P. D., Boschker H. T. S. (2017). A biosynthesis view on nutrient stress in coastal phytoplankton. Limnol. Oceanogr. 62, 490–506. doi: 10.1002/lno.10439
Gruber N. (2016). Elusive marine nitrogen fixation. Proc. Natl. Acad. Sci. U. S. A. 113, 4246–4248. doi: 10.1073/pnas.1603646113
Haynes S. J., Darnajoux R., Han E., Oleynik S., Zimble E., Zhang X. (2022). Quantification of biological nitrogen fixation by Mo-independent complementary nitrogenases in environmental samples with low nitrogen fixation activity. Sci. Rep. 12, 22011. doi: 10.1038/s41598-022-24860-9
Held N. A., Webb E. A., McIlvin M. M., Hutchins D. A., Cohen N. R., Moran D. M., et al. (2020). Co-occurrence of Fe and P stress in natural populations of the marine diazotroph Trichodesmium. Biogeosciences 17, 2537–2551. doi: 10.5194/bg-17-2537-2020
Holl C. M., Montoya J. P. (2005). Interactions between nitrate uptake and nitrogen fixation in continuous cultures of the marine diazotroph Trichodesmium (Cyanobacteria). J. Phycol. 41, 1178–1183. doi: 10.1111/j.1529-8817.2005.00146.x
Hong H., Shen R., Zhang F., Wen Z., Chang S. A., Lin W., et al. (2017). The complex effects of ocean acidification on the prominent N2-fixing cyanobacterium Trichodesmium. Science 356, 527–531. doi: 10.1126/science.aal2981
Hutchins D. A., Capone D. G. (2022). The marine nitrogen cycle: new developments and global change. Nat. Rev. Microbiol. 20, 401–414. doi: 10.1038/s41579-022-00687-z
Hutchins D. A., Fu F.-X., Zhang Y., Warner M. E., Feng Y., Portune K., et al. (2007). CO2 control of Trichodesmium N2 fixation, photosynthesis, growth rates, and elemental ratios: Implications for past, present, and future ocean biogeochemistry. Limnol. Oceanogr. 52, 1293–1304. doi: 10.4319/lo.2007.52.4.1293
Hutchins D. A., Tagliabue A. (2024). Feedbacks between phytoplankton and nutrient cycles in a warming ocean. Nat. Geosci. 17, 495–502. doi: 10.1038/s41561-024-01454-w
Jacq V., Ridame C., L’Helguen S., Kaczmar F., Saliot A. (2014). Response of the unicellular diazotrophic cyanobacterium Crocosphaera watsonii to iron limitation. PloS One 9, e86749. doi: 10.1371/journal.pone.0086749
Jiang H., Hutchins D. A., Zhang H., Feng Y., Zhang R., Sun W., et al. (2024). Complexities of regulating climate by promoting marine primary production with ocean iron fertilization. Earth-Sci. Rev. 249, 104675. doi: 10.1016/j.earscirev.2024.104675
Kapili B. J., Barnett S. E., Buckley D. H., Dekas A. E. (2020). Evidence for phylogenetically and catabolically diverse active diazotrophs in deep-sea sediment. ISME J. 14, 971–983. doi: 10.1038/s41396-019-0584-8
Karl D. M. (2014). Microbially mediated transformations of phosphorus in the sea: new views of an old cycle. Annu. Rev. Mar. Sci. 6, 279–337. doi: 10.1146/annurev-marine-010213-135046
Karl D. M., Björkman K. M. (2015). “Chapter 5 - dynamics of dissolved organic phosphorus,” in Biogeochemistry of Marine Dissolved Organic Matter, 2nd ed. Eds. Hansell D. A., Carlson C. A. (Academic Press, Boston), 233–334.
Karl D. M., Letelier R., Hebel D. V., Bird D. F., Winn C. D. (1992). “Trichodesmium Blooms and New Nitrogen in the North Pacific Gyre,” in Marine Pelagic Cyanobacteria: Trichodesmium and other Diazotrophs. Eds. Carpenter E. J., Capone D. G., Rueter J. G. (Springer Netherlands, Dordrecht), 219–237.
Karl D. M., Michaels A. F., Bergman B., Capone D. G., Carpenter E. J., Letelier R. M., et al. (2002). “Dinitrogen fixation in the world’s oceans,” in The Nitrogen Cycle at Regional to Global Scales. Eds. Boyer E. W., Howarth R. W. (Springer Netherlands, Dordrecht), 47–98.
Katye E. A., Sarah E. F., Meredith G. H. (2021). Reactive nitrogen cycling in the atmosphere and ocean. Annu. Rev. Earth Planet. Sci. 49, 523–550. doi: 10.1146/annurev-earth-083120-052147
Kittu L. R., Paul A. J., Fernández-Méndez M., Hopwood M. J., Riebesell U. (2023). Coastal N2 fixation rates coincide spatially with nitrogen loss in the humboldt upwelling system off Peru. Glob. Biogeochem. Cycle 37, e2022GB007578. doi: 10.1029/2022GB007578
Klawonn I., Eichner M. J., Wilson S. T., Moradi N., Thamdrup B., Kümmel S., et al. (2020). Distinct nitrogen cycling and steep chemical gradients in Trichodesmium colonies. ISME J. 14, 399–412. doi: 10.1038/s41396-019-0514-9
Klawonn I., Lavik G., Böning P., Marchant H. K., Dekaezemacker J., Mohr W., et al. (2015). Simple approach for the preparation of 15N2-enriched water for nitrogen fixation assessments: evaluation, application and recommendations. Front. Microbiol. 6. doi: 10.3389/fmicb.2015.00769
Knapp A. N. (2012). The sensitivity of marine N2 fixation to dissolved inorganic nitrogen. Front. Microbiol. 3. doi: 10.3389/fmicb.2012.00374
Knapp A. N., Dekaezemacker J., Bonnet S., Sohm J. A., Capone D. G. (2012). Sensitivity of Trichodesmium erythraeum and Crocosphaera watsonii abundance and N2 fixation rates to varying NO3- and PO43- concentrations in batch cultures. Aquat. Microb. Ecol. 66, 223–236. doi: 10.3354/ame01577
Krupke A., Mohr W., LaRoche J., Fuchs B. M., Amann R. I., Kuypers M. M. M. (2015). The effect of nutrients on carbon and nitrogen fixation by the UCYN-A/haptophyte symbiosis. ISME J. 9, 1635–1647. doi: 10.1038/ismej.2014.253
Küpper H., Šetlík I., Seibert S., Prášil O., Šetlikova E., Strittmatter M., et al. (2008). Iron limitation in the marine cyanobacterium Trichodesmium reveals new insights into regulation of photosynthesis and nitrogen fixation. New Phytol. 179, 784–798. doi: 10.1111/j.1469-8137.2008.02497.x
Kusano M., Fukushima A., Redestig H., Saito K. (2011). Metabolomic approaches toward understanding nitrogen metabolism in plants. J. Exp. Bot. 62, 1439–1453. doi: 10.1093/jxb/erq417
Kustka A. B., Sañudo-Wilhelmy S. A., Carpenter E. J., Capone D. G., Burns J. A., Sunda W. G. (2003). Iron requirements for dinitrogen- and ammonium-supported growth in cultures of Trichodesmium (IMS 101): Comparison with nitrogen fixation rates and iron: carbon ratios of field populations. Limnol. Oceanogr. 48, 1869–1884. doi: 10.4319/lo.2003.48.5.1869
Langlois R. J., Mills M. M., Ridame C., Croot P., LaRoche J. (2012). Diazotrophic bacteria respond to Saharan dust additions. Mar. Ecol.-Prog. Ser. 470, 1–14. doi: 10.3354/meps10109
Lee K. S., Palatinszky M., Pereira F. C., Nguyen J., Fernandez V. I., Mueller A. J., et al. (2019). An automated Raman-based platform for the sorting of live cells by functional properties. Nat. Microbiol. 4, 1035–1048. doi: 10.1038/s41564-019-0394-9
Lee M. D., Walworth N. G., McParland E. L., Fu F.-X., Mincer T. J., Levine N. M., et al. (2017). The Trichodesmium consortium: conserved heterotrophic co-occurrence and genomic signatures of potential interactions. ISME J. 11, 1813–1824. doi: 10.1038/ismej.2017.49
Lenes J. M., Darrow B. P., Cattrall C., Heil C. A., Callahan M., Vargo G. A., et al. (2001). Iron fertilization and the Trichodesmium response on the West Florida shelf. Limnol. Oceanogr. 46, 1261–1277. doi: 10.4319/lo.2001.46.6.1261
Lewis W. H., Tahon G., Geesink P., Sousa D. Z., Ettema T. J. G. (2021). Innovations to culturing the uncultured microbial majority. Nat. Rev. Microbiol. 19, 225–240. doi: 10.1038/s41579-020-00458-8
Li X., Fonseca-Batista D., Roevros N., Dehairs F., Chou L. (2018). Environmental and nutrient controls of marine nitrogen fixation. Prog. Oceanogr. 167, 125–137. doi: 10.1016/j.pocean.2018.08.001
Liu S., Moon C. D., Zheng N., Huws S., Zhao S., Wang J. (2022). Opportunities and challenges of using metagenomic data to bring uncultured microbes into cultivation. Microbiome 10, 76. doi: 10.1186/s40168-022-01272-5
Liu J., Zhou L., Ke Z., Li G., Tan Y. (2020). Phosphorus deficiency induced by aluminum in a marine nitrogen-fixing cyanobacterium Crocosphaera watsonii WH0003. Chemosphere 246, 125641. doi: 10.1016/j.chemosphere.2019.125641
Lory C., Van Wambeke F., Fourquez M., Barani A., Guieu C., Tilliette C., et al. (2022). Assessing the contribution of diazotrophs to microbial Fe uptake using a group specific approach in the Western Tropical South Pacific Ocean. ISME Commun. 2, 41. doi: 10.1038/s43705-022-00122-7
Louchard D., Münnich M., Gruber N. (2023). On the role of the amazon river for N2 fixation in the western tropical atlantic. Glob. Biogeochem. Cycle 37, e2022GB007537. doi: 10.1029/2022GB007537
Ludwig C. A. (1938). The availability of different forms of nitrogen to a green alga. Am. J. Bot. 25, 448–458. doi: 10.1002/j.1537-2197.1938.tb09243.x
Mak E. W. K., Turk-Kubo K. A., Caron D. A., Harbeitner R. C., Magasin J. D., Coale T. H., et al. (2024). Phagotrophy in the nitrogen-fixing haptophyte. Environ. Microbiol. Rep. 16, e13312. doi: 10.1111/1758-2229.13312
Mareš J., Johansen J. R., Hauer T., Zima J. Jr., Ventura S., Cuzman O., et al. (2019). Taxonomic resolution of the genus Cyanothece (Chroococcales, Cyanobacteria), with a treatment on Gloeothece and three new genera, Crocosphaera, Rippkaea, and Zehria. J. Phycol. 55, 578–610. doi: 10.1111/jpy.12853
Marki A., Fischer R., Browning T. J., Louropoulou E., Ptáčník R., Gledhill M. (2020). Stoichiometry of Fe, Mn and Co in the marine diazotroph Crocosphaera subtropica ATCC51142 in Fe- and P-limited continuous cultures. Mar. Ecol.-Prog. Ser. 656, 19–33. doi: 10.3354/meps13523
Martínez-Pérez C., Mohr W., Löscher C. R., Dekaezemacker J., Littmann S., Yilmaz P., et al. (2016). The small unicellular diazotrophic symbiont, UCYN-A, is a key player in the marine nitrogen cycle. Nat. Microbiol. 1, 16163. doi: 10.1038/nmicrobiol.2016.163
Martínez-Pérez C., Mohr W., Schwedt A., Dürschlag J., Callbeck C. M., Schunck H., et al. (2018). Metabolic versatility of a novel N2-fixing Alphaproteobacterium isolated from a marine oxygen minimum zone. Environ. Microbiol. 20, 755–768. doi: 10.1111/1462-2920.14008
Martiny A. C., Lomas M. W., Fu W., Boyd P. W., Chen Y. L., Cutter G. A., et al. (2019). Biogeochemical controls of surface ocean phosphate. Sci. Adv. 5, eaax0341. doi: 10.1126/sciadv.aax0341
Massana R. (2024). The nitroplast: A nitrogen-fixing organelle. Science 384, 160–161. doi: 10.1126/science.ado8571
Masuda T., Furuya K., Kodama T., Takeda S., Harrison P. J. (2013). Ammonium uptake and dinitrogen fixation by the unicellular nanocyanobacterium Crocosphaera watsonii in nitrogen-limited continuous cultures. Limnol. Oceanogr. 58, 2029–2036. doi: 10.4319/lo.2013.58.6.2029
Masuda T., Inomura K., Kodama T., Shiozaki T., Kitajima S., Armin G., et al. (2022). Crocosphaera as a major consumer of fixed nitrogen. Microbiol. Spectr. 10, e0217721. doi: 10.1128/spectrum.02177-21
Masuda T., Mareš J., Shiozaki T., Inomura K., Fujiwara A., Prášil O. (2024). Crocosphaera watsonii – A widespread nitrogen-fixing unicellular marine cyanobacterium. J. Phycol. 60, 604–620. doi: 10.1111/jpy.13450
Mériguet Z., Vilain M., Baudena A., Tilliette C., Habasque J., Lebourges-Dhaussy A., et al. (2023). Plankton community structure in response to hydrothermal iron inputs along the Tonga-Kermadec arc. Front. Mar. Sci. 10. doi: 10.3389/fmars.2023.1232923
Mills M. M., Turk-Kubo K. A., van Dijken G. L., Henke B. A., Harding K. J., Wilson S. T., et al. (2020). Unusual marine cyanobacteria/haptophyte symbiosis relies on N2 fixation even in N-rich environments. ISME J. 14, 2395–2406. doi: 10.1038/s41396-020-0691-6
Mohr W., Grosskopf T., Wallace D. W., LaRoche J. (2010). Methodological underestimation of oceanic nitrogen fixation rates. PloS One 5, e12583. doi: 10.1371/journal.pone.0012583
Mohr W., Lehnen N., Ahmerkamp S., Marchant H. K., Graf J. S., Tschitschko B., et al. (2021). Terrestrial-type nitrogen-fixing symbiosis between seagrass and a marine bacterium. Nature 600, 105–109. doi: 10.1038/s41586-021-04063-4
Moisander P. H., Beinart R. A., Hewson I., White A. E., Johnson K. S., Carlson C. A., et al. (2010). Unicellular cyanobacterial distributions broaden the oceanic N2 fixation domain. Science 327, 1512–1514. doi: 10.1126/science.1185468
Moisander P. H., Benavides M., Bonnet S., Berman-Frank I., White A. E., Riemann L. (2017). Chasing after non-cyanobacterial nitrogen fixation in marine pelagic environments. Front. Microbiol. 8. doi: 10.3389/fmicb.2017.01736
Moisander P. H., Zhang R., Boyle E. A., Hewson I., Montoya J. P., Zehr J. P. (2012). Analogous nutrient limitations in unicellular diazotrophs and Prochlorococcus in the South Pacific Ocean. ISME J. 6, 733–744. doi: 10.1038/ismej.2011.152
Moore C. M., Mills M. M., Achterberg E. P., Geider R. J., LaRoche J., Lucas M. I., et al. (2009). Large-scale distribution of Atlantic nitrogen fixation controlled by iron availability. Nat. Geosci. 2, 867–871. doi: 10.1038/ngeo667
Moore C. M., Mills M. M., Arrigo K. R., Berman-Frank I., Bopp L., Boyd P. W., et al. (2013). Processes and patterns of oceanic nutrient limitation. Nat. Geosci. 6, 701–710. doi: 10.1038/ngeo1765
Moutin T., Broeck N. V. D., Beker B., Dupouy C., Rimmelin P., Bouteiller A. L. (2005). Phosphate availability controls Trichodesmium spp. biomass in the SW Pacific Ocean. Mar. Ecol.-Prog. Ser. 297, 15–21. doi: 10.3354/meps297015
Mulholland M. R., Bronk D. A., Capone D. G. (2004). Dinitrogen fixation and release of ammonium and dissolved organic nitrogen by Trichodesmium IMS101. Aquat. Microb. Ecol. 37, 85–94. doi: 10.3354/ame037085
Mulholland M. R., Capone D. G. (1999). Nitrogen fixation, uptake and metabolism in natural and cultured populations of Trichodesmium spp. Mar. Ecol.-Prog. Ser. 188, 33–49. doi: 10.3354/meps188033
Mulholland M. R., Floge S., Carpenter E. J., Capone D. G. (2002). Phosphorus dynamics in cultures and natural populations of Trichodesmium spp. Mar. Ecol.-Prog. Ser. 239, 45–55. doi: 10.3354/meps239045
Mulholland M. R., Ohki K., Capone D. G. (1999). Nitrogen utilization and metabolism relative to patterns of N2 fixation in cultures of Trichodesmium NIBB1067. J. Phycol. 35, 977–988. doi: 10.1046/j.1529-8817.1999.3550977.x
Mulholland M. R., Ohki K., Capone D. G. (2001). Nutrient controls on nitrogen uptake and metabolism by natural populations and cultures of Trichodesmium (Cyanobacteria). J. Phycol. 37, 1001–1009. doi: 10.1046/j.1529-8817.2001.00080.x
Ohki K., Zehr J. P., Falkowski P. G., Fujita Y. (1991). ). Regulation of nitrogen-fixation by different nitrogen sources in the marine non-heterocystous cyanobacterium Trichodesmium sp. NIBB1067. Arch. Microbiol. 156, 335–337. doi: 10.1007/BF00248706
Orchard E. D. (2010). Phosphorus physiology of the marine cyanobacterium Trichodesmium (United States – Massachusetts: Massachusetts Institute of Technology). Doctoral thesis.
Orchard E. D., Ammerman J. W., Lomas M. W., Dyhrman S. T. (2010a). Dissolved inorganic and organic phosphorus uptake in Trichodesmium and the microbial community: The importance of phosphorus ester in the Sargasso Sea. Limnol. Oceanogr. 55, 1390–1399. doi: 10.4319/lo.2010.55.3.1390
Orchard E. D., Benitez-Nelson C. R., Pellechia P. J., Lomas M. W., Dyhrman S. T. (2010b). Polyphosphate in Trichodesmium from the low-phosphorus sargasso sea. Limnol. Oceanogr. 55, 2161–2169. doi: 10.4319/lo.2010.55.5.2161
Orchard E. D., Webb E. A., Dyhrman S. T. (2009). Molecular analysis of the phosphorus starvation response in Trichodesmium spp. Environ. Microbiol. 11, 2400–2411. doi: 10.1111/j.1462-2920.2009.01968.x
Orcutt K. M., Lipschultz F., Gundersen K., Arimoto R., Michaels A. F., Knap A. H., et al. (2001). A seasonal study of the significance of N2 fixation by Trichodesmium spp. at the Bermuda Atlantic Time-series Study (BATS) site. Deep Sea Res. Part II: Topical Stud. Oceanogr. 48, 1583–1608. doi: 10.1016/S0967-0645(00)00157-0
Paerl H. W., Prufertbebout L. E., Guo C. Z. (1994). Iron-stimulated N2 fixation and growth in natural and cultured populations of the planktonic marine cyanobacteria Trichodesmium spp. Appl. Environ. Microbiol. 60, 1044–1047. doi: 10.1128/aem.60.3.1044-1047.1994
Pereira N., Shilova I. N., Zehr J. P. (2016). Molecular markers define progressing stages of phosphorus limitation in the nitrogen-fixing cyanobacterium, Crocosphaera. J. Phycol. 52, 274–282. doi: 10.1111/jpy.12396
Pereira N., Shilova I. N., Zehr J. P. (2019). Use of the high-affinity phosphate transporter gene, pstS, as an indicator for phosphorus stress in the marine diazotroph Crocosphaera watsonii (Chroococcales, Cyanobacteria). J. Phycol. 55, 752–761. doi: 10.1111/jpy.12863
Polyviou D., Baylay A. J., Hitchcock A., Robidart J., Moore C. M., Bibby T. S. (2018). Desert dust as a source of iron to the globally important diazotroph Trichodesmium. Front. Microbiol. 8. doi: 10.3389/fmicb.2017.02683
Post A. F., Rihtman B., Wang Q. (2012). Decoupling of ammonium regulation and ntcA transcription in the diazotrophic marine cyanobacterium Trichodesmium sp. IMS101. ISME J. 6, 629–637. doi: 10.1038/ismej.2011.121
Qu P., Fu F.-X., Kling J. D., Huh M., Wang X., Hutchins D. A. (2019). Distinct responses of the nitrogen-fixing marine cyanobacterium Trichodesmium to a thermally variable environment as a function of phosphorus availability. Front. Microbiol. 10. doi: 10.3389/fmicb.2019.01282
Rabouille S., Randall B., Talec A., Raimbault P., Blasco T., Latifi A., et al. (2021). Independence of a marine unicellular diazotroph to the presence of NO3–. Microorganisms 9, 2073. doi: 10.3390/microorganisms9102073
Rabouille S., Tournier L., Duhamel S., Claquin P., Crispi O., Talec A., et al. (2022). Organic phosphorus scavenging supports efficient growth of diazotrophic cyanobacteria under phosphate depletion. Front. Microbiol. 13. doi: 10.3389/fmicb.2022.848647
Rahav E., Herut B., Spungin D., Levi A., Mulholland M. R., Berman-Frank I. (2022). Heterotrophic bacteria outcompete diazotrophs for orthophosphate in the Mediterranean Sea. Limnol. Oceanogr. 67, 159–171. doi: 10.1002/lno.11983
Riemann L., Rahav E., Passow U., Grossart H. P., de Beer D., Klawonn I., et al. (2022). Planktonic aggregates as hotspots for heterotrophic diazotrophy: the plot thickens. Front. Microbiol. 13. doi: 10.3389/fmicb.2022.875050
Robidart J. C., Church M. J., Ryan J. P., Ascani F., Wilson S. T., Bombar D., et al. (2014). Ecogenomic sensor reveals controls on N2-fixing microorganisms in the North Pacific Ocean. ISME J. 8, 1175–1185. doi: 10.1038/ismej.2013.244
Roe K. L., Barbeau K. A. (2014). Uptake mechanisms for inorganic iron and ferric citrate in Trichodesmium erythraeum IMS101. Metallomics 6, 2042–2051. doi: 10.1039/c4mt00026a
Roe K. L., Barbeau K. A., Mann E. L., Haygood M. G. (2012). Acquisition of iron by Trichodesmium and associated bacteria in culture. Environ. Microbiol. 14, 1681–1695. doi: 10.1111/j.1462-2920.2011.02653.x
Rose S. A., Robicheau B. M., Tolman J., Fonseca-Batista D., Rowland E., Desai D., et al. (2024). Nitrogen fixation in the widely distributed marine γ-proteobacterial diazotroph Candidatus Thalassolituus haligoni. Sci. Adv. 10, eadn1476. doi: 10.1126/sciadv.adn1476
Rouco M., Frischkorn K. R., Haley S. T., Alexander H., Dyhrman S. T. (2018). Transcriptional patterns identify resource controls on the diazotroph Trichodesmium in the Atlantic and Pacific oceans. ISME J. 12, 1486–1495. doi: 10.1038/s41396-018-0087-z
Rubin M., Berman-Frank I., Shaked Y. (2011). Dust- and mineral-iron utilization by the marine dinitrogen-fixer Trichodesmium. Nat. Geosci. 4, 529–534. doi: 10.1038/ngeo1181
Rueter J. G. (1988). Iron stimulation of photosynthesis and nitrogen fixation in anabaena 7120 and Trichodesmium (cyanophyceae). J. Phycol. 24, 249–254. doi: 10.1111/j.1529-8817.1988.tb04240.x
Ruttenberg K. C. (2014). “10.13 - The global phosphorus cycle,” in Treatise on Geochemistry (Second Edition). eds. Holland H. D., Turekian K. K. (Oxford: Elsevier), 499–588.
Saito M. A., Bertrand E. M., Dutkiewicz S., Bulygin V. V., Moran D. M., Monteiro F. M., et al. (2011). Iron conservation by reduction of metalloenzyme inventories in the marine diazotroph Crocosphaera watsonii. Proc. Natl. Acad. Sci. U. S. A. 108, 2184–2189. doi: 10.1073/pnas.1006943108
Saito M. A., Goepfert T. J., Ritt J. T. (2008). Some thoughts on the concept of colimitation: Three definitions and the importance of bioavailability. Limnol. Oceanogr. 53, 276–290. doi: 10.4319/lo.2008.53.1.0276
Sandh G., Ran L., Xu L., Sundqvist G., Bulone V., Bergman B. (2011). Comparative proteomic profiles of the marine cyanobacterium Trichodesmium erythraeum IMS101 under different nitrogen regimes. Proteomics 11, 406–419. doi: 10.1002/pmic.201000382
Sañudo-Wilhelmy S. A., Kustka A. B., Gobler C. J., Hutchins D. A., Yang M., Lwiza K., et al. (2001). Phosphorus limitation of nitrogen fixation by Trichodesmium in the central Atlantic Ocean. Nature 411, 66–69. doi: 10.1038/35075041
Sañudo-Wilhelmy S. A., Tovar-Sanchez A., Fu F.-X., Capone D. G., Carpenter E. J., Hutchins D. A. (2004). The impact of surface-adsorbed phosphorus on phytoplankton Redfield stoichiometry. Nature 432, 897–901. doi: 10.1038/nature03125
Sanz-Luque E., Bhaya D., Grossman A. R. (2020). Polyphosphate: A multifunctional metabolite in cyanobacteria and algae. Front. Plant Sci. 11. doi: 10.3389/fpls.2020.00938
Scholin C. A., Birch J., Jensen S., Marin Iii R., Massion E., Pargett D., et al. (2017). The quest to develop ecogenomic sensors: a 25-year history of the environmental sample processor (ESP) as a case study. Oceanography 30 (4), 100–113. doi: 10.5670/oceanog.2017.427
Shao Z., Luo Y. (2022). Controlling factors on the global distribution of a representative marine non-cyanobacterial diazotroph phylotype (Gamma A). Biogeosciences 19, 2939–2952. doi: 10.5194/bg-19-2939-2022
Shi D., Kranz S. A., Kim J. M., Morel F. M. (2012). Ocean acidification slows nitrogen fixation and growth in the dominant diazotroph Trichodesmium under low-iron conditions. Proc. Natl. Acad. Sci. U. S. A. 109, E3094–E3100. doi: 10.1073/pnas.1216012109
Shiozaki T., Furuya K., Kodama T., Kitajima S., Takeda S., Takemura T., et al. (2010). New estimation of N2 fixation in the western and central Pacific Ocean and its marginal seas. Glob. Biogeochem. Cycle 24, GB1015. doi: 10.1029/2009GB003620
Shiozaki T., Furuya K., Kodama T., Takeda S. (2009). Contribution of N2 fixation to new production in the western North Pacific Ocean along 155°E. Mar. Ecol.-Prog. Ser. 377, 19–32. doi: 10.3354/meps07837
Shiozaki T., Ijichi M., Kodama T., Takeda S., Furuya K. (2014). Heterotrophic bacteria as major nitrogen fixers in the euphotic zone of the Indian Ocean. Glob. Biogeochem. Cycle 28, 1096–1110. doi: 10.1002/2014GB004886
Simon J., Klotz M. G. (2013). Diversity and evolution of bioenergetic systems involved in microbial nitrogen compound transformations. Biochim. Biophys. Acta Bioenerg. 1827, 114–135. doi: 10.1016/j.bbabio.2012.07.005
Snow J. T., Polyviou D., Skipp P., Chrismas N. A., Hitchcock A., Geider R., et al. (2015). Quantifying integrated proteomic responses to iron stress in the globally important marine diazotroph Trichodesmium. PloS One 10, e0142626. doi: 10.1371/journal.pone.0142626
Sohm J. A., Capone D. G. (2006). Phosphorus dynamics of the tropical and subtropical north Atlantic: Trichodesmium spp. versus bulk plankton. Mar. Ecol.-Prog. Ser. 317, 21–28. doi: 10.3354/meps317021
Sohm J. A., Hilton J. A., Noble A. E., Zehr J. P., Saito M. A., Webb E. A. (2011a). Nitrogen fixation in the south atlantic gyre and the benguela upwelling system. Geophys. Res. Lett. 38, L16608. doi: 10.1029/2011GL048315
Sohm J. A., Mahaffey C., Capone D. G. (2008). Assessment of relative phosphorus limitation of Trichodesmium spp. in the North Pacific, North Atlantic, and the north coast of Australia. Limnol. Oceanogr. 53, 2495–2502. doi: 10.4319/lo.2008.53.6.2495
Sohm J. A., Webb E. A., Capone D. G. (2011b). Emerging patterns of marine nitrogen fixation. Nat. Rev. Microbiol. 9, 499–508. doi: 10.1038/nrmicro2594
Spungin D., Berman-Frank I., Levitan O. (2014). Trichodesmium’s strategies to alleviate phosphorus limitation in the future acidified oceans. Environ. Microbiol. 16, 1935–1947. doi: 10.1111/1462-2920.12424
Stihl A., Sommer U., Post A. F. (2001). Alkaline phosphatase activities among populations of the colony-forming diazotrophic cyanobacterium Trichodesmium spp. (cyanobacteria) in the Red Sea. J. Phycol. 37, 310–317. doi: 10.1046/j.1529-8817.2001.037002310.x
Tagliabue A., Bowie A. R., Boyd P. W., Buck K. N., Johnson K. S., Saito M. A. (2017). The integral role of iron in ocean biogeochemistry. Nature 543, 51–59. doi: 10.1038/nature21058
Tang W., Li Z., Cassar N. (2019). Machine learning estimates of global marine nitrogen fixation. J. Geophys. Res.: Biogeosci. 124, 717–730. doi: 10.1029/2018JG004828
Thomas J. H., Mullineaux P. M., Cronshaw A. D., Chaplin A. E., Gallon J. R. (1982). The effects of structural analogues of amino acids on ammonium assimilation and acetylene reduction (Nitrogen fixation) in Gloeocapsa (Gloeothece) sp. CCAP 1430/3. Microbiology 128, 885–893. doi: 10.1099/00221287-128-4-885
Thompson A., Carter B. J., Turk-Kubo K., Malfatti F., Azam F., Zehr J. P. (2014). Genetic diversity of the unicellular nitrogen-fixing cyanobacteria UCYN-A and its prymnesiophyte host. Environ. Microbiol. 16, 3238–3249. doi: 10.1111/1462-2920.12490
Thompson A. W., Foster R. A., Krupke A., Carter B. J., Musat N., Vaulot D., et al. (2012). Unicellular cyanobacterium symbiotic with a single-celled eukaryotic alga. Science 337, 1546–1550. doi: 10.1126/science.1222700
Thompson A. W., Zehr J. P. (2013). Cellular interactions: lessons from the nitrogen-fixing cyanobacteria. J. Phycol. 49, 1024–1035. doi: 10.1111/jpy.12117
Tripp H. J., Bench S. R., Turk K. A., Foster R. A., Desany B. A., Niazi F., et al. (2010). Metabolic streamlining in an open-ocean nitrogen-fixing cyanobacterium. Nature 464, 90–94. doi: 10.1038/nature08786
Tschitschko B., Esti M., Philippi M., Kidane A. T., Littmann S., Kitzinger K., et al. (2024). Rhizobia–diatom symbiosis fixes missing nitrogen in the ocean. Nature 630, 899–904. doi: 10.1038/s41586-024-07495-w
Tuit C., Waterbury J. B., Ravizza G. (2004). Diel variation of molybdenum and iron in marine diazotrophic cyanobacteria. Limnol. Oceanogr. 49, 978–990. doi: 10.4319/lo.2004.49.4.0978
Turk-Kubo K. A., Achilles K. M., Serros T. R., Ochiai M., Montoya J. P., Zehr J. P. (2012). Nitrogenase (nifH) gene expression in diazotrophic cyanobacteria in the Tropical North Atlantic in response to nutrient amendments. Front. Microbiol. 3. doi: 10.3389/fmicb.2012.00386
Turk-Kubo K. A., Gradoville M. R., Cheung S., Cornejo-Castillo F. M., Harding K. J., Morando M., et al. (2022). Non-cyanobacterial diazotrophs: global diversity, distribution, ecophysiology, and activity in marine waters. FEMS Microbiol. Rev. 47, fuac046. doi: 10.1093/femsre/fuac046
Tyrrell T., Marañón E., Poulton A. J., Bowie A. R., Harbour D. S., Woodward E. M. S. (2003). Large-scale latitudinal distribution of Trichodesmium spp. in the Atlantic Ocean. J. Plankton Res. 25, 405–416. doi: 10.1093/plankt/25.4.405
Tzubari Y., Magnezi L., Be’er A., Berman-Frank I. (2018). Iron and phosphorus deprivation induce sociality in the marine bloom-forming cyanobacterium Trichodesmium. ISME J. 12, 1682–1693. doi: 10.1038/s41396-018-0073-5
Umbricht J., Filella A., Klett A., Vogts A., Benavides M., Voss M. (2024). Uptake of dissolved inorganic nitrogen and N2 fixation by Crocosphaera watsonii under climate change scenarios. Front. Mar. Sci. 11. doi: 10.3389/fmars.2024.1388214
Van Mooy B. A. S., Fredricks H. F., Pedler B. E., Dyhrman S. T., Karl D. M., Koblížek M., et al. (2009). Phytoplankton in the ocean use non-phosphorus lipids in response to phosphorus scarcity. Nature 458 (7234), 69–72. doi: 10.1038/nature07659
Van Mooy B. A. S., Hmelo L. R., Sofen L. E., Campagna S. R., May A. L., Dyhrman S. T., Koblížek M., et al. (2012). Quorum sensing control of phosphorus acquisition in Trichodesmium consortia. ISME J. 6, 422–429. doi: 10.1038/ismej.2011.115
Vieyra-Mexicano C., Souza V., Pajares S. (2024). Distribution of the N2-fixing cyanobacterium Candidatus Atelocyanobacterium thalassa in the Mexican Pacific upwelling system under two contrasting El Niño Southern Oscillation conditions. Environ. Microbiol. Rep. 16, e13237. doi: 10.1111/1758-2229.13237
Walworth N. G., Fu F.-X., Lee M. D., Cai X., Saito M. A., Webb E. A., et al. (2018). Nutrient-colimited Trichodesmium as a nitrogen source or sink in a future ocean. Appl. Environ. Microbiol. 84, e02137–e02117. doi: 10.1128/aem.02137-17
Wang X., Browning T. J., Achterberg E. P., Gledhill M. (2022b). Phosphorus limitation enhances diazotroph zinc quotas. Front. Microbiol. 13. doi: 10.3389/fmicb.2022.853519
Wang S., Koedooder C., Zhang F., Kessler N., Eichner M., Shi D., et al. (2022a). Colonies of the marine cyanobacterium Trichodesmium optimize dust utilization by selective collection and retention of nutrient-rich particles. iScience 25, 103587. doi: 10.1016/j.isci.2021.103587
Webb E. A., Foster R. A., Villareal T. A., Waterbury J. B., Zehr J. P. (2022). “Trichodesmium,” in Bergey’s Manual of Systematics of Archaea and Bacteria, eds. Trujillo M. E., Dedysh S., DeVos P., Hedlund B., Kämpfer P., Rainey F. A., et al. (Hoboken, New Jersey: John Wiley & Sons), 1–12.
Webb E. A., Jakuba R. W., Moffett J. W., Dyhrman S. T. (2007). Molecular assessment of phosphorus and iron physiology in Trichodesmium populations from the western Central and western South Atlantic. Limnol. Oceanogr. 52, 2221–2232. doi: 10.4319/lo.2007.52.5.2221
White A. E., Granger J., Selden C., Gradoville M. R., Potts L., Bourbonnais A., et al. (2020). A critical review of the 15N2 tracer method to measure diazotrophic production in pelagic ecosystems. Limnol. Oceanogr.: Methods 18, 129–147. doi: 10.1002/lom3.10353
White A. E., Spitz Y. H., Karl D. M., Letelier R. M. (2006). Flexible elemental stoichiometry in Trichodesmium spp. and its ecological implications. Limnol. Oceanogr. 51, 1777–1790. doi: 10.4319/lo.2006.51.4.1777
Wu J., Chung S., Wen L., Liu K., Chen Y., Chen H., et al. (2003). Dissolved inorganic phosphorus, dissolved iron, and Trichodesmium in the oligotrophic South China Sea. Glob. Biogeochem. Cycle 17, 1008. doi: 10.1029/2002GB001924
Yamaguchi T., Sato M., Gonda N., Takahashi K., Furuya K. (2020). Phosphate diester utilization by marine diazotrophs Trichodesmium erythraeum and Crocosphaera watsonii. Aquat. Microb. Ecol. 85, 211–218. doi: 10.3354/ame01951
Yang N., Lin Y., Merkel C. A., DeMers M. A., Qu P., Webb E. A., et al. (2022). Molecular mechanisms underlying iron and phosphorus co-limitation responses in the nitrogen-fixing cyanobacterium Crocosphaera. ISME J. 16, 2702–2711. doi: 10.1038/s41396-022-01307-7
Yang N., Merkel C. A., Lin Y., Levine N. M., Hawco N. J., Jiang H., et al. (2021). Warming iron-limited oceans enhance nitrogen fixation and drive biogeographic specialization of the globally important cyanobacterium Crocosphaera. Front. Mar. Sci. 8. doi: 10.3389/fmars.2021.628363
Yao W., Kvale K. F., Koeve W., Landolfi A., Achterberg E. P., Bertrand E. M., et al. (2022). Simulated future trends in marine nitrogen fixation are sensitive to model iron implementation. Glob. Biogeochem. Cycle 36, e2020GB006851. doi: 10.1029/2020GB006851
Yuan Z., Browning T. J., Zhang R., Wang C., Du C., Wang Y., et al. (2023). Potential drivers and consequences of regional phosphate depletion in the western subtropical North Pacific. Limnol. Oceanogr. Lett. 8, 509–518. doi: 10.1002/lol2.10314
Zehr J. P., Capone D. G. (2020). Changing perspectives in marine nitrogen fixation. Science 368, eaay9514. doi: 10.1126/science.aay9514
Zehr J. P., Capone D. G. (2021a). “Biogeography of N2 Fixation in the Surface Ocean,” in Marine Nitrogen Fixation (Springer International Publishing, Cham), 117–141.
Zehr J. P., Capone D. G. (2021b). “Factors Controlling N2 Fixation,” in Marine Nitrogen Fixation (Springer International Publishing, Cham), 95–115.
Zehr J. P., Capone D. G. (2021c). “Fundamentals of N2 Fixation,” in Marine Nitrogen Fixation (Springer International Publishing, Cham), 9–29.
Zehr J. P., Capone D. G. (2021d). “Microorganisms and Habitats,” in Marine Nitrogen Fixation (Springer International Publishing, Cham), 43–61.
Zehr J. P., Capone D. G. (2024). Unsolved mysteries in marine nitrogen fixation. Trends Microbiol. 32 (6), 532–545. doi: 10.1016/j.tim.2023.08.004
Zehr J. P., Foster R. A., Waterbury J. B., Webb E. A. (2022). “Crocosphaera,” in Bergey’s Manual of Systematics of Archaea and Bacteria, eds. Trujillo M. E., Dedysh S., DeVos P., Hedlund B., Kämpfer P., Rainey F. A., et al. (Hoboken, New Jersey: John Wiley & Sons), 1–9.
Zehr J. P., Kudela R. M. (2011). Nitrogen cycle of the open ocean: from genes to ecosystems. Annu. Rev. Mar. Sci. 3, 197–225. doi: 10.1146/annurev-marine-120709-142819
Zehr J. P., Montoya J. P., Jenkins B. D., Hewson I., Mondragon E., Short C. M., et al. (2007). Experiments linking nitrogenase gene expression to nitrogen fixation in the North Pacific subtropical gyre. Limnol. Oceanogr. 52, 169–183. doi: 10.4319/lo.2007.52.1.0169
Zehr J. P., Shilova I. N., Farnelid H. M., Muñoz-Marín M. D. C., Turk-Kubo K. A. (2016). Unusual marine unicellular symbiosis with the nitrogen-fixing cyanobacterium UCYN-A. Nat. Microbiol. 2, 16214. doi: 10.1038/nmicrobiol.2016.214
Zhang F., Hong H., Kranz S. A., Shen R., Lin W., Shi D. (2019). Proteomic responses to ocean acidification of the marine diazotroph Trichodesmium under iron-replete and iron-limited conditions. Photosynth. Res. 142, 17–34. doi: 10.1007/s11120-019-00643-8
Zhang X., Song Y., Liu D., Keesing J. K., Gong J. (2015). Macroalgal blooms favor heterotrophic diazotrophic bacteria in nitrogen-rich and phosphorus-limited coastal surface waters in the Yellow Sea. Estuarine Coast. Shelf Sci. 163, 75–81. doi: 10.1016/j.ecss.2014.12.015
Zhang X., Ward B. B., Sigman D. M. (2020). Global nitrogen cycle: critical enzymes, organisms, and processes for nitrogen budgets and dynamics. Chem. Rev. 120, 5308–5351. doi: 10.1021/acs.chemrev.9b00613
Zhu Z., Fu F.-X., Qu P., Mak E. W. K., Jiang H., Zhang R., et al. (2020). Interactions between ultraviolet radiation exposure and phosphorus limitation in the marine nitrogen-fixing cyanobacteria Trichodesmium and Crocosphaera. Limnol. Oceanogr. 65, 363–376. doi: 10.1002/lno.11304
Keywords: diazotrophs, nutrients, biological nitrogen fixation, total dissolved nitrogen, total dissolved phosphorus, dissolved iron
Citation: Cao W, Yang Q, Ji F and Liu C (2024) Influence of N, P, and Fe availability on Braarudosphaera bigelowii, Trichodesmium, Crocosphaera, and noncyanobacterial diazotrophs: a review. Front. Mar. Sci. 11:1467599. doi: 10.3389/fmars.2024.1467599
Received: 20 July 2024; Accepted: 16 September 2024;
Published: 04 October 2024.
Edited by:
Sophie Rabouille, UMR7621 Laboratoire d’Océanographie Microbienne (LOMIC), FranceReviewed by:
Michael William Lomas, Bigelow Laboratory For Ocean Sciences, United StatesXiaolei Wang, Ocean University of China, China
Copyright © 2024 Cao, Yang, Ji and Liu. This is an open-access article distributed under the terms of the Creative Commons Attribution License (CC BY). The use, distribution or reproduction in other forums is permitted, provided the original author(s) and the copyright owner(s) are credited and that the original publication in this journal is cited, in accordance with accepted academic practice. No use, distribution or reproduction is permitted which does not comply with these terms.
*Correspondence: Qunhui Yang, eWFuZ3FoQHRvbmdqaS5lZHUuY24=