- 1Key Laboratory of Marine Biotechnology of Zhejiang Province, School of Marine Sciences, Ningbo University, Ningbo, Zhejiang, China
- 2College of Life and Environmental Sciences, Huangshan University, Huangshan, China
- 3Eco-Environmental Conservation Research Centre, Huangshan University, Huangshan, China
To investigate the effects of ocean acidification (OA) and nitrogen limitation on macroalgae growth and photophysiological responses, Gracilariopsis lemaneiformis was cultured under two main conditions: ambient (Low CO2, LC, 390 μatm) and CO2 enriched (High CO2, HC, 1000 μatm), with low (LN, 7 μmol L-1) and high (HN, 56 μmol L-1) nitrate. High CO2 levels decreased growth under both LN and HN treatments. HC reduced Chl a, carotenoids, phycoerythrin (PE), and phycocyanin (PC) under HN conditions, while only Chl a decreased under LN conditions. NO3- uptake rate was restricted under LN compared to HN, while HC enhanced it under HN. Net photosynthetic O2 evolution rates did not differ between CO2 and nitrate treatments. Dark respiration rates were higher under HN, further boosted by HC. The stimulated effective quantum yield (Y(II)) corresponded to decreased non-photochemical quenching (NPQ) under HN conditions. Nitrate, not CO2, showed significant effects on the relative electron transport rate (rETRmax), light use efficiency (α) and saturation light intensity (Ik) that with lowered rETRmax and α under LN culture. Our results indicate that OA may negatively affect Gracilariopsis lemaneiformis growth and alter its photophysiological performance under different nutrient conditions.
1 Introduction
As the impact of human activities intensifies, the combustion of fossil fuels and deforestation activities have led to a continuous and rapid increase in atmospheric CO2 concentration. Since the industrial revolution, atmospheric CO2 levels have risen from 280 ppmv to about 422.8 ppmv, as recorded by the Mauna Loa Observatory (MLO) in Hawaii (NOAA) as of January 2024 (www.CO2.earth). If the energy structure remains constant, these levels are projected to keep increasing and expected to reach 800 - 1000 ppmv according to the Intergovernmental Panel on Climate Change report (IPCC, 2021). As the main sink of anthropogenic CO2, the ocean absorbs about 2.5 ± 0.6 Pg C per year according to Global Carbon Budget 2020 by Friedlingstein et al. (2020) which greatly offsets global warming (Häder and Gao, 2023). However, the increase in dissolved CO2 in seawater also brings about changes in the marine carbonate system, mainly reflected by a decrease in seawater pH and carbonate ion concentration (CO32-), and an increase in hydrogen ion (H+), dissolved CO2 (pCO2) and bicarbonate concentration (HCO3-), which is termed as ocean acidification (OA) (Doney et al., 2009). OA as a widespread stressor, has attracted significant attention in recent years and has been suggested to affect marine organisms differently owing to variations in their sensitivity, leading to significant ecological changes (Kroeker et al., 2013). Recent studies have further shown that the ecological effects of OA are not limited to the individual organisms, affecting both the broader community and the entire marine ecosystem (Guinotte and Fabry, 2008; Riebesell and Gattuso, 2015; Nagelkerken and Connell, 2022).
Macroalgae are widely distributed in the intertidal zone and play an important role in carbon flux and sequestration, biogeochemical cycles, and the stability of coastal ecosystems (Falkowski and Raven, 2013; Zhang et al., 2017). Anthropogenic CO2 dissolution induced OA may superimpose a lower pH caused by coastal eutrophication on a more acidic condition (Cai et al., 2011; Capone and Hutchins, 2013), which means that physical and chemical environment of aquaculture water will change both globally and regionally. Changing seawater carbonate composition may have divergent effects on marine macroalgae inhabiting in different intertidal zones, because the utilization mode and capacity of dissolved inorganic carbon (DIC) are significantly different (Giordano et al., 2005; Raven et al., 2008). Most macroalgae can use both CO2 and HCO3- to counteract the photosynthetic substrate limitation of lowered dissolved CO2 in seawater (Hepburn et al., 2011), and possess different CO2 concentration mechanisms (CCMs) to overcome the reliance on a lowered rate of HCO3- dehydration to CO2 by using intracellular and/or extracellular carbonic anhydrase (Beer et al., 2002; Dudgeon and Kübler, 2020). Therefore, enhanced pCO2 and HCO3- may benefit species with lowered CCM capacity, however the neutral and negative effects have also been observed, which are sometimes regulated by other co-varied environmental factors, such as light intensity, nutrient status and temperature (Raven et al., 2011; Celis-Plá et al., 2015; Yang et al., 2021; Zhou et al., 2022). An in-situ study of the ocean natural CO2 gradients (CO2 seeps) has proven that high CO2/low pH will disproportionately changes the species abundance of macroalgae and induces the shifts in community structure, subsequently leading to altered coastal habitats and ecosystem services (Hall-Spencer and Harvey, 2019).
In recent years, numerous studies have focused on the response of marine macroalgae to OA, which has greatly improved our understanding of the biological repercussions of OA (Koch et al., 2013). However, insufficient attention has been paid to non-calcareous species, particularly fleshy species, which are of significant ecological and economic value in coastal aquaculture. Among the OA-related studies on macroalgae, only a few have focused on photosynthesis and the physiology of aquaculture species (Tan et al., 2023). Several studies have shown that the effects of OA on marine organisms can be regulated by other covariate environmental drivers (Riebesell and Gattuso, 2015; Häder and Gao, 2023). Considering the multiple co-varied environmental factors in the context of climate change and local variations, the effect of OA in synergy with these environmental drivers on the growth, photosynthesis, yield, and quality of aquaculture species in coastal areas remains relatively unexplored.
Nutrient status is more diverse in coastal areas and aquaculture ponds due to global changes and aquaculture activity. Seawater warming may lead to enhanced stratification and a shallower upper mixed layer, which limits nutrient mixing from the bottom (Hutchins and Fu, 2017). In the context of global change, algal blooms caused by microalgae and macroalgae (green and golden tides) frequently occur in coastal waters, which also induce nutrient limitations and negatively affect nutrient availability (Feng et al., 2024). Therefore, the growth and productivity of seaweed in mariculture systems may be significantly affected by nutrient deficiency. Nitrogen limitation as a result of high biomass density cultures has been suggested to negatively influence the productivity of G. lemaneiformis, and seasonal fluctuations in N supplementation may further aggravate it, leading to a disconnection between the culture and the availability of dissolved nutrients in the seawater (Du et al., 2013; Martín et al., 2013). The marine macroalga Gracilariopsis lemaneiformis grown under nitrogen-limited conditions, exhibits significantly decreased growth and subsequently induces disease outbreaks (Wang et al., 2018). Variations in nutrient supply have significant implications for the phenotype, production, and quality of macroalgal cultivation (Celis-Plá et al., 2015; Ober and Thornber, 2017; Bao et al., 2019; Zhou et al., 2022; Li P. et al., 2023; Li T. et al., 2023).
Gracilariopsis lemaneiformis, a red alga (Rhodophyta) with high ecological and economic value, is widely recognized for its high nutritional value as a food or the main feed source for aquaculture animals (Zou and Gao, 2013). In addition, it serves as an important raw material in the agar industry owing to its high agar content (McHugh, 1991). Consequently, the yield of G. lemaneiformis cultivated offshore in China has been increasing annually, and some varieties have special characteristics such as high temperature resistance, high productivity, and high concentration of agar (Meng et al., 2009; Lu et al., 2012; Wang et al., 2016). However, considering the anticipated future ocean changes in coastal areas, understanding how G. lemaneiformis will respond to OA at different nitrate levels is of interest and requires further research. In this study, G. lemaneiformis was used to investigate the effects of OA on its growth, pigmentation, and photosynthetic performance at low and high nitrate concentrations. The findings of this study offer fundamental insights and theoretical guidance for the cultivation of this algal species in different nutrient environments under conditions of seawater acidification.
2 Materials and methods
2.1 Algae culture condition
Gracilariopsis lemaneiformis thalli was collected from Nanao Island of Guangzhou Province, China, and was pre-cultured in laboratory condition with seawater collected from Southern China Sea of Southeast Asia Time-series Station (SEATS) (salinity 33 ppt, with almost undetectable phosphate and nitrate concentration and can be used as background nutrients) (Lu et al., 2020) enriched with Aquil medium (Morel et al., 1979) at 20°C and irradiance with fluorescent tube under 100 μmol m-2s-1 (L:D cycle of 12:12). For the experimental setup, thalli of ca. 0.1g in a 500 mL balloon flask were continuous bubbled with low (LC, 390 μatm) and high CO2 (HC, 1000 μatm) air at flow rate of ca. 300 mL min-1 in respective CO2 chamber (HP1000G-D, Ruihua, China) under modified Aquil Medium (Morel et al., 1979) that with low (ca. 7 μmol L-1) and high (ca, 56 μmol L-1) nitrate enrichment. Culture light and temperature were the same as those used in the pre-culture conditions. The LC and HC culture media were replaced with freshly prepared medium saturated with low and high CO2 bubbling every other day. To maintain a consistent pH, the biomass of the thalli was regulated to not exceed 0.2 g following medium replacement in each treatment, ensuring that the pH fluctuation remained below 0.05 throughout each treatment. All relevant measurements were performed after 12 days of incubation, and each treatment had three biological replicates.
2.2 pH measurement
During the culture period, the pH values of the different CO2 and nitrate treatments were measured every other day using a pH meter (Mettler Toledo DL15 Titrator, Sweden). Before pH measurement, 3 points calibration (pH 4.01, 7.01, and 10.01) was performed with NBS buffer solution (National Bureau of Standards, Hanna).
2.3 Relative growth rate measurement and determination of dry weight-fresh weight relationship
The relative growth rate (RGR) of the different treatments was determined by changes in fresh weight (FW) and was calculated using the following equation:
where, A and B indicate the fresh weights at the end and beginning of the culture period (t), respectively.
To determine the relationship between dry and fresh weight, ca. 0.03g to 0.14g thalli of each treatment were randomly collected during the culture and dried in an oven for 24 hours under 80°C. Dry weight change as a function of fresh weight was built with liner regression and the slopes of different treatments were obtained accordingly.
