- 1Dept of Marine Biology, Texas A&M University, Galveston, TX, United States
- 2Dept of Ecology and Conservation Biology, Texas A&M University, College Station, TX, United States
Introduction: Predators, such as sharks, play important ecological roles in coastal ecosystems when abundant and these roles change over ontogeny. However, these shifts in ecological role are often not evaluated in light of feeding performance. Bite force is an ecologically relevant metric of feeding performance that has been associated with a species’ foraging niche, which may serve as a key factor that constrains prey selection both within and among sympatric species.
Methods: This study applies an interdisciplinary approach to discern the ecomechanical relationships of sympatric bull (Carcharhinus leucas), blacktip (Carcharhinus limbatus), and bonnethead sharks (Sphyrna tiburo) using feeding biomechanics and bulk stable isotopes (δ13C, δ15N). We sought to (1) estimate theoretical bite force and scaling patterns over ontogeny of each species, (2) quantify niche breadth and overlap among species, and (3) characterize the relationship between ecological niche shifts and changes in bite force over ontogeny.
Results: Importantly, we found that smaller conspecifics exhibited positive allometric scaling of bite force, whereas larger conspecifics exhibited isometric scaling. Associations between bite force and ontogenetic niche shifts in habitat and diet were found in bull and bonnethead sharks, but not in blacktip sharks. Additionally, isotopic niche breadth was greatest in generalist bull sharks, followed by specialist bonnethead and blacktip sharks.
Discussion: These findings highlight animal performance measures as critical drivers ofecological relationships both within and among species. Size-based differences in bite force scaling should be considered when evaluating feeding performance in other taxa, which may be an important determinant of ontogenetic dietary shifts.
1 Introduction
Bite force is an ecologically relevant metric of feeding performance that has been associated with niche diversification and partitioning in terrestrial and aquatic taxa (Huber et al., 2009; Franco-Moreno et al., 2021; Masson et al., 2023). Feeding performance is often correlated with the ecological role of an organism, and can change considerably over ontogeny (Gignac and Erickson, 2016; Santana and Miller, 2016). Compared to adults, juvenile conspecifics are often at a disadvantage when accessing dietary resources due to smaller gapes and lower bite forces (Werner and Gilliam, 1984; Herrel and Gibb, 2006). Many species respond to this selection pressure by increasing bite force at a faster rate than body size, resulting in significant positive allometric scaling of bite force (e.g., Kolmann and Huber, 2009; Marshall et al., 2012; Erickson et al., 2014), in addition to increased jaw mineralization and changes in tooth morphology over ontogeny (Ferrara et al., 2011; Cullen and Marshall, 2019). Positive allometric scaling of bite force is often a result of increased cross-sectional area of the jaw closing muscles and/or mechanical advantage of the jaw lever system (Westneat, 2003; Herrel and Gibb, 2006). Since larger individuals are capable of handling most prey items owing to their larger absolute sizes and bite forces compared to smaller conspecifics, they are no longer under pressure to continue increasing bite force via positive allometric scaling (Herrel and Gibb, 2006; Huber et al., 2009; Habegger et al., 2012). Therefore, the timing of a shift in bite force scaling is likely of ecological significance.
The relationship between bite performance and the mechanical demands of prey processing can result in resource partitioning within or among species (Marshall et al., 2012; Santana and Miller, 2016). The critical period where bite force no longer limits feeding in large conspecifics often corresponds with a dietary shift in the size, diversity, or material properties of prey (Wainwright, 1988; Gignac and Erickson, 2016). While this relationship has been investigated in a variety of species (e.g., mammals, fishes, reptiles; Hernandez and Motta, 1997; Gignac and Erickson, 2016; Stanchak et al., 2023), there is limited information on elasmobranchs (sharks, skates, and rays; but see Kolmann and Huber, 2009).
Elasmobranchs perform a variety of ecological roles (e.g., direct predation, risk-mediated behavior in prey, nutrient transportation) and these roles change over ontogeny (Heithaus et al., 2010; Navia et al., 2016; Dedman et al., 2024). Known examples include sharks that undergo ontogenetic niche shifts that impact their trophic position, dietary breadth, and contributes to intra- and interspecific resource partitioning (Kinney et al., 2011; Werry et al., 2011; Navia et al., 2016). For some large-bodied species, this results in a transition from mesopredator to top predator (Daly et al., 2013; Heupel et al., 2014). A powerful method to discern the ecological niche and trophic relationships of these species includes the use of natural biochemical tracers such as stable isotopes.
Although stomach content analysis was traditionally the most common method to determine shark feeding ecology, this approach can be limiting since it only provides a snapshot of prey consumption. The application of stable isotope analysis (SIA) in ecological studies is now widespread since it can be performed non-lethally and generally does not require large sample sizes compared to stomach content analysis (Shiffman et al., 2012; Pethybridge et al., 2018). Bulk carbon (δ13C) and nitrogen (δ15N) stable isotopes are used to characterize carbon sources of food webs and trophic position, respectively (Peterson and Fry, 1987). This is due to predictable differences in trophic fractionation of each element, where there is minor enrichment of 13C and higher enrichment of 15N with each trophic level (Peterson and Fry, 1987; Post, 2002). These fractionation patterns facilitate the tracking of carbon sources and habitat (via δ13C) while also providing a proxy for trophic position (via δ15N). However, it appears that physiological condition can also impact the isotopic signature of sharks, such as when stressed or gravid (Karlson et al., 2018; Whiteman et al., 2018; Shipley et al., 2022). Therefore, δ13C and δ15N can be used in combination to estimate the ecological niche of sharks and any changes over ontogeny (Swanson et al., 2015; Matich et al., 2019).
Bull (Carcharhinus leucas), blacktip (Carcharhinus limbatus), and bonnethead sharks (Sphyrna tiburo) are sympatric species that use estuarine habitats as juveniles and transition to coastal environments during sub-adult and adult life stages (Bethea et al., 2015; Livernois et al., 2021). However, they exhibit different life history characteristics (e.g., maximum body size, age at maturity, fecundity) and ecological niches (Cliff and Dudley, 1991; Castro, 1996; Lombardi-Carlson et al., 2003; Gallagher et al., 2017; Peterson et al., 2020). Bull sharks are considered dietary generalists that feed on a wide range of prey (bony fishes, sea turtles, marine mammals, and other elasmobranchs), whereas blacktip and bonnethead sharks are both dietary specialists that primarily feed on bony fishes or benthic crustaceans, respectively (Cliff and Dudley, 1991; Plumlee and Wells, 2016). All three species purportedly undergo ontogenetic shifts in diet, but only bull and bonnethead sharks appear to consume increasingly greater proportions of potentially difficult-to-handle prey such as other elasmobranchs and hard-shelled crabs, respectively (Bethea et al., 2007; Werry et al., 2011; Matich et al., 2021a). These prey are difficult to process and consume due to their robust mechanical properties, which may require puncturing stiff, tough, or thick skin, as well as shearing calcified materials that include bone or shell (Frazzetta, 1988; Creager and Porter, 2018). Although previous studies have measured bite force or ecological niches in sharks (e.g., Mara et al., 2010; Habegger et al., 2012; Plumlee and Wells, 2016), the goals of this study were to compare bite force scaling patterns and ecological niches in species with known, well-established differences in prey preference, as well as compare how feeding performance impacts the trophic ecology of sharks both within and among species. To investigate these ecomechanical relationships in bull, blacktip, and bonnethead sharks, our objectives were to (1) estimate theoretical bite force and scaling patterns over ontogeny in small versus large conspecifics, (2) quantify niche breadth and overlap among species via stable isotopes, and (3) characterize the relationship between ecological niche shifts and changes in bite force over ontogeny.
2 Materials and methods
2.1 Sample collection
Bull (N = 31), blacktip (N = 42), and bonnethead sharks (N = 41) were opportunistically sampled from fishing charters or from routine long-line surveys conducted by Texas Parks and Wildlife Department in Galveston, Texas (29°18’51.8”N, 94°48’60.0”W) from March to October (2013 – 2016). All sharks caught from both sources complied with state and federal regulations based on species-specific size and catch limits per the status of the local stocks. These species were selected since they are common along the Texas coast and exhibit differences in prey preference. Of these 114 sharks, 82 were used to estimate theoretical bite force (bull: N = 24; blacktip: N = 30; bonnethead: N = 28) and 86 were used to analyze δ13C and δ15N stable isotopes (bull: N = 25; blacktip: N = 27; bonnethead: N = 34). Sample sizes varied between these analyses due to the availability of intact shark heads (for theoretical bite force measurements) across all age classes of each species. Sex was identified for each shark and measurements of total (TL; cm) and fork length (FL; cm) were recorded (Table 1). Muscle tissue samples (~ 5 g) were taken from the epaxial region near the anterior dorsal fin. Shark heads and muscle tissue samples were transported on ice for up to 30 minutes before storage at -20°C for further analysis. Muscle was used for isotopic analyses since it was readily available from the sampled sharks. However, shark muscle has isotopic incorporation rates on the scale of months to > 1 year, which may limit the detection of ontogenetic niche shifts (MacNeil et al., 2005; Kim et al., 2012; Malpica-Cruz et al., 2012). Due to this slow tissue turnover rate, the isotopic variation may be smoothed out across seasons when sharks were sampled, but it is possible that some seasonal effects may remain. Since the bias in shark size distributions across seasons (i.e., spring, summer, fall) is a confounding factor in our study that is exacerbated by limited sample sizes per species-season grouping (Supplementary Figure S1), we could not effectively account for variability in isotopic baselines resulting from seasonal movements.
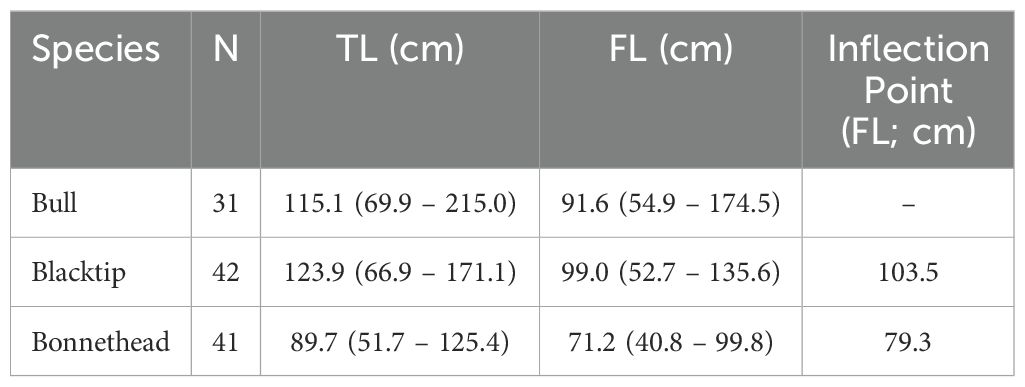
Table 1. Sample size (N), mean total length (TL; min – max), mean fork length (FL; min – max), and inflection point (as FL) of the generalized additive model separating small and large individuals for each shark species.
2.2 Theoretical bite force modeling
Unilateral dissections of the adductor mandibulae complex were performed on each specimen and individual muscles were identified following Motta and Wilga (1995). These jaw adductor muscles included the preorbitalis dorsalis (POD), preorbitalis ventralis (POV), quadratomandibularis dorsal divisions 1 – 4 (QD 1, QD 2, QD 3, QD 4), and quadratomandibularis ventral division (QV) (Figure 1). All subdivisions were measured separately except for the QD 2 and QD 3 subdivisions in bonnethead sharks since the small size of the QD 3 muscle made it difficult to isolate and measure without being damaged. Three-dimensional coordinates were recorded while the jaws were closed using a Patriot digitizer (Polhemus, Colchester, VT, USA) for the origin and insertion of each muscle subdivision, a single bite point (the anterior margin of the functional tooth row on the lower jaw), and the jaw joint. The tip of the snout was treated as the center of this coordinate system. Although measurements with the jaws fully closed may slightly underestimate maximal bite force (Ferrara et al., 2011), previous studies found no significant difference between this theoretical method of estimation and in vivo bite force measured from sharks under tetanic stimulation (Huber and Motta, 2004; Huber et al., 2005; Mara et al., 2010).
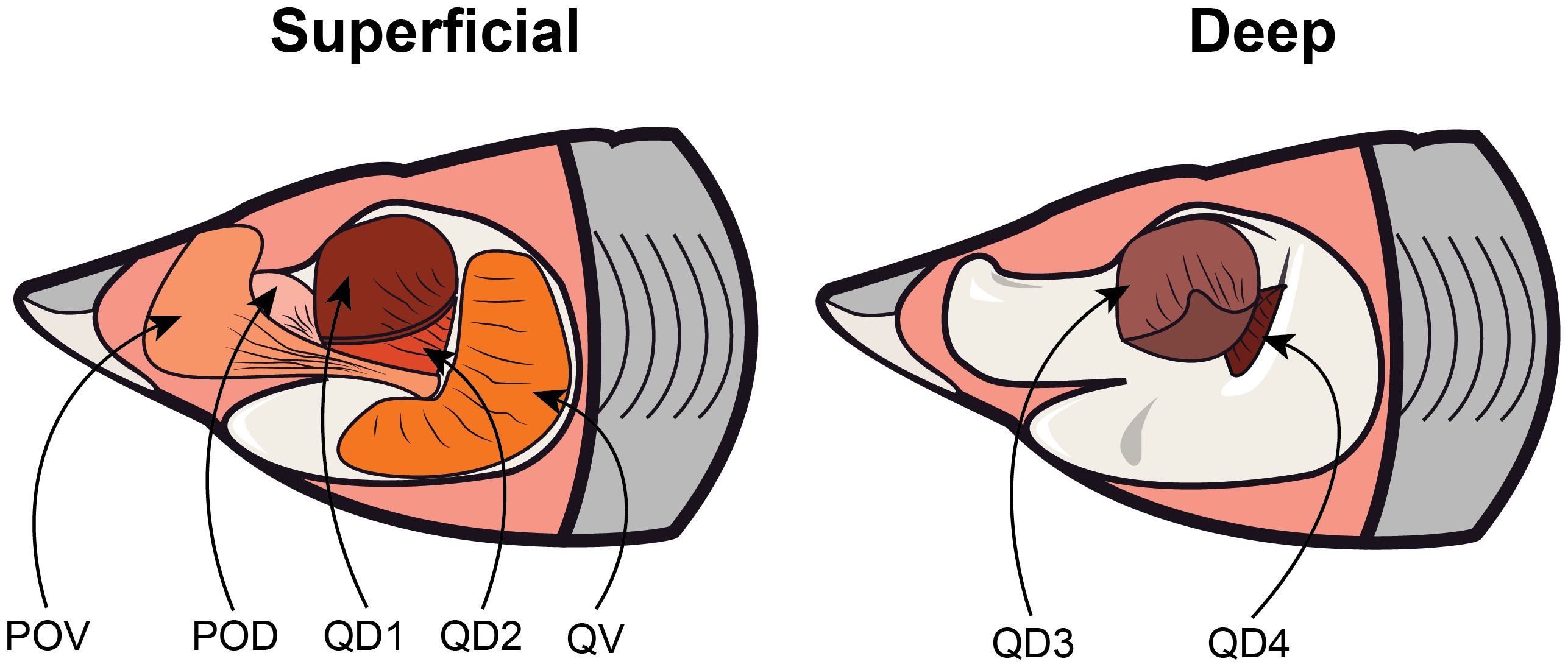
Figure 1. Anatomical illustration of the six jaw adductor muscles that were excised and measured to quantify theoretical bite force. Muscles are separated into a ‘superficial’ and ‘deep’ view to improve visibility of these muscles. Muscles shown here include the preorbitalis ventralis (POV), preorbitalis dorsalis (POD), quadratomandibularis dorsal divisions 1 – 4 (QD 1, QD 2, QD 3, QD 4), and quadratomandibularis ventral division (QV). Muscles QD1 and QD3 are shown pulled back to reveal underlying muscle divisions. .
Subsequently, each muscle subdivision was excised and bisected through the center of mass perpendicular to the principal fiber direction. The center of mass was determined by freely suspending each muscle from a pin at different points with a plumb line. These lines were traced, and their point of intersection denoted the center of mass for a given muscle (Huber and Motta, 2004; Habegger et al., 2012). Cross-sections of each muscle were photographed with a Fujifilm FinePix XP70 digital camera and the anatomical cross-sectional area (CSA; cm2) was measured using ImageJ (version 1.46; National Institutes of Health, Bethesda, MD, USA). To calculate the maximal force production or theoretical maximum tetanic tension (PO; N) of each muscle (Powell et al., 1984), CSAs of the POD, POV, QD 1, QD 2, QD 3, and QV were multiplied by the specific tension (TS; N cm-2) of shark white muscle (28.9 N cm-2), whereas the CSA of the QD 4 subdivision was multiplied by the TS of shark red muscle (14.2 N cm-2; Lou et al., 2002):
Three-dimensional force vectors were calculated using the coordinates of the origin and insertion points of each muscle and PO. In-lever (LI) distances were calculated as the distance between the insertion of each muscle subdivision and the jaw joint. A resolved in-lever (RLI) was calculated by using a weighted average of all individual LI based upon the proportional contribution of each muscle to total force production (Huber et al., 2005). Out-lever (LO) distance was calculated as the distance between the anterior bite point (ABP) and the jaw joint. Mechanical advantage (MA) for the ABP was calculated as the ratio of RLI to LO. Based upon a previously implemented theoretical bite force model used in multiple studies of elasmobranchs (Huber et al., 2005, 2006; Kolmann and Huber, 2009; Mara et al., 2010; Habegger et al., 2012), the present study developed a similar model in R v4.3.1 (R Core Team, 2023) using a custom script:
where ABF is the anterior bite force and FPOD, FPOV, FQD1, FQD2, FQD3, FQD4, FQV, are the force vectors generated by each subdivision of the adductor mandibulae complex. To achieve a measurement of ABF in the direction perpendicular to the jaws, a plane was created using the ABP and both jaw joint positions to generate an orthogonal unit vector ():
This was conducted by taking the cross product of unit vectors for the ABP () and the jaw joint () divided by the magnitude (Equation 5). New muscle forces () in the plane orthogonal to the ABP and jaw joint were generated by taking the dot product of the orthogonal unit vector and the original force vectors for each muscle subdivision () (Equation 6). This total unilateral force production was multiplied by the MA to account for the lever mechanics of the system and doubled to account for bilateral force production of the adductor mandibulae complex (Equation 7).
2.3 Stable isotope analysis
Muscle tissue samples were dried in an oven at 60°C for 72 h. Since lipids and nitrogenous compounds (urea and trimethylamine N-oxide) are known to alter both δ13C and δ15N values in elasmobranch muscle (Post et al., 2007; Logan and Lutcavage, 2010; Hussey et al., 2012; Kim and Koch, 2012), these compounds were extracted following the general recommendations of Carlisle et al. (2017). Lipids were extracted from muscle tissue samples by rinsing with petroleum ether in a Dionex Accelerated Solvent Extractor, followed by rinsing the samples with deionized water to remove nitrogenous compounds (Kim and Koch, 2012). Samples were subsequently dried in an oven for 24 h at 60°C to remove any residual solvent and then homogenized using a mortar and pestle.
Approximately 600 μg of each homogenized muscle tissue sample was processed and sent to the Light Stable Isotope Mass Spectrometry Lab at the University of Florida (Gainesville, FL, USA) for analysis. Carbon and nitrogen isotope composition was analyzed using a Carlo Erba NA 1500 elemental analyzer (Thermo Scientific, Waltham, MA, USA) coupled to a Thermo Delta V Advantage continuous flow isotope ratio mass spectrometer (Thermo Electron, Waltham, MA, USA). Stable isotope ratios are expressed in δ-notation as per mil (‰) using the following equation: δX = [(Rsample/Rstandard) – 1)] × 1000, where X is 13C or 15N and R is 13C/12C or 15N/14N. The standard reference material for δ13C was carbonate from Vienna Peedee Belemnite (VPDB) and atmospheric nitrogen (AIR) for δ15N. Analytical precision for instrumentation (as standard deviation; SD) was ± 0.10 and ± 0.08 ‰ for δ13C and δ15N, respectively. Instrumentation accuracy was determined based upon a USGS40 standard (L-glutamic acid), where mean (± SD) differences from certified values were 0.16 ± 0.09 ‰ for δ13C and 0.20 ± 0.20 ‰ for δ15N (n = 22 replicates).
2.4 Statistical analyses
All statistical analyses were performed in R v4.3.1 (R Core Team, 2023) and the level of significance was set at α = 0.05. Sharks within each species were separated into small and large size classes (when possible) to determine scaling relationships of bite force over ontogeny. Previous studies have shown that there is the potential for smaller individuals to exhibit positive allometric scaling of bite force, whereas adults may exhibit isometry (Herrel et al., 2005; Habegger et al., 2012). To determine a threshold for where this change was likely to occur, the root of the second derivative (inflection point) was determined from a generalized additive model (GAM) of ABF versus FL using the mgcv (v1.9.0; Wood, 2011) and gratia (v0.8.2; Simpson, 2024) R packages. These GAMs were fitted using a Gaussian response distribution with restricted maximum likelihood estimation (REML), where a thin plate regression spline with 5 basis functions was selected to constrain the flexibility of the smooth term. This relationship was evaluated using FL since it has been found to be a more precise measurement than TL (Kohler et al., 1996). Species size thresholds were expanded by 3 cm for both size classes per species to better capture the trend of the scaling relationships. Goodness-of-fit for these models were evaluated using QQ plots and plots of the residuals, which confirmed that these residuals were approximately normally distributed.
Scaling relationships of ABF over body size (FL) were evaluated for small and large classes of individuals within each species using the allometric power function Y = aXb, where a represents the y-intercept and b denotes the slope. This relationship was fit using Bayesian ordinary least squares (OLS) regression. We used OLS regression to estimate allometric scaling relationships since it has been shown to be relatively robust to small amounts of measurement error and concerns have been raised on the use of reduced major axis regression due to the error variance structure and symmetric relationship, among other issues (Smith, 2009; Kilmer and Rodríguez, 2017). The Bayesian regression was fit separately on each size class per species using a Gaussian response distribution with regularizing priors (on the scaling coefficients and standard deviation) using the rstan R package (Stan Development Team, 2023), where the no-U-turn sampler (NUTS) (Hoffman and Gelman, 2014) from Hamiltonian Monte Carlo was used via Stan. We ran 4 Markov chains of 4000 iterations each where the first 2000 were treated as the warmup, resulting in 8000 samples from the posterior distribution. Model convergence was assessed by inspecting trace plots of the parameters, effective sample size, and the diagnostic (where < 1.01 is indicative of model convergence by multiple chains exhibiting stationarity and reaching a common distribution; Gabry et al., 2019; Vehtari et al., 2021). Goodness-of-fit was assessed via posterior predictive checks and R2 for Bayesian regression (Gabry et al., 2019; Gelman et al., 2019). After the model was run, trace plots exhibited stationarity, effective sample size was relatively high, and all values were ≤ 1.002, indicating the model had converged on the posterior distribution and there was low sample autocorrelation. Since the predicted isometric slope of force over body length is 2 (Hill, 1950), all estimates >2 were indicative of positive allometry, values <2 were considered negative allometry, and values no different from 2 were considered to be isometric.
Changes in δ13C and δ15N over FL were analyzed by species to investigate any potential ontogenetic niche shifts. These models were fit using GAMs since most relationships appeared to be non-linear. When fitting these models, we used a Gaussian response distribution and REML for smoothing parameter estimation via the mgcv R package. The smooth term was estimated using thin plate regression splines with five basis functions to constrain the flexibility of this relationship. Goodness-of-fit was evaluated by inspection of QQ plots and plots of the residuals, which confirmed that these residuals were approximately normally distributed.
Isotopic niche breadth was estimated for each species to evaluate the relative resource use of these sympatric sharks. We estimated niche breadth as 95% probability ellipse areas via Bayesian inference using the nicheROVER package (v1.1.2; Lysy et al., 2023) in R. This was performed by drawing 5000 samples from the posterior distribution (Normal-Inverse-Wishart) for each of the parameters (covariance matrix, mean δ13C, mean δ15N) (Swanson et al., 2015). To compare isotopic niche breadth among the three species, we conducted pairwise comparisons by calculating the percentage of posterior estimates that were larger in each species relative to the total number of samples from the posterior distribution (i.e., 5000; Daly et al., 2013; Yurkowski et al., 2018). Although sampling bias of shark body sizes across species and seasons was present for our data, we also performed the same steps to estimate niche breadth for these subgroups as an exploratory analysis to evaluate variability within species.
Isotopic niche overlap was evaluated to determine the extent to which species may be using the same resources. Niche overlap was defined as the probability of one species’ niche falling within the niche space of another (Swanson et al., 2015) and was calculated using the nicheROVER R package. Due to the asymmetric directionality of this measure, niche overlap was calculated in each direction per pair of species. This was performed using the 95% probability ellipses of niche breadth, where uncertainty in niche overlap was reported using Bayesian 95% credible intervals.
3 Results
3.1 Scaling of bite force
Theoretical measures of bite force displayed sigmoid patterns over ontogeny in blacktip and bonnethead sharks, with greater than 10-fold increases in bite force across all species (Supplementary Figure S2). For a given body size, bite force was greatest in bull sharks, followed by blacktip and bonnethead sharks. Bite force values ranged from 70 to 1297 N in bull sharks, 30 to 448 N in blacktip sharks, and 8 to 104 N in bonnethead sharks (Supplementary Figure S2). By evaluating the derivative of the GAMs characterizing bite force over ontogeny, inflection points were found for blacktips (103.5 cm FL) and bonnetheads (79.3 cm FL), but not bull sharks. The lack of a detected inflection point in bull sharks was likely due to the inclusion of few adults that were also on the smaller end of this age class. Since an inflection point was not found for bull sharks, they were analyzed as a single group.
Scaling relationships of bite force over increasing body length were generally the same when comparing among species of the same size class (small/large) but differed between these groups within each species. When all bull sharks were analyzed together, ABF scaled with significant positive allometry (Table 2; Figure 2). While ABF of small blacktips also scaled with significant positive allometry, the scaling relationship was isometric for large blacktip sharks (Table 2; Figure 2). Likewise, the scaling model estimated a positive allometric relationship for small bonnethead sharks, but an isometric relationship for large conspecifics (Table 2; Figure 2). Uncertainty in the slope estimates varied by species and size class, but was smallest for the bull shark model that included individuals of all sizes (Table 2).
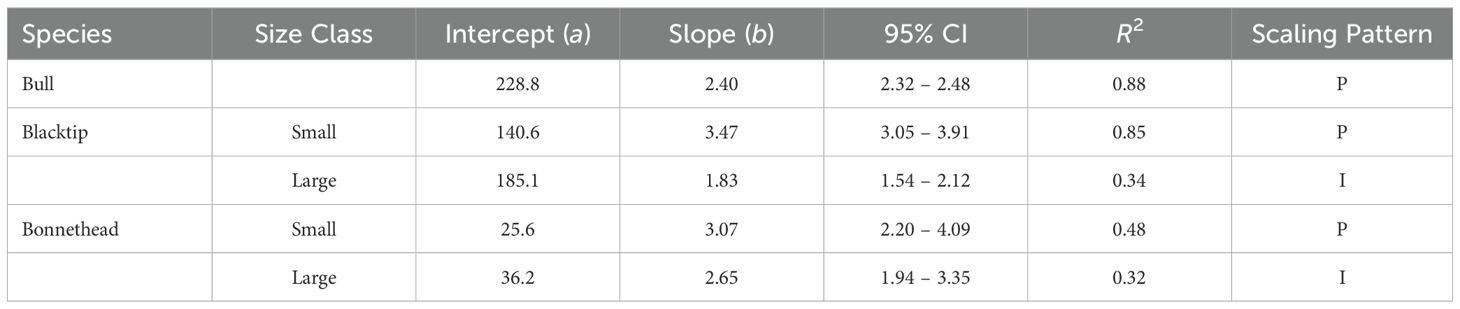
Table 2. Model coefficients for scaling relationships of bite force over ontogeny across all three shark species. Slopes were compared against isometric relationships (slope = 2) to determine if they exhibited positive allometry (P), negative allometry (N), or isometry (I) using Bayesian 95% credible intervals (CI).
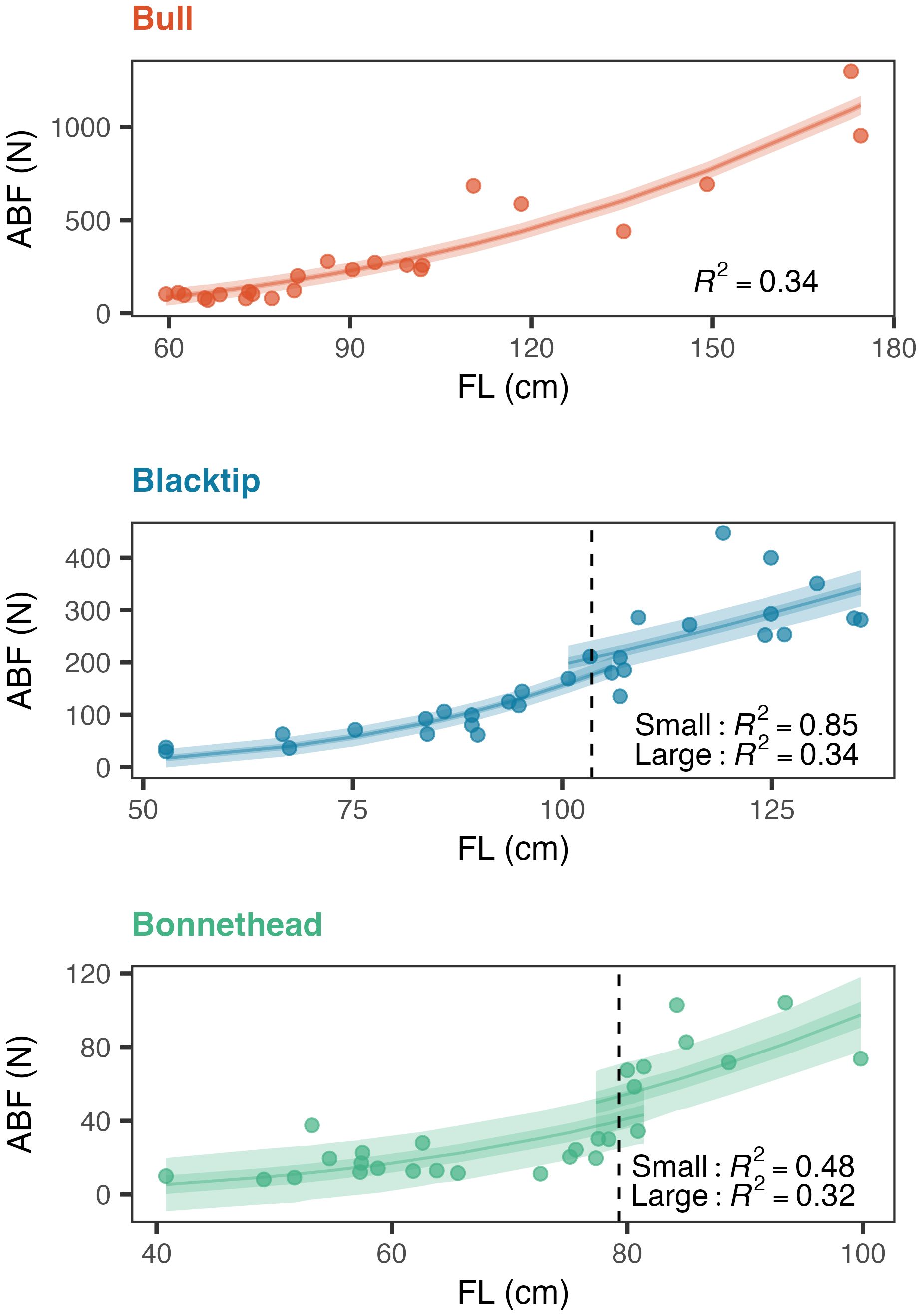
Figure 2. Estimated anterior bite force (ABF) scaling relationships over body size (fork length; FL) in bull, blacktip, and bonnethead sharks. Vertical dashed lines denote the estimated thresholds for distinguishing small from large conspecifics. Mean scaling relationships are shown (solid, colored lines) for each species in addition to uncertainty (50 and 95% credible intervals) in these estimates.
3.2 Patterns of δ13C and δ15N over ontogeny
All prepared muscle samples had C:N ratios within the recommended range below 3.5 (mean ± SD: 3.2 ± 0.1), indicating that lipids were not interfering with interpretation of δ13C values (Post et al., 2007). All three species exhibited significant changes in δ13C over ontogeny (p < 0.05) that each differed from one another, where bull and bonnethead sharks displayed non-linear patterns (Figure 3). Specifically, bull sharks showed a rapid decrease in δ13C until reaching ~80 cm FL, after which δ13C only slightly increased over ontogeny. By comparison, blacktip sharks displayed a relatively linear increased in δ13C over ontogeny and bonnethead sharks exhibited a parabolic increase and subsequent decrease of δ13C that peaked at ~80 cm FL. Likewise, only blacktip sharks showed a significant change in δ15N over ontogeny, where δ15N values slightly decreased until reaching ~80 cm FL after which it increased (Figure 3). It should be noted that, while blacktip sharks displayed statistically significant isotopic shifts over ontogeny, the magnitude of these changes were only approximately 1‰.
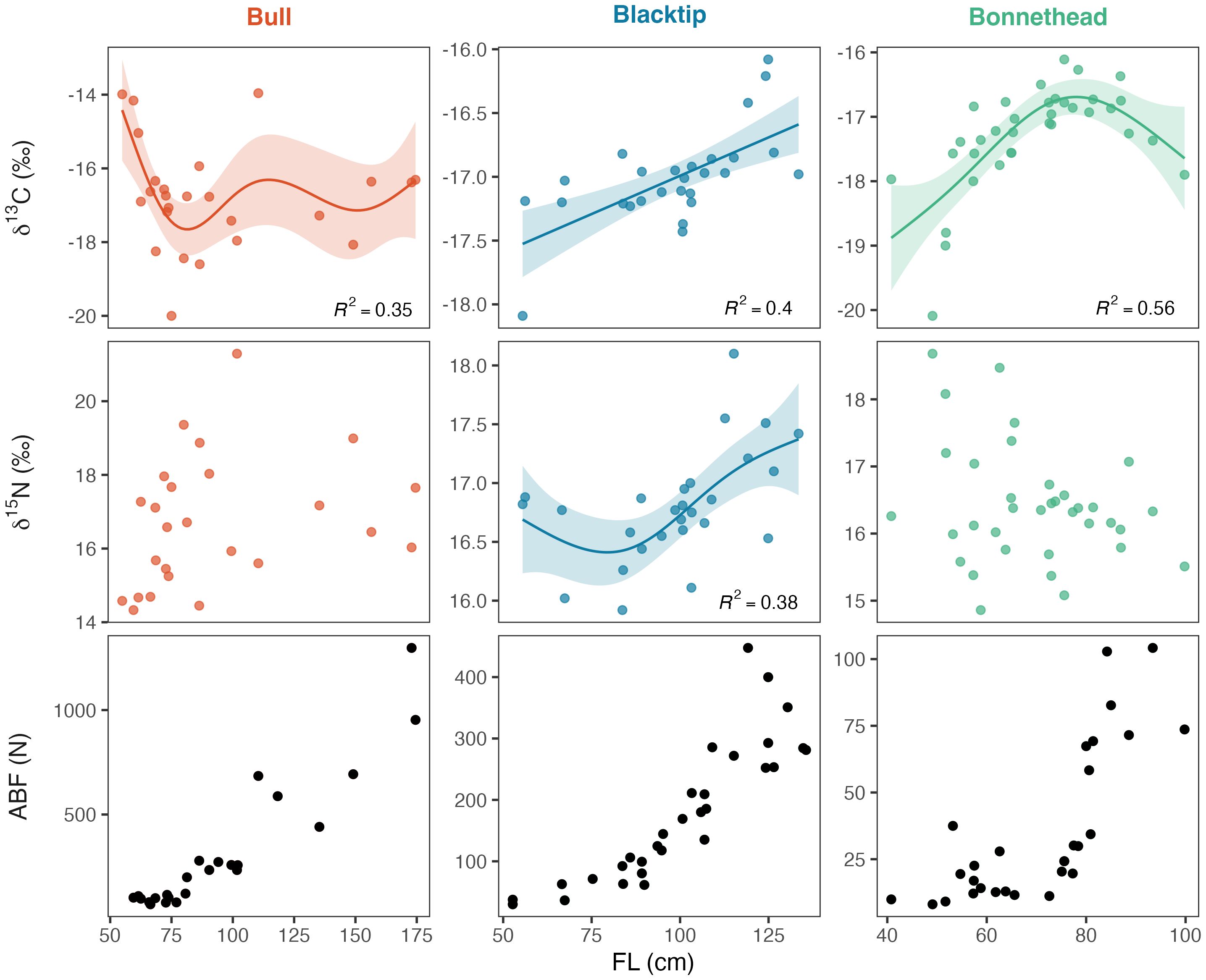
Figure 3. Relationships between fork length (FL) and δ13C, δ15N, and anterior bite force (ABF) in bull, blacktip, and bonnethead sharks. Only significant relationships between FL and stable isotopes (δ13C and δ15N) are shown.
In bull and bonnethead sharks, isotopic niche shifts appeared to coincide with rapid increases in bite force (Figure 3). Bull sharks displayed notable increases in bite force at 75-80 cm FL, which was also the body size range where δ13C started to increase. Although the pattern for δ15N was less clear in bull sharks, it was at this body size that an asymptote appeared to be reached (Figure 3). In bonnethead sharks, the sharp increase in bite force occurred where the relationship between δ13C and body size reached its peak (Figure 3). As in bull sharks, the ontogenetic trend in δ15N was not significant, but variability in δ15N appeared to decrease during the period of rapidly increasing bite force in bonnetheads. Unlike in the other two species, increases in blacktip bite force were also associated with increases in δ13C and δ15N with no visible niche shifts occurring (Figure 3).
3.3 Isotopic niche breadth and overlap
Bull sharks exhibited the largest isotopic niche breadth among all three species, which was due to greater variability in both δ13C and δ15N (Figure 4; Table 3). Niche breadth of bonnethead and blacktip sharks were approximately 3× and 10× smaller than bull sharks, respectively. However, mean isotopic values were very similar across all species. Pairwise species comparisons showed that bull sharks exhibited the greatest niche breadth of all three species with 100% probability, whereas bonnethead sharks showed greater niche breadth than blacktips with 100% probability. In the exploratory analysis of intraspecific niche variability among seasons, there did appear to be minor differences in the estimated niche ellipses and niche breadth per species (Supplementary Figures S3, S4). While it is possible that seasonal movements may have contributed to differences in species’ niches, we cannot rule out the effects of shark body size as being more influential. Importantly, differences among seasons within species was much lower than differences among species per season.
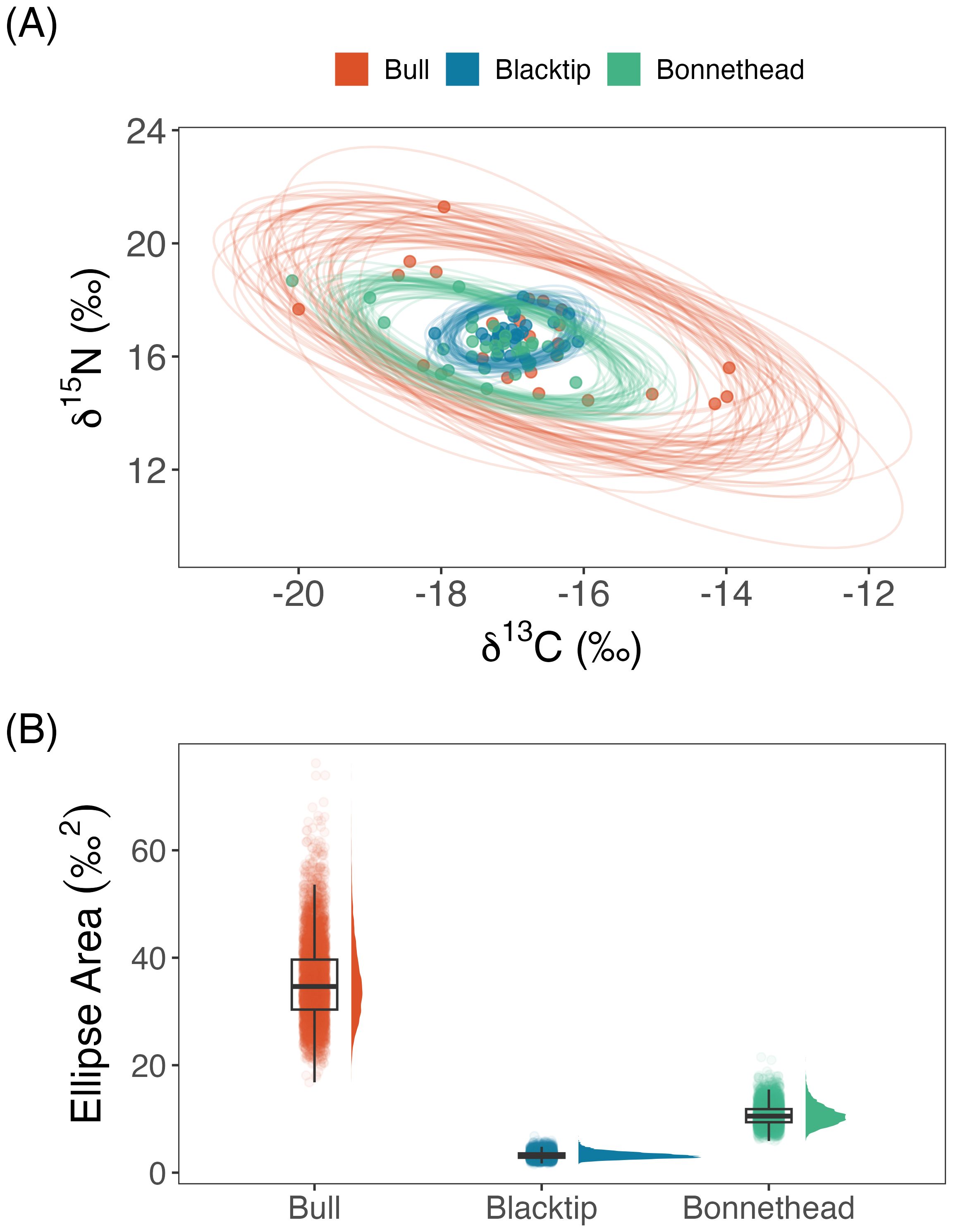
Figure 4. Isotopic niche breadth is compared among bull, blacktip, and bonnethead sharks using δ13C and δ15N. (A) Niche space is shown as an isotopic biplot, which includes a sample of 50 estimated 95% ellipses drawn from the posterior distribution per species. (B) Niche breadth is compared among species based on the estimated ellipses from the full posterior distribution. Comparisons are shown using individual point estimates, as well as boxplots and density plots that summarize these distributions.
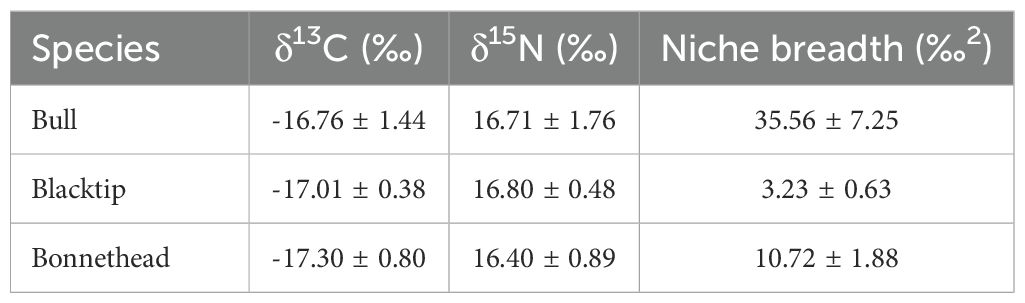
Table 3. Summary of δ13C, δ15N, and isotopic niche breadth (mean ± SD) for all shark species sampled from Galveston, Texas, USA.
The extent of niche overlap varied widely among species, but the isotopic niches of blacktip and bonnethead sharks fell entirely within that of bull sharks (Figures 4A, 5). Blacktip shark niche area was entirely overlapped by the bull shark niche with a mean (95% CI) of 100% (100-100%) overlap. Similarly, the bonnethead isotopic niche was fully overlapped by that of the bull shark niche with a mean (95% CI) of 99% (94-100%) overlap. The next highest level of niche overlap was that of blacktip sharks by bonnetheads, where the mean (95% CI) probability of overlap was 94% (81-100%). In contrast, overlap was lowest when inspecting these relationships in the opposite direction. Specifically, blacktips overlapped bull shark niche space at the lowest probability, with a mean estimate of 22%.
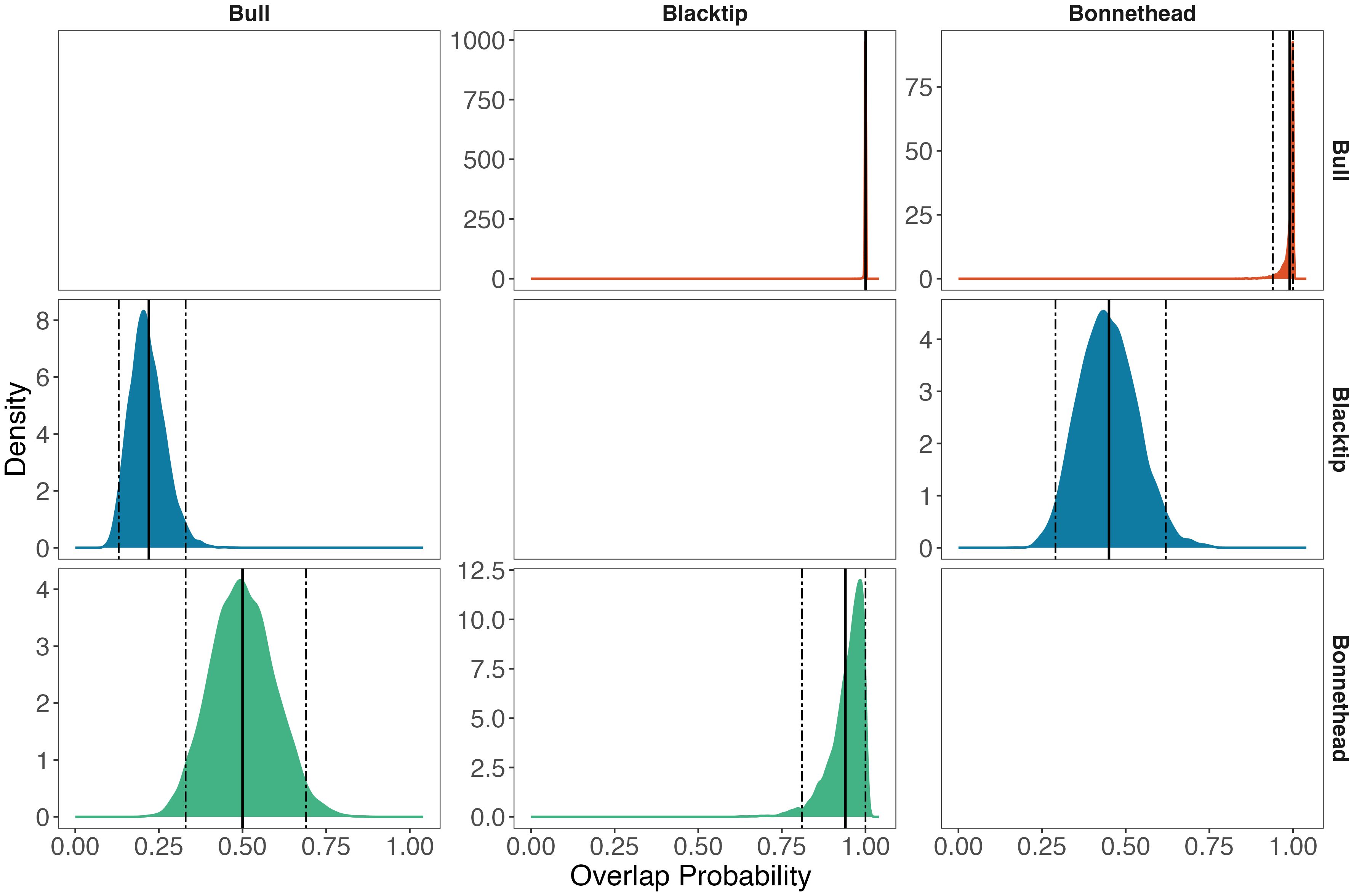
Figure 5. Distributions of estimated niche overlap between pairs of species based on samples from the Bayesian posterior distribution. Overlap is presented as the probability that the isotopic niche of the column species falls within the isotopic niche of the row species. The solid vertical lines denote the mean estimate, while dashed vertical lines denote the 95% credibility interval.
4 Discussion
This study demonstrates that scaling patterns of bite force differ between small and large size classes of blacktip and bonnethead sharks, which supports the findings of Habegger et al. (2012) and suggests that this relationship may be occurring in elasmobranchs more widely. Smaller sharks exhibited significant positive allometric scaling, whereas larger sharks displayed isometric scaling of bite force. This rapid increase in ABF is expected to be especially important at small body sizes, which could allow greater access to dietary resources compared to co-occurring predators that experience isometric ontogenetic trajectories (Hernandez and Motta, 1997; Kolmann and Huber, 2009; Habegger et al., 2012). Once sharks attain large body sizes, selection pressure on maximal bite force is likely relaxed due to the ability of these predators to puncture or crush most prey items (Aguirre et al., 2003; Herrel and Gibb, 2006; Huber et al., 2009). However, this may not be the case for durophagous bonnethead sharks, which do not necessarily crush the carapace of large crab prey before consumption and may rely more heavily on chemical digestion (Myrberg and Gruber, 1974; Wilga and Motta, 2000; Mara et al., 2010; Jhaveri et al., 2015). This pattern of positive allometric scaling at small size classes followed by isometric scaling of bite force at large size classes differs from the majority of other studies that have investigated scaling of bite force over ontogeny (e.g., in fishes, reptiles, mammals, birds; van der Meij and Bout, 2004; Marshall et al., 2012; Law et al., 2016; Kolmann et al., 2018) since they evaluated all conspecifics together; by doing so, any potential size-specific differences in scaling patterns have been obscured (Habegger et al., 2012). Therefore, future studies should evaluate juveniles and adults separately when estimating bite force scaling patterns over ontogeny.
For bull and blacktip sharks, the mean maximum force required by their teeth to puncture teleost or elasmobranch prey is 40.93 N and 17.08 N, respectively (Whitenack and Motta, 2010). Since these forces are achieved by even the smallest conspecifics measured in the present study (bull: 70.22 N, blacktip: 29.96 N), other contributing factors likely influence the ability of these sharks to consume potential prey. These factors may include greater forces required to fracture skeletal elements of prey, as well as the ability to maintain a firm grip on large prey during lateral head-shaking behavior for the removal of smaller pieces (Huber et al., 2006; Habegger et al., 2012). High bite forces in lacertid lizards corresponded with increased prey handling efficiency (Herrel et al., 2001; Verwaijen et al., 2002). A similar pattern was found in finches with respect to seed husking time (van der Meij and Bout, 2006). This would result in an increase in net energy intake for an individual and would likely enhance fitness (Emerson et al., 1994; Anderson et al., 2008; Pfaller et al., 2011; Timm, 2013). Some sharks may also exhibit this relationship, but this has yet to be directly tested.
Although the limited number of large bull sharks in this study likely prevented the separation of small and large size classes to estimate different scaling relationships, Habegger et al. (2012) also estimated positive bite force scaling in small conspecifics and isometric scaling in large individuals. In addition to this relationship, multiple sources of ecological data for bull sharks from this study and prior studies strongly support a shift in habitat and diet concurrent with a change in bite force scaling pattern from positive allometry to isometry. Young-of-the-year (YoY) bull sharks use the freshwater-influenced upper reaches of bays and estuaries as nursery habitats and move progressively towards marine environments as they get larger (Heupel et al., 2010; Werry et al., 2011). This freshwater to marine transition was observed by shifts in both δ13C (this study; Werry et al., 2011) and trace elements in their vertebrae (Werry et al., 2011; Livernois et al., 2021). High δ13C values observed in neonates is likely a residual maternal isotopic signature, which impedes the ecological interpretation of these data (Matich et al., 2010; Olin et al., 2011). Similar to the ontogenetic pattern of δ15N measured by Werry et al. (2011), our study also found a (non-significant) curvilinear trend of increasing δ15N over ontogeny that reached an asymptote. While this is may be a result of bull sharks reaching their highest trophic position in the food web (Werry et al., 2011; Daly et al., 2013), Matich et al. (2021b) reported that δ15N actually decreased with increasing salinity where larger bull sharks were caught. Therefore, this relationship may be influenced by a spatially variable δ15N baseline at the study area. No maternal isotopic signal was observed for δ15N in neonates (unlike δ13C), which may result from these young sharks feeding from an equivalent δ15N baseline compared to the habitat of their mothers (Olin et al., 2011). In addition to these relationships, a rapid habitat transition (based on vertebral δ13C and trace elements) occurs at approximately 130 cm TL (~104 cm FL; Werry et al., 2011; Livernois et al., 2021), which falls towards the end of the positive allometric scaling phase of ABF in bull sharks (Habegger et al., 2012). This period of rapidly increasing bite force may facilitate the shift in habitat and diet of bull sharks by allowing them to consume prey in new habitats that are larger or more difficult to process (e.g., puncture), but also provide a greater source of energy for growth and development (Snover, 2008; Habegger et al., 2012; Hussey et al., 2017). This idea is supported by findings from Werry et al. (2011), which found that bull sharks larger than 120 cm TL (~96 cm FL) displayed greater dietary breadth that included the consumption of larger prey, including greater proportions of elasmobranchs, reptiles, and birds. Additionally, a reduction in predation risk once bull sharks attain larger sizes has been suggested as a driver of shifts to coastal habitats in this species (Simpfendorfer et al., 2005; Werry et al., 2012).
Unlike bull sharks, blacktip sharks did not appear to demonstrate major shifts in habitat or diet in association with bite force. No changes in isotopic patterns were found to precede or follow the change in scaling pattern of ABF in blacktip sharks, where only a slight change in habitat and diet was found based on stable isotopes. This aligns with numerous studies in vertebrates, where there is a lack of association between diet and changes in bite force (Huber et al., 2006; Habegger et al., 2011; Ferguson et al., 2015). YoY and juvenile blacktip sharks are typically found in the brackish waters of estuaries and bays as part of their nursery habitat before moving into nearshore coastal habitats as sub-adults and adults (Castro, 1996; Heupel and Hueter, 2002; Livernois et al., 2021). No maternal signal was observed for δ13C in this species in the present study, where the range in observed values was ~2‰. The increase in δ13C over ontogeny is likely a result of moving from estuarine nursery habitats to coastal areas as well as an increase in trophic position, which are both expected to result in higher δ13C values (Peterson and Fry, 1987; Garcia et al., 2007; Pethybridge et al., 2018). A significant non-linear relationship in δ15N over ontogeny showed increasing δ15N values from YoY blacktips through juvenile and adult size classes. Similar to δ13C, there was only a small difference (~2‰) between the minimum and maximum δ15N values measured. This may also indicate a slight dietary shift, or possibly a shift in baseline δ15N in the diet of these age classes. A previous study of blacktip shark trophic ecology found no ontogenetic changes in δ15N, but did record major changes in prey composition over ontogeny when analyzing stomach contents (Matich et al., 2021a). Moreover, this study found that smaller blacktips fed primarily on soft-bodied clupeid fish and penaeid shrimp prey (e.g., white shrimp Litopenaeus setiferus, Atlantic croaker Micropogonias undulatus), whereas larger conspecifics often consumed functionally difficult sciaenid, ariid, and elasmobranch prey (e.g., red drum Sciaenops ocellatus, gafftopsail catfish Bagre marinus, Atlantic sharpnose shark Rhizoprionodon terraenovae) (Matich et al., 2021a). Therefore, these increases in bite force may result in greater capture and handling success for these larger and more functionally difficult prey species at sub-adult and adult size classes.
A shift in the habitat of bonnethead sharks preceded the change in the pattern of ABF scaling, but did not show a clear change with diet. Similar to blacktip sharks, bonnetheads also use estuaries and bays as nurseries before moving into coastal marine habitats (Froeschke et al., 2010; Bethea et al., 2015). This habitat shift is also associated with an increasing reliance on benthic prey species as adults, including blue crabs (Callinectes sapidus) and other crustaceans (Cortés et al., 1996; Bethea et al., 2007; Plumlee and Wells, 2016). The trend of decreasing δ13C in the largest bonnetheads in our study may be due to seasonal movements offshore to follow migrating blue crabs during spawning periods (Heupel et al., 2006; Driggers et al., 2014; Plumlee and Wells, 2016). Bonnethead sharks consume an increasing proportion of blue crabs over ontogeny, and this is best characterized as a quadratic relationship between predator and prey body size (Cortés et al., 1996). However, a large increase in bite force may not be necessary to consume all crab prey since approximately 20% of crabs found in the stomachs of juvenile bonnetheads (~60 – 75 cm FL) could not be crushed by sharks in this size range (Mara et al., 2010). Therefore, this large increase in ABF may only occur in adult bonnethead sharks to provide greater access to much larger crabs than could be consumed by YoY and juveniles due to bite force or gape limitations.
Bull sharks showed the greatest niche breadth among the species investigated, which supports findings from previous studies. Bull sharks are well-documented generalists at the population level (Snelson et al., 1984; Werry et al., 2011; TinHan and Wells, 2021). Previous studies measuring the isotopic niche of bull sharks did not share similar values for niche breadth (due to different metrics), but they did observe large niche breadths in this species across different life stages (Daly et al., 2013; Every et al., 2017; Gallagher et al., 2017). Bonnethead sharks exhibited the next largest niche breadth (mean: 10.72‰2), but these values were closer to those measured in blacktips (mean: 3.23‰2) than bull sharks (mean: 35.56‰2). This corroborated the results of Gallagher et al. (2017), which measured significantly smaller niche breadth in blacktips compared to bull sharks, as well as other studies that reported larger niche sizes in bonnetheads compared to blacktip sharks (Peterson et al., 2020; Plumlee et al., 2023). Therefore, our findings are parsimonious with previous studies that have used stomach contents and stable isotopes to classify bull sharks as dietary generalists, while classifying blacktip and bonnethead sharks as specialists. The exploratory analysis of intraspecific variability in niche breadth showed relatively minor differences in conspecifics across seasons, especially compared to differences found among species. However, the sampling bias in our study likely precluded a more thorough examination of the impact of seasonal movements on estimated niche breadth. Additionally, our isotope values are not able to resolve individual-level specialization (or generalism) since we only use a single tissue sample per individual and did not collect environmental samples to determine proportions of prey contributions to a shark’s diet. Therefore, further studies are warranted that more thoroughly investigate dietary preferences of these species through multi-tissue analysis of isotopic tracers as well as account for variation in isotopic baselines due to seasonal movements.
The generalist diet of bull sharks coincides with the large bite forces they achieve, which is greater than the force required to puncture their putative prey, even after one year of growth. The tooth morphology of this species also suggests a generalist diet, where the high extent of heterodonty in bull sharks can be used to shear pieces of tissue from large prey items or prey that are otherwise functionally difficult to consume (Cullen and Marshall, 2019). By comparison, the smaller niche breadth of durophagous bonnethead and piscivorous blacktip sharks reflects their dietary specialization (Plumlee and Wells, 2016; Matich et al., 2021a). Both of these species also possessed a dentition that is expected to be best-suited to their dietary preferences, with gracile teeth present in blacktips and molariform teeth found in bonnethead sharks (Cullen and Marshall, 2019). However, it is worth noting that bonnetheads frequently ingest aquatic vegetation (likely while attempting to capture benthic prey), and have been reported to digest and obtain nutrients from these items due to the presence of enzymes in the hindgut that can break down plant materials (Leigh et al., 2018).
In addition to their larger niche breadth, bull sharks fully overlapped the isotopic niche space of both blacktip and bonnethead sharks. Since teleost fishes represent a major prey item of both bull and blacktip sharks, it is not surprising that the entire isotopic niche of blacktips fell within that of bull sharks since the latter is a dietary generalist. Despite the small proportion of benthic invertebrates consumed by bull sharks (TinHan and Wells, 2021), the isotopic niche of this species exhibited a high level of overlap with bonnethead sharks. Since the isotopic niches of these species are only measured in two dimensions, this is likely an artifact of not including a more discriminating isotopic marker (e.g., δ34S) for differentiating prey from benthic environments relative to water column species (Plumlee and Wells, 2016; Weber et al., 2023; Raoult et al., 2024). However, this high level of overlap may also be influenced by 15N-enriched nutrient run-off, which would alter the isotopic baseline of these coastal and bay systems that receive high freshwater input from the highly developed Greater Houston Area (McClelland et al., 1997; Vander Zanden et al., 2005; Matich et al., 2021b). The high level of niche overlap of bonnethead sharks onto blacktip sharks is likely reflective of the same issues faced when comparing the former against bull sharks. Plumlee and Wells (2016) found that using δ34S helped discriminate species’ niches, where δ34S was more depleted in bonnethead sharks than blacktip sharks. This is indicative of bonnethead sharks primarily foraging on epibenthic prey, whereas blacktip sharks forage higher in the water column (Peterson and Fry, 1987; Plumlee and Wells, 2016). Therefore, niche overlap is likely lower than calculated between the epipelagic (bull and blacktip sharks) and demersal species (bonnethead sharks) in this study.
5 Conclusions
This study demonstrates the value of integrating animal performance measures with natural isotopic tracers to characterize how feeding biomechanics may play a role in influencing ecological relationships. The interdisciplinary nature of this study provides a new perspective that is often overlooked in how animals interact with their environment and we recommend future studies also consider an integrative approach to address species-habitat interactions. We found that scaling patterns of bite force in bull, blacktip, and bonnethead sharks were consistent across species and were also informative in characterizing ontogenetic niche shifts. Associations between bite force and ontogenetic niche shifts in habitat and diet were found in bull and bonnethead sharks, but not in blacktip sharks. The lack of major changes in habitat or diet for smaller blacktips that exhibited positive scaling of bite force may be the result of not accounting for the size and material properties of prey items compared to the use of natural isotopic tracers. All three species appeared to exhibit a habitat shift (to varying degrees) from nurseries within estuaries to coastal habitats in the Gulf of Mexico based upon changes in δ13C, which occurred concurrently with increases in bull shark trophic position and specialization of bonnetheads on low trophic level crustaceans. Estimation of isotopic niche breadth confirmed that bull sharks are dietary generalists, whereas blacktip and bonnethead sharks were dietary specialists. Niche overlap between bonnethead sharks and the other two species was higher than expected using δ13C and δ15N isotopes. Future studies should evaluate net energy intake of primary prey items within each species to determine if changes in energetic requirements over ontogeny (with changes in body size and metabolic state) are important drivers of bite force scaling and ontogenetic niche shifts.
Data availability statement
The datasets presented in this study can be found in online repositories. The names of the repository/repositories and accession number(s) can be found below: https://zenodo.org/doi/10.5281/zenodo.12210047.
Ethics statement
The animal study was approved by Texas A&M University Institutional Animal Care and Use Committee. The study was conducted in accordance with the local legislation and institutional requirements.
Author contributions
JC: Conceptualization, Data curation, Formal analysis, Funding acquisition, Investigation, Methodology, Software, Validation, Visualization, Writing – original draft, Writing – review & editing. CM: Conceptualization, Funding acquisition, Investigation, Methodology, Project administration, Resources, Supervision, Writing – review & editing.
Funding
The author(s) declare financial support was received for the research, authorship, and/or publication of this article. This work was supported by a Merit Fellowship from Texas A&M University, an Excellence Fellowship from the College of Agriculture and Life Sciences at Texas A&M University, as well as a Graduate Boost Fellowship from the Research and Graduate Studies Office at Texas A&M University at Galveston. This work was funded in part by Texas Sea Grant Grants-in-Aid of Graduate Research, Erma Lee and Luke Mooney Graduate Travel Grants, and Texas A&M University Department of Marine Biology Mini Grants.
Acknowledgments
We would like to thank Erica Atkins, Thomas TinHan, Jeffrey Plumlee, Patricia Faulkner, Emily Williams, Katherine Adams, Nicole Glenn, and Aubree Jones for collecting samples that were used in this study. We would also like to thank Daniel Gutierrez, Kendal Clark, Aubree Jones, Sarah Hoskinson, Alexis Hornsby, and Erica Atkins for assistance with lab work. We also thank Dr. Blair Sterba-Boatwright for his valuable assistance in developing a bite force scaling model.
Conflict of interest
The authors declare that the research was conducted in the absence of any commercial or financial relationships that could be construed as a potential conflict of interest.
Publisher’s note
All claims expressed in this article are solely those of the authors and do not necessarily represent those of their affiliated organizations, or those of the publisher, the editors and the reviewers. Any product that may be evaluated in this article, or claim that may be made by its manufacturer, is not guaranteed or endorsed by the publisher.
Supplementary material
The Supplementary Material for this article can be found online at: https://www.frontiersin.org/articles/10.3389/fmars.2024.1452984/full#supplementary-material
References
Aguirre L. F., Herrel A., Van Damme R., Matthysen E. (2003). The implications of food hardness for diet in bats. Funct. Ecol. 17, 201–212. doi: 10.1046/j.1365-2435.2003.00721.x
Anderson R. A., McBrayer L. D., Herrel A. (2008). Bite force in vertebrates: opportunities and caveats for use of a nonpareil whole-animal performance measure. Biol. J. Linn. Soc. 93, 709–720. doi: 10.1111/j.1095-8312.2007.00905.x
Bethea D. M., Ajemian M. J., Carlson J. K., Hoffmayer E. R., Imhoff J. L., Grubbs R. D., et al. (2015). Distribution and community structure of coastal sharks in the northeastern Gulf of Mexico. Environ. Biol. Fishes 98, 1233–1254. doi: 10.1007/s10641-014-0355-3
Bethea D. M., Hale L., Carlson J. K., Cortés E., Manire C. A., Gelsleichter J. (2007). Geographic and ontogenetic variation in the diet and daily ration of the bonnethead shark, Sphyrna tiburo, from the eastern Gulf of Mexico. Mar. Biol. 152, 1009–1020. doi: 10.1007/s00227-007-0728-7
Carlisle A. B., Litvin S. Y., Madigan D. J., Lyons K., Bigman J. S., Ibarra M., et al. (2017). Interactive effects of urea and lipid content confound stable isotope analysis in elasmobranch fishes. Can. J. Fisheries Aquat. Sci. 74, 419–428. doi: 10.1139/cjfas-2015-0584
Castro J. I. (1996). Biology of the blacktip shark, Carcharhinus limbatus, off the southeastern United States. Bull. Mar. Sci. 59, 508–522.
Cliff G., Dudley S. F. (1991). Sharks caught in the protective gill nets off Natal, South Africa. 4. The bull shark Carcharhinus leucas Valenciennes. South Afr. J. Mar. Sci. 10, 253–270. doi: 10.2989/02577619109504636
Cortés E., Manire C. A., Hueter R. E. (1996). Diet, feeding habits, and diel feeding chronology of the bonnethead shark, sphyrna tiburo, in Southwest Florida. Bull. Mar. Sci. 58, 353–367.
Creager S. B., Porter M. E. (2018). Stiff and tough: a comparative study on the tensile properties of shark skin. Zoology 126, 154–163. doi: 10.1016/j.zool.2017.10.002
Cullen J. A., Marshall C. D. (2019). Do sharks exhibit heterodonty by tooth position and over ontogeny? A comparison using elliptic Fourier analysis. J. Morphology 280, 687–700. doi: 10.1002/jmor.20975
Daly R., Froneman P. W., Smale M. J. (2013). Comparative feeding ecology of bull sharks (Carcharhinus leucas) in the coastal waters of the southwest Indian Ocean inferred from stable isotope analysis. PloS One 8, e78229. doi: 10.1371/journal.pone.0078229
Dedman S., Moxley J. H., Papastamatiou Y. P., Braccini M., Caselle J. E., Chapman D. D., et al. (2024). Ecological roles and importance of sharks in the Anthropocene Ocean. Science 385, adl2362. doi: 10.1126/science.adl2362
Driggers W. B., Frazier B. S., Adams D. H., Ulrich G. F., Jones C. M., Hoffmayer E. R., et al. (2014). Site fidelity of migratory bonnethead sharks Sphyrna tiburo (L. 1758) to specific estuaries in South Carolina, USA. J. Exp. Mar. Biol. Ecol. 459, 61–69. doi: 10.1016/j.jembe.2014.05.006
Emerson S. B., Greene H. W., Charnov E. L. (1994). “Allometric aspects of predator-prey interactions,” in Ecological Morphology: Integrative Organismal Biology (University of Chicago Press, Chicago), 123–139.
Erickson G. M., Gignac P. M., Lappin A. K., Vliet K. A., Brueggen J. D., Webb G. J. W. (2014). A comparative analysis of ontogenetic bite-force scaling among Crocodylia. J. Zoology 292, 48–55. doi: 10.1111/jzo.12081
Every S. L., Pethybridge H. R., Fulton C. J., Kyne P. M., Crook D. A. (2017). Niche metrics suggest euryhaline and coastal elasmobranchs provide trophic connections among marine and freshwater biomes in northern Australia. Mar. Ecol. Prog. Ser. 565, 181–196. doi: 10.3354/meps11995
Ferguson A. R., Huber D. R., Lajeunesse M. J., Motta P. J. (2015). Feeding performance of king mackerel, Scomberomorus cavalla. J. Exp. Zoology Part A: Ecol. Genet. Physiol. 323, 399–413. doi: 10.1002/jez.1933
Ferrara T., Clausen P., Huber D., McHenry C., Peddemors V., Wroe S. (2011). Mechanics of biting in great white and sandtiger sharks. J. Biomechanics 44, 430–435. doi: 10.1016/j.jbiomech.2010.09.028
Franco-Moreno R. A., Polly P. D., Toro-Ibacache V., Hernández-Carmona G., Aguilar-Medrano R., Marín-Enríquez E., et al. (2021). Bite force in four pinniped species from the west coast of Baja California, Mexico, in relation to diet, feeding strategy, and niche differentiation. J. Mammal Evol. 28, 307–321. doi: 10.1007/s10914-020-09524-7
Frazzetta T. (1988). The mechanics of cutting and the form of shark teeth (Chondrichthyes, Elasmobranchii). Zoomophology 108, 93–107. doi: 10.1007/BF00539785
Froeschke J., Stunz G. W., Wildhaber M. L. (2010). Environmental influences on the occurrence of coastal sharks in estuarine waters. Mar. Ecol. Prog. Ser. 407, 279–292. doi: 10.3354/meps08546
Gabry J., Simpson D., Vehtari A., Betancourt M., Gelman A. (2019). Visualization in Bayesian workflow. J. R. Stat. Society. Ser. A: Stat Soc. 182, 389–402. doi: 10.1111/rssa.12378
Gallagher A. J., Shiffman D. S., Byrnes E. E., Hammerschlag-Peyer C. M., Hammerschlag N. (2017). Patterns of resource use and isotopic niche overlap among three species of sharks occurring within a protected subtropical estuary. Aquat. Ecol. 51, 435–448. doi: 10.1007/s10452-017-9627-2
Garcia A. M., Hoeinghaus D. J., Vieira J. P., Winemiller K. O. (2007). Isotopic variation of fishes in freshwater and estuarine zones of a large subtropical coastal lagoon. Estuarine Coast. Shelf Sci. 73, 399–408. doi: 10.1016/j.ecss.2007.02.003
Gelman A., Goodrich B., Gabry J., Vehtari A. (2019). R-squared for bayesian regression models. Am. Statistician 73, 307–309. doi: 10.1080/00031305.2018.1549100
Gignac P. M., Erickson G. M. (2016). Ontogenetic bite-force modeling of Alligator mississippiensis: implications for dietary transitions in a large-bodied vertebrate and the evolution of crocodylian feeding. J. Zoology 299, 229–238. doi: 10.1111/jzo.12349
Habegger M. L., Motta P. J., Huber D. R., Dean M. N. (2012). Feeding biomechanics and theoretical calculations of bite force in bull sharks (Carcharhinus leucas) during ontogeny. Zoology 115, 354–364. doi: 10.1016/j.zool.2012.04.007
Habegger M. L., Motta P. J., Huber D. R., Deban S. M. (2011). Feeding biomechanics in the Great Barracuda during ontogeny. J. Zoology 283, 63–72. doi: 10.1111/j.1469-7998.2010.00745.x
Heithaus M. R., Frid A., Vaudo J. J., Worm B., Wirsing A. J. (2010). “Unraveling the ecological importance of elasmobranchs,” in Sharks and Their Relatives II (CRC Press, Boca Raton, FL), 611–638.
Hernandez L. P., Motta P. J. (1997). Trophic consequences of differential performance: ontogeny of oral jaw-crushing performance in the sheepshead, Archosargus probatocephalus (Teleostei, Sparidae). J. Zoology 243, 737–756. doi: 10.1111/j.1469-7998.1997.tb01973.x
Herrel A., Gibb A. C. (2006). Ontogeny of performance in vertebrates. Physiol. Biochem. Zoology 79, 1–6. doi: 10.1086/498196
Herrel A., Van Damme R., Vanhooydonck B., De Vree F. (2001). The implications of bite performance for diet in two species of lacertid lizards. Can. J. Zoology 79, 662–670. doi: 10.1139/z01-031
Herrel A., Van Wassenbergh S., Wouters S., Adriaens D., Aerts P. (2005). A functional morphological approach to the scaling of the feeding system in the African catfish, Clarias gariepinus. J. Exp. Biol. 208, 2091–2102. doi: 10.1242/jeb.01604
Heupel M., Hueter R. (2002). The importance of prey density in relation to the movement patterns of juvenile blacktip sharks (Carcharhinus limbatus) within a coastal nursery area. Mar. Freshw. Res. 53, 543–550. doi: 10.1071/MF01132
Heupel M., Knip D., Simpfendorfer C., Dulvy N. (2014). Sizing up the ecological role of sharks as predators. Mar. Ecol. Prog. Ser. 495, 291–298. doi: 10.3354/meps10597
Heupel M. R., Simpfendorfer C. A., Collins A. B., Tyminski J. P. (2006). Residency and movement patterns of bonnethead sharks, Sphyrna tiburo, in a large Florida estuary. Environ. Biol. Fishes 76, 47–67. doi: 10.1007/s10641-006-9007-6
Heupel M. R., Yeiser B. G., Collins A. B., Ortega L., Simpfendorfer C. A. (2010). Long-term presence and movement patterns of juvenile bull sharks, Carcharhinus leucas, in an estuarine river system. Mar. Freshw. Res. 61, 1–10. doi: 10.1071/MF09019
Hoffman M. D., Gelman A. (2014). The No-U-Turn Sampler: Adaptively setting path lengths in Hamiltonian Monte Carlo. J. Mach. Learn. Res. 15, 1593–1623.
Huber D. R., Claes J. M., Mallefet J., Herrel A. (2009). Is extreme bite performance associated with extreme morphologies in sharks? Physiol. Biochem. Zoology 82, 20–28. doi: 10.1086/588177
Huber D. R., Eason T. G., Hueter R. E., Motta P. J. (2005). Analysis of the bite force and mechanical design of the feeding mechanism of the durophagous horn shark Heterodontus francisci. J. Exp. Biol. 208, 3553–3571. doi: 10.1242/jeb.01816
Huber D. R., Motta P. J. (2004). Comparative analysis of methods for determining bite force in the spiny dogfish Squalus acanthias. J. Exp. Zoology Part A: Comp. Exp. Biol. 301, 26–37. doi: 10.1002/jez.a.20003
Huber D. R., Weggelaar C. L., Motta P. J. (2006). Scaling of bite force in the blacktip shark Carcharhinus limbatus. Zoology 109, 109–119. doi: 10.1016/j.zool.2005.12.002
Hussey N. E., DiBattista J. D., Moore J. W., Ward E. J., Fisk A. T., Kessel S., et al. (2017). Risky business for a juvenile marine predator? Testing the influence of foraging strategies on size and growth rate under natural conditions. Proc. R. Soc. B: Biol. Sci. 284, 20170166. doi: 10.1098/rspb.2017.0166
Hussey N. E., Olin J., Kinney M. J., McMeans B. C., Fisk A. T. (2012). Lipid extraction effects on stable isotope values (δ13C and δ15N) of elasmobranch muscle tissue. J. Exp. Mar. Biol. Ecol. 434–435, 7–15. doi: 10.1016/j.jembe.2012.07.012
Jhaveri P., Papastamatiou Y. P., German D. P. (2015). Digestive enzyme activities in the guts of bonnethead sharks (Sphyrna tiburo) provide insight into their digestive strategy and evidence for microbial digestion in their hindguts. Comp. Biochem. Physiol. Part A: Mol. Integr. Physiol. 189, 76–83. doi: 10.1016/j.cbpa.2015.07.013
Karlson A. M. L., Reutgard M., Garbaras A., Gorokhova E. (2018). Isotopic niche reflects stress-induced variability in physiological status. R. Soc. Open Sci. 5, 171398. doi: 10.1098/rsos.171398
Kilmer J. T., Rodríguez R. L. (2017). Ordinary least squares regression is indicated for studies of allometry. J. Evolutionary Biol. 30, 4–12. doi: 10.1111/jeb.12986
Kim S. L., Koch P. L. (2012). Methods to collect, preserve, and prepare elasmobranch tissues for stable isotope analysis. Environ. Biol. Fishes 95, 53–63. doi: 10.1007/s10641-011-9860-9
Kim S. L., Martinez del Rio C., Casper D., Koch P. L. (2012). Isotopic incorporation rates for shark tissues from a long-term captive feeding study. J. Exp. Biol. 215, 2495–2500. doi: 10.1242/jeb.070656
Kinney M., Hussey N., Fisk A., Tobin A., Simpfendorfer C. (2011). Communal or competitive? Stable isotope analysis provides evidence of resource partitioning within a communal shark nursery. Mar. Ecol. Prog. Ser. 439, 263–276. doi: 10.3354/meps09327
Kohler N. E., Casey J. G., Turner P. A. (1996). Length-Length and Length-Weight Relationships for 13 Shark Species from the Western North Atlantic (Narragansett, RI, USA, NOAA).
Kolmann M. A., Dean Grubbs R., Huber D. R., Fisher R., Lovejoy N. R., Erickson G. M. (2018). Intraspecific variation in feeding mechanics and bite force in durophagous stingrays. J. Zoology 304, 225–234. doi: 10.1111/jzo.12530
Kolmann M. A., Huber D. R. (2009). Scaling of feeding biomechanics in the horn shark Heterodontus francisci: ontogenetic constraints on durophagy. Zoology 112, 351–361. doi: 10.1016/j.zool.2008.11.002
Law C. J., Young C., Mehta R. S. (2016). Ontogenetic scaling of theoretical bite force in southern sea otters (Enhydra lutris nereis). Physiol. Biochem. Zoology 89, 347–363. doi: 10.1086/688313
Leigh S. C., Papastamatiou Y. P., German D. P. (2018). Seagrass digestion by a notorious ‘carnivore.’. Proc. R. Soc. B: Biol. Sci. 285, 20181583. doi: 10.1098/rspb.2018.1583
Livernois M. C., Mohan J. A., TinHan T. C., Richards T. M., Falterman B. J., Miller N. R., et al. (2021). Ontogenetic patterns of elemental tracers in the vertebrae cartilage of coastal and oceanic sharks. Front. Mar. Sci. 8. doi: 10.3389/fmars.2021.704134
Logan J. M., Lutcavage M. E. (2010). Reply to Hussey et al.: The requirement for accurate diet-tissue discrimination factors for interpreting stable isotopes in sharks. Hydrobiologia 654, 7–12. doi: 10.1007/s10750-010-0376-7
Lombardi-Carlson L., Cortés E., Parsons G., Manire C. (2003). Latitudinal variation in life-history traits of bonnethead sharks, Sphyrna tiburo, (Carcharhiniformes: Sphyrnidae) from the eastern Gulf of Mexico. Mar. Freshw. Res. 54, 875–883. doi: 10.1071/MF03023
Lou F., Curtin N. A., Woledge R. C. (2002). Isometric and isovelocity contractile performance of red musle fibres from the dogfish Scyliorhinus canicula. J. Exp. Biol. 205, 1585–1595. doi: 10.1242/jeb.205.11.1585
Lysy M., Stasko A. D., Swanson H. K. (2023). nicheROVER: Niche Region and Niche Overlap Metrics for Multidimensional Ecological Niches. Available online at: https://CRAN.R-project.org/package=nicheROVER (Accessed March 8, 2024).
MacNeil M., Skomal G., Fisk A. (2005). Stable isotopes from multiple tissues reveal diet switching in sharks. Mar. Ecol. Prog. Ser. 302, 199–206. doi: 10.3354/meps302199
Malpica-Cruz L., Herzka S. Z., Sosa-Nishizaki O., Lazo J. P., Trudel M. (2012). Tissue-specific isotope trophic discrimination factors and turnover rates in a marine elasmobranch: empirical and modeling results. Can. J. Fisheries Aquat. Sci. 69, 551–564. doi: 10.1002/art.39222
Mara K. R., Motta P. J., Huber D. R. (2010). Bite force and performance in the durophagous bonnethead shark, Sphyrna tiburo. J. Exp. Zoology Part A: Ecol. Genet. Physiol. 313, 95–105. doi: 10.1002/jez.576
Marshall C. D., Guzman A., Narazaki T., Sato K., Kane E. A., Sterba-Boatwright B. D. (2012). The ontogenetic scaling of bite force and head size in loggerhead sea turtles (Caretta caretta): implications for durophagy in neritic, benthic habitats. J. Exp. Biol. 215, 4166–4174. doi: 10.1242/jeb.074385
Masson R. S., Daoues K., Measey J., Herrel A. (2023). The evolution of bite force and head morphology in scincid lizards: diet and habitat use as possible drivers. Biol. J. Linn. Soc. 140, 58–73. doi: 10.1093/biolinnean/blad052
Matich P., Heithaus M. R., Layman C. A. (2010). Size-based variation in intertissue comparisons of stable carbon and nitrogen isotopic signatures of bull sharks (Carcharhinus leucas) and tiger sharks (Galeocerdo cuvier). Can. J. Fisheries Aquat. Sci. 67, 877–885. doi: 10.1139/F10-037
Matich P., Kiszka J. J., Heithaus M. R., Le Bourg B., Mourier J. (2019). Inter-individual differences in ontogenetic trophic shifts among three marine predators. Oecologia 189, 621–636. doi: 10.1007/s00442-019-04357-5
Matich P., Plumlee J. D., Weideli O. C., Fisher M. (2021a). New insights into the trophic ecology of blacktip sharks (Carcharhinus limbatus) from a subtropical estuary in the western Gulf of Mexico. J. Fish Biol. 98, 470–484. doi: 10.1111/jfb.14592
Matich P., Shipley O. N., Weideli O. C. (2021b). Quantifying spatial variation in isotopic baselines reveals size-based feeding in a model estuarine predator: implications for trophic studies in dynamic ecotones. Mar. Biol. 168, 108. doi: 10.1007/s00227-021-03920-0
McClelland J. W., Valiela I., Michener R. H. (1997). Nitrogen-stable isotope signatures in estuarine food webs: A record of increasing urbanization in coastal watersheds. Limnology Oceanography 42, 930–937. doi: 10.4319/lo.1997.42.5.0930
Motta P. J., Wilga C. A. D. (1995). Anatomy of the feeding apparatus of the lemon shark, Negaprion brevirostris. J. Morphology 226, 309–329. doi: 10.1002/jmor.1052260307
Myrberg A. A., Gruber S. H. (1974). The behavior of the bonnethead shark, Sphyrna tiburo. Copeia 1974, 358–374. doi: 10.2307/1442530
Navia A. F., Mejía-Falla P. A., López-García J., Giraldo A., Cruz-Escalona V. H. (2016). How many trophic roles can elasmobranchs play in a marine tropical network? Mar. Freshw. Res. 68, 1–12. doi: 10.1071/MF16161
Olin J. A., Hussey N. E., Fritts M., Heupel M. R., Simpfendorfer C. A., Poulakis G. R., et al. (2011). Maternal meddling in neonatal sharks: implications for interpreting stable isotopes in young animals. Rapid Commun. Mass Spectrometry 25, 1008–1016. doi: 10.1002/rcm.4946
Peterson B. J., Fry B. (1987). Stable isotopes in ecosystem studies. Annu. Rev. Ecol. Systematics 18, 293–320. doi: 10.1146/annurev.es.18.110187.001453
Peterson C. T., Grubbs R. D., Mickle A. (2020). Trophic ecology of elasmobranch and teleost fishes in a large subtropical seagrass ecosystem (Florida Big Bend) determined by stable isotope analysis. Environ. Biol. Fish 103, 683–701. doi: 10.1007/s10641-020-00976-7
Pethybridge H. R., Choy C. A., Polovina J. J., Fulton E. A. (2018). Improving marine ecosystem models with biochemical tracers. Annu. Rev. Mar. Sci. 10, 199–228. doi: 10.1146/annurev-marine-121916-063256
Pfaller J. B., Gignac P. M., Erickson G. M. (2011). Ontogenetic changes in jaw-muscle architecture facilitate durophagy in the turtle Sternotherus minor. J. Exp. Biol. 214, 1655–1667. doi: 10.1242/jeb.048090
Plumlee J. D., Branham C., Ryburn S. J., Fodrie F. J. (2023). Trophic partitioning among seasonally resident predators in a temperate estuary. Mar. Ecol. Prog. Ser. 721, 119–133. doi: 10.3354/meps14422
Plumlee J. D., Wells R. D. (2016). Feeding ecology of three coastal shark species in the northwest Gulf of Mexico. Mar. Ecol. Prog. Ser. 550, 163–174. doi: 10.3354/meps11723
Post D. M. (2002). Using stable isotopes to estimate trophic position: models, methods and assumptions. Ecology 83, 703–718. doi: 10.1890/0012-9658(2002)083[0703:USITET]2.0.CO;2
Post D. M., Layman C. A., Arrington D. A., Takimoto G., Quattrochi J., Montaña C. G. (2007). Getting to the fat of the matter: models, methods and assumptions for dealing with lipids in stable isotope analyses. Oecologia 152, 179–189. doi: 10.1007/s00442-006-0630-x
Powell P. L., Roy R. R., Kanim P., Bello M. A., Edgerton V. R. (1984). Predictability of skeletal muscle tension from architectural determinations in Guinea pig hindlimbs. J. Appl. Physiol. 57, 1715–1721. doi: 10.1152/jappl.1984.57.6.1715
Raoult V., Phillips A. A., Nelson J., Niella Y., Skinner C., Tilcock M. B., et al. (2024). Why aquatic scientists should use sulfur stable isotope ratios (δ34S) more often. Chemosphere 355, 141816. doi: 10.1016/j.chemosphere.2024.141816
R Core Team (2023). R: A Language and Environment for Statistical Computing. Available online at: https://www.r-project.org/ (Accessed June 5, 2023).
Santana S. E., Miller K. E. (2016). Extreme postnatal scaling in bat feeding performance: a view of ecomorphology from ontogenetic and macroevolutionary perspectives. Integr. Comp. Biol. 56, 459–468. doi: 10.1093/icb/icw075
Shiffman D. S., Gallagher A. J., Boyle M. D., Hammerschlag-Peyer C. M., Hammerschlag N. (2012). Stable isotope analysis as a tool for elasmobranch conservation research: a primer for non-specialists. Mar. Freshw. Res. 63, 635–643. doi: 10.1071/MF11235
Shipley O. N., Olin J. A., Whiteman J. P., Bethea D. M., Newsome S. D. (2022). Bulk and amino acid nitrogen isotopes suggest shifting nitrogen balance of pregnant sharks across gestation. Oecologia 199, 313–328. doi: 10.1007/s00442-022-05197-6
Simpfendorfer C. A., Freitas G. G., Iley T. R., Heupel E. R. (2005). Distribution and Habitat Partitioning of immature Bull Sharks (Carcharhinus leucas) in a Southwest Florida.Estuaries 28, 78–85. doi: 10.1007/BF02732755
Simpson G. L. (2024). gratia: Graceful ggplot-Based Graphics and Other Functions for GAMs Fitted using mgcv. Available online at: https://gavinsimpson.github.io/gratia/ (Accessed February 8, 2024).
Smith R. J. (2009). Use and misuse of the reduced major axis for line-fitting. Am. J. Phys. Anthropology 140, 476–486. doi: 10.1002/ajpa.21090
Snelson F. F., Mulligan T. J., Williams S. E. (1984). Food habits, occurrence, and population structure of the bull shark, Carcharhinus leucas, in Florida coastal lagoons. Bull. Mar. Sci. 34, 71–80.
Snover M. L. (2008). Ontogenetic habitat shifts in marine organisms: Influencing factors and the impact of climate variability. Bull. Mar. Sci. 83, 53–67.
Stanchak K. E., Faure P. A., Santana S. E. (2023). Ontogeny of cranial musculoskeletal anatomy and its relationship to allometric increase in bite force in an insectivorous bat (Eptesicus fuscus). Anatomical Rec. 306, 2842–2852. doi: 10.1002/ar.25213
Stan Development Team (2023). RStan: the R interface to Stan. Available online at: https://mc-stan.org/ (Accessed June 2, 2023).
Swanson H. K., Lysy M., Power M., Stasko A. D., Johnson J. D., Reist J. D. (2015). A new probabilistic method for quantifying n-dimensional ecological niches and niche overlap. Ecology 96, 318–324. doi: 10.1890/14-0235.1
Timm L. L. (2013). Feeding biomechanics and craniodental morphology in otters (Lutrinae) (College Station, TX, USA: Texas A&M University).
TinHan T. C., Wells R. J. D. (2021). Spatial and ontogenetic patterns in the trophic ecology of juvenile bull sharks (Carcharhinus leucas) from the Northwest gulf of Mexico. Front. Mar. Sci. 8. doi: 10.3389/fmars.2021.664316
van der Meij M. A. A., Bout R. G. (2004). Scaling of jaw muscle size and maximal bite force in finches. J. Exp. Biol. 207, 2745–2753. doi: 10.1242/jeb.01091
van der Meij M. A. A., Bout R. G. (2006). Seed husking time and maximal bite force in finches. J. Exp. Biol. 209, 3329–3335. doi: 10.1242/jeb.02379
Vander Zanden M. J., Vadeboncoeur Y., Diebel M. W., Jeppesen E. (2005). Primary consumer stable nitrogen isotopes as indicators of nutrient source. Environ. Sci. Technol. 39, 7509–7515. doi: 10.1021/es050606t
Vehtari A., Gelman A., Simpson D., Carpenter B., Burkner P.-C. (2021). Rank-normalization, folding, and localization: An improved Ȓ for assessing convergence of MCMC (with Discussion). Bayesian Anal. 16, 667–718. doi: 10.1214/20-BA1221
Verwaijen D., Van Damme R., Herrel A. (2002). Relationships between head size, bite force, prey handling efficiency and diet in two sympatric lacertid lizards. Funct. Ecol. 16, 842–850. doi: 10.1046/j.1365-2435.2002.00696.x
Wainwright P. C. (1988). Morphology and ecology: functional basis of feeding constraints in Caribbean labrid fishes. Ecology 69, 635–645. doi: 10.2307/1941012
Weber S., Cullen J. A., Fuentes M. M. P. B. (2023). Isotopic niche overlap among foraging marine turtle species in the Gulf of Mexico. Ecol. Evol. 13, e10741. doi: 10.1002/ece3.10741
Werner E. E., Gilliam J. F. (1984). The ontogenetic niche and species interactions in size-structured populations. Annu. Rev. Ecol. Systematics 15, 393–425. doi: 10.1146/annurev.es.15.110184.002141
Werry J. M., Lee S. Y., Lemckert C. J., Otway N. M. (2012). Natural or artificial Habitat-use by the bull shark, carcharhinus leucas. PloS One 7, e49796. doi: 10.1371/journal.pone.0049796
Werry J., Lee S., Otway N., Hu Y., Sumpton W. (2011). A multi-faceted approach for quantifying the estuarine-nearshore transition in the life cycle of the bull shark, Carcharhinus leucas. Mar. Freshw. Res. 62, 1421–1431. doi: 10.1071/MF11136
Westneat M. W. (2003). A biomechanical model for analysis of muscle force, power output and lower jaw motion in fishes. J. Theor. Biol. 223, 269–281. doi: 10.1016/S0022-5193(03)00058-4
Whiteman J. P., Kim S. L., McMahon K. W., Koch P. L., Newsome S. D. (2018). Amino acid isotope discrimination factors for a carnivore: physiological insights from leopard sharks and their diet. Oecologia 188, 977–989. doi: 10.1007/s00442-018-4276-2
Whitenack L., Motta P. (2010). Performance of shark teeth during puncture and draw: implications for the mechanics of cutting. Biol. J. Linn. Soc. 100, 271–286. doi: 10.1111/j.1095-8312.2010.01421.x
Wilga C., Motta P. (2000). Durophagy in sharks: feeding mechanics of the hammerhead Sphyrna tiburo. J. Exp. Biol. 203, 2781–2796. doi: 10.1242/jeb.203.18.2781
Wood S. N. (2011). Fast stable restricted maximum likelihood and marginal likelihood estimation of semiparametric generalized linear models. J. R. Stat. Society. Ser. B: Stat. Method. 73, 3–36. doi: 10.1111/j.1467-9868.2010.00749.x
Keywords: allometric scaling, bite force, feeding biomechanics, sharks, stable isotopes, trophic ecology
Citation: Cullen JA and Marshall CD (2024) Scaling of bite force corresponds with ontogenetic niche shifts in coastal elasmobranchs. Front. Mar. Sci. 11:1452984. doi: 10.3389/fmars.2024.1452984
Received: 21 June 2024; Accepted: 08 November 2024;
Published: 28 November 2024.
Edited by:
David M. P. Jacoby, Lancaster University, United KingdomReviewed by:
Peter Gausmann, Ruhr University Bochum, GermanyAaron Carlisle, University of Delaware, United States
Copyright © 2024 Cullen and Marshall. This is an open-access article distributed under the terms of the Creative Commons Attribution License (CC BY). The use, distribution or reproduction in other forums is permitted, provided the original author(s) and the copyright owner(s) are credited and that the original publication in this journal is cited, in accordance with accepted academic practice. No use, distribution or reproduction is permitted which does not comply with these terms.
*Correspondence: Joshua A. Cullen, am9zaGN1bGxlbjEwQGdtYWlsLmNvbQ==
†Present address: Joshua A. Cullen, Institute of Marine Sciences, University of California Santa Cruz, Santa Cruz, CA, USA