- 1College of Geography and Environmental Science, Henan University, Kaifeng, China
- 2Henan Key Laboratory of Earth System Observation and Modeling, Henan University, Kaifeng, China
- 3Key Laboratory of Geospatial Technology for the Middle and Lower Yellow River Regions (Henan University), Ministry of Education, Kaifeng, China
- 4Key Research Institute of Yellow River Civilization and Sustainable Development and Collaborative Innovation Center on Yellow River Civilization Jointly Built by Henan Province and Ministry of Education, Henan University, Kaifeng, China
- 5College of Hydraulic and Environmental Engineering, China Three Gorges University, Yichang, China
- 6Faculty of Infrastructure Engineering, Dalian University of Technology, Dalian, China
- 7College of Mechanical and Electrical Engineering, Henan University of Technology, Zhengzhou, China
Concentration of trace elements (CTEs) is a significant environmental concern worldwide. This study assessed CTE levels in the Lower Indus River (LIR) by analyzing CTEs in water, sediments, tissues of fish (Cirrhinus mrigala), and macrophytes using electrothermal atomic absorption spectrometry (ETAAS) and flame atomic absorption spectrometry (FAAS). The study shows that CTE range—arsenic (As, 58.7–112.1 μg/L), lead (Pb, 59.9–95.6 μg/L), cadmium (Cd, 3.8–8.1 μg/L), nickel (Ni, 40.9–63.4 μg/L), and zinc (Zn, 590.7–847.6 μg/L)—and water parameters (temperature, pH, COD, BOD, turbidity, and alkalinity) exceeded WHO (World Health Organization) acceptable limits. The CTEs (mg/kg dried basis) were analyzed in fish tissues, and As, Cd, Cr, Cu, Hg, Ni, and Zn have the following accumulation order: liver > gill > muscle. In contrast, Ag and Pb were present in higher amounts in gills than in the liver (gill > liver > muscle). Prediction of bioavailability of CTEs, with the extraction of sediment load with EDTA, revealed that As, Cd, and Zn were among the most bioavailable elements in the LIR. Health risk assessment indicated that the presence of CTEs in the fish could pose potential adverse health effects on humans. The study emphasizes significant ecological and health concerns due to fish consumption in the affected region, noting high risks of non-carcinogenic effects. These insights are essential for policymakers and stakeholders in Sindh Province to manage and reduce trace element pollution.
Highlights
● Elevated trace elements in the Lower Indus River exceed WHO limits, posing health risks.
● High bioaccumulation of As, Cd, and Zn in fish liver and gills raises safety concerns.
● Shannon and Simpson indices revealed substantial seasonal fish diversity and abundance variations.
● Results revealed that people face substantial health hazards (THQ > 1).
● Comprehensive evaluation highlights urgent need for environmental remediation efforts.
1 Introduction
The accelerated industrial and agricultural evolution has led to rising concentrations of trace elements, posing a serious threat to both fish and human health (Stankovic et al., 2014; Cao et al., 2019). Several trace elements are introduced into water bodies, which can accumulate along flows, sediment load, and seafood chain, causing subtle harm or mortality in native fish species (Maurya and Malik, 2018; Rani et al., 2021). Suspended sediment load adsorbs the contaminants from the flowing water, consequently reducing their level in the water column. Trace elements are abeyant in sediments and considered latent contaminants, though they are discharged into the flowing water in response to specific disruptions, posing detrimental effects on ecological systems (Bazrafshan et al., 2016; He et al., 2019). The bedload also supplies both sustenance and habitats for aquatic fauna. Accordingly, trace elements could be directly or indirectly hazardous to aquatic fauna, and their effects can be identified on land through their accumulation and concentration in food chains (El-Kady and Abdel-Wahhab, 2018).
Moreover, analyzing the concentration of trace elements (CTEs) in sediment loads associated with populated regions can reveal human impacts on habitats (Paul et al., 2021) and aid in assessing the risks posed by sewage discharge into water bodies. Very few studies have comprehensively examined the CTEs and evaluated the toxicity and pollution levels in the Lower Indus River (LIR). This study points to the CTEs in the LIR, the primary freshwater resource in the southern region of Sindh Province. Rapid development has resulted in deteriorating water quality, increased water pollution, and heightened vulnerability of the aquatic environment in this region (Azizullah et al., 2021; Noor et al., 2023; Boota et al., 2024). Numerous investigations have examined the contamination of trace elements in different water bodies of Chenab and Indus Rivers (Nawab et al., 2018; Bhat et al., 2021), but the majority of the research was solely focused on the chemical analysis, consequent risk appraisal, and its impact in the muscle of certain fish species.
Cirrhinus mrigala (Cyprinidae family) holds considerable economic value in the river basin (Chatha et al., 2023) and is the sole species in the LIR that is still sought for commercial fisheries. This species was chosen as the sample based on its frequency of occurrence, notable local economic importance in the LIR basin, considerable capacity for accumulating trace elements, and function as a biomarker for assessing environmental contamination. Nevertheless, inadequately regulated fishery, channel regulation, and contamination have collectively led to a significant decrease in both species in the basin (Sarker et al., 2021). This species is highly susceptible to the bioaccumulation of persisting contaminants, primarily due to their high lipid content, long lifespan, and diet predominantly composed of bottom-dwelling organisms (Maurya et al., 2019; Shi et al., 2024). C. mrigala is considered to accumulate great amounts of trace elements (Sanyal et al., 2015; Raman et al., 2022). Although contamination has been acknowledged as a significant threat to the survival of C. mrigala and Clupisoma naziri, its specific impact on C. mrigala still needs to be adequately investigated. Several recent studies attempt to demonstrate a correlation between water bodies pollution and histological changes in C. mrigala. However, further studies are needed to explore these issues in greater depth.
This research aims to assess the fish assemblage and evaluate trace element contamination in sediments, fish tissues, and aquatic macrophytes’ downstream of the hydraulic structure. Presently, there are limited data on contamination in water, sediment loads, and this common fish species in the basin, as well as the extent of human contact and associated health impacts. Hence, it is imperative to obtain comprehensive data to ascertain the health hazards related to the mostly consumed fish species.
2 Materials and methods
2.1 Study area
The Indus River is the largest river in southern Asia and a vital lifeline for the country’s growing economy and cultural heritage. Indus River is the 12th biggest transboundary drainage watershed, ~912,000 km2 (Ali and De Boer, 2007), and almost 80% of agriculture depends on this river (Ijaz et al., 2020). The Indus River originated from the Himalayas in the Tibetan region with a total length of 2,880 km extending across portions of India, China, Afghanistan, and Pakistan (Ali and De Boer, 2007). The Indus River System (IRS) serves as the principal waterway network in Pakistan, encompassing the primary Indus River along with its affiliated eastern tributaries, such as Soan, Chenab, and Ravi Rivers, as well as the western tributaries, including Kabul and Swat Rivers (Boota et al., 2022). These rivers traverse a significant portion of the country’s densely populated industrial and agricultural areas. The river’s water quality is continuously tainted by trace elements’ contamination and associated compounds downstream (D/S) of the industrial states in Peshawar, Amangarh, and Nowshera, as well as by small-scale industrial facilities such as sugar, ghee, fabric, tanneries, newspaper, medicinal, and plastic industries, resulting in a detrimental impact on the ecosystem’s health. Swat River, situated in the northern area of Khyber Pakhtunkhwa, is a sub-tributary of the Kabul River. It stretches for approximately 240 km, covering a basin area of approximately 14,000 km2. This river serves as a source of potable water for household consumption and facilitates the irrigation of extensive territories in District Swat, Malakand, and the lower Peshawar Valley.
Furthermore, it plays a crucial role in replenishing the nearby aquifers and natural springs (Raman et al., 2022). The primary eastern tributaries of the Indus River include the Soan River, Chenab River, and Ravi River. The Soan River, originating from the springs in Bun village near Murree, is a significant watercourse in the northern Panjab. The Soan River flows for approximately 250 km through the Pothohar region before joining the Indus River at Pirpiyahi near Kalabagh. The increasing discharge of chemical substances from the Sihala Industrial Estate, as well as effluents from urban and wastewater treatment facilities in Rawalpindi and Islamabad, is causing a decline in the quality of the freshwater ecosystem within this river (Zakaullah, 2020). The Chenab River, serving as the principal eastern tributary of the Indus River, has its source in the Lahaul and Spiti districts of Himachal Pradesh, India. The Chenab River is significantly contaminated by the major urbanized industrial zones in Sialkot, Gujarat, Gujranwala, Faisalabad, Jhang, and Multan (Kumar et al., 2023). The Ravi River serves as a transboundary watercourse delineating the borders of India and Pakistan, spanning approximately 725 km. Reports indicate that the river is experiencing a significant level of pollution, primarily attributed to the negligent discharge of substantial quantities of industrial waste, urban and agricultural runoff, and sewage from drainage systems in the region (Haider and Ali, 2013). The cumulative human activities within the Indus Basin are leading to significant pollution of both the riverine water and the surrounding atmosphere with heavy metals (HMs) and other associated compounds. This contamination has the potential to have lasting impacts on the wellbeing of aquatic organisms and the region’s inhabitants in the foreseeable future. The research was conducted in the LIR downstream of the concrete diversion between Guddu and Sukkur Barrage (GSB) in Sindh Province. All the standard methods recommended by fish biologists and limnologists were applied during the field expeditions to assess the impacts of SB on the D/S aquatic ecosystem. The present study was conducted in 2022, in which the 670-km talweg length of LIR located at D/S of the GSB was studied for the ecological impact assessment (Boota et al., 2021). Figure 1 and Table 1 depict the orographic record of the LIR, and a total of nine sites with coordinates (longitudes and latitudes) were labeled.
2.2 Sample collection
Water, sediment, and fish samples were collected at the D/S of the SB for the three seasons, such as the pre-monsoon (PRS), post-monsoon (POS), and monsoonal (MSS) season, of the LIR. Water samples were taken manually at 0.5 m depth using polyethylene-terephthalate bottles (0.5 L capacity), previously soaked in 10% HNO3 for 24 h and then rinsed three to four times with local water. To represent the catch areas, surface sediment load (0.15 m depth) was obtained from four to six spots of the same station by using a disposable plastic tube (acid washed with 0.1 m inner diameter and 0.15 m depth), and samples were kept in 0.5 L of polyethylene-terephthalate canisters. Surficial sediments, obtained at a depth of 5 cm with 30 samples distributed across nine channel sections, were acquired at marginal locations utilizing a polypropylene collector during both dry (n = 18) and wet (n = 14) seasons. The specimens were preserved in a polyethylene container and placed in a freezer at −20°C. Before the commencement of analytical processes, the specimens underwent a thawing process, were sifted through a sieve with a mesh size of less than 74 μm using ultrapure water (Milli-Q®—Millipore/Merck, Darmstadt, Germany), and subsequently dehydrated in an oven set at a temperature of 40°C. The commonly consumed fish samples of C. mrigala (100 cm maximum size), with more than 40 individual samples, were collected using trammel nets, killed with percussive stunning (Van der Oost et al., 2003), and kept at 4°C until processing and analysis.
2.3 Analytical procedure of trace elements in water, sediment, and fish
Water samples from each site were filtered through 0.45-μm cellulose acetate filter paper, acidified with 0.2% (v/v) HNO3 (Merck Pro-appraisal), and stored at 4°C until the time of analysis (Rice et al., 2012). Every set of 40 specimens underwent processing alongside three analytical control samples that were subjected to identical treatment as the specimens. The average concentration of the control samples was deducted from the specimens. The sediment samples were dried out at 40°C until a consistent weight is achieved, sieved with a mesh size (100 μm), and processed using a recoverable (environment accessible) approach. A sample of sediment weighing 200 mg was collected and treated with 6 mL of HNO3 (sub-boiling grade) and 2 mL of chloridic acid (sub-boiling grade), with the microwave oven (speedwave Berghof, Germany) in polytetrafluoroethylene (PTFE) tubes (Arain et al., 2008). The C. mrigala muscle, gills, and liver samples (cut from the area adjacent to the dorsal fin) were extracted, washed with 10% HNO3, and rinsed with water. The biological samples were ground and blended with a vibrational agate ball mill for 5 min. Then, 100 mg of each sample was taken (several organs), and 1 mL of 30% hydrogen oxide (ultrapure) was subjected to drying in an oven at a temperature of 60°C for 48 h in PTFE tubes (Arain et al., 2008). Detailed discussion of physiochemical parameters determined in the laboratory is not explained here but it is provided (Association, 1995).
2.4 Ecological indices
For the aim of the current research, H (Shannon, 1948) and four pertinent indices were measured to describe the fish ecological/aquatic study (Washington, 1984). These indices are essential tools for explaining populations and community structures by ecologists (Thilakarathne et al., 2024). Diversity indices provide important information about the abundance and distribution of fish species in a community and are an important tool for understanding community structure (Ullah et al., 2023). Shannon index (H) and Simpson index (D) were used to calculate fish species diversity (Shannon, 1948; Simpson, 1949). The indices were formulated as follows:
where n is the total number of individuals in a sample, ni is the number of individual species i in the sample, S is the number of species in a sample/population, and pi is the important probability in element i (element i relativized by row total).
2.5 Bioaccumulation factors and human health risk appraisal
Bioaccumulation factors (BAFs) such as biota-water accumulation factor (BWAF) and biota-sediment accumulation factor (BSAF) were used to assess the accumulation of trace elements in response to environmental factors (La Colla et al., 2021).
where Ctb represents the CTEs in the fish tissues (biotic) and Cta represents the CTEs in abiotic systems (BWAF and BSAF). In this research, BAFs were computed for each tissue and expressed as mean across sampling sites. Fish tissue classification is based on the BSAF (Tu et al., 2022), namely, microconcentrator (1 < BSAF < 2), deconcentrator (BSAF < 1), and macroconcentrator (BSAF > 2).
In addition, the target hazard quotient (THQ) was computed to estimate the non-carcinogenic human health appraisal of fish consumption (Varol and Sünbül, 2018), as well as for general population and fishermen (a sensitive group), based on the equations below:
where Ef represents the exposure frequency (1 day/year—general population and 10 day/year—fisherman), Ed is the exposure period (70 years, average lifetime), Fir is the food ingestion rate (g−1 person/day), C. mrigala consumption rate was about 400 g in this manuscript, C is the CTEs in fish muscle (mg/kg, dry weight), Ord is the oral reference dose (mg/kg/day), BWm is the mean adult’s body weight (60 kg), and MTn is the mean exposure period for non-carcinogens (365 days/year, supposed to be 70 years). Based on Avigliano et al. (2019), only elements that exceeded the limit of detection (LOD) in more than 50% of samples. When THQ < 1, then it indicates that the exposure level is lower than the oral reference dose, indicating no risk to health (Barone et al., 2015). Total THQ (TTHQ) was computed as the sum of the individual THQ trace element values (Tabezar et al., 2023).
There are no particular fish consumption data for Pakistan; therefore, we used the values presented in Eqani et al. (2013) where general inhabitants consume 10 g/day and fishermen consume 100 g/day based on the survey conducted along the Chenab River, Pakistan.
2.6 Analytical procedure of macrophytes
The extraction of trace elements in leaf samples—As (0.2 g dry wt), Hg (0.5 g dry wt.), and other elements (1.0 g dry wt)—followed similar protocols for fish samples (Kassaye et al., 2016). Total As was determined by electro-thermal atomic absorption spectrometry (ETAAS), using a spectrometer using Zeeman-effect background correction, and Pd(NO3)2 as a chemical modifier (Mazej and Germ, 2009). Total mercury was determined using cold vapor atomic absorbed spectrometry (FIMS 400 Perkin-Elmer), with a flow injection appraisal system (FIAS) and auto-sampler (AS90) operated by the Winlab-Perkin-Elmer software (Sedak et al., 2022). The other trace elements were measured by using flame atomic absorption spectrometry (FAAS), using a Varian spectrometer (AAS240FS, Santa Clara) equipped with deuterium background correction (Lino et al., 2019). Adopted approach accuracy with certificate reference material is not provided here but is explained (Association, 1995; Lino et al., 2016). In addition, control impact factor (CIF) was employed in order to normalize the fluctuation in levels of concentration observed in samples taken from both U/S and D/S of the barrage location. The CIF was applied to standardize the CTEs changes in the study reach (Puche et al., 2020).
where Cdown and Cup are the average concentration of each element in D/S and U/S samples. The CIF ranges 0–1 (depicts a significant increase or decreasing CTEs in the impacted area), and CIF having a 0.5 value represents no change.
2.7 Statistical analysis
All statistical analyses were performed using OriginPro 2019 and IBM SPSS Statistics 26.0 (IBM Corp., Armonk, NY, USA). Descriptive statistics, including mean and standard deviation, were calculated for the CTEs in water, sediment, and fish tissues. A one-way analysis of variance (ANOVA) was performed to evaluate significant variations in trace elements across different sampling locations and time periods. Post-hoc comparisons were performed using Tukey’s HSD test. Pearson’s correlation coefficients were computed to assess the relationships between CTEs in fish tissues and those in water and sediment samples. A significance level of p < 0.05 was applied to all analyses.
3 Results
3.1 Fish assemblage
A total of 42 fish species belonging to 10 families were collected during PRS, POS, and MSS. Eleven fish species (C. mrigala, Labeo rohita, Cyprinus carpio, Tor putitora, C. naziri, Labeo dyocheilus, Schizothorax plagiostomus, Oreochromis niloticus, Channa punctate, and Macrognathus armatus) are commercially and economically important in the study region. Lowest fish individual’s species (62) were observed at S3 (Table 2), and the number of fish species in the right bank (RB) was higher than the number of fish species found in the left bank in sampling sites. Fish assemblage exhibited high spatial variation (distribution extent), and fish abundance/distribution is highly dependent on habitat conditions (water flow condition) and habitat destruction. Fish species were disturbed (water level fluctuations) during the MSS. However, a few species (Crossocheilus diplochilius, Xenentodon cancila, Puntius sophore, and Puntius sarana) were equally distributed from more than half of the sampling sites during PRS, POS, and MSS.
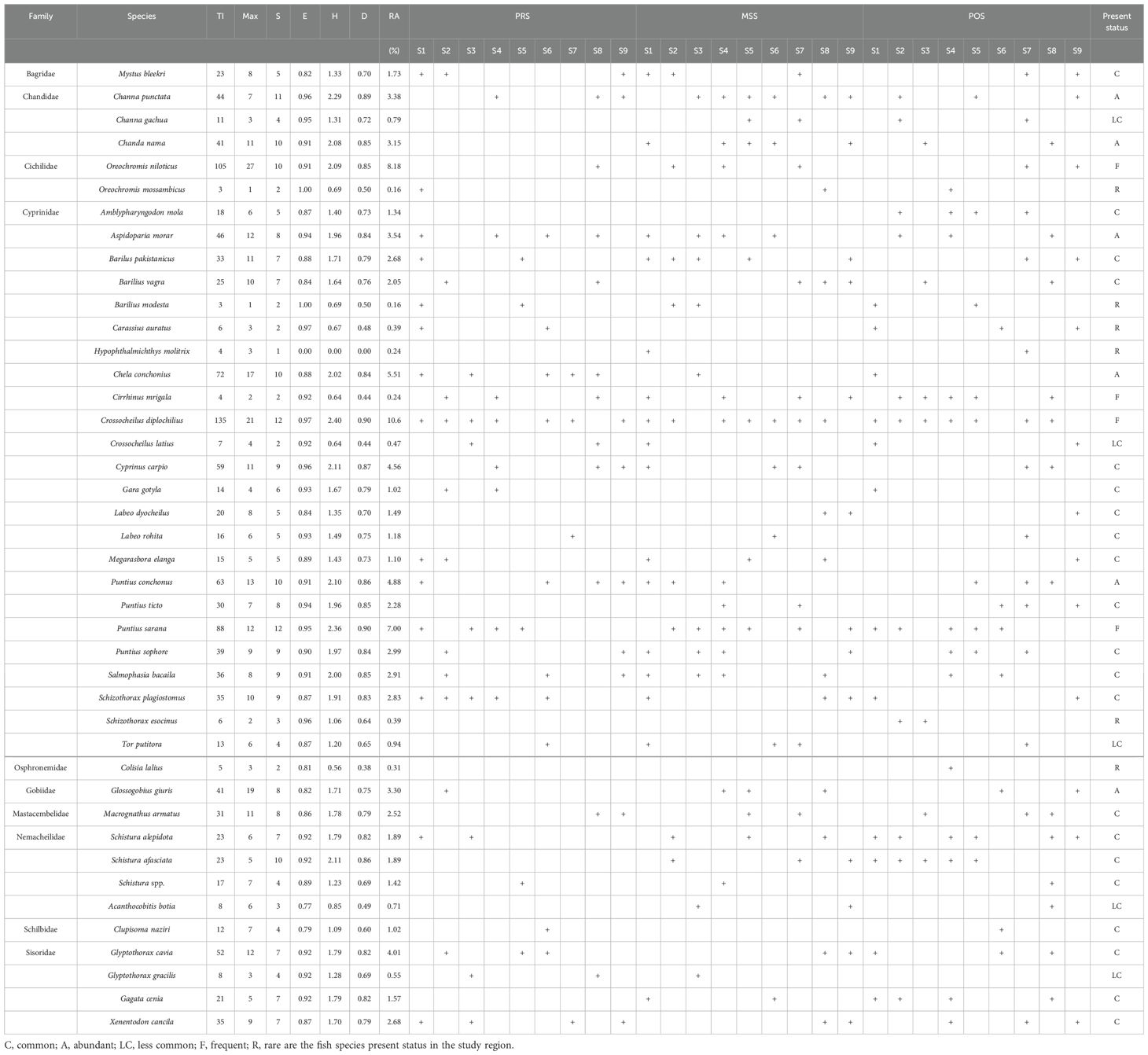
Table 2. Fish diversity, presence (+)/absence data collected, total individuals (TI), relative abundance (RA), trends of four simulated indices (S, E, H, and D) computed and present status in the study area.
Fish species (Amblypharyngodon mola, Schizothorax esocinus, and Colisia lalius) were only found in POS, and L. dyocheilus was completely absent during POS. Few species were present during MSS and POS, but none of the following species were found in PRS: Hypophthalmichthys molitrix, Puntius ticto, Acanthocobitis botia, and Gagata cenia. The average size was observed for non-commercial (20.07 ± 12.97 cm) and commercial fishes (92.65 ± 79.5 cm). It is essential to mention that most captured fishes were non-commercial (smaller size). In contrast, limited commercial fishes were observed in the study region, indicating the impacts of low river flow, intensive fishing, and natural and seasonal variations.
3.2 Diversity indices
Among the detected fishes (1,168 individuals belonging to 42 various species), C. diplochilius (9.54%), Chela conchonius (6.51%), C. mrigala (7.18%), P. sarana (7.00%), Puntius conchonus (5.88%) C. carpio (5.56%), and Glyptothorax cavia (3.01%) were the most frequent species. Abundant captured species included Aspidoparia morar (2.54%), Glossogobius giuris (4.30%), Chanda nama (2.15%), P. sophore (3.99%), Salmophasia bacaila (3.91%), S. plagiostomus (2.03%), Barilus pakistanicus (2.08%), X. cancila (2.98%), M. armatus (2.12%), P. ticto (2.68%), and Barilius vagra (2.05%). Rarely captured species included S. esocinus (0.39%), Carassius auratus (0.39%), C. lalius (0.31%), H. molitrix (0.24%), Oreochromis mossambicus (0.16%), and Barilius modesta (0.16%) (Table 2).
The maximum number of fish individuals was encountered on S6 (159), while the minimum was found on S3 (79). Regarding species richness, 27 species were identified, and only 7 species (minimum) were found to be present on S3. Comprehensive details for relative abundance, fish catch, species richness, and indices are illustrated in Figure 2.
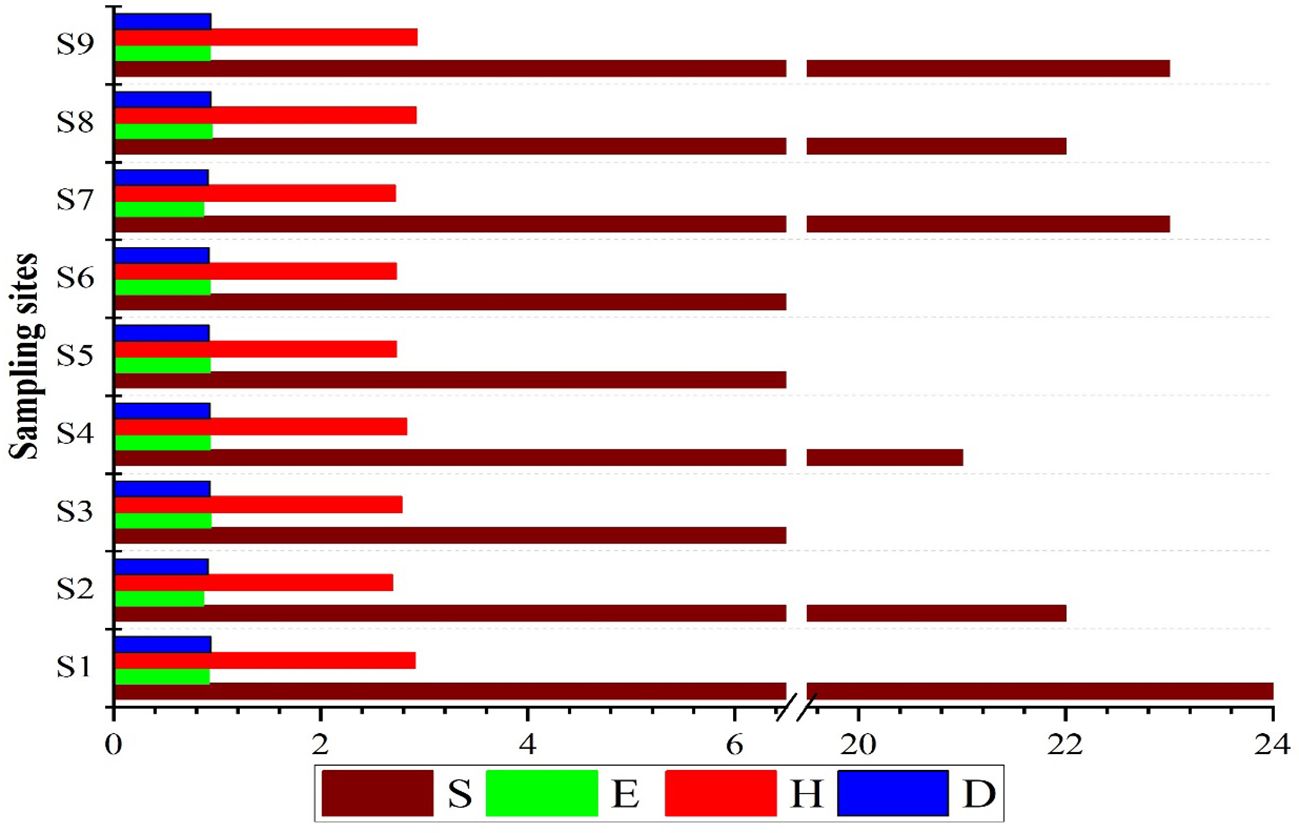
Figure 2. Comprehensive details for the total number of fish specimens, species richness, and four indices computed for nine sampling sites.
3.3 Distribution of trace elements in water and sediments
3.3.1 Appraisal of water quality
The physio-chemical water analysis shows that most of the parameters (EC, TDS, TSS, DO, SAR, K+, Na+, Ca2+, Mg2+, HCO3-, SO42-, and salinity) are within the World Health Organization (WHO) standards (World Health Organization, 2002). In contrast, different parameters such as temperature (maximum at S9, 26.4°C), pH (maximum at S4, 8.85), alkalinity (maximum at S4, 125 mg/L), COD (maximum at S3, 75 mg/L), BOD (maximum at S3, 59 mg/L), and turbidity (maximum at S2, 45.8 NTU) deviate from the WHO standard values, as shown in Figure 3. Ten CTEs in both water and sediment in the LIR are summarized in Table 3. Arsenic concentration in water (58.7–112.1 μg/L) was observed, which is 7–10 times higher than the permissible limit in drinking water (10 μg/L). High concentration of Pb (59.9–95.6 μg/L) was observed, which is >5.9–9.5 times than the permissible limit in water. Levels of Ag and Hg ranged between the LOD, and Cu (18.9–24.4 μg/L) and Cr (0.20–9.4 μg/L) lie within the permissible limits. The CTEs of Cd (3.8–8.1 μg/L), Ni (40.9–63.4 μg/L), and Zn (590.7–847.6 μg/L) also exceeded the recommended values, reflecting that agro-industrial activities may be the primary cause in LIR pollution.
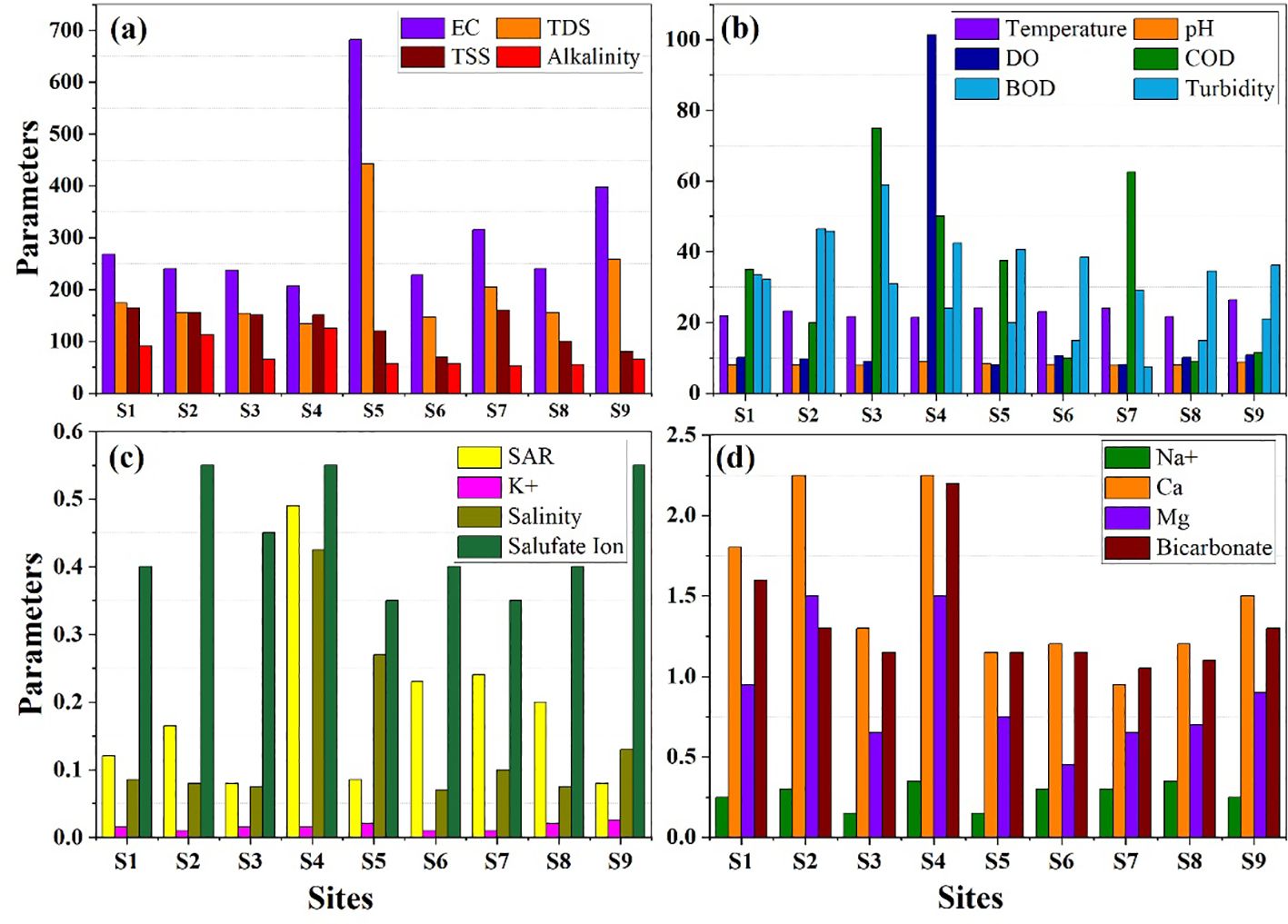
Figure 3. Water quality concentration parameters for WHO standards as: (A) EC, TDS, TSS and Alkalinity concentration; (B) Concentration of Temperature, pH, DO, COD, BOD and Turbidity; (C) SAR, K+, Salinity and Sulfate ion concentration; and (D) Concentration of Na+, Ca, Mg, and Bicarbonate
3.3.2 Assessment of sediment
The pH values in sediment samples were observed to be 8.00 and 8.32, and CTEs in sediment are significantly higher than in water, aligning with the earlier research studies (An and Kampbell, 2003). It is evident that As, Cd, Cr, Cu, Co, Ni, Pb, and Zn continually exceeded the LODs, ranging from 5.0 to 969.6 mg/kg dry weight, while Ag and Hg were within the LOD. The correlation between water and sediment was analyzed using Pearson’s correlation coefficient, which revealed a strong positive correlation between Cr (r ~ 0.944) and Cu (r ~ 0.972) in water, with statistically significant p-values supporting the strength of these relationships. In addition, Ag showed relatively lower concentrations within the sampling sites, while a strong correlation was observed in the sediment between Cd (r ~ 0.915) and Ni (r ~ 0.90) in the LIR, with statistically significant p-values (Figure 4).
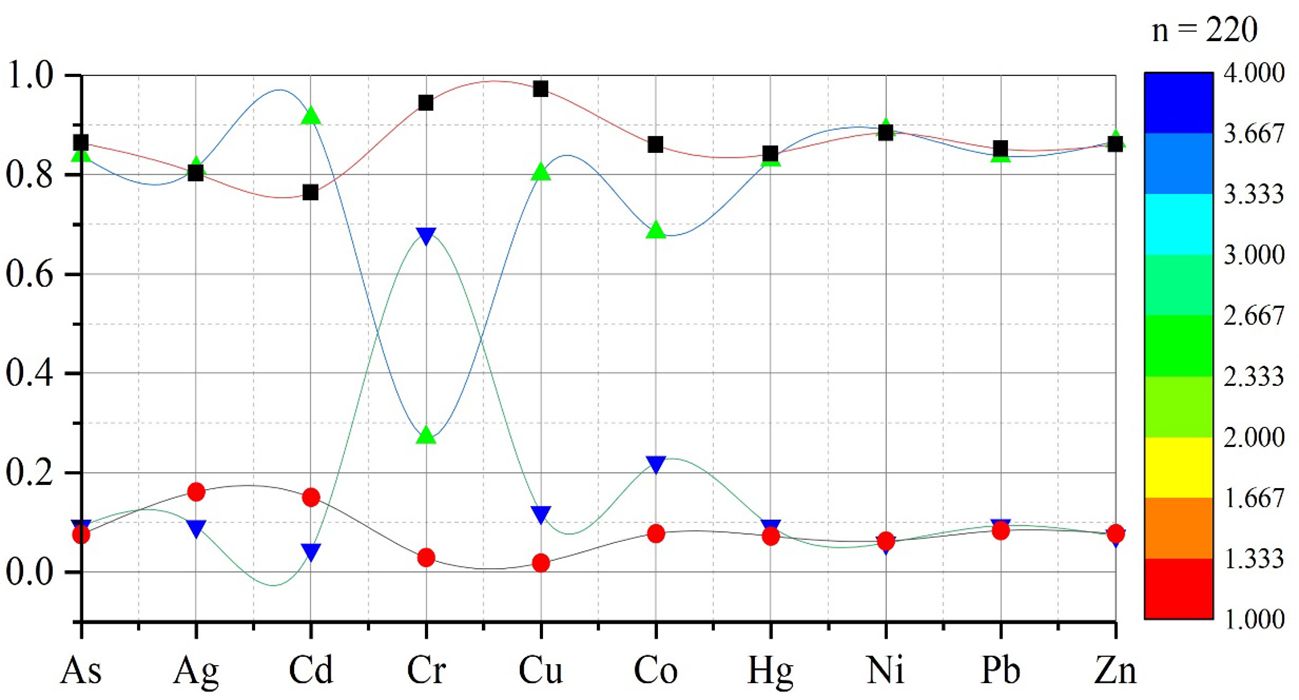
Figure 4. CTEs and upper line illustrate the water (r-value) from 0.864 to 0.861; the line from 0.839 to 0.868 represents the sediment (r-value); the line from 0.076 to 0.078 depicts water (p-value), and the line from 0.093 to 0.073 represents the sediment (p-value).
3.4 Extractable (bioavailable) trace elements
Figure 5 shows the percentage of bioavailable elements extracted by ethylenediaminetetraacetic acid (EDTA) from the overall trace elements in the sediment load of the LIR. It was observed that there is no apparent correlation between overall and bioavailable CTEs; given that trace element distributions are linked to solubility, accessible CTEs might be similar to bioavailable CTEs. The range values (mg/kg dried basis) for EDTA-extracted elements were observed as follows: As (0.23–0.46), Ag (0.225–0.425), Cd (0.21–0.39), Cr (0.07–0.12), Cu (0.2–0.29), Co (0.09–0.27), Hg (0.514–0.908), Ni (0.28–0.47), Pb (0.07–0.36), and Zn (1.8–3.16). The ranking of EDTA-extracted CTEs is not similar to the ranking of total CTEs. The range values for the percentage of extracted values were observed as follows: As (1.72–2.59), Ag (2.715–3.565), Cd (3.55–4.65), Cr (0.39–0.62), Cu (0.7–1.35), Co (2.23–5.34), Hg (1.16–1.672), Ni (1.56–2.18), Pb (0.35–1.58), and Zn (1.65–4.05), respectively.
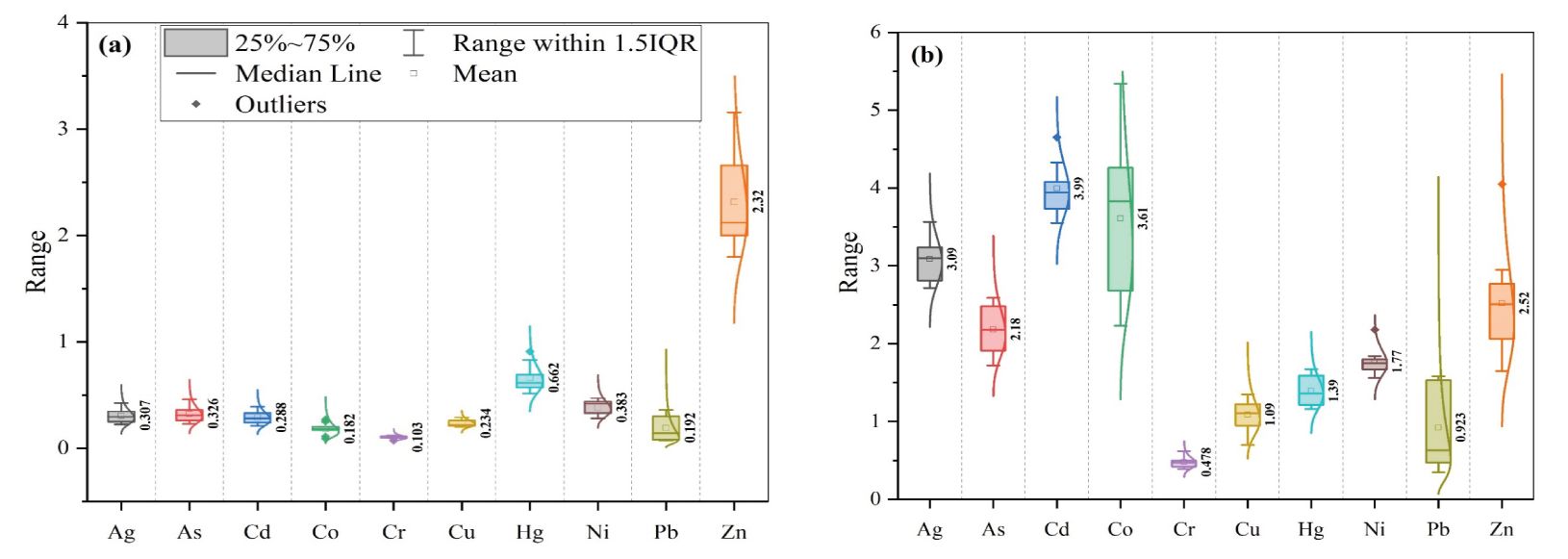
Figure 5. (A) Box plots of EDTA–extractable trace elements from sediment samples and (B) percentage of extracted trace elements with total CTEs in the LIR, with the fittings of normal distributions and box values showing the mean values of metal concentrations.
3.5 CTEs in fish samples
C. mrigala, locally called Mrigal, Mori, or Morakhi, is frequently consumed by inhabitants of different regions in Pakistan and is utilized as a bioassay indicator to assess channel pollution caused by hazardous substances. Nine trace elements (in mg/kg dried basis) were analyzed in fish muscle, liver, and gills (Figure 6A), and As, Cd, Cr, Cu, Hg, Ni, and Zn had the accumulation order of liver > gill > muscle, respectively. In contrast, Ag and Pb were present in higher amounts in the gills than in the liver. Mean accumulation trend in muscle was observed as follows: Zn > As > Pb > Cu > Ni > Cr > Cd > Hg > Ag; mean accumulation order in the liver was as follows: Zn > Cu > Cd > P > As > Ni > Cr > Hg > Ag; and mean accumulation order in the gills was as follows: Zn > Pb > As > Cu > Ni > Cd > Cr > Hg > Ag, respectively.
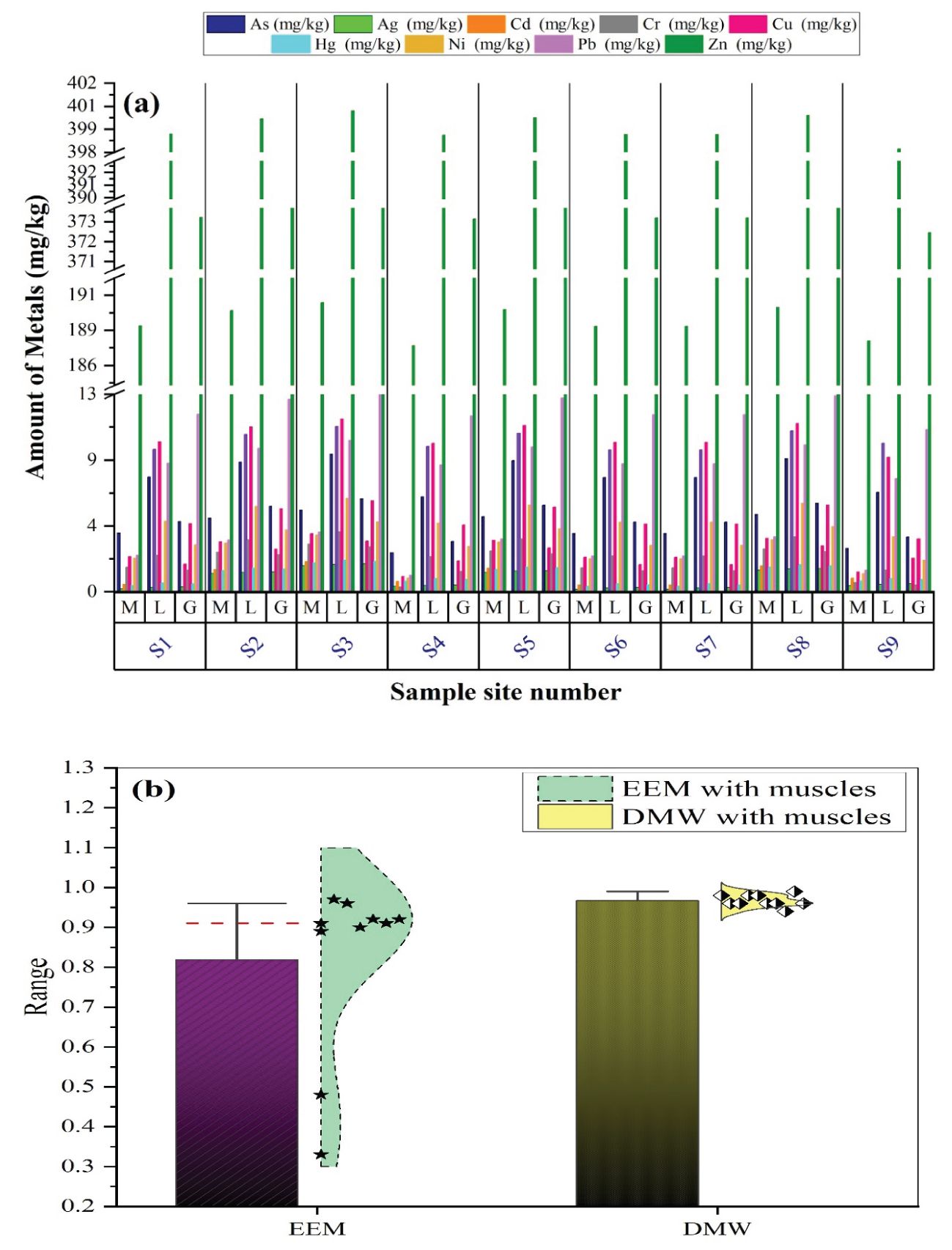
Figure 6. (A) CTEs in M—muscles, L—liver, and G—gills (mg/kg dry wt), (B) appraisal of bar graph—% in muscles (EEM and DMW) with the best-fit kernel smooth distributions. The solid black line represents the error bars, and the red dotted line represents the median lines.
The coefficient of correlation for trace elements in C. mrigala muscle with dissolved components in water and EDTA-extracted components from sediment is also depicted in Figure 6B. Owing to the low correlation between trace element pairs, the p-values and Spearman coefficient of correlation are not provided (−0.27 < r < 0.43, 0.059 < p < 0.89). Lead has a moderate coefficient of correlation, with r = 0.60 between liver and sediment and r = 0.59 (p < 0.001) between gills and sediment, respectively (not illustrated here). The standard length was not correlated with the trace element composition, as there was no significant co-variation between this parameter and element concentrations (analysis of co-variance and Spearman coefficient of correlation, p > 0.049).
4 Discussion
4.1 Bioaccumulation factors
The impact of anthropogenic activity on natural aquatic systems results in concerning amounts of trace elements and metalloids in various environmental components (such as water, sediment load, and biota) worldwide. Notably, the LIR has been significantly influenced by human activities due to agricultural practices and the development of urban regions. Table 4 shows the fish tissues’ BAFs (BWCF and BSCF), with BWCF values > 1 for all tissues. The BWCF values were higher in the liver than in the muscle and gills (muscle presented the lowest values). In particular, the maximum BWCF values were observed in the liver (up to 11,719), and BWCF values in gills were as follows: As (9.2), Cr (210), Cu (305), Ni (240), Pb (1,160), and Zn (2,140), respectively. In contrast, BWCF values were higher in gills (up to 2,140) and lowest in the muscles. Concentrations of Cr, Cu, Ni, Hg, and Zn (values above LOD) in muscle were accounted for to estimate the non-carcinogenic population health risk linked to fish consumption. The CTEs in fish from the present study and other academic works are documented in Table 4. Data obtained from publicly available sources revealed that CTEs in fish tissues exhibited considerable variability, influenced by factors such as geographical location and species of the specimens captured (Table 4).
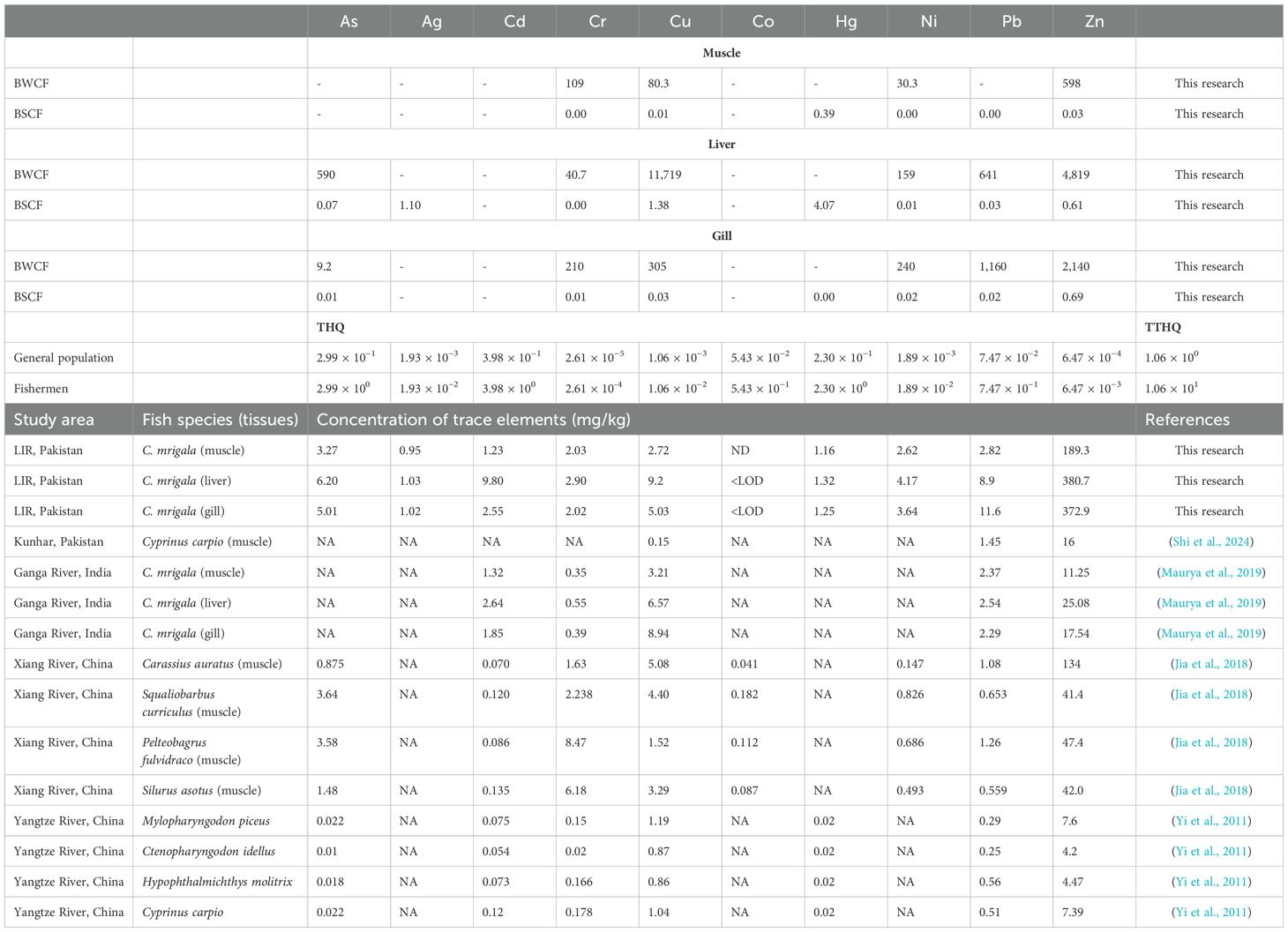
Table 4. Bioaccumulation factors (BWCF and BSCF), THQ, and TTHQ via consumption of fish and fish tissue comparative analysis between this study and previous studies.
4.2 Impact of barrage on aquatic biota
Figure 7 illustrates the conceptual diagram of the hydraulic structure’s impact on the fish fauna. The establishment of barrages and dams along river courses exerts notable effects on the aquatic organisms inhabiting downstream regions, including fish and macrophytes. During periods of reduced flow, such as the low-flow season, the water level decreases either gradually or until it reaches the minimum downstream level of the river. This scenario leads to disastrous circumstances for the aquatic resources, causing disruptions in the fish migration patterns for reproductive purposes. Throughout the investigation, it was determined that the preservation of the minimum ecological water flow was lacking, leading to disruptions in ecological coherence and the abundance of fisheries resources.
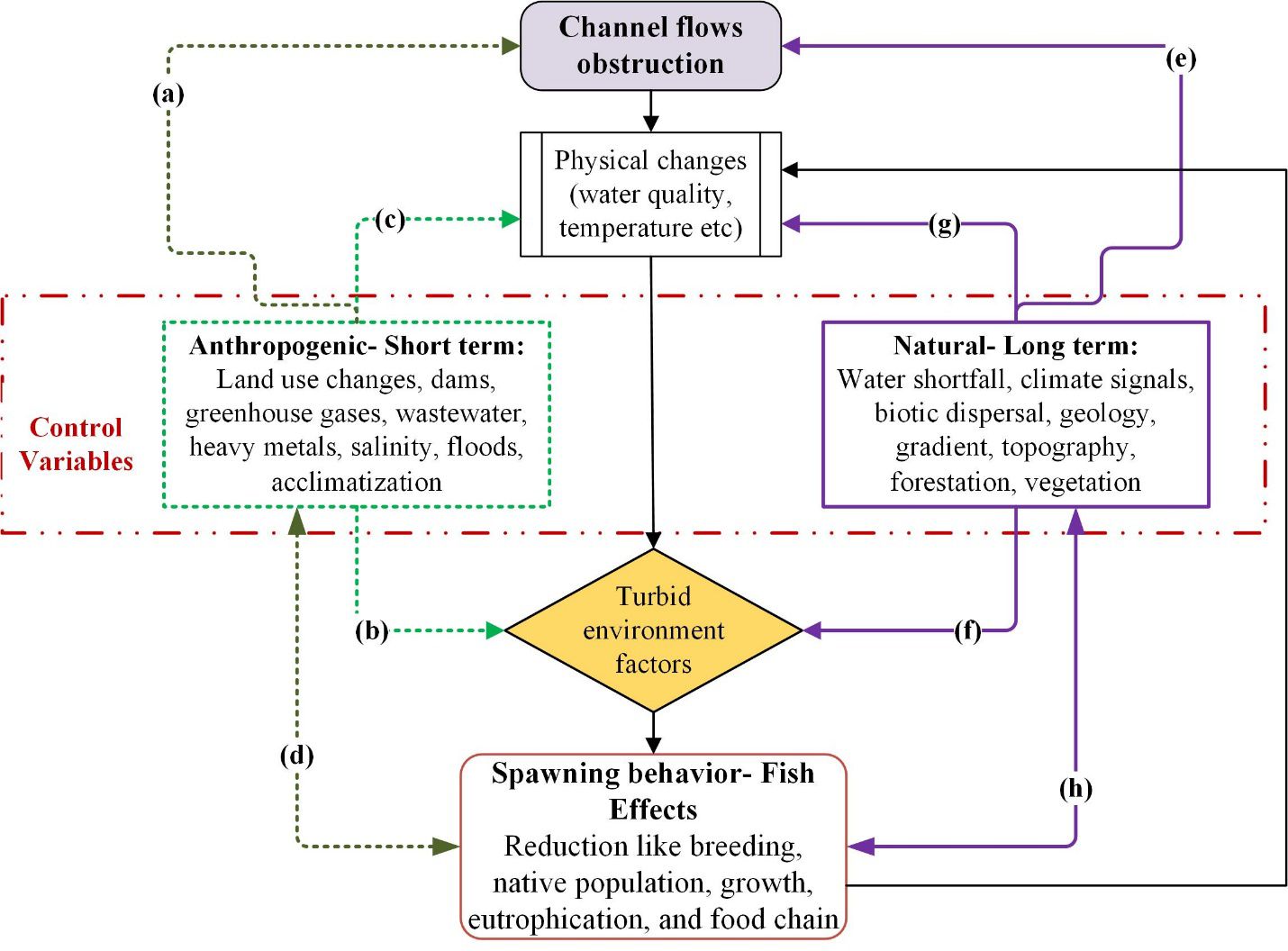
Figure 7. (A–H) Conceptual model describes the hydraulic structure impact by summarizing the anthropogenic (dotted lines) and natural factors (solid lines) on the aquatic fish fauna.
Fish communities exhibit a high level of responsiveness to the river system’s geomorphological attributes and hydrological conditions. From the perspective of fish diversity and the management of fisheries resources, a river habitat must provide an appropriate environment for the growth, spawning, and shelter of fish, as well as ensure a stable food supply. Before the establishment of the GSB barrage, species such as Tor putitora, Schizothorax plagiostomus, Labeo rohita, Cyprinus carpio, and Cirrhinus mrigala were frequently observed in this particular section of the river. After a span of 25 years, the populations of the aforementioned four indigenous species have dwindled to a minimum level, whereas C. carpio, an alien species, has not only acclimated to this particular habitat but has also emerged as a prevalent species due to its versatile behavior and significant reproductive capacity. The fishermen community documented that Tor putitora and Schizothorax plagiostomus were notably more prevalent in previous years. However, their numbers have currently experienced a substantial decline due to habitat fragmentation and diminished water flow.
It is evident that no significant measures have been taken to develop the fisheries resources D/S of the barrage. This stretch of the river has been severely degraded due to the extremely low flow from the GSB. Even the population of fishermen affected by the construction is currently deprived of fishing rights from the pond area. In this regard, a survey of local fishermen and fish retailers living and working on the LIR near the GSB was conducted to know the fisheries’ resources and their economic status. Before the construction of GSB, the total annual catch was approximately 32 tons and this pattern has been adversely affected by the reduction in flows during the winter season. This situation has created some severe concerns within the local community. Most of the professional fishermen and their next generation have changed professions due to low fish catch. Fishermen community along the affected river stretch depends on fish twice or thrice a month for food.
Winter days are better for catching and selling fish because the fishermen do not require refrigeration or cooling system storage. Moreover, the local community prefers to eat fish during the winter season. Sometimes, when they find a good fish catch, they sell it in the local market. Most of the commercial fishermen who were interviewed were members of joint families, where some family members attended to the main occupation while others were fishing. All fishing is done with nylon gillnets, angling, and electric current. Most fishing is done in a daily basis during the fall and winter seasons, and generally, fishing is prohibited during the monsoon season. The fishermen prefer to fish in shallow and slow-moving water. They begin fishing in the late afternoon and continue through the night. The following day, fishermen take the fish to the nearest markets in different cities. Thus, the health risks from consuming the fish (THQ and TTHQ of CTEs for the general population vs. fishermen) were elucidated.
4.3 Human health assessment
Table 4 illustrates a notable disparity in the BSAF for muscle tissue compared to the concentrations documented in the Ganga River, India. For instance, the muscle tissue of C. mrigala displayed a Cr level of 0.35 mg/kg (Maurya et al., 2019), implying a heightened degree of Cr accumulation within the geographical scope of our research. Our study also indicates elevated levels of Cu in liver samples compared to muscle samples collected from the Ganga River (Maurya et al., 2019) and the 0.15 mg/kg detected in C. carpio muscle samples from Kunhar, Pakistan (Shi et al., 2024). This suggests a possible increased environmental occurrence or accessibility of Cu within the geographical scope of our research. The muscle tissue in our study exhibited higher BWCF than the C. carpio from the Yangtze River, China (Yi et al., 2011). This indicates significant Zn accumulation in the indigenous fish population. The BWCF and BSCF values in our investigation exhibit elevated levels for numerous trace elements compared to other geographical areas. Specifically, the BWCF for Zn in liver tissue surpasses all previously documented literature, suggesting a notably increased rate of accumulation in our locality. The distribution of trace elements among various tissues, such as muscle, liver, and gill, enhances our understanding of the bioaccumulation and retention mechanisms in fish. Notably, liver tissue exhibits notably elevated concentrations of Cu and Zn, highlighting its significance as a pivotal organ involved in the detoxification and sequestration.
Table 4 aligns with the assessment of THQ for each trace element and the consequent TTHQ arising from the consumption of fish among the broader population and fishermen living in proximity to the LIR. The current study examined the THQs of CTEs resulting from the intake of LIR fish, following the order As > Hg > Cd > Co > Pb > Cu > Ni > Ag > Zn > Cr for the general population, while for fishermen, it was As > Hg > Cd > Co > Pb > Cu > Ni > Ag > Zn > Cr, respectively. The mean THQs of As, Ag, Cd, Cr, Cu, Co, Hg, Ni, Pb, and Zn were 2.99 × 10−1, 1.93 × 10−3, 3.98 × 10−1, 2.61 × 10−5, 1.06 × 10−3, 5.43 × 10−1, 3.90 × 10−1, 1.89 × 10−3, 7.47 × 10−2, and 6.47 × 10−4 for the general population, and 2.99 × 100, 1.93 × 10−2, 3.98 × 100, 2.61 × 10−4, 1.06 × 10−2, 5.43 × 100, 3.90 × 100, 1.89 × 10−2, 7.47 × 10−1, and 6.47 × 10−3, respectively, for fishermen. The THQ values for As, Hg, and Cd for fishermen, resulting from fish consumption from the LIR, are > 1, indicating a significant health risk due to the intake of these elements. When considering overall fish consumption, As presents the highest THQ risk, followed by Hg and Cd, across the entire study reach. This implies that there is no evidence of an unacceptable non-carcinogenic hazard to the general population from consuming fish sourced from the LIR. The TTHQ of CTEs through the ingestion of fish sourced from the LIR was 1.22 × 10−1 (within the safety limit) and 1.22 × 100 (exceeding the safety limit) for the general population and fishermen, respectively.
Table 4 also provides a comparative analysis of trace elements found in various fish species from different rivers across Pakistan, India, and China. In the LIR, C. mrigala shows significant trace element accumulation, with liver tissues exhibiting the highest concentrations, particularly for elements such as Cr, Ni, and Zn. The gills also show elevated levels, although generally lower than those in the liver. In the Ganga River, C. mrigala displays similar patterns, with trace elements like Cr, Ni, and Zn most concentrated in the liver tissues. This suggests a consistent trend of bioaccumulation in fish liver across different regions (Maurya et al., 2019). Fish from the Xiang River, such as C. auratus and Squaliobarbus curriculus, also exhibit varying trace element concentrations. S. curriculus, in particular, shows higher levels of Zn, indicating significant environmental exposure (Jia et al., 2018). In contrast, species from the Yangtze River, including Mylopharyngodon piceus and C. carpio, generally display lower trace element concentrations across all tissues, suggesting relatively lower pollution levels in this region (Yi et al., 2011). Overall, the data highlight significant regional differences in trace element contamination, with fish from the LIR and Ganga Rivers showing higher levels of accumulation compared to those from the Xiang and Yangtze Rivers.
Cr can exist in multiple oxidation states, with hexavalent Cr being particularly notable for its pronounced toxicity and carcinogenic properties. Prolonged exposure to hexavalent Cr may result in respiratory complications, compromised immune function, and damage to the kidneys and liver. It is a carcinogenic agent associated with the development of lung cancer. Cu, a crucial trace element necessary for a variety of physiological processes, can have detrimental effects if consumed in excess. Acute Cu toxicity manifests gastrointestinal disturbances, hepatic and renal impairment, and hemolysis. Long-term exposure to Cu can result in hepatic injury, renal insufficiency, and disorders in the nervous system. Prolonged exposure to elevated Zn concentrations may lead to Cu deficiency, compromised immune response, and changes in lipid profiles, consequently elevating the susceptibility to cardiovascular disorders. Prolonged Cd exposure can induce renal impairment, depletion of bone minerals resulting in osteoporosis and fractures, and respiratory complications like emphysema. In children, Pb exposure can result in developmental delays, reduced intelligence quotient (IQ), attention deficits, and disturbances in behavior. Ni exposure can induce allergic responses, such as dermatitis, asthma, and various respiratory ailments. Ag exhibits restricted toxicity; however, extended contact with elevated concentrations may result in argyria, characterized by the skin turning a blue-gray hue due to Ag accumulation (Shi et al., 2024).
The comparative study highlights the distinctive toxicological characteristics of our study region, providing significant data to enhance the global understanding of fish pollution and associated health hazards. This can guide future comparative investigations and meta-analyses. Our research offers novel perspectives on the bioaccumulation and potential health implications of trace elements in fish sourced from the LIR, Pakistan, while also drawing attention to notable variations in comparison to other geographical areas. This emphasