- 1Laboratorio de Embriología Comparada, Programa de Biología Integrativa, Instituto de Ciencias Biomédicas (ICBM), Facultad de Medicina, Universidad de Chile, Santiago, Chile
- 2Programa de Doctorado en Ciencias Morfológicas, Facultad de Medicina, Universidad de La Frontera, Temuco, Chile
- 3Departamento de Especialidades Médicas, Facultad de Medicina, Universidad de La Frontera, Temuco, Chile
- 4Escuela de Tecnología Médica, Facultad de Salud, Universidad Santo Tomás, Osorno, Chile
- 5Departamento de Medicina Interna, Facultad de Medicina, Universidad de La Frontera, Temuco, Chile
- 6Núcleo Científico y Tecnológico en Biorecursos (BIOREN), Universidad de La Frontera, Temuco, Chile
- 7Physiology Development and Neuroscience Department, University of Cambridge, Cambridge, United Kingdom
- 8Escuela de Medicina Veterinaria, Facultad de Recursos Naturales y Medicina Veterinaria, Universidad Santo Tomás, Talca, Chile
Introduction: Hypoxia has a teratogenic effect on the fish during embryonic development. Nevertheless, the effects on the larval stage are not yet known. Therefore, the aim of this study was to assess the effects of hypoxia on the number of neurons and their apoptotic rate in the spinal cord of Salmo salar alevins after hatching.
Methods: We used a total of 400 alevins, establishing both hypoxia and control (normoxia) groups (n = 8), considering post-hatching days 1, 3, 5, and 7, each with 50 individuals. Transversal sections of 50 μm thickness were cut from the alevin body. We performed cresyl-violet staining and counted the spinal cord neurons. Also, immunohistochemistry for HIF-1α and caspase-3 were used. For statistical analysis ANOVA one-way and Tukey's Test were used.
Results: HIF-1α was expressed in spinal neurons in both the hypoxic and normoxic groups, with the former being significantly higher. Both the hypoxic and normoxic groups evidenced the process of neuronal apoptosis, with the hypoxic groups demonstrating a higher significance. The number of neurons in the spinal cord was significantly lower in the hypoxic group.
Discussion: We found that when oxygen levels in the aquatic environment were low in Salmo salar farming alevins post-hatch, the number of spinal neurons dropped by half. These results contribute to increasing our knowledge of the biological development of salmon, in particular the genesis of the spinal cord, and the effects of hypoxic conditions on the development of this structure of the nervous system.
1 Introduction
From its early egg stage to the juvenile form, the Atlantic salmon Salmo salar develops in a freshwater environment. Subsequently, the juveniles move to the ocean, where the processes of growth and maturation continue. This cycle occurs both in nature and in fish farms. Salmonids live in cold waters and are very sensitive to oxygen deficiencies (Krasnov et al., 2021). They are also affected by stressors such as climate change, with its effect on rising environmental temperatures. In other species, such as zebrafish, it has been observed that very high temperatures, of the order of 34 degrees Celsius, affect exploratory behavior, the level of anxiety, and social preference of individuals (Angiulli et al., 2020), as well as the expression of proteins in the central nervous system (Toni et al., 2019). Regarding the effects of temperature, it has been reported that its increases are strongly related to hypoxia (Roman et al., 2019).
Hypoxia is defined as a critically low-oxygen condition in water (Cerqueira et al., 2024). Conditions of low oxygen concentration in water often occur in nature, but the risk of hypoxia is especially high in fish farming, where recirculating water systems (RAS) are used, as well as in sea cages with large stocking densities (Krasnov et al., 2021; Canosa and Bertucci, 2023). Studies indicate that hypoxia conditions can adversely impact development, growth, reproduction, and survival (Krasnov et al., 2021; Canosa and Bertucci, 2023). Furthermore, prolonged exposure to hypoxia causes low feed intake, growth retardation, and a high tendency to disease (Kestemont and Baras, 2001).
The Hypoxia Inducible Factor (HIF) plays an important role in vertebrate gene expression when oxygen levels in the medium are low. It also participates in some developmental processes, like the growth of the heart and brain as well as the formation of blood vessels (Rojas et al., 2007). Regarding the harmful effects caused by hypoxia, these are particularly relevant in the salmon aquaculture industry. Hypoxic environments primarily cause deformations of the spinal cord, a reduction in the individual’s body length, and in more severe cases, a C-shaped curvature of the body. Deformations of the caudal fin and defects in the ocular bulb and periocular cartilage have also been described (Castro et al., 2011; Rojas et al., 2024; Pellón et al., 2016; Shang and Wu, 2004). Nevertheless, so far, very few studies have focused on the effects of hypoxia in the juvenile period of this species (Castro et al., 2011).
Fish contain a variety of hypoxia adaptation-related proteins. Zhu et al. (2013) classified these proteins into four types based on the cellular processes they participate in: i) oxygen sensing, ii) oxygen transport, iii) erythropoiesis, and iv) angiogenesis. Several oxygen-sensing proteins have been reported to regulate hypoxia adaptation in fish. Any change in their expression can cause different biological processes to occur, such as changes in cell volume and cytoskeletal organization, membrane stretching or compaction, molecular chaperones that monitor how much protein unfolding occurs, and DNA damage sensors (Mandic et al., 2009; Richards, 2011; Zhu et al., 2013). Fish can use the hypoxia adaptation-related proteins to detect oxygen concentrations, trigger adaptive responses, or trigger programmed cell death when their tolerance limit exceeds (Mandic et al., 2009; Richards, 2011). The transcriptional complex HIF is an oxygen-sensing protein highly conserved among vertebrates (Zhu et al., 2013).
It is well known that hypoxic conditions remarkably increase apoptosis. Unlike necrosis, this type of cell death follows a genetic programme characterized by the activation of a group of specific proteases (caspases) that confer distinctive cell morphology (AnvariFar et al., 2017). These morphological changes are presented in two stages: 1) nuclear and cytoplasmic condensation, and 2) cell disruption into the so-called apoptotic bodies. Its manifestation allows to recognize the apoptotic process. As noted previously, caspase activation is an essential condition to trigger cell death by apoptosis. During the process of programmed cell death, caspases constitutively expressed as inactive proenzymes, activated in cascade (Chang and Yang, 2000).
Mostly, HIF controls oxygen homeostasis (Rojas et al., 2007; Semenza, 2008). HIF is a heterodimeric transcription factor made up of one α- and one β-subunit (Heise et al., 2007). There are three known isoforms of the α-subunit (1α, 2α, and 3α), all of which are unstable under normal oxygen concentrations in the medium (normoxia). The β-subunit is the constitutive known variant (1β) (Nikinmaa and Rees, 2005; Rahman and Thomas, 2007).
The isoform HIF-1α is involved in the process of apoptosis induced by hypoxia, and it is present in both the cytoplasm and the nuclear cell compartments. It has been demonstrated to regulate the expression of at least 70 different genes (Greijer and van der Wall, 2004; Acker and Acker, 2004). In normoxia conditions, HIF-1α is degraded by hydroxylation and subsequently destroyed, whereas under hypoxia, HIF-1α binds to HIF-1β, leading to HIF-1 and thus triggering the stimulation and activation of different genes induced by hypoxia (Shingo et al., 2006).
There are cells that present high levels of HIF-1α but are not necessarily killed by hypoxia, fact that suggests that HIF-1α could also act as an anti-apoptotic factor (Greijer and van der Wall, 2004). It has been suggested that when cells are exposed to chronic hypoxia conditions, an over-expression of HIF-1α would cause an increase in anti-apoptotic factors like erythropoietin (EPO) (Bianciardi et al., 2006). However, the exact mechanisms behind this process are still not fully understood.
It has also been documented that the degree of severity of hypoxia determines if HIF-1α acts as a pro-apoptotic or anti-apoptotic agent. Under mild hypoxia conditions, the amount of the protein p53 is low, HIF-1α is phosphorylated and consequently forms dimers with HIF-1β. This causes the activation of anti-apoptotic genes like EPO (Halterman et al., 1999; Suzuki et al., 2001). On the other hand, when chronic hypoxia conditions are severe, HIF-1α is dephosphorylated, which causes increase in cellular levels of p53. This leads to the formation of a new transcription complex involving the activation of genes such as Bax (Halterman et al., 1999; Piret et al., 2002; Calvert et al., 2006).
In zebrafish, in particular, hypoxic conditions clearly delay the embryonic development, affecting its rate and altering its normal timing (for example, the tail grows faster than the head). This result in several malformations due to an increase in apoptosis. In wild populations, these alterations occur in the early stages of the life cycle, subsequently reducing the fitness of individuals and their chances of survival. Spinal deformity also results in external abnormalities, manifesting as alterations in the axial curvature (Shang and Wu, 2004; Castro et al., 2011).
On the other hand, it is well known that the relationship between the concentration of oxygen in the aquatic environment and the rate of fish mortality shows a dose-dependent response, where lower concentrations of oxygen produce higher rates of mortality (Shang and Wu, 2004; Chan et al., 2007). Researchers have also reported a loss of cardiac gating in zebrafish embryos exposed to hypoxia (Shang and Wu, 2004; Chan et al., 2007).
In a previous study, we have described the development of the spinal cord of salmon (Salmo salar) during the post-hatching period under normoxia conditions (Hernández and Rojas, 2013) and more recently we have reported an association between exposure to hypoxia and the deformation of the body and fin, as well as a correlation between hypoxia and the total length of the fry and fin (Rojas et al., 2024). Hypoxia affects not just the events of the fry development, as its effects can be evidenced throughout the entire ontogeny of the fish.
The hypothesis underlying the present work suggests that the salmon alevin spinal cord exposed to hypoxia conditions during the first seven days after hatching, exhibits a decrease in the neuronal number concomitantly with an increased rate of apoptosis. Our study aims to: i) Asses the shape of the salmon alevin spinal cord; ii) Evaluate the number of normal and apoptotic neurons in the alevin spinal cord under normal and low oxygen levels; iii) To count the number of HIF-1α-positive neurons in the alevin spinal cord when oxygen levels in the aquatic environment are normal and when they are low; and iv) To count the number of spinal neurons that are caspase-3-positive and to correlate it with the alevin behavior and the development of the nervous system during the first week post-hatching.
2 Material and methods
We cultured four hundred Salmo salar fertilized eggs at the Aquaculture Research Center of the University of Chile, located in Castro, Chiloe (latitude 42°27’48” S, longitude 73°48’29” W). Fresh river water in continuous flow at a temperature of 8–9°C and 90–100% oxygen saturation was used as culture medium.
The water condition was continually monitored in real time using an oximeter (Oxiguard®) expressing oxygen levels both as a percentage and in parts per million. The oximeter also indicates the temperature and pH of the system, allowing for proper control of the water acidity or alkalinity. If a decrease in the oxygen level is detected, the system automatically agitates the water’s surface to increase oxygen transfer at the air-water interface. Also, in order to regulate the temperature of the aquatic environment. we use water chillers (Chiller HYH-0.5D, Lando©, Longgang District, Shenzhen, China). In addition, we use filtration and monitoring systems to control the presence of contaminants, ensuring optimal water quality. Fry nutritional needs are satisfied by the content of their yolk sacs, hence, there is no need to supplementary feeding.
The ages of 1-, 3-, 5-, and 7 days post-hatching were selected because they correspond to a stage in which the nervous system is just beginning to differentiate (Hernández and Rojas, 2013). During the developmental period covered in the present study, the gray matter does not yet acquire the inverted Y shape, typically displayed in the spinal cord of the juvenile (smolt) and adult salmon. However, in the juvenile and mature salmon specimens, the spinal neurons in the gray matter are cytoarchitectonically organized, adopting the form of an inverted “Y” around the central canal of the spinal cord (González and Martín, 1998).
At hatching time, which is equal to 495 accumulated thermal units (ATU) the alevins were divided into two groups of 200 individual each and were further cultured in two different environments: Group 1) a control group in normoxia (100–90% oxygen saturation), and Group 2) a study group in hypoxic conditions (60% oxygen saturation). The 60% oxygen saturation level of the water in the culture tank is achieved by regulating the flow of water entering the tank using a stopcock that controls the speed of the water flow until the oximeter (Oxiguard®) registers a 60% value. Subsequently, the adequate opening of the stopcock is maintained, and the saturation level is monitored continuously using the indicated oximeter. We anesthetized and euthanized 50 alevins of each group at days 1, 3, 5, and 7 post-hatching (corresponding to 504, 522, 540, and 558 ATU, respectively) by exposing them to 5% benzocaine (BZ-20®, Veterquimica, Chile). Then, we fixed the alevins in 10% buffered formalin, embedded them in paraffin, cut them in transversal sections 5 μm thickness, on a microtome (Microm HM315R, Thermo Fisher Scientific, Waltham, MA, USA), and mounted the histological sections on glass slides.
A total of 40 coronal histological sections were collected from each individual at the level of the dorsal fin and caudal to the yolk sac (Figure 1). This comprises approximately one-third of the spinal cord of the alevin. Then, we used cresyl violet to stain these tissue sections following a standard routine protocol. Immunohistochemical procedure was then performed to assess HIF-1 factor and caspase-3 expression on the spinal cord neurons.
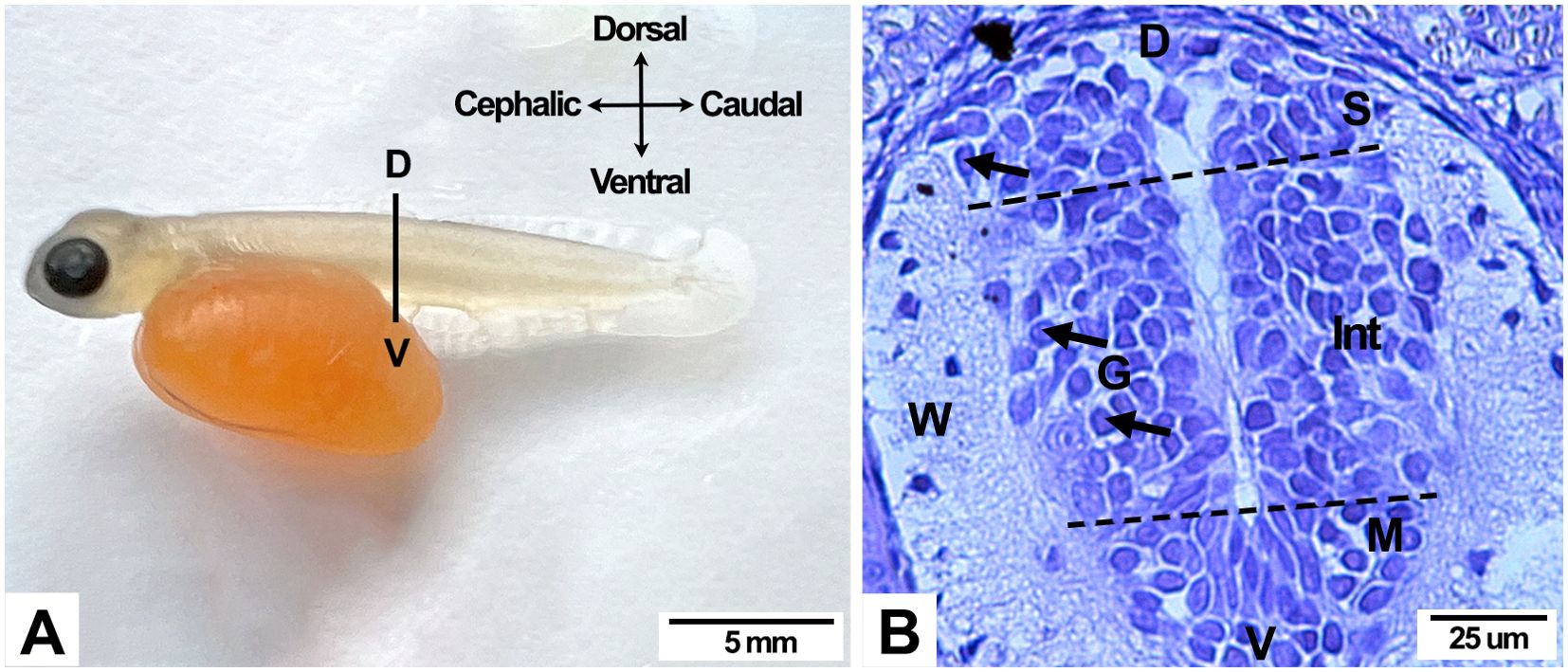
Figure 1. Anatomical and histological cross sections from a 504 ATU Salmo salar alevin. (A) The gross anatomical level of the body section, caudal to the yolk sac (continuous line). (B) Histological section of the spinal cord at the anatomical level represented in (A). The mantle zone (G) contains neuronal bodies and neuroglia, which correspond to gray matter. Some neurons display the initial growth cone of the axon (arrows). Motoneurons in early stages of differentiation are located in the ventral (V) region of the mantle zone, while sensorial neurons occupy the dorsal (D) region. Interneurons (In) develop between both regions. Axons primarily constitute the white matter (W). D, Dorsal; M, Motor neuron zone; S, Sensory neuron zone; V, Ventral. Staining, Cresyl violet.
Transverse histological sections (5 µm thickness) were taken from the salmon alevins, mounted on a positive charged slide, and subsequently treated using the immunohistochemical method for detecting HIF-1α and with the anti-caspase-3 antibody (E-8) which is recommended for detection of caspase-3.
Briefly, the immunohistochemical procedure was as follows: The histological sections were treated with 3% hydrogen peroxide in methanol (v/v) for 15 minutes to block the activity of endogenous peroxidase. Next, the unspecific background was blocked using 3% PBS+BSA for 30 minutes. Then, antigenic recovery was performed with an antigen unmasking solution (Vector Laboratories, Newark, CA, USA). After that, the primary antibody Rabbit polyclonal HIF-1α (Cat. #H-206, Santa Cruz Biotechnology, Santa Cruz, CA, USA) was added to phosphate buffered saline (PBS) that had been diluted 1:100. The anti-HIF1α antibody is a rabbit polyclonal antibody that was raised against human HIF-1α amino acids 557–780 (Cat. #H-206, Santa Cruz Biotechnology, Santa Cruz, CA, USA) and gave positive reactions in cell nuclei and cytoplasm. The primary antibody was detected by incubation with anti-rabbit IgG Horseradish Peroxidase-conjugated Antibody (HRP) for 15 minutes. Finally, diaminobenzidine (Vector Laboratories, Newark, CA, USA) was used to reveal the enzymatic reaction.
For the study of apoptosis, the samples were permeabilized by incubation in 0.1% triton X-100 diluted in PBS for 5 minutes. After washing with PBS, 200 uL of blocking buffer (PBS 0.1% Tween 20 + 5% horse serum) were added. The samples were incubated for 1-2 hours at room temperature in a humidified chamber. After that, 100 mL of a 1:200 dilution of the anti-caspase-3 antibody (Cat. #E-8, Santa Cruz Biotechnology, Santa Cruz, CA, USA) in blocking buffer was added. The anti-caspase-3 monoclonal antibody (Cat. #E-8, Santa Cruz Biotechnology, Santa Cruz, CA, USA) is used to detect the caspase-3 protein of human origin. The antibody targets amino acids 1-277, which embody the full form of the caspase-3 precursor, and gave positive reactions in cytoplasm. This incubation step was then left to sit overnight in a humidified chamber at 4°C. The next day, the samples were washed in 0.1% Tween in PBS for 20 minutes at room temperature, and then the chromogen was added to finally perform the Hematoxylin-Eosin counterstaining procedure.
For the two antibodies used in the study, the negative controls were obtained by performing the complete immunohistochemical technique but omitting the primary antibodies. As an immunohistochemistry-positive control, skin from Salmo salar alevins that had been exposed to 40% hypoxia was used. As previously described, 40% hypoxia conditions were obtained by controlling the water flow rate in the tank and continuous monitoring of the oxygen concentration. These samples were obtained from previous studies (Hernández, 2011). These controls show the specificity of the primary antibodies.
Neuronal quantification was performed on digital images using the dissector method, which consists of quantifying the number of neurons contained in optical dissectors or quadrants (West et al., 1991).
The data was grouped according to normoxia or hypoxia groups for the statistical analysis at 1, 3, 5, or 7 post-hatching days. The Shapiro-Wilk test analyzed the data for normal distribution, and a one-way ANOVA with the Tukey test determined the statistical differences between groups. The statistical analysis was performed using the GraphPad Prism 5.0 statistical program (GraphPad Software, San Diego, CA, USA). A p-value of less than 0.05 was considered significant. All experimental procedures were done according to the Bioethical Committee of the Universidad de La Frontera (N° 145 061_20).
3 Results
3.1 Spinal cord morphology of cultured alevins under normoxia and hypoxia conditions
A 1-day post-hatching alevin cultivated under normoxia conditions is depicted in Figure 1A. A cross-body section, caudal to the yolk sack and extending from the dorsal to the pelvic fins, allows us to show the alevin spinal cord (Figure 1B), where the gray and white matter can be observed (Figure 1B). In the gray matter, the ventricular (ependymal), mantle, and marginal zones can be discerned, but it is difficult to discriminate the ventricular from the mantle zones. Neurons and neurons are located in the mantle area. Ventrally located motoneurons can be recognized from day 3 in normoxia and day 1 in hipoxia (Figure 2). Interneurons located in the intermedia zone and the dorsally located sensorial neurons are recognizable from day 3 in both experimental conditions (Figure 2). Neuronal cell bodies can be distinguished from the glial cells due to their rounded nucleus and soma. Occasionally, the initial axonal growth cone can be seen in some of the spinal neurons (Figure 1B).
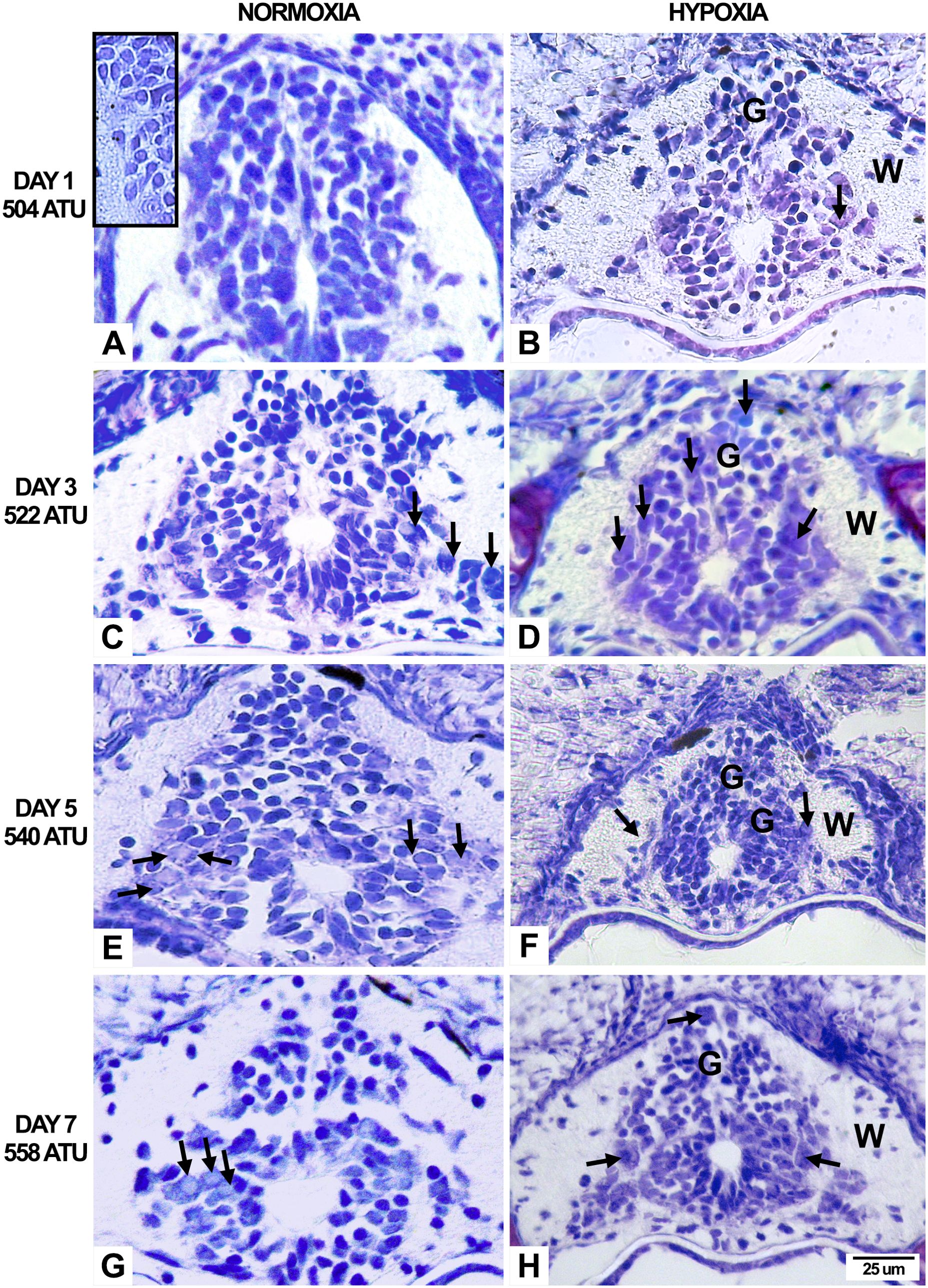
Figure 2. Histological sections of Salmo salar alevin spinal cord under normoxia and hypoxia conditions. Transversal sections of the spinal cord under normoxia (A, C, E, G) and hypoxia (B, D, F, H) conditions at day 1 at 504 ATU (A, B), day 3 at 522 ATU (C, D), day 5 at 540 ATU (E, F), and day 7 at 558 ATU (G, H) posthatching. Under both conditions, there is a noticeable increase in the number of neurons as development progresses. However, comparing spinal cord sections under hypoxia and normoxia reveals a decrease in the population of neurons under hypoxia. Motoneurons, cell bodies, and interneurons are visible on day 3 under normoxia and on day 1 under hypoxia (arrows). G, Gray matter; W, White matter.
Along the entire period studied (from 504 to 558 ATU), the alevin spinal cord has been in the process of formation. The majority of the cells in the gray matter (Figures 1B, 2) correspond to a neuroepithelium formed by neuroblasts, which can differentiate as neurons or glia. Neuronal cells can be recognized as they characteristically present rounded somas and nuclei with a scant and very thin cytoplasm. Some of them evidence cytoplasmic extensions (bipolar neuroblast) and, in a few cases, an incipient axonal growth cone can be observed (Figures 1, 2). Other cells in the gray matter correspond to glioblasts, but they develop later. The number of neurons increases because during the developmental period analyzed they are continually differentiating from the neuroblast stage and then increase in size once they reach the final place in the spinal cord cytoarchitecture.
Figure shows that as the ages of the alevins increase under both experimental conditions (normoxia and hypoxia), a concomitant increase in the total number of spinal cord neurons differentiating from the neuroblast stage is evidenced. Furthermore, when comparing normoxia vs. hypoxia spinal cords for each age, a smaller number of neurons can be seen in the hypoxia condition compared to normoxia (Figure 2). Motoneurons and interneurons (arrows) can be evidenced for the first time at the third day post hatching in normoxia and from the first day in hypoxia, but other motoneurons initiate the differentiation process between 5 and 7 days.
3.2 Quantification of spinal cord neurons in cultured alevins under normoxia and hypoxia conditions
Neuronal counts show a consistent and gradual increase in the number of spinal neurons in both groups, which aligns with the corresponding stage of development. However, in all studied groups, significant differences in the number of spinal neurons were found when the hypoxic and normoxia were compared (p ≤ 0.05). Significant reductions in the number of neurons were detected in all groups cultured under hypoxia conditions (Figure 3).
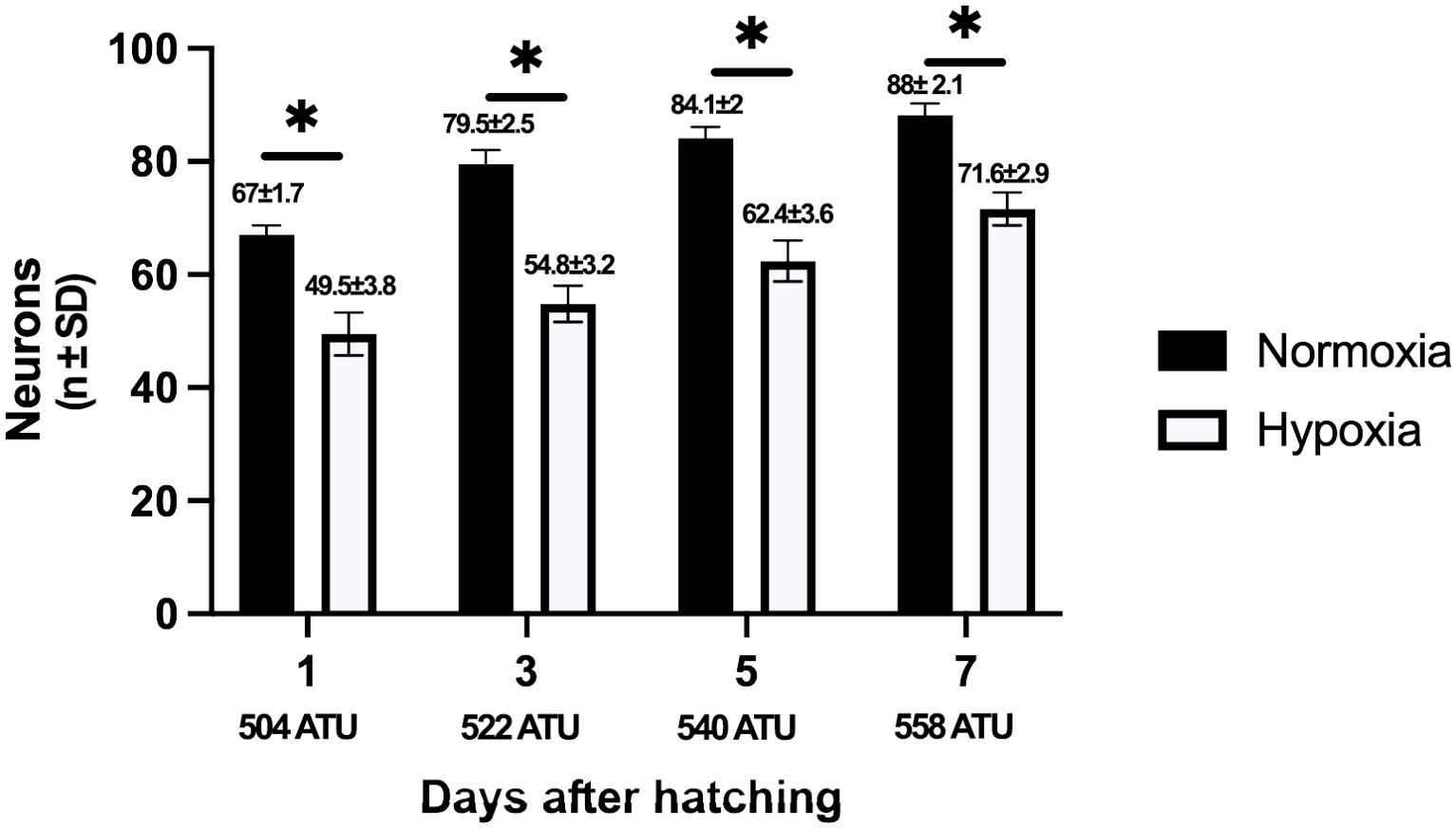
Figure 3. Quantification of the number of spinal neurons in post-hatching alevin spinal cord cultured in hypoxic and normoxic conditions. Following euthanasia, each fish yielded a total of 40 histological coronal sections, each with a thickness of 5 µm. Sections 1, 10, and 20 of each sample were selected and stained with Cresyl violet for neuronal quantification. Neurons were recognized by their larger and round soma, the presence of a nucleus, and their distribution in the gray matter. As expected, the number of spinal neurons increases in both groups as development progresses; however, when the number of neurons between normoxia and hypoxia conditions was compared, a clear and statistically significant decrease was observed at all the studied ages under the hypoxia condition. The mean and standard deviation in neurons are indicated above each bar. Asterisks indicate statistically significant differences (p<0.05). A one-way ANOVA was used.
3.3 Quantification of HIF-1-positive neurons in the spinal cord of alevins under normoxia and hypoxia conditions
In terms of HIF-1α and caspase-3 expression, we saw positive labeling in both normoxia and hypoxia. This labeling increased from three days to five days in both cases, but at seven days, the caspase-3 mark showed much more positive labeling than the HIF-1α mark (Figure 4).
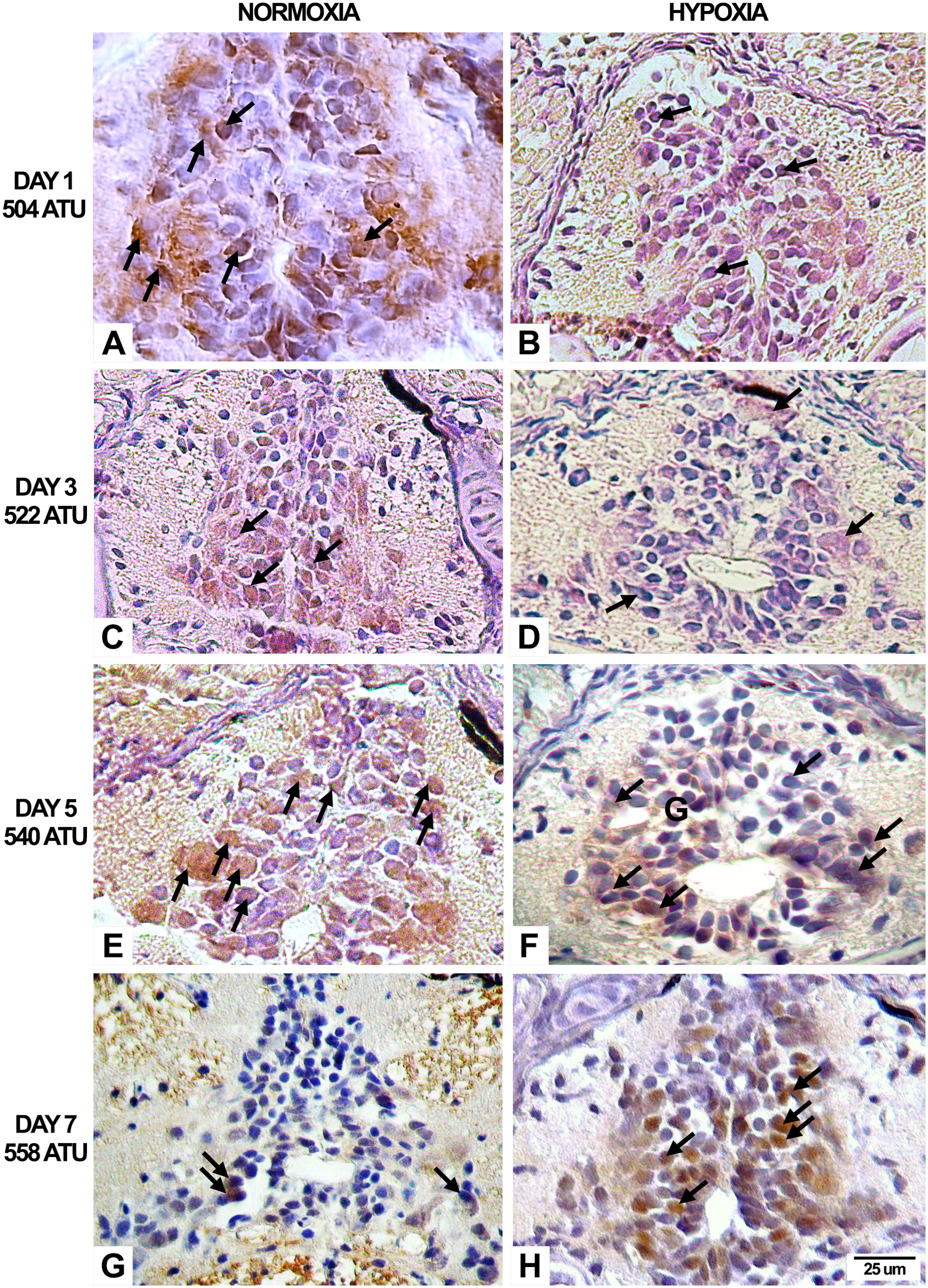
Figure 4. HIF-1α immunohistochemistry expression in the alevin spinal cord under normoxia and hypoxia conditions. The left column shows HIF-1 immunoexpression under normoxia condition, where (A, C, E, G) correspond to 1 (504 ATU), 3 (522 ATU), 5 (540 ATU), and 7 (558 ATU) days, respectively. In the right column, (B, D, F, H) illustrate the results for the same ages under hypoxia condition. The percentage of positive neurons during the first day post-hatching is similar in normoxia and hypoxia (3 to 4%). As development progresses, these numbers consistently increase to 6% and 29% at 3 days, to 10% and 38% at 5 days, and 11% and 47% at 7 days, for normoxia and hypoxia conditions, respectively. Under both experimental conditions, the ventricular (germinal) cells, motoneurons, interneurons, and sensory neurons expressed the immunopositive reaction in both the nuclear and cytoplasmic compartments. Arrows indicate positive labeling. Counterstaining, Hematoxylin.
All of the control groups showed a positive reaction for HIF-1 in the nucleus and cytoplasm of the spinal neurons (Figure 5). All groups exposed to hypoxia conditions showed the same positive reaction to HIF-1 (Figure 5). This response increases as the post-hatching development progresses, with its maximum expression observed at day 7 post-hatching.
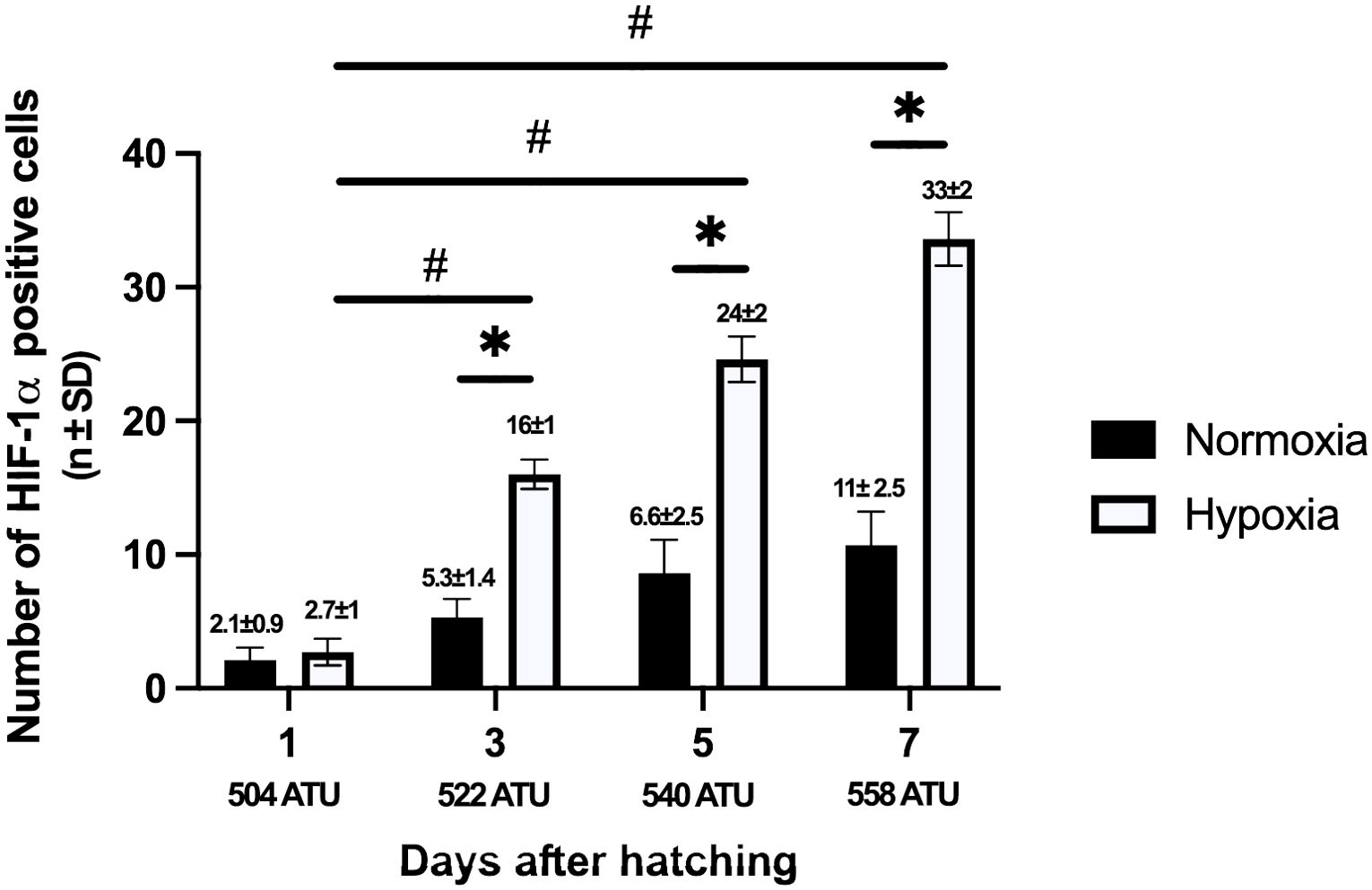
Figure 5. Numbers of HIF-1α positive spinal neurons in alevins cultured under normoxic and hypoxic conditions on days 1, 3, 5, and 7. For each alevin, 10 histological sections were selected and subjected to the HIF-1α immunohistochemistry procedure. Under both experimental conditions, the number of positively labeled cells increases as development progresses. The hypoxic group observed a greater increase in labeled cells from day 3 onwards compared to the normoxic group, with statistically significant differences between days 3, 5, and 7. The mean and standard deviation in the numbers of labeled neurons are indicated above each bar. Asterisks indicate statistically significant differences (p<0.05) between normoxic and hypoxic conditions, and the # indicates statistically significant differences from days 1 to 3, 5, and 7 (p<0.05). A one-way ANOVA was used.
3.4 Quantification of caspase-3-positive neurons in the spinal cord of alevins cultured under normoxia and hypoxia conditions
Immunohistochemistry for Caspase-3 evidenced neuronal cell death both in the hypoxic and normoxic groups (Figures 6, 7). Caspase-3-positive neurons increased along the development, but they were more abundant under the hypoxic condition, and the difference was statistically significant (p<0.05). The percentage for each of the studied ages is as follows: Under normoxic conditions, at the 1st post-hatching day, the percentage of apoptotic neurons reached 3%, while in hypoxia it was 6%. At the third post-hatching day, those percentages increased to 5% in normoxia and 9% in hypoxia. At the 5th day, 6% of apoptotic cells were observed under normoxia and 11% in hypoxia. Finally, at the seventh day, 7% were observed in the normoxic and 12% in the hypoxic groups.
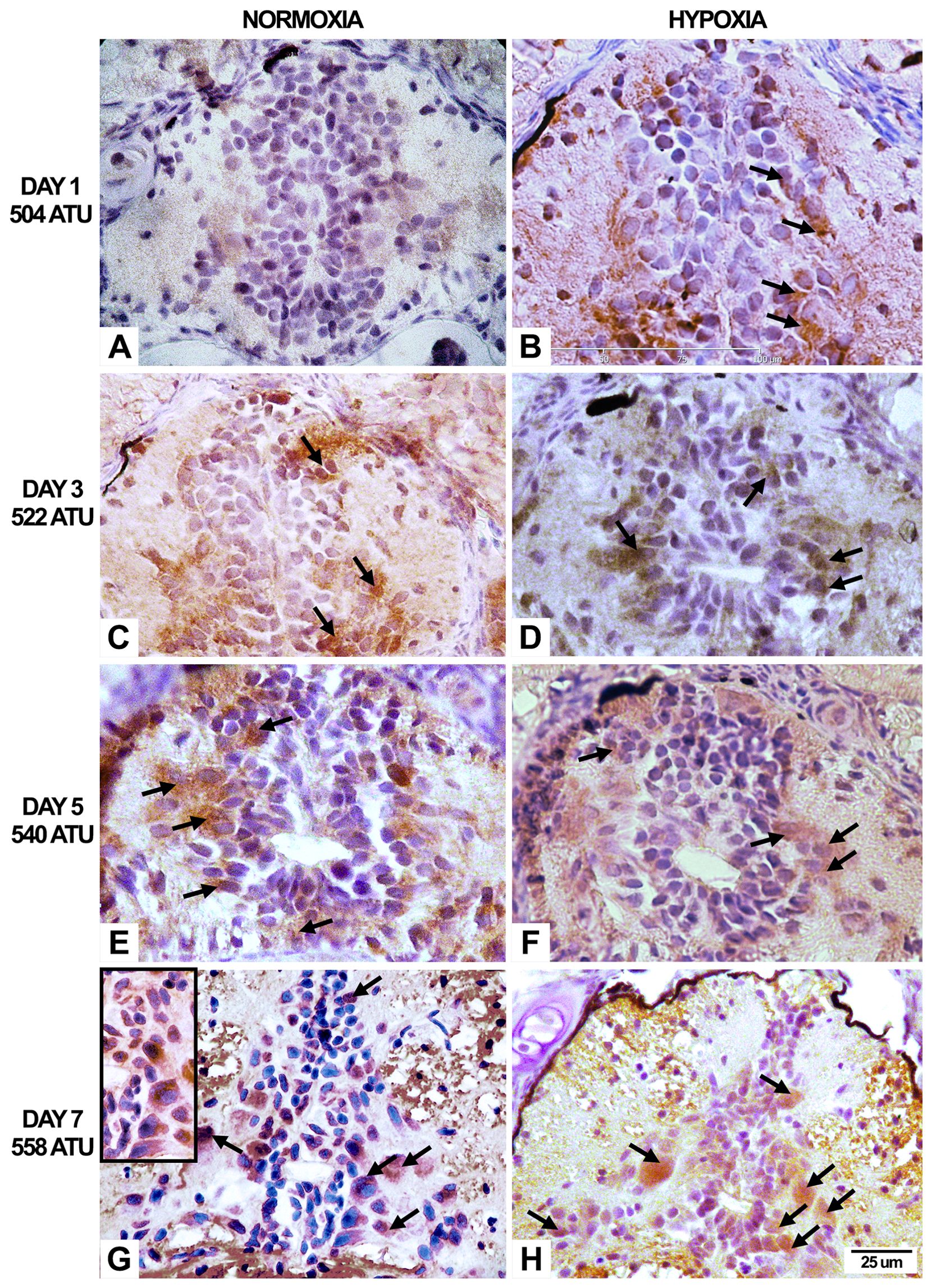
Figure 6. Immunohistochemical expression of Caspase-3 in spinal neurons in alevins cultured under normoxic and hypoxic conditions. The positive immune reaction is expressed at the level of the cytoplasmic cell compartment. Under normoxic conditions (A, C, E, G), the anti-caspase antibody clearly labels motoneurons cells from 3 to 7 days (arrows). On the other hand, in the hypoxic spinal cords (B, D, F, H), labeled cells, which correspond to motor and sensory neurons as well as interneurons, were observed from the first day of culture. G, Gray matter; Staining, Hematoxylin.
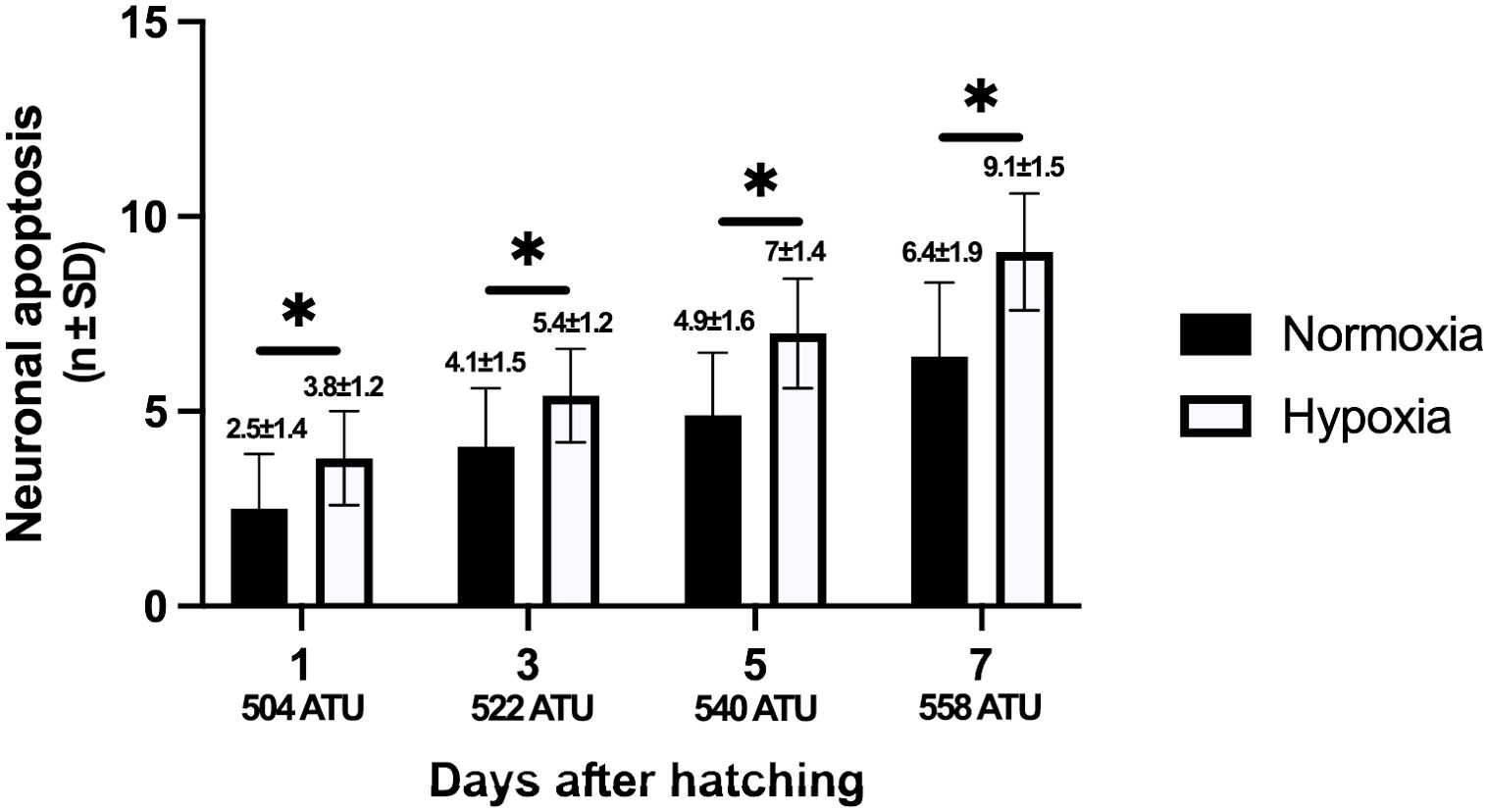
Figure 7. Numbers of apoptotic spinal neurons, indicated by positive immunohistochemistry reaction to caspase-3 antibody. In both conditions, the number of labeled cells increases concomitantly with age; however, statistically significant differences were found when comparing the number of marked cells between the hypoxic and normoxic groups. Higher increases were observed at 3 and 5 days under hypoxic conditions. Scores over each bar indicate the mean value and standard deviation. Asterisks indicate statistically significant differences (p<0.05). A one-way ANOVA was used.
4 Discussion
This study encompasses the early post-hatching ages 1, 3, 5, and 7 days of salmon (Salmo salar), which in accumulated thermal units correspond to 504, 522, 540, and 558 ATU. These ages were selected because they correspond to the stage of differentiation of neuroblasts into neurons (Hernández and Rojas, 2013). The stage of high sensitivity of nerve cells to possible influences from various internal morphogens as well as aquatic teratogens agents, which can potentially alter their normal development) (Adeyemi et al., 2015). The concept “state of high sensitivity in neuroembryological development” refers to the stage of differentiation of neuroblasts into neurons. During this period, nerve cells are extremely susceptible to possible influences from various internal morphogens as well as aquatic teratogen agents, which can potentially alter their normal development.
The control group confirms the fact that initially the spinal cord neurons develop, forming a circular cluster around the central canal of the spinal cord until the 5 post-hatching days. According to Anderson and Waxman (1983a) and Ilies et al. (2018), the continuous incorporation of new neurons and glial cells contributes to the growth of the central nervous system in fish. In rainbow trout (Oncorhynchus mykiss), the proliferative activity and motoneurone recruitment persist at the spinal cord central canal during larval and some postlarval stages (Alunni et al., 2011). However, the proliferative rate at the cervical and caudal levels became very low after a fish length of 120 mm. A recruitment of new cells from the central canal, formerly acting as a germinal zone from which neuroblasts and glioblasts originate during embryonic stages, occurs in the trout spinal cord at all the stages considered. This proliferative capacity parallels the enormous ability of fish to regenerate axons and even whole neurones in the central nervous system after injuries or experimentally induced lesions (Alunni et al., 2011). Additionally, Sîrbulescu et al. (2017) found that multiple stem cell populations support additive neurogenesis, which mediates the development of the adult spinal cord. It has been known for some decades that spinal cord growth involves the recruitment of new neurons, generated through the differentiation of neuroblasts from the germinal layer (Sipahi and Zupanc, 2018).
We have shown in this study that the number of spinal neurons increased from day 1 to day 7 in both the control and hypoxic groups. This is similar to other teleosts where the process of neurogenesis, which occurs concurrently with post-hatching development, has been well documented. During teleost post-hatching development, there is an increase in the number of neurons in different regions of the nervous system (Anderson and Waxman, 1985; Chernoff et al., 2002; Zupanc and Clint, 2003; Takeda et al., 2008; Zupanc and Sirbulescu, 2011). The dorsal root ganglia and motor neurons are the first to evidence this increase (Leonard et al., 1978; Birse et al., 1980). In the same line, other studies have reported that adult freshwater Gymnotifor maintains a high capacity for regeneration and neurogenesis (Zupanc and Clint, 2003; Zupanc and Sirbulescu, 2011). Similarly, studies have reported that adult Sternachus can produce new neurons and glial cells in the spinal cord from ependymal cells that maintain their mitotic capability (Anderson and Waxman, 1983a, b).
At all stages of development studied, alevins that were grown in hypoxic conditions had fewer spinal cord neurons than those grown in normoxic conditions. Our result is consistent with reports in previous studies (Shang and Wu, 2004; Finn, 2007; Chan et al., 2007). A low-oxygen environment can slow down development (Goda et al., 2003; Kelly et al., 2020; Rojas et al., 2024) and induced changes in cell differentiation (Studer et al., 2000; Acker and Acker, 2004; Gustafsson et al., 2005). On the other hand, studies on salmon have shown that high concentration of oxygen in the aquatic medium speeds up alevin development and greatly increases survival (Hamor and Garside, 1976).
Furthermore, we discovered positive labeling with the HIF-1α factor antibody, which indicates hypoxia in spinal neurons from both the hypoxic and normoxic groups. HIF-1α expression was significantly higher in the group exposed to hypoxic conditions. This finding is consistent with reports by other authors in fish (Xiao, 2015), salmon (Vuori et al., 2006; Kelly et al., 2020), zebrafish (Kajimura et al., 2006; Rojas et al., 2007), and carp (Sollid et al., 2006). Furthermore, chronic hypoxia can increase up to 10 times the level of HIF-1α (Bianciardi et al., 2006).
Hypoxia exposure may also lead to a decrease in the rate of neuronal cell division in alevins. Hypoxia could have a greater impact on this decline (Zupanc and Sirbulescu, 2011). Researchers have linked age, size, and fish longitudinal growth to the regeneration capacity and the number of neurons in the spinal cord (Birse et al., 1980; Anderson and Waxman, 1983a). Some studies propose a concomitant decrease in fish regenerative capacity as age and size increase (Birse et al., 1980). However, the similarity between larval and adult regeneration in zebrafish mirrors the plasticity of undifferentiated neurons during the early stages of development (Zeng et al., 2022). Zebrafish have demonstrated some ability to regenerate parts of their nervous system after an injury (Goldman et al., 2001; Lee et al., 2022). Hypoxic conditions, on the other hand, can significantly slow its growth (Shang and Wu, 2004; Chan et al., 2007; Kelly et al., 2020), which can change how cells differentiate after hatching (Fetcho and McLean, 2010). This relates to studies indicating an association between exposure to hypoxia and the deformation of the body and fin, as well as an agreement between hypoxia and the total length of the fry and fin (Rojas et al., 2024).
In this study, we observed an increase in caspase-3 expression in the alevin groups subjected to hypoxia compared to those cultured in normoxia conditions. Researchers have found a correlation between an increase in caspase-3 activity and an increase in cell death resulting from hypoxia (Saikumar et al., 1998). Previous reports have confirmed this finding (Casaccia-Bonnefil, 2000; Saito et al., 2005; Zeng et al., 2022). Exposure to hypoxic environments triggers a statistically significant increase in the rate of cell death. Caspase-3 levels in developing zebrafish embryos are typically low (Yabu et al., 2001; Yamashita, 2003; Alderman et al., 2018), and reports of apoptosis during the juvenile period have also been published (Cole and Ross, 2001; Williams and Holder, 2000).
Regarding the reduction in motor function, in the present investigation we observed that the hipoxic alevins remained at the bottom of the incubators, displaying considerably less mobility than their counterparts cultured under normoxia conditions. This finding corroborates previous observations reported by other authors (McLean and Fetcho, 2009; Fetcho and McLean, 2010). After seven days post-hatching, these cells begin to migrate and form the dorsal and ventral lateral columns. The specific motor activity associated with swimming initiates the recruitment of spinal cord neurons to form active neural networks that ultimately give rise to more efficient and tuned neuronal circuits.
This study explores markers and the neurogenic outcome after hypoxia. Although HIF-1α and caspase-3 have been studied under hypoxic conditions in various species (Xiao, 2015), salmon (Vuori et al., 2006; Kelly et al., 2020), zebrafish (Kajimura et al., 2006; Rojas et al., 2007), and carp (Sollid et al., 2006) to date, this diagnostic methodology has not been used in salmon farming. In general, salmon farming freshwater centers limit themselves to cultivating fish under production objectives. To optimize the industrial process, attention is paid to the water quality, oxygenation, pH, temperature, and levels of contaminants. Histological studies in order to assess the state of tissues and organs of the fish are outside its scope and purpose. It is important to remember that, even if the water quality is optimal, an inadequate physiological state of the gills or skin during the first week post-hatching may not allow for good oxygenation. The markers used in this work, in particular HIF-1α, can serve as markers of tissue hypoxia (Castro et al., 2011).
The results reported here contribute to increasing our knowledge about the biological development of Salmo salar, the understanding of the genesis of the spinal cord, and the hypoxic effects on the development of this nervous system structure. This study could also be very helpful for establishing salmon farming conditions because it shows how hypoxia can affect the fry growing and how immunocytochemical techniques using HIF-1α can be used to evidence tissues that are developed under low oxygen concentrations. In future research, HIF-1α and caspase-3 will be overexpressed or knocked down to directly study their roles in spinal cord development when oxygen levels are low.
Indeed, we are planning future studies to assess the long-term effects of hypoxia during fry development. These studies will aim to evaluate how an early hypoxic event (during the fry phase) could affect the number and morphology of spinal cord neurons during subsequent stages of the fish ontogeny. We consider this line of research as crucial in determining the plausibility of spinal cord-level nervous system plasticity and the preservation of the regenerative capacity of teleost fish after early exposure to hypoxia conditions.
5 Conclusions
In farmed Salmo salar alevins, low oxygen levels reduce the number of spinal neurons and raise HIF-1α levels and apoptosis in the spinal cord post-hatch. These results contribute to increasing our knowledge of salmon’s biological development, in particular the genesis of the spinal cord and the effects of hypoxic conditions on the development of this nervous system structure.
Data availability statement
The original contributions presented in the study are included in the article/supplementary material. Further inquiries can be directed to the corresponding authors.
Ethics statement
The Scientific Ethics Committee of the Universidad de La Frontera has approved the experimental protocol (N°061_20). The study was conducted in accordance with the local legislation and institutional requirements.
Author contributions
MR: Conceptualization, Data curation, Formal Analysis, Methodology, Project administration, Validation, Writing – original draft, Writing – review & editing. HH: Data curation, Investigation, Methodology, Writing – original draft, Writing – review & editing. CSm: Formal Analysis, Writing – original draft, Writing – review & editing. MP: Data curation, Writing – original draft, Writing – review & editing. CSa: Formal Analysis, Writing – original draft, Writing – review & editing. RS: Data curation, Formal Analysis, Writing – original draft, Writing – review & editing. KB: Formal Analysis, Writing – original draft, Writing – review & editing. RC: Data curation, Investigation, Methodology, Writing – original draft, Writing – review & editing.
Funding
The author(s) declare that financial support was received for the research, authorship, and/or publication of this article. This study was supported by grants from the Dirección de Investigación y Desarrollo, Universidad de La Frontera, Chile (DIUFRO DI20-0033).
Conflict of interest
The authors declare that the research was conducted in the absence of any commercial or financial relationships that could be construed as a potential conflict of interest.
The reviewer DC declared a shared affiliation with the author KB to the handling editor at the time of review.
Publisher’s note
All claims expressed in this article are solely those of the authors and do not necessarily represent those of their affiliated organizations, or those of the publisher, the editors and the reviewers. Any product that may be evaluated in this article, or claim that may be made by its manufacturer, is not guaranteed or endorsed by the publisher.
References
Acker T., Acker H. (2004). Cellular oxygen sensing need in CNS function: Physiological and pathological implications. J. Exp. Biol. 207, 3171–3188. doi: 10.1242/jeb.01075
Adeyemi J. A., da Cunha Martins-Junior A., Barbosa F. Jr. (2015). Teratogenicity, genotoxicity and oxidative stress in zebrafish embryos (Danio rerio) co-exposed to arsenic and atrazine. Comp. Biochem. Physiol. Part C.: Toxicol. Pharmacol. 172, 7–12. doi: 10.1016/j.cbpc.2015.04.001
Alderman S. L., Leishman E. M., Fuzzen M. L., Bernier N. J. (2018). Corticotropin-releasing factor regulates caspase-3 and may protect developing zebrafish from stress-induced apoptosis. Gen. Comp. Endocrinol. 265, 207–213. doi: 10.1016/j.ygcen.2018.05.025
Alunni A., Pierucci F., Aita M., Margotta V., De Vita R., Alfei L. (2011). Proliferative activity and motoneurone recruitment persist at the spinal cord central canal during larval and some postlarval stages in the rainbow trout (Oncorhynchus mykiss). Eur. J. Histochem. 45, 191–202. doi: 10.4081/1629
Anderson M., Waxman S. (1983a). Caudal spinal cord of the teleost Sternachus albifrons. Anatomical. Records. 205, 85–92. doi: 10.1002/ar.1092050111
Anderson M., Waxman S. (1983b). Regeneration of spinal neurons in inframammalian vertebrates: Morphological and developmental aspects. J. Hirnforschung. 24, 371–398.
Anderson M., Waxman S. (1985). Neurogenesis in adult vertebrate spinal cord in situ and in vitro: A new model system. Ann. New York Acad. Sci. 457, 213–233. doi: 10.1111/j.1749-6632.1985.tb20807.x
Angiulli E., Pagliara V., Cioni C., Frabetti F., Pizzetti F., Alleva E., et al. (2020). Increase in environmental temperature affects exploratory behaviour, anxiety and social preference in Danio rerio. Sci. Rep. 10, 5385. doi: 10.1038/s41598-020-62331-1
AnvariFar H., Amirkolaie A. K., Miandare H. K., Ouraji H., Jalali M. A., Üçüncü S. I. (2017). Apoptosis in fish: environmental factors and programmed cell death. Cell Tissue Res. 368, 425–439. doi: 10.1007/s00441-016-2548-x
Bianciardi P., Fantacci M., Caretti A., Ronchi R., Milano G., Morel S., et al. (2006). Chronic in vivo hypoxia in various organs: Hypoxia-inducible factor-1α and apoptosis. Biochem. Biophys. Res. Commun. 342, 875–880. doi: 10.1016/j.bbrc.2006.02.042
Birse S., Leonard R., Coggeshall R. (1980). Neuronal increase in various areas of the nervous system of the guppy, Lebistes. J. Comp. Neurol. 194, 291–301. doi: 10.1002/cne.901940202
Calvert J., Cahill J., Yamaguchi-Okada M., Zhang J. (2006). Oxygen treatment after experimental hypoxia-ischemia in neonatal rats alters expression of HIF-1α and its downstream target genes. J. Appl. Physiol. 101, 853–865. doi: 10.1152/japplphysiol.00268.2006
Canosa L. F., Bertucci J. I. (2023). The effect of environmental stressors on growth in fish and its endocrine control. Front. Endocrinol. 14. doi: 10.3389/fendo.2023.1109461
Casaccia-Bonnefil P. (2000). Cell death in the oligodendrocyte linage: A molecular perspective of life/death decisions in development and disease. Glia 29, 124–135. doi: 10.1002/(sici)1098-1136(20000115)29:2<124::aid-glia5>3.0.co;2-o
Castro R., Bustos-Obregón E., Rojas M. (2011). Hypoxia is like an ethiological factor in vertebral column deformity of salmon (Salmo salar). Aquaculture 316, 13–19. doi: 10.1016/j.aquaculture.2011.03.012
Cerqueira V., Pimentel J., Korus J., Bravo F., Amorim J., Oliveira M., et al. (2024). Forecasting ocean hypoxia in salmonid fish farms. Front. Aquac. 3. doi: 10.3389/faquc.2024.1365123
Chan C. Y., Lam W. P., Wai M. S., Wang M., Foster E. L., Yew D. (2007). Perinatal hypoxia induces anterior chamber changes in the eyes of offspring fish. J. Reprod. Dev. 53, 1159–1167. doi: 10.1262/jrd.19018
Chang H. Y., Yang X. (2000). Proteases for cell suicide: functions and regulation of caspases. Microbiol. Mol. Biol. Rev. 64, 821–846. doi: 10.1128/MMBR.64.4.821-846.2000
Chernoff E., Sato K., Corn A., Karcavich R. (2002). Spinal cord regeneration: Intrinsic properties and emerging mechanisms. Semin. Cell Dev. Biol. 13, 361–368. doi: 10.1016/s1084952102000927
Cole L. K., Ross L. S. (2001). Apoptosis in the developing zebrafish embryo. Dev. Biol. 240, 123–142. doi: 10.1006/dbio.2001.0432
Fetcho J., McLean D. L. (2010). Some principles of organization of spinal neurons underlying locomotion in zebrafish and their implications. Ann. New York Acad. Sci. 1198, 94–104. doi: 10.1111/j.1749-6632.2010.05539.x
Finn R. N. (2007). The physiology and toxicology of salmonid eggs and larvae in relation to water quality criteria. Aquat. Toxicol. 81, 337–354. doi: 10.1016/j.aquatox.2006.12.021
Goda N., Ryan H. E., Khadivi B., McNulty W., Rickert R., Randall J. (2003). Hypoxia-inducible factor 1α is essential for cell cycle arrest during hypoxia. Mol. Cell. Biol. 23, 359–369. doi: 10.1128/MCB.23.1.359-369.2003
Goldman D., Hankin M., Li Z., Dai X., Ding J. (2001). Transgenic zebrafish for studying nervous system development and regeneration. Transgenic Res. 10, 21–33. doi: 10.1023/a:1008998832552
González G. G., Martín M. V. (1998). Aspectos Biológicos, anatomía microscópica y enfermedades infecciosas de los salmónidos (Madrid, Spain: Museo Nacional de Ciencias Naturales).
Greijer A. E., van der Wall E. (2004). The role of hypoxia inducible factor 1 (HIF-1) in hypoxia induced apoptosis. J. Clin. Pathol. 57, 1009–1014. doi: 10.1136/jcp.2003.015032
Gustafsson M., Zheng X., Pereira T., Grandin K., Jin S., Lundkvist J., et al. (2005). Hypoxia requires notch signaling to maintain the undifferentiated cell state. Dev. Cell 9, 617–628. doi: 10.1016/j.devcel.2005.09.010
Halterman M. W., Miller C. C., Federoff H. J. (1999). Hypoxia-inducible factor-1alpha mediates hypoxia-induced delayed neuronal death that involves p53. J. Neurosci. 19, 6818–6824. doi: 10.1523/JNEUROSCI.19-16-06818.1999
Hamor T., Garside E. T. (1976). Developmental rates of embryos of Atlantic salmon, Salmo salar L., in response to various levels of temperature, dissolved oxygen, and water exchange. Can. J. Zool. 54, 1912–1917. doi: 10.1139/z76-221
Heise K., Estevez M. S., Puntarullo S., Galleano M., Nikinmaa M., Portner H. O., et al. (2007). Effects of seasonal and latitudinal cold on oxidative stress parameters and activation of hypoxia inducible factor (HIF-1) in zoarcid fish. J. Comp. Physiol. B. 177, 765–777. doi: 10.1007/s00360-007-0173-4
Hernández H. (2011). Efecto de la hipoxia en el desarrollo de la médula espinal en salmón (Salmo salar). Universidad de Chile, Santiago, Chile.
Hernández H., Rojas M. (2013). Desarrollo de la médula espinal de Salmón (Salmo salar) durante el período post-eclosional. Int. J. Morphol. 31, 172–176. doi: 10.4067/S0717-95022013000100029
Ilies I., Sipahi R., Zupanc G. K. (2018). Growth of adult spinal cord in knifefish: Development and parametrization of a distributed model. J. Theor. Biol. 437, 101–114. doi: 10.1016/j.jtbi.2017.10.012
Kajimura S., Aida K., Duan C. (2006). Understanding hypoxia-Induced gene expression in early development: In vitro and in vivo analysis of hypoxia-inducible factor 1-regulated zebra fish insulin-like growth factor binding protein 1 gene expression. Mol. Cell. Biol. 26, 1142–1155. doi: 10.1128/MCB.26.3.1142-1155.2006
Kelly T., Johnsen H., Burgerhout E., Tveiten H., Thesslund T., Andersen Ø., et al. (2020). Low oxygen stress during early development influences regulation of hypoxia-response genes in farmed atlantic salmon (Salmo salar). G3 (Bethesda. Md.) 10, 3179–3188. doi: 10.1534/g3.120.401459
Kestemont P., Baras E. (2001). “Environmental factors and feed intake: mechanisms and interactions,” in Food intake in fish. Eds. Houlihan D. F., Boujard T., Jobling M. (Blackwell Science, Oxford), 131–156.
Krasnov A., Burgerhout E., Johnsen H., Tveiten H., Bakke A. F., Lund H., et al. (2021). Development of Atlantic Salmon (Salmo salar L.) Under Hypoxic Conditions Induced Sustained Changes in Expression of Immune Genes and Reduced Resistance to Moritella viscosa. Front. Ecol. Evol. 9. doi: 10.3389/fevo.2021.722218
Lee H. C., Lai W. L., Lin C. Y., Zeng C. W., Sheu J. C., Chou T. B., et al. (2022). Anp32a promotes neuronal regeneration after spinal cord injury of zebrafish embryos. Int. J. Mol. Sci. 23, 15921. doi: 10.3390/ijms232415921
Leonard R., Coggeshall R., Willis W. (1978). A documentation of an age related increase in neuronal and axonal numbers in the stingray, Dasyatis sabina, Leseuer. J. Comp. Neurol. 179, 13–22. doi: 10.1002/cne.901790103
Mandic M., Todgham A. E., Richards J. G. (2009). Mechanisms and evolution of hypoxia tolerance in fish. Proc. Biol. Sci. 276, 735–744. doi: 10.1098/rspb.2008.1235
McLean D. L., Fetcho J. R. (2009). Spinal interneurons differentiate sequentially from those driving the fastest swimming movements in larval zebrafish to those driving the slowest ones. J. Neurosci. 29, 13566–13577. doi: 10.1523/JNEUROSCI.3277-09.2009
Nikinmaa M., Rees B. (2005). Oxygen-dependent gene expression in fishes. Am. J. Physiology-Regulatory. Integr. Comp. Physiol. 288, 1079–1090. doi: 10.1152/ajpregu.00626.2004
Pellón M., Rojas M., Saint-Pierre G., Hartley R., del Sol M. (2016). Morphology of the eyeball, orbit and retina of atlantic salmon (Salmo salar) alevins under hypoxic conditions. Int. J. Morphol. 34, 320–329. doi: 10.4067/S0717-95022016000100046
Piret J. P., Mottet D., Raes M., Michiels C. (2002). Is HIF-1 alpha a pro-or anti-apoptotic protein? Biochem. Pharmacol. 64, 889–892. doi: 10.1016/S0006-2952(02)01155-3
Rahman S., Thomas P. (2007). Molecular cloning, characterization and expression of two hypoxia-inducible factor alpha subunits, HIF-1alpha and HIF-2 alpha, in a hypoxia-tolerant marine teleost, atlantic croaker (Micropogonias undulatus). Gene 396, 273–282. doi: 10.1016/j.gene.2007.03.009
Richards J. G. (2011). Physiological, behavioral and biochemical adaptations of intertidal fishes to hypoxia. J. Exp. Biol. 214, 191–199. doi: 10.1242/jeb.047951
Rojas D., Perez-Munizaga D., Centanin L., Antonelli M., Wappner P., Allende M., et al. (2007). Cloning of hif-1 alfa and hif-2 alfa and mRNA expression pattern during development in zebrafish. Gene Expression Patterns. 7, 339–345. doi: 10.1016/j.modgep.2006.08.002
Rojas M., Salvatierra R., Smok C., Sandoval C., Souza- Mello V., del Sol M. (2024). Effect of hypoxia on the post-hatching growth of the body of the fry and the caudal fin of the Atlantic Salmon (Salmo salar). Front. Mar. Sci. 11. doi: 10.3389/fmars.2024.1425671
Roman M. R., Brandt S. B., Houde E. D., Pierson J. J. (2019). Interactive effects of hypoxia and temperature on coastal pelagic zooplankton and fish. Front. Mar. Sci. 6. doi: 10.3389/fmars.2019.00139
Saikumar P., Dong Z., Patel Y., Hall K., Hopfer U., Weinberg J., et al. (1998). Role of hypoxia-induced bax translocation and cytochrome c release in reoxygenation injury. Oncogene 17, 3401–3415. doi: 10.1038/sj.onc.1202590
Saito A., Maier C., Narasimhan P., Nishi T., Song Y. S., Yu F., et al. (2005). Oxidative stress and neuronal death/survival signaling in cerebral ischemia. Mol. Neurobiol. 31, 105–116. doi: 10.1385/MN:31:1-3:105
Semenza G. (2008). Regulation of oxygen homeostasis by hypoxia-inducible factor 1. Physiology 24, 97–106. doi: 10.1152/physiol.00045.2008
Shang E., Wu R. (2004). Aquatic hypoxia is a teratogen and affects fish embryonic development. Environ. Sci. Technol. 38, 4763–4767. doi: 10.1021/es0496423
Shingo K., Katsumi A., Cunming D. (2006). Understanding hypoxia-induced gene expression in early development: in vitro and in vivo analysis of hypoxia-inducible factor 1-regulated zebra fish insulin-like growth factor binding protein 1 gene expression. Mol. Cell. Biol. 26, 1142–1155. doi: 10.1128/MCB.26.3.1142-1155.2006
Sipahi R., Zupanc G. K. (2018). Stochastic cellular automata model of neurosphere growth: Roles of proliferative potential, contact inhibition, cell death, and phagocytosis. J. Theor. Biol. 445, 151–165. doi: 10.1016/j.jtbi.2018.02.025
Sîrbulescu R. F., Ilieş I., Meyer A., Zupanc G. K. H. (2017). Additive neurogenesis supported by multiple stem cell populations mediates adult spinal cord development: A spatiotemporal statistical mapping analysis in a teleost model of indeterminate growth. Dev. Neurobiol. 77, 1269–1307. doi: 10.1002/dneu.22511
Sollid J., Rissanen E., Tranberg H., Thorstensen T., Vuori K., NiIkinmaa M., et al. (2006). HIF-1α and iNOS in crucian carp gills during hypoxia-induced transformation. J. Comp. Physiol. B. 176, 359–369. doi: 10.1007/s00360-005-0059-2
Studer L., Csete M., Sang-Hun L., Kabbani N., Walikonis J., Wold B., et al. (2000). Enhanced proliferation, survival, and dopaminergic differentiation of CNS precursors in lowered oxygen. J. Neurosci. 20, 7377–7383. doi: 10.1523/JNEUROSCI.20-19-07377.2000
Suzuki H., Tomida A., Tsuruo T. (2001). Dephosphorylated hypoxia-inducible factor 1 alpha as a mediator of p53-dependent apoptosis during hypoxia. Oncogene 20, 5779–5788. doi: 10.1038/sj.onc.1204742
Takeda A., Nakano M., Goris R. C., Funakoshi K. (2008). Adult neurogenesis with 5-HT expression in lesioned goldfish spinal cord. Dev. Neurosci. 151, 1132–1141. doi: 10.1016/j.neuroscience.2007.10.059
Toni M., Angiullia E., Miccolia G., Cionia C., Allevac E., Frabettid F., et al. (2019). Environmental temperature variation affects brain protein expression and cognitive abilities in adult zebrafish (Danio rerio): A proteomic and behavioural study. J. Proteomics 204, 103396. doi: 10.1016/j.jprot.2019.103396
Vuori K., Koskinen H., Krasnov A., Koivumaki P., Afanasyev S., Vuorinen P., et al. (2006). Developmental disturbances in early-stage mortality (M74) of baltic salmon fry as studied by changes in gene expression. BMC Genomics 7, 56. doi: 10.1186/1471-2164-7-56
West M. J., Slomianka L., Gundersen H. J. (1991). Unbiased stereological estimation of the total number of neurons in the subdivisions of the rat hippocampus using the optical fractionator. Anatomical. Rec. 231, 482–497. doi: 10.1002/ar.1092310411
Williams J. A., Holder N. (2000). Cell turnover in neuromasts of zebrafish larvae. Hearing. Res. 143, 171–181. doi: 10.1016/s0378-5955(00)00039-3
Xiao W. (2015). The hypoxia signaling pathway and hypoxic adaptation in fishes. Sci. China Life Sci. 58, 148–155. doi: 10.1007/s11427-015-4801-z
Yabu T., Kishi S., Okasaki T., Yamashita M. (2001). Characterization of zebrafish caspase-3 and induction of apoptosis through ceramide generation in fish fathead minnow tailbud cells and zebrafish embryo. Biochem. J. 360, 39–47. doi: 10.1042/0264-6021:3600039
Yamashita M. (2003). Apoptosis in zebrafish development. Comp. Biochem. Physiol. Part B.: Biochem. Mol. Biol. 136, 731–742. doi: 10.1016/j.cbpc.2003.08.013
Zeng C. W., Sheu J. C., Tsai H. J. (2022). Hypoxia-responsive subtype cells differentiate into neurons in the brain of zebrafish embryos exposed to hypoxic stress. Cell Transplant. 31, 1–18. doi: 10.1177/09636897221077930
Zhu C. D., Wang Z. H., Yan B. (2013). Strategies for hypoxia adaptation in fish species: a review. J. Comp. Physiol. B. 183, 1005–1013. doi: 10.1007/s00360-013-0762-3
Zupanc G. K., Clint S. C. (2003). Potential role of radial glia in adult neurogenesis of teleost fish. Glia 43, 77–86. doi: 10.1002/glia.10236
Keywords: development, hypoxia, neuroplasticity, salmon, spinal cord
Citation: Rojas M, Hernández H, Smok C, Pellón M, Sandoval C, Salvatierra R, Birditt K and Castro R (2024) Effect of hypoxia in the post-hatching development of the salmon (Salmo salar L.) spinal cord. Front. Mar. Sci. 11:1451254. doi: 10.3389/fmars.2024.1451254
Received: 19 June 2024; Accepted: 30 September 2024;
Published: 14 October 2024.
Edited by:
Mattia Toni, Sapienza University of Rome, ItalyReviewed by:
Daniel Sobrido Cameán, University of Cambridge, United KingdomYu-Chia Chen, University of Helsinki, Finland
Doaa M Mokhtar, Assiut University, Egypt
Copyright © 2024 Rojas, Hernández, Smok, Pellón, Sandoval, Salvatierra, Birditt and Castro. This is an open-access article distributed under the terms of the Creative Commons Attribution License (CC BY). The use, distribution or reproduction in other forums is permitted, provided the original author(s) and the copyright owner(s) are credited and that the original publication in this journal is cited, in accordance with accepted academic practice. No use, distribution or reproduction is permitted which does not comply with these terms.
*Correspondence: Cristian Sandoval, Y3Jpc3RpYW4uc2FuZG92YWxAdWZyb250ZXJhLmNs; Rodrigo Castro, cm9kcmlnb2Nhc3Ryb0BzYW50b3RvbWFzLmNs