2.4 Pigmentation measurement
For the chlorophyll a (Chl a) and carotenoid measurement, ca. 0.05 g thalli were selected and extracted with methanol at 4°C overnight, and the supernatant were determined spectrophotometrically (DU800, Beckman, Fullerton, California, USA). Chl a and carotenoids were calculated following the methods described by Porra (2002) and Parsons and Strickland (1963). Phycoerythrin (PE) and phycocyanin (PC) determination, ca. 0.05 g thalli were randomly selected and ground into powder with 6 mL phosphate buffer (pH 6.80), and the supernatant was scanned with a spectrophotometer at wavelengths of 455 nm, 564 nm, 592 nm, 618 nm, and 645 nm; PE and PC were calculated using the equation from Beer and Eshel (1985).
2.5 NO3- uptake rate measurement
For the NO3- uptake rate measurement, 15 ml culture medium from different treatments were sampled at the beginning and after 24h of culture. NO3- concentration was determined using a nutrient autoanalyzer (QuAAtro, Bran-Luebbe Corporation, Germany).
2.6 Net photosynthesis and dark respiration measurements
Net photosynthetic oxygen evolution rates and dark respiration were measured using a Clark-type oxygen electrode (YSI 5300A, USA), and the methods which were detailed by Yang et al. (2021). Two points calibration (zero and 100%) of the oxygen electrode was performed prior to the measurements. Excess superfluous sodium sulfite (Na2SO3) added in distilled water to remove O2 was used for zero-point correction, and distilled water bubbled with air until saturation was used for 100%-point correction. For the photosynthesis measurement, oxygen evolution rate was measured under culture light of 100 μmol m-2 s-1 and high light of 400 μmol m-2 s-1 (near saturation light intensity, according to the rapid light curve).
2.7 Induction cure and rapid light curve measurements
The induction curves of the different treatments were measured after 15 min of dark adaptation using an XE-PAM (Walz, Germany). The actinic light was set at 156 μmol m-2 s-1 and the saturation pulse intensity was 5000 μmol m-2 s-1. For the rapid light curve measurement, thalli exposure under gradually increased actinic light of 156, 226, 337, 533, 781, 1077, 1593 and 2130 μmol m-2 s-1 for 10s in each light level, and subsequently with a saturation pulse. Maximum quantum yield (FV/FM), was acquired from the first point of induction curve and effective quantum yield (Y(II)) and non-photochemical quenching (NPQ) were derived from the average of last 3 points of induction curve, and calculated using the following equation:
FM represents the peak fluorescence attained after a 15-minute dark adaptation period. FM’ denotes the light-adapted maximum chlorophyll fluorescence observed under actinic light conditions. F0 signifies the baseline fluorescence, while Ft indicates the stable fluorescence level maintained during the light exposures.
For the rapid light curve fitting, maximum relative electron transport rate (rETRmax), light use efficiency (α) and saturation light intensity (Ik) were detailed in Xu and Gao (2012) which was following the equation from Webb et al. (1974):
Y denote the relative electron transport rate (rETR), x represents the photon flux density, rETRmax signify the peak electron transport rate, α symbolize the efficiency of light utilization, and Ik stand for the saturation light intensity.
2.8 Statistical analysis
Two-way or three-way analysis of variance (ANOVA) was used to determine the individual or interactive effects of CO2, nitrate and light intensity on the observed parameters at a confidence level of 95% (P = 0.05), and Tukey’s multiple comparisons were used to establish differences among treatments.
3 Results
3.1 pH variation during culture
The pH of different CO2 and nitrate treatments were all kept in stable during the culture period, with averaged pH values of 8.20 ± 0.03, 7.84 ± 0.03, 8.22 ± 0.04 and 7.85 ± 0.04 under LC-LN, HC-LN, LC-HN and HC-HN condition (Figure 1).
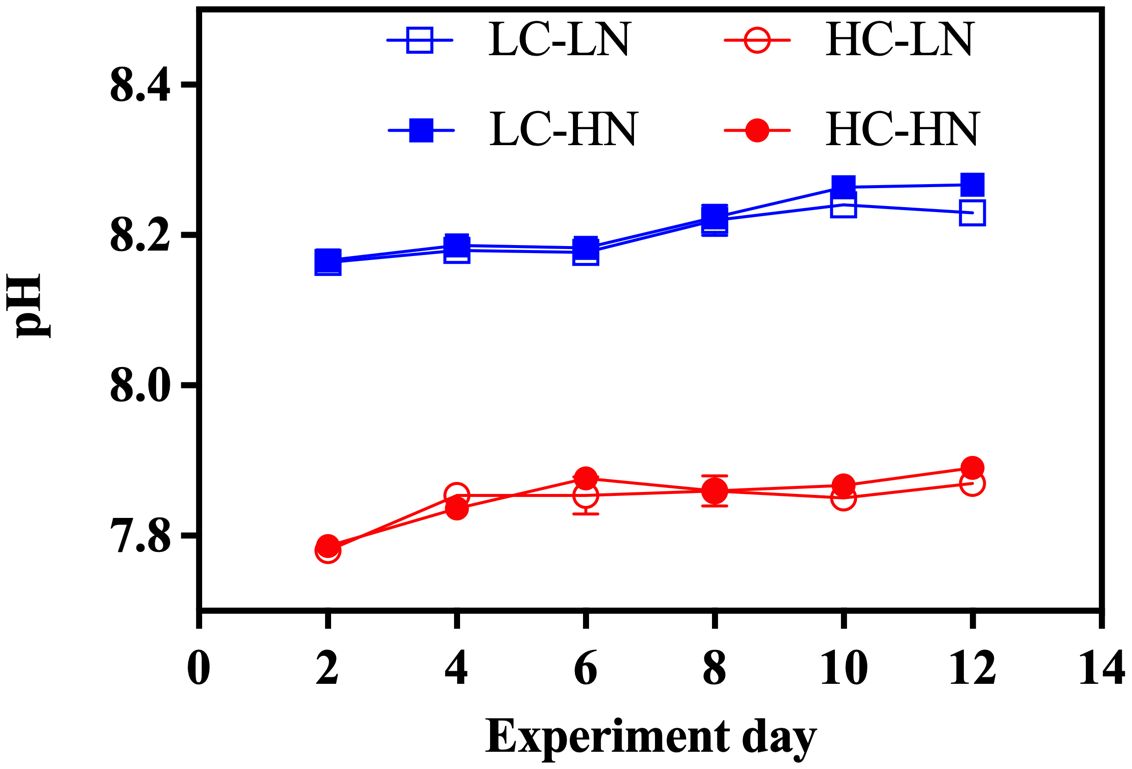
Figure 1. The measured pH of low CO2 (LC) and high CO2 (HC) culture under low nitrate (LN) and high nitrate (HN) conditions during the culture (n=3).
3.2 Growth rate and dry weight-fresh weight relationship
The growth rate was significantly affected by both CO2 (Two Way ANOVA, P< 0.0001) and nitrate concentrations (Two-Way ANOVA, P< 0.05) (Table 1). High CO2 levels significantly decreased growth rate by 30.56% (P< 0.05) and 39.36% (P< 0.05) in the LN and HN treatments, respectively (Figure 2). No significant difference in growth rate was observed between LN and HN under HC conditions (P > 0.05); however, a significant decrease in growth rate of 25.86% was observed under LN conditions (P< 0.05) (Figure 2).
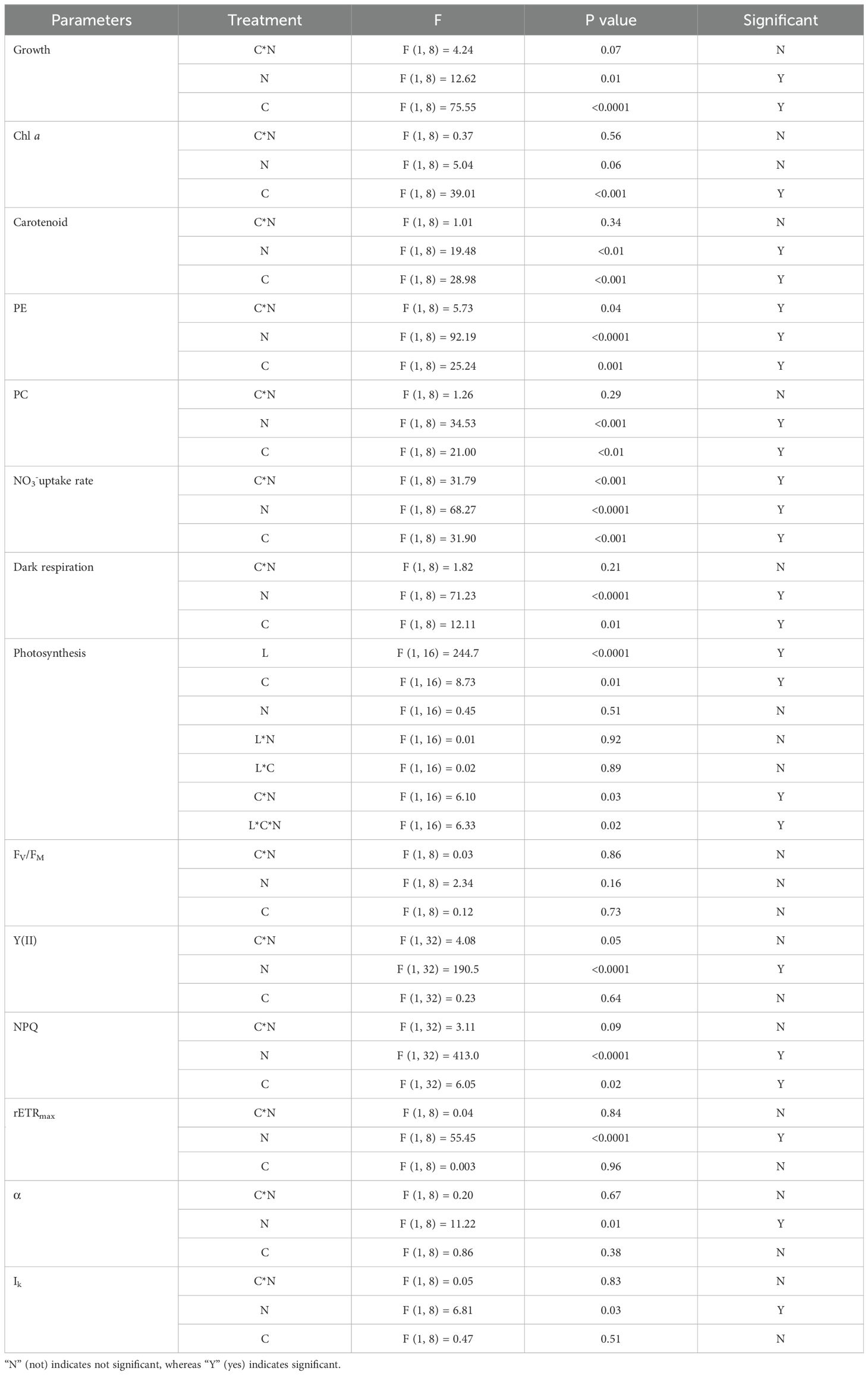
Table 1. Two- or three-way ANOVA analysis of individual and interactive effects of CO2 (C), nitrate level (N), and irradiance treatments (L) on the studied parameters with confidence interval of 95% (P< 0.05).
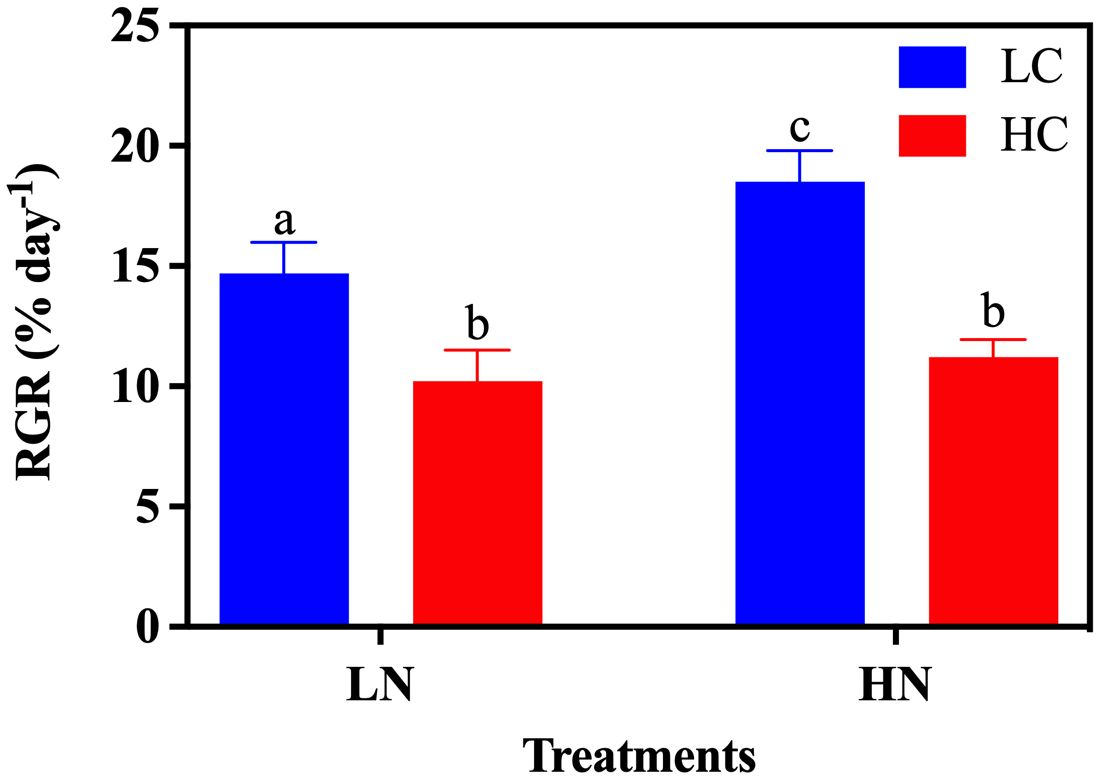
Figure 2. The relative growth rate (RGR) of Gracilariopsis lemaneiformis grown under different CO2 and nitrate conditions (n = 3). Different lowercase letters above the bar indicate significant differences between treatments.
All treatments showed a significant linear relationship between dry and fresh weights (P< 0.0001) (Figure 3). Linear regression curves of dry weight as a function of fresh weight showed different slopes under different CO2 and nitrate treatments (Figures 3A–D). Generally, HC (Figures 3C, D) showed higher slopes than LC cultures (Figures 3A, B), and LN (Figures 3A, C)-grown algae showed higher slopes than the HN treatments (Figures 3B, D), indicating that high CO2 and low nitrate treatments increased the dry matter mass per unit fresh weight.
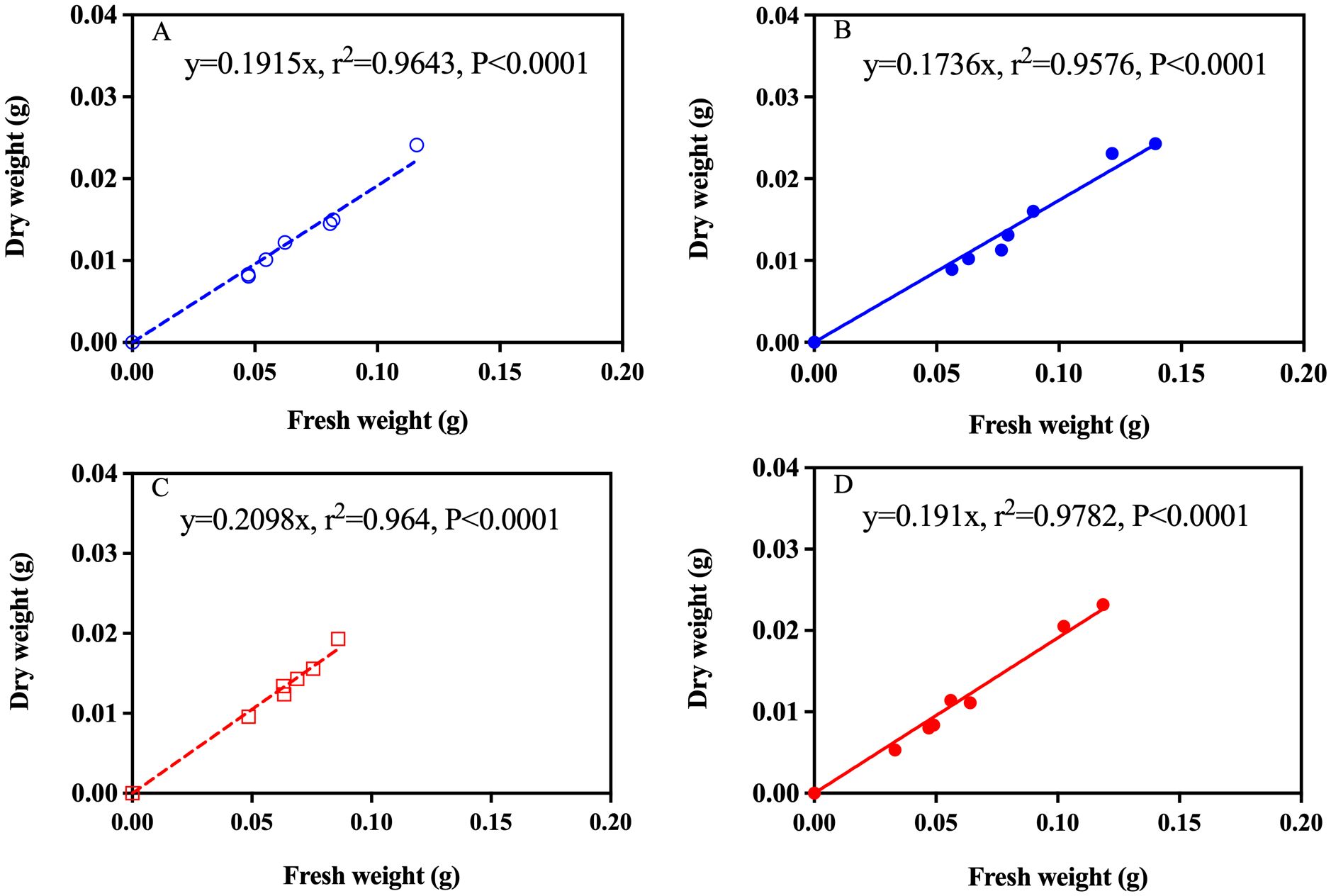
Figure 3. The linear regression of dry and fresh weights of Gracilariopsis lemaneiformis grown under (A) LC-LN, (B) LC-HN, (C) HC-LN, and (D) HC-HN conditions (n = 3).
3.3 Pigmentations
After being grown under LN conditions for more than 12 days, the color of the algal body changed from reddish-brown to pale-yellow in both LC and HC, indicating that pigmentation was affected by different nitrate treatments (Figure 4). Chl a, carotenoids, PE, and PC were all significantly affected by CO2 and nitrate concentrations (all P< 0.05, Two-Way ANOVA), except for Chl a which was not affected by nitrate (P > 0.05) (Table 1). Compared with the LC culture, HC significantly decreased Chl a by 15.07% (P< 0.05) and 17.18% (P< 0.05) under LN and HN conditions, respectively (Figure 5A). There were no significant changes in carotenoid, PE, and PC between LC and HC in LN cultures (all P > 0.05); however, HC decreased carotenoid, PE, and PC levels by 8.74% (P< 0.05), 28.59% (P< 0.05), and 21.99% (P< 0.05), respectively, in HN cultures (Figures 5B–D). No significant changes in Chl a and carotenoids were observed between LN and HN in both the LC and HC treatments (P > 0.05) (Figures 5A, B), except for a slight increase in carotenoid content in HN under LC conditions (P< 0.05) (Figure 5B). Compared with the HN culture, LN significantly decreased PE by 46.22% and 38.90% (P< 0.05) (Figure 5C), and PC by 26.97% and 23.47% (P< 0.05) under LC and HC conditions (Figure 5D).
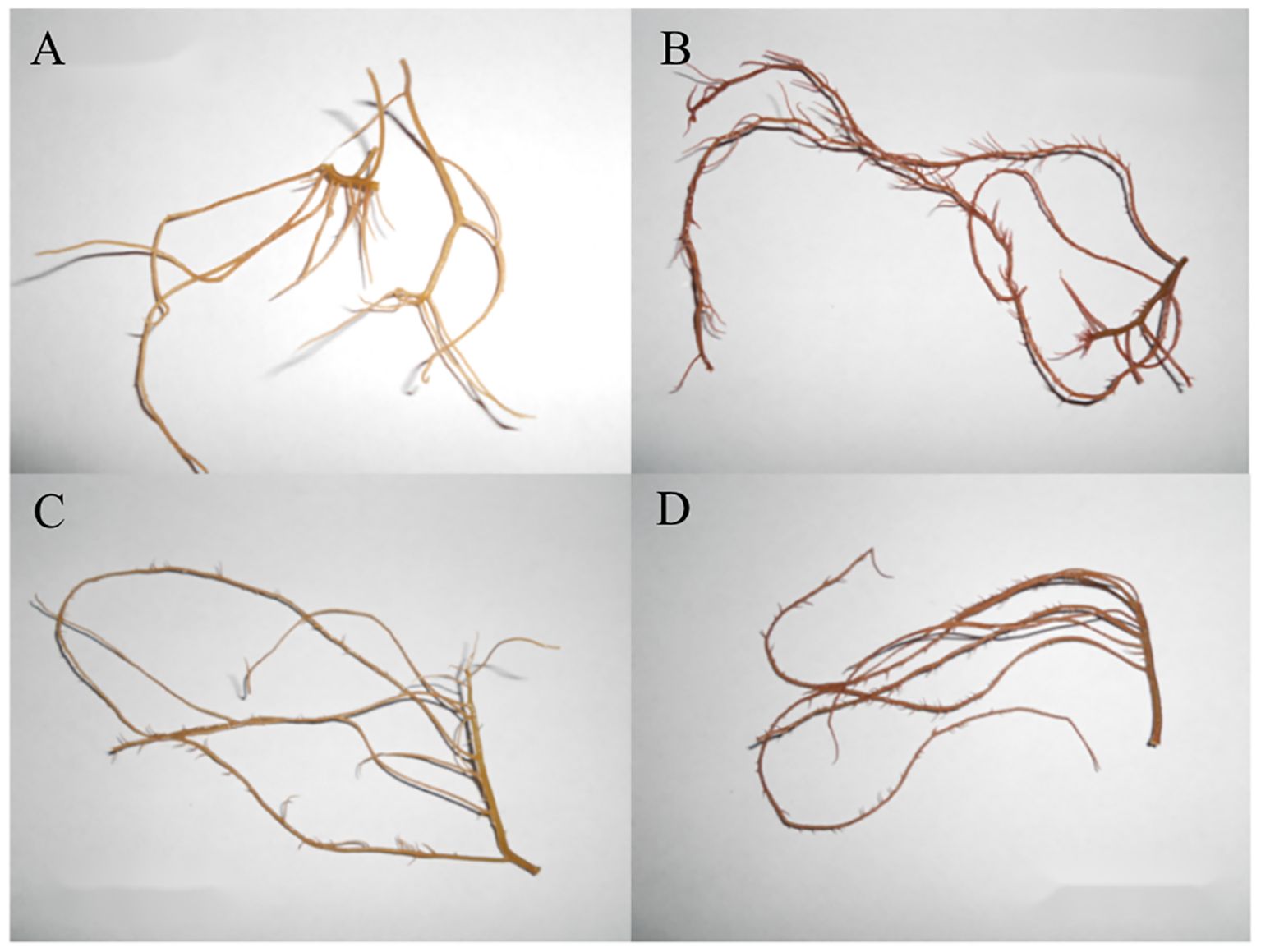
Figure 4. Photograph of Gracilariopsis lemaneiformis thalli grown under LC-LN (A), LC-HN (B), HC-LN (C), and HC-HN (D) conditions for 12 days.
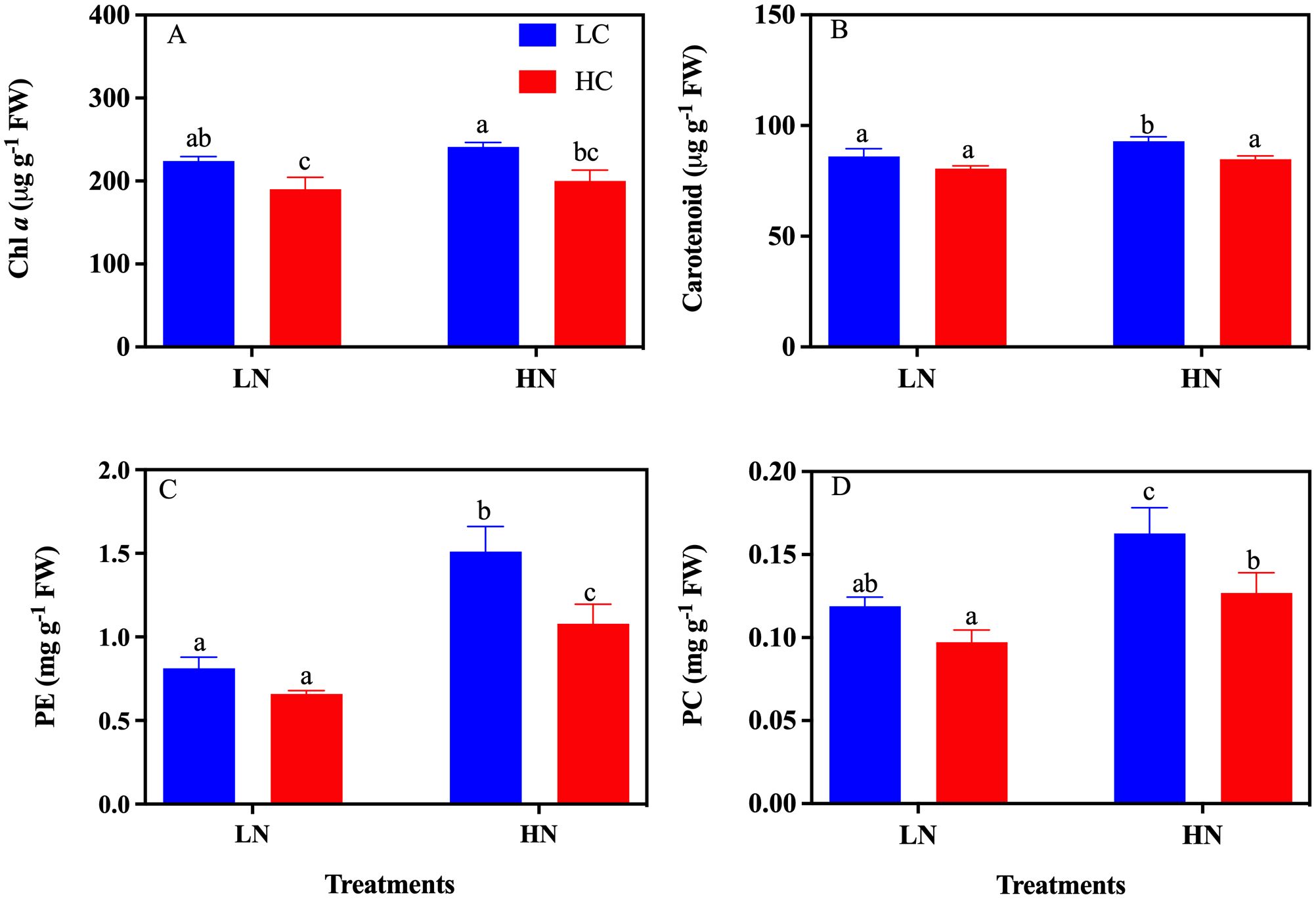
Figure 5. The pigment contents of Gracilariopsis lemaneiformis grown under different CO2 and nitrate conditions (n = 3). (A) Chl a, (B) carotenoid, (C) phycoerythrin (PE), and (D) phycocyanin (PC). Different lowercase letters above the bar indicate significant differences between treatments.
3.4 NO3- uptake rate
The nutrient uptake rate showed different responses in CO2 and NO3- treatments. Both the individual and interactive effects of CO2 and nitrate concentrations on the NO3- uptake rate were observed (Two-Way ANOVA, all P< 0.05) (Table 1). Under the LN treatment, NO3- was consumed in both LC and HC culture after 24h of culture, and no significant difference in the NO3- uptake rate was observed between the CO2 treatments (Figure 6). High CO2 levels significantly stimulated the NO3- uptake rate under HN culture conditions (P< 0.05). Under HN treatments, a significant stimulation of the NO3- uptake rate by 259.44% (P< 0.05) was detected under HC compared with the LC treatment (Figure 6).
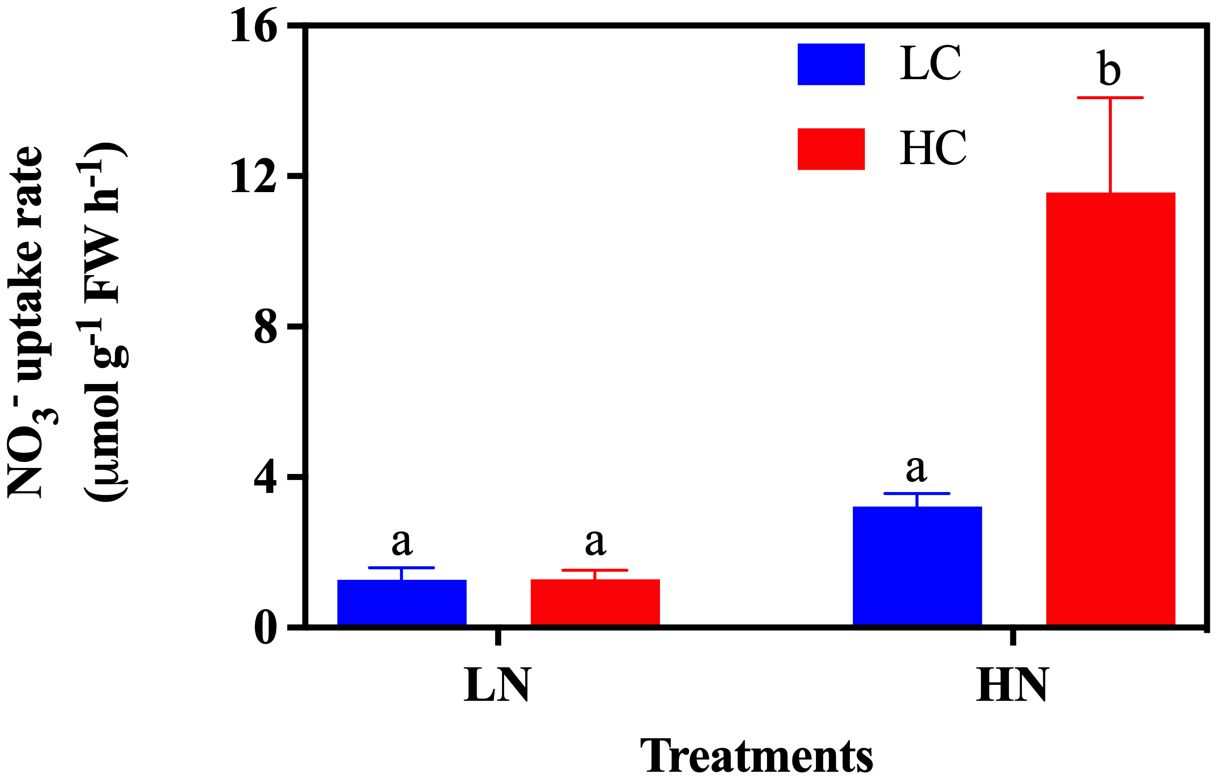
Figure 6. The NO3- uptake rate of Gracilariopsis lemaneiformis grown under different CO2 and nitrate conditions (n = 3). Different lowercase letters above the bar indicate significant differences between treatments.
3.5 Net photosynthesis and dark respiration
High-light exposure significantly stimulated the net photosynthetic evolution rate of O2 (Figures 7A, B). There was no significant change in the net photosynthetic evolution rate of O2 between the CO2 and nitrate treatments, either with exposure to low or high light (P > 0.05) (Figures 7A, B). Both the CO2 and nitrate concentrations significantly affected the dark respiration rate, with a 24.23% increase (P< 0.05) (Table 1) under HC compared to the LC treatment under HN (Figure 7C). HN enhanced dark respiration rates by 55.18% (P< 0.05) and 65.38% (P< 0.05) under LC and HC conditions, respectively, compared to the LN culture (Figure 7C).
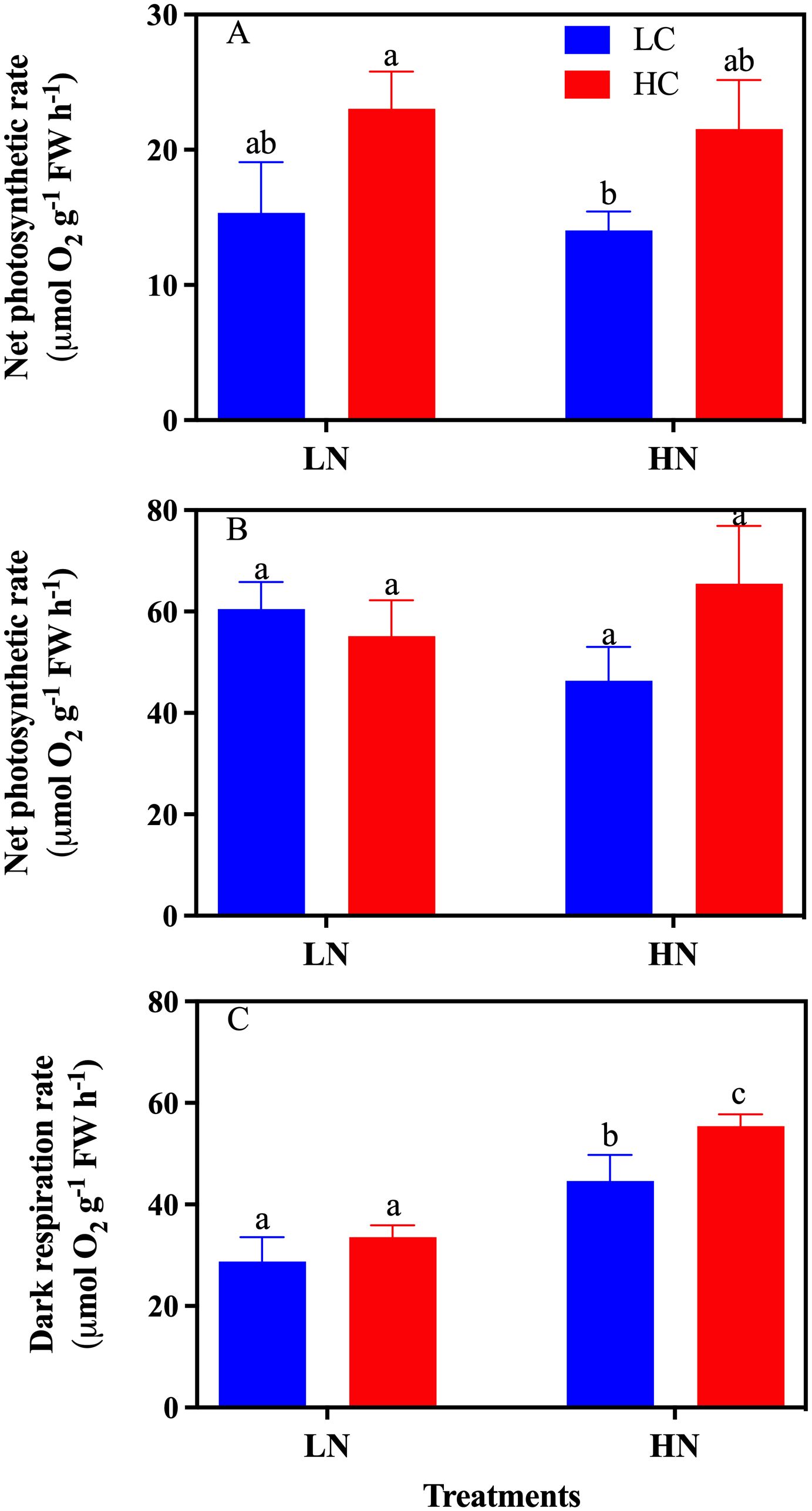
Figure 7. The net photosynthetic O2 evolution rate and dark respiration of Gracilariopsis lemaneiformis grown under different CO2 and nitrate conditions (n = 3). (A) 100 μmol m-2s-1, (B) 400 μmol m-2s-1, and (C) dark respiration rate. Different lowercase letters above the bar indicate significant differences between treatments.
3.6 Fluorescence from rapid light cure and induction curve
Based on the measured rapid light curves (Figure 8A), the fitted parameters of the maximum relative electron transport rate (rETRmax), light-use efficiency (α), and saturation light intensity (Ik) were obtained (Table 2). There were significant effects of nitrate concentration (all P< 0.05), but not CO2 (all P > 0.05) on the maximum of relative electron transport rate (rETRmax), light use efficiency (α), and saturation light intensity (Ik) (Table 1). In the LN culture, rETRmax decreased by 26.24% (P< 0.05) and 24.95% (P< 0.05), and α by 16.72% (P< 0.05) and 12.53% (P< 0.05) in the LC and HC treatments, respectively, compared to the HN culture (Table 1). There was a slight but not significant increase in Ik under HN compared with the LN treatment (P > 0.05). No interactive effects of nitrate and CO2 were detected on rETRmax, α or Ik (Two-Way ANOVA, P > 0.05) (Table 1).
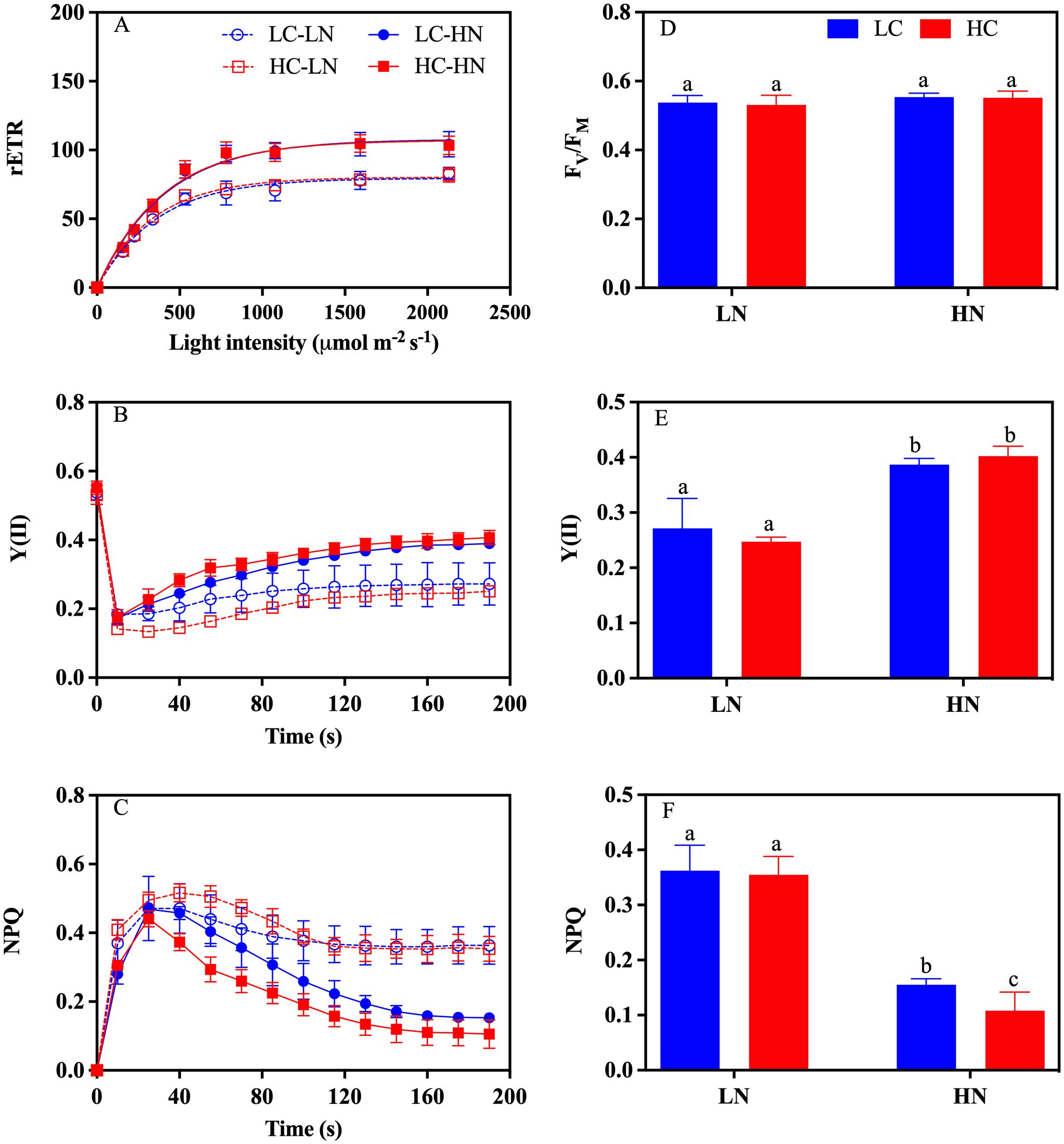
Figure 8. The chlorophyll fluorescence parameters of Gracilariopsis lemaneiformis under different CO2 and nitrate conditions. (A) The rapid light curve, changes of (B) Y(II), and (C) NPQ from the induction curve and the (D) FV/FM, (E) Y(II), and (F) NPQ derived from the induction curve. Except for (E) Y (II) and (F) NPQ, where n=9, all other parameters have a sample size of 3 (n=3).
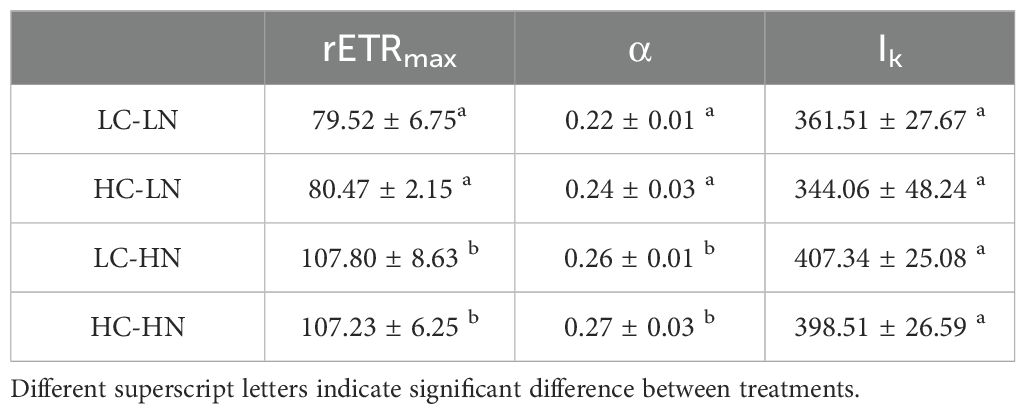
Table 2. The calculated maximum of relative electron transport rate (rETRmax), light use efficiency (α), and saturation light intensity (Ik) from the rapid light curve of Figure 8 (n = 3).
According to the measured induction curve (Figures 8B, C), the FV/FM, Y(II), and NPQ values were acquired (Figures 8D–F). No significant changes in FV/FM were detected among the treatments (P > 0.05) (Figure 8D). Y(II) and NPQ were significantly affected by nitrate concentration (P< 0.05) (Table 1), with 29.83% (P< 0.05) and 38.55% (P< 0.05) decrease in Y(II) and 133.33% (P< 0.05) and 227.72% (P< 0.05) increase in NPQ in the LC and HC treatments, respectively, in comparison with the HN culture (Figures 8D, E). Significant effects of CO2 on NPQ were observed in the HN treatment, with a 30.33% (P< 0.05) (Table 1) lower NPQ under HC than under LC culture (Figure 8F).
4 Discussion
Macroalgae are the major contributors to nearshore primary productivity, accounting for approximately 10% of global marine primary productivity (Smith, 1981). The large-scale cultivation of macroalgae is regarded as an important means of enhancing ocean carbon sink capacity, improving the eutrophication level in coastal waters, and mitigating climate change (Gao and McKinley, 1994; Duarte et al., 2017; Gao and Beardall, 2022). Macroalgae are subject to global challenges like ocean warming and elevated ultraviolet radiation, as well as regional influences including nutrient levels, salinity fluctuations, and light changes (Boyd et al., 2018; Ji and Gao, 2021). Therefore, investigating intricate coastal environmental shifts at a regional scale under global change may have significant implications on macroalgal cultivation.
Our results showed that high CO2 levels had significant negative effects on the growth of G. lemaneiformis regardless of LN or HN conditions. Both high CO2 and low nitrate treatments enhanced the dry mass of G. lemaneiformis relative to the fresh weight. Nitrate limitation lowered phycoerythrin (PE) and phycocyanin (PC) content and had significant effects on photosynthetic performance, including decreased dark respiration rate, effective quantum yield (Y(II)), maximum relative electron transport rate (rETRmax), and light use efficiency rate (α), and increased non-photochemical quenching (NPQ). High CO2 synergy with high nitrate significantly decreased the pigmentation of G. lemaneiformis and NPQ, which stimulated the NO3- uptake rate and enhanced dark respiration.
Previous studies have shown that OA can have profound and differential effects on different types of marine macroalgae (Ji and Gao, 2021). For example, seawater acidification can significantly affect the calcification process and the abundance of most calcified algae (Gao et al., 1993; Kuffner et al., 2008), although other findings have indicated that the calcification rate of some calcifying species is not affected by the protective external layer or the creation of a microenvironment (Ji and Gao, 2021). Non-calcifying species, owing to the difference in inorganic carbon use among different types of macroalgae, exhibit diverse growth and physiological responses (Koch et al., 2013) which usually depend on the energy balance between synthesis and consumption (Häder and Gao, 2023). Considering the complex marine environment caused by global changes and local environmental variations, the responses of macroalgal growth and photosynthesis to OA exhibit interspecific differences (Ji and Gao, 2021). Even within the same species, different responses may arise when plants are exposed to different growth environments or cultivation systems. Farming of G. lemaneiformis in southern China usually begins from November to May of the following year. During the cultivation period, a series of environmental changes will be experienced, such as the change of seawater temperature from low to high (from 11°C to above 26°C in Nanao, Shantou, China), and the decrease of water nutrient concentration and light intensity with the increase of cultivation density (Zou and Gao, 2009; 2014). Therefore, for the G. lemaneiformis, growth and photosynthesis are modulated and may show divergent responses to OA under co-varied environmental conditions. High CO2 did not change the growth rate of G. lemaneiformis under both low and high nitrate condition when grown under natural solar light with average PAR level of 107.3 kJ m-2 (Zhou et al., 2022). Stimulated growth rates were observed in high CO2 cultures under either low or high light conditions at a high culture temperature of 28°C (Chen et al., 2017). In the present study, high CO2 levels had significant negative effects on the growth of G. lemaneiformis regardless of LN or HN conditions. There was no significant difference in photosynthetic oxygen evolution among the treatments, indicating saturation of the photosynthetic rate under the current seawater DIC and light levels (Yang et al., 2021). The respiration rate showed an increasing trend under both LC and HC compared to the LN treatment, with the increase being more pronounced under HC and HN conditions. This suggests that the energy fixed by algal photosynthesis is consumed at a higher rate under high CO2/low pH conditions, potentially being exacerbated by stimulated photorespiration and other methods of energy consumption (Gao et al., 2012), thereby resulting in growth inhibition under the current culture conditions.
Under LN conditions, the absorption rate of NO3- was inhibited and exhibited no significant differences between the LC and HC treatments, suggesting that reduced nitrogen concentration in the aquatic environment has a more profound impact than elevated CO2 levels and is the primary driving factor. Although HC treatment significantly enhanced the absorption rate of NO3- under HN conditions, it did not increase growth assimilation. This phenomenon is potentially associated with the partitioning of energy within the photosynthetic carbon and nitrogen metabolism, as the accumulation of carbon and nitrogen can be affected by OA (Chen et al., 2018).
The dry weight-fresh weight relationship in macroalgae varied among species (Wickham et al., 2019) and may differed when the environmental condition changed (Manns et al., 2017). The increased ratio of dry mass to fresh weight in G. lemaneiformis, induced by elevated CO2 levels or diminished nitrate availability, signified a reduction in water content, suggesting an alteration in water retention capacity. This could be associated with modifications in the chemical composition of both soluble and insoluble organic substances, potentially including changes in the carbohydrate profiles and protein concentrations (Manns et al., 2017). A previous study showed that HC culture could increase the soluble carbohydrate content of G. lemaneiformis but decrease the soluble protein or AA contents (Chen et al., 2017, 2018). Where Gracilariopsis sp. grown in an extremely high inorganic C concentrations (5% CO2) showed enhanced soluble carbohydrate concentration (Andria et al., 1999). Thus the composition and content of organic substances will change under different culture conditions, and subsequently determine the accumulation of organic matter (C and N) (Andria et al., 1999). Given that the chemical composition of G. lemaneiformis was not determined in this study, the effects of OA and low nitrogen on carbohydrates, proteins, and minerals remain to be explored in future research.
The pigment composition and content of macroalgae can be regulated by complex environmental changes, such as CO2, light intensity, nutrients, and temperature, both individually and interactively (Häder and Figueroa, 1997; Zou and Gao, 2009; Yang et al., 2021; Zhou et al., 2022). Decreased photosynthetic pigments of Chl a, carotenoids, phycoerythrin (PE), and phycocyanin (PC) content due to high CO2 under nitrogen-replete conditions in this study were in accordance with numerous previous studies (García-Sánchez et al., 1994; Andria et al., 1999). Synergistic effects of nutrient deficiency with high CO2 have also been reported in previous studies; for example, Chl a, carotenoids, and PE were reduced by elevated CO2 under N-and P deficiency condition (Zhou et al., 2022). In the present study, the transition of thalli color from reddish-brown to pale-yellow under nitrogen-limited conditions indicated that pigment composition and synthesis are regulated by nitrogen availability. In contrast to the general decline in pigment content observed under the HN treatment in HC conditions, the modest decrease in Chl a and the unchanged levels of carotenoids, PE, and PC under the LN treatment imply that nitrogen concentration predominantly influences the effect of OA on pigments. Specifically, the influence of low-nitrogen conditions on pigments appears to be greater than the effect of OA. Compared to the accessory light-harvesting pigments of carotenoids, PE, and PC, Chl a was not affected under the present LN conditions in comparison with HN, which suggests that energy allocation is preferential to the main light-harvesting pigment synthesis.
In the present study, high CO2 levels did not change FV/FM and Y(II). The unchanged FV/FM under LN treatment suggests that the open Photosystem II is unaffected. However, when the actinic light intensity was close to the culture conditions, the decrease in photochemical efficiency indicated that actual photochemical capacity was significantly inhibited by LN. Simultaneously, the reduction in rETRmax showed that nitrogen limitation had a significant effect on the electron transport chain of Photosystem II. An increase in NPQ generally characterizes the ability of algal photosynthetic mechanisms to dissipate excess light energy in the form of heat, which is an important photoprotective mechanism (Muller et al., 2001; Ruban, 2016; Stadnichuk and Krasilnikov, 2023). Under low nitrogen conditions, a higher NPQ indicates that the excitation energy generated by light quanta absorbed by the photosynthetic mechanism is in an excess state under the current cultivation light intensity.
5 Conclusions
Our findings suggest that OA may negatively affect the growth of G. lemaneiformis, with photophysiological performance differentially modulated by nutrient status. These results contribute to a deeper understanding of the potential effects of ongoing oceanic changes in coastal zones, caused by regional and global changes in marine primary producers, particularly within the macroeconomic context of the seaweed farming industry. Our study highlights the need for further research, both controlled indoor experiments and field investigations, to unravel the intricate interplay between OA and nutrient limitation in macroalgae in the context of global change.
Data availability statement
The original contributions presented in the study are included in the article/supplementary material. Further inquiries can be directed to the corresponding authors.
Author contributions
YY: Writing – original draft, Visualization, Methodology, Investigation, Formal analysis. WL: Writing – review & editing, Visualization, Resources, Methodology, Formal analysis, Conceptualization. YL: Writing – review & editing, Visualization, Methodology. NX: Writing – review & editing, Visualization, Supervision, Resources, Methodology, Funding acquisition, Formal analysis, Conceptualization.
Funding
The author(s) declare financial support was received for the research, authorship, and/or publication of this article. This study was supported by the Key Program of Science and Technology of Ningbo (2022Z172, 2023Z118), the National Natural Science Foundation of China (31672674), and Eco-Environmental Conservation Research Centre of Xin’an River Basin (XECC), Huangshan University (kypt202002). The funders had no role in study design, data collection and analysis, decision to publish or preparation of the manuscript.
Conflict of interest
The authors declare that the research was conducted in the absence of any commercial or financial relationships that could be construed as a potential conflict of interest.
The author(s) declared that they were an editorial board member of Frontiers, at the time of submission. This had no impact on the peer review process and the final decision.
Publisher’s note
All claims expressed in this article are solely those of the authors and do not necessarily represent those of their affiliated organizations, or those of the publisher, the editors and the reviewers. Any product that may be evaluated in this article, or claim that may be made by its manufacturer, is not guaranteed or endorsed by the publisher.
References
Andria J. R., Vergara J. J., Perez-Llorens J. L. (1999). Biochemical responses and photosynthetic performance of Gracilaria sp. (Rhodophyta) from Cádiz, Spain, cultured under different inorganic carbon and nitrogen levels. Eur. J. Phycol. 34, 497–504. doi: 10.1017/S0967026299002334
Bao M., Hu L., Fu Q., Gao G., Li X., Xu J. (2019). Different photosynthetic responses of Pyropia yezoensis to ultraviolet radiation under changing temperature and photosynthetic active radiation regime. Photochem. Photobiol. 95, 1213–1218. doi: 10.1111/php.13108
Beer S., Bjork M., Hellblom F., Axelsson L. (2002). Inorganic carbon utilization in marine angiosperms (seagrasses). Funct. Plant Biol. 29, 349–354. doi: 10.1071/PP01185
Beer S., Eshel A. (1985). Determining phycoerythrin and phycocyanin concentrations in aqueous crude extracts of red algae. Mar. Freshw. Res. 36, 785–792. doi: 10.1071/MF9850785
Boyd P. W., Collins S., Dupont S., Fabricius K., Gattuso J.-P., Havenhand J., et al. (2018). Experimental strategies to assess the biological ramifications of multiple drivers of global ocean change-a review. Global Change Biol. 24, 2239–2261. doi: 10.1111/gcb.14102
Cai W.-J., Hu X., Huang W.-J., Murrell M. C., Lehrter J. C., Lohrenz S. E., et al. (2011). Acidification of subsurface coastal waters enhanced by eutrophication. Nat. Geosci. 4, 766–770. doi: 10.1038/ngeo1297
Capone D. G., Hutchins D. A. (2013). Microbial biogeochemistry of coastal upwelling regimes in a changing ocean. Nat. Geosci. 6, 711–717. doi: 10.1038/ngeo1916
Celis-Plá P. S. M., Hall-Spencer J. M., Horta P. A., Milazzo M., Korbee N., Cornwall C. E., et al. (2015). Macroalgal responses to ocean acidification depend on nutrient and light levels. Front. Mar. Sci. 2, 26. doi: 10.3389/fmars.2015.00026
Chen B., Zou D., Du H., Ji Z. (2018). Carbon and nitrogen accumulation in the economic seaweed Gracilaria lemaneiformis affected by ocean acidification and increasing temperature. Aquaculture 482, 176–182. doi: 10.1016/j.aquaculture.2017.09.042
Chen B., Zou D., Zhu M., Yang Y. (2017). Effects of CO2 levels and light intensities on growth and amino acid contents in red seaweed Gracilaria lemaneiformis. Aquac. Res. 48, 2683–2690. doi: 10.1111/are.2017.48.issue-6
Doney S. C., Fabry V. J., Feely R. A., Kleypas J. A. (2009). Ocean acidification: The other CO2 problem. Annu. Rev. Mar. Sci. 1, 169–192. doi: 10.1146/annurev.marine.010908.163834
Du R., Liu L., Wang A., Wang Y. (2013). Effects of temperature, algae biomass and ambient nutrient on the absorption of dissolved nitrogen and phosphate by Rhodophyte Gracilaria asiatica. Chin. J. Oceanol. Limnol. 31, 353–365. doi: 10.1007/s00343-013-2114-2
Duarte C. M., Wu J., Xiao X., Bruhn A., Krause-Jensen D. (2017). Can seaweed farming play a role in climate change mitigation and adaptation? Front. Mar. Sci. 4. doi: 10.3389/fmars.2017.00100
Dudgeon S., Kübler J. E. (2020). A multistressor model of carbon acquisition regulation for macroalgae in a changing climate. Limnol. Oceanogr. 65, 2541–2555. doi: 10.1002/lno.11470
Falkowski P. G., Raven J. A. (2013). Aquatic Photosynthesis (Princeton, New Jersey: Princeton University).
Feng Y., Xiong Y., Hall-Spencer J. M., Liu K., Beardall J., Gao K., et al. (2024). Shift in algal blooms from micro-to macroalgae around China with increasing eutrophication and climate change. Global Change Biol. 69, e17018. doi: 10.1111/gcb.17018
Friedlingstein P., O’sullivan M., Jones M., Andrew R., Hauck J., Olsen A., et al. (2020). Global carbon budget 2020. Earth Syst. Sci. Data 12, 3269–3340. doi: 10.5194/essd-12-3269-2020
Gao K., Aruga Y., Asada K., Ishihara T., Akano T., Kiyohara M. (1993). Calcification in the articulated coralline alga Corallina pilulifera, with special reference to the effect of elevated CO2 concentration. Mar. Biol. 117, 129–132. doi: 10.1007/BF00346434
Gao K., Beardall J. (2022). Using macroalgae to address UN sustainable development goals through CO2 remediation and improvement of the aquaculture environment. Appl. Phycol. 3, 360–367. doi: 10.1080/26388081.2022.2025617
Gao K., McKinley K. R. (1994). Use of macroalgae for marine biomass production and CO2 remediation: a review. J. Appl. Phycol. 6, 45–60. doi: 10.1007/BF02185904
Gao K., Xu J., Gao G., Li Y., Hutchins D. A., Huang B., et al. (2012). Rising CO2 and increased light exposure synergistically reduce marine primary productivity. Nat. Climate Change 2, 519–523. doi: 10.1038/nclimate1507
García-Sánchez M. J., Fernández J. A., Niell X. (1994). Effect of inorganic carbon supply on the photosynthetic physiology of Gracilaria tenuistipitata. Planta 194, 55–61. doi: 10.1007/BF00201034
Giordano M., Beardall J., Raven J. A. (2005). CO2 concentrating mechanisms in algae: mechanisms, environmental modulation, and evolution. Annu. Rev. Plant Biol. 56, 99–131. doi: 10.1146/annurev.arplant.56.032604.144052
Guinotte J. M., Fabry V. J. (2008). Ocean acidification and its potential effects on marine ecosystems. Ann. New York Acad. Sci. 1134, 320–342. doi: 10.1196/annals.1439.013
Häder D. P., Figueroa F. L. (1997). Photoecophysiology of marine macroalgae. Photochem. Photobiol. 66, 1–14. doi: 10.1111/j.1751-1097.1997.tb03132.x
Häder D.-P., Gao K. (2023). Aquatic productivity under multiple stressors. Water 15, 817. doi: 10.3390/w15040817
Hall-Spencer J. M., Harvey B. P. (2019). Ocean acidification impacts on coastal ecosystem services due to habitat degradation. Emerg. Topics Life Sci. 3, 197–206. doi: 10.1042/ETLS20180117
Hepburn C., Pritchard D., Cornwall C., McLeod R., Beardall J., Raven J., et al. (2011). Diversity of carbon use strategies in a kelp forest community: implications for a high CO2 ocean. Global Change Biol. 17, 2488–2497. doi: 10.1111/j.1365-2486.2011.02411.x
Hutchins D. A., Fu F. (2017). Microorganisms and ocean global change. Nat. Microbiol. 2, 17058. doi: 10.1038/nmicrobiol.2017.58
IPCC (2021). Climate Change 2021: The Physical Science Basis (Cambridge, United Kingdom and New York, NY, USA: Contribution of Working Group I to the Sixth Assessment Report of the Intergovernmental Panel on Climate ChangePress., C. U. P. I).
Ji Y., Gao K. (2021). “Chapter two - Effects of climate change factors on marine macroalgae: A review,” in Advances in Marine Biology. Ed. Sheppard C. (San Diego, CA: Academic Press), 91–136.
Koch M., Bowes G., Ross C., Zhang X.-H. (2013). Climate change and ocean acidification effects on seagrasses and marine macroalgae. Global Change Biol. 19, 103–132. doi: 10.1111/j.1365-2486.2012.02791.x
Kroeker K. J., Kordas R. L., Crim R., Hendriks I. E., Ramajo L., Singh G. S., et al. (2013). Impacts of ocean acidification on marine organisms: quantifying sensitivities and interaction with warming. Global Change Biol. 19, 1884–1896. doi: 10.1111/gcb.12179
Kuffner I. B., Andersson A. J., Jokiel P. L., Rodgers K., Mackenzie F. T. (2008). Decreased abundance of crustose coralline algae due to ocean acidification. Nat. Geosci. 1, 114–117. doi: 10.1038/ngeo100
Li P., Chen H., Zhang J., Feng X., Xiao B., Hu Y., et al. (2023). Effects of nutrient deficiency on the branch phenotype of the macroalgae Gracilariopsis lemaneiformis (Rhodophyta). Aquaculture 562, 738794. doi: 10.1016/j.aquaculture.2022.738794
Li T., Jiang Y., Lin S., Zhong S., Jian S., Chen W., et al. (2023). Environmental irradiance and nitrogen source variability trigger the intracellular carbon and nitrogen reallocation of Gracilariopsis lemaneiformis (Rhodophyta). Aquaculture 575, 739802. doi: 10.1016/j.aquaculture.2023.739802
Lu Z., Gan J., Dai M., Zhao X., Hui C. R. (2020). Nutrient transport and dynamics in the South China Sea: A modeling study. Prog. Oceanogr. 183, 102308. doi: 10.1016/j.pocean.2020.102308
Lu N., Zang X., Zhang X., Chen H., Feng X., Zhang L. (2012). Gene cloning, expression and activity analysis of manganese superoxide dismutase from two strains of Gracilaria lemaneiformis (Gracilariaceae, Rhodophyta) under heat stress. Molecules 17, 4522–4532. doi: 10.3390/molecules17044522
Manns D., Nielsen M. M., Bruhn A., Saake B., Meyer A. S. (2017). Compositional variations of brown seaweeds Laminaria digitata and Saccharina latissima in Danish waters. J. Appl. Phycol. 29, 1493–1506. doi: 10.1007/s10811-017-1056-z
Martín L. A., Rodríguez M. C., Matulewicz M. C., Fissore E. N., Gerschenson L. N., Leonardi P. I. (2013). Seasonal variation in agar composition and properties from Gracilaria gracilis (Gracilariales, Rhodophyta) of the Patagonian coast of Argentina. Phycol. Res. 61, 163–171. doi: 10.1111/pre.12000
McHugh D. J. (1991). “Worldwide distribution of commercial resources of seaweeds including Gelidium,” in Juanes J. A., Santelices B., McLachlan J. L. eds. International Workshop on Gelidium. Developments in Hydrobiology (Dordrecht: Springer). doi: 10.1007/978-94-011-3610-5_2
Meng L., Xu D., Chen W. Z., Zhang X. C. (2009). Selection and characterization of a new strain of Gracilaria lemaneiformis. Periodical Ocean Univ. China 39, S94–S98. doi: 10.16441/j.cnki.hdxb.2009.s1.019
Morel F. M. M., Rueter J. G., Anderson D. M., Guillard R. R. L. (1979). Aquil: A chemically defined phytoplankton culture medium for trace metal studies. J. Phycol. 15, 135–141. doi: 10.1111/j.1529-8817.1979.tb02976.x
Muller P., Li X.-P., Niyogi K. K. (2001). Non-photochemical quenching. A response to excess light energy. Plant Physiol. 125, 1558–1566. doi: 10.1104/pp.125.4.1558
Nagelkerken I., Connell S. D. (2022). Ocean acidification drives global reshuffling of ecological communities. Global Change Biol. 28, 7038–7048. doi: 10.1111/gcb.16410
Ober G. T., Thornber C. S. (2017). Divergent responses in growth and nutritional quality of coastal macroalgae to the combination of increased pCO2 and nutrients. Mar. Environ. Res. 131, 69–79. doi: 10.1016/j.marenvres.2017.09.003
Parsons T. R., Strickland J. D. H. (1963). Discussion of spectrophotometric determination of marine-plant pigments, with revised equations for ascertaining chlorophylls and carotenoids. J. Mar. Res. 21, 155–163. doi: 10.1016/0011-7471(65)90662-5
Porra R. J. (2002). The chequered history of the development and use of simultaneous equations for the accurate determination of chlorophylls a and b. Photosynth. Res. 73, 149–156. doi: 10.1023/A:1020470224740
Raven J. A., Cockell C. S., De La Rocha C. L. (2008). The evolution of inorganic carbon concentrating mechanisms in photosynthesis. Philos. Trans. R Soc. Lond. Ser. B: Biol. Sci. 363, 2641–2650. doi: 10.1098/rstb.2008.0020
Raven J. A., Giordano M., Beardall J., Maberly S. C. (2011). Algal and aquatic plant carbon concentrating mechanisms in relation to environmental change. Photosynth. Res. 109, 281–296. doi: 10.1007/s11120-011-9632-6
Riebesell U., Gattuso J.-P. (2015). Lessons learned from ocean acidification research. Nat. Climate Change 5, 12–14. doi: 10.1038/nclimate2456
Ruban A. V. (2016). Nonphotochemical chlorophyll fluorescence quenching: mechanism and effectiveness in protecting plants from photodamage. Plant Physiol. 170, 1903–1916. doi: 10.1104/pp.15.01935
Smith S. (1981). Marine macrophytes as a global carbon sink. Science 211, 838–840. doi: 10.1126/science.211.4484.838
Stadnichuk I. N., Krasilnikov P. M. (2023). Relationship between non-photochemical quenching efficiency and the energy transfer rate from phycobilisomes to photosystem II. Photosynth. Res. 159 (2), 177–189. doi: 10.21203/rs.3.rs-2760691/v1
Tan H., Das S. K., Zainee N. F. A., Yana R., Rozaimi M. (2023). Ocean acidification and aquacultured seaweeds: progress and knowledge gaps. J. Mar. Sci. Eng. 11, 78. doi: 10.3390/jmse11010078
Wang Y., Feng Y., Liu X., Zhong M., Chen W., Wang F., et al. (2018). Response of Gracilaria lemaneiformis to nitrogen deprivation. Algal Res. 34, 82–96. doi: 10.1016/j.algal.2018.07.005
Wang Y., Feng Y., Wang H., Zhong M., Chen W., Du H. (2016). Physiological and proteomic analyses of two Gracilaria lemaneiformis strains in response to high-temperature stress. J. Appl. Phycol. 28, 1847–1858. doi: 10.1007/s10811-015-0723-1
Webb W. L., Newton M., Starr D. (1974). Carbon dioxide exchange of Alnus rubra. Oecologia 17, 281–291. doi: 10.1007/BF00345747
Wickham S. B., Darimont C. T., Reynolds J. D., Starzomski B. M. (2019). Species-specific wet-dry mass calibrations for dominant Northeastern Pacific Ocean macroalgae and seagrass. Aquat. Bot. 152, 27–31. doi: 10.1016/j.aquabot.2018.09.006
Xu J., Gao K. (2012). Future CO2-induced ocean acidification mediates the physiological performance of a green tide alga. Plant Physiol. 160, 1762–1769. doi: 10.1104/pp.112.206961
Yang Y., Li W., Li Y., Xu N. (2021). Photophysiological responses of the marine macroalga Gracilariopsis lemaneiformis to ocean acidification and warming. Mar. Environ. Res. 163, 105204. doi: 10.1016/j.marenvres.2020.105204
Zhang Y., Zhang J., Liang Y., Li H., Li G., Chen X., et al. (2017). Carbon sequestration processes and mechanisms in coastal mariculture environments in China. Sci. China Earth Sci. 60, 2097–2107. doi: 10.1007/s11430-017-9148-7
Zhou W., Wu H., Huang J., Wang J., Zhen W., Wang J., et al. (2022). Elevated-CO2 and nutrient limitation synergistically reduce the growth and photosynthetic performances of a commercial macroalga Gracilariopsis lemaneiformis. Aquaculture 550, 737878. doi: 10.1016/j.aquaculture.2021.737878
Zou D., Gao K. (2009). Effects of elevated CO2 on the red seaweed Gracilaria lemaneiformis (Gigartinales, Rhodophyta) grown at different irradiance levels. Phycologia 48, 510–517. doi: 10.2216/08-99.1
Zou D., Gao K. (2013). Thermal acclimation of respiration and photosynthesis in the marine macroalga Gracilaria lemaneiformis (Gracilariales, Rhodophyta). J. Phycol. 49, 61–68. doi: 10.1111/jpy.12009
Keywords: Gracilariopsis lemaneiformis, culture, ocean acidification, growth, nitrate, photosynthetic performance
Citation: Yang Y, Li W, Li Y and Xu N (2024) Effects of ocean acidification and nitrogen limitation on the growth and photophysiological performances of marine macroalgae Gracilariopsis lemaneiformis. Front. Mar. Sci. 11:1453569. doi: 10.3389/fmars.2024.1453569
Received: 23 June 2024; Accepted: 25 July 2024;
Published: 19 August 2024.
Edited by:
Peng Jin, University of Guangzhou, ChinaReviewed by:
Wanchun Guan, Wenzhou Medical University, ChinaYong Zhang, Fujian Normal University, China
Yaping Wu, Jiangsu Ocean University, China
Copyright © 2024 Yang, Li, Li and Xu. This is an open-access article distributed under the terms of the Creative Commons Attribution License (CC BY). The use, distribution or reproduction in other forums is permitted, provided the original author(s) and the copyright owner(s) are credited and that the original publication in this journal is cited, in accordance with accepted academic practice. No use, distribution or reproduction is permitted which does not comply with these terms.
*Correspondence: Wei Li, bGl2aWxpa2VAMTYzLmNvbQ==; Nianjun Xu, eHVuaWFuanVuQG5idS5lZHUuY24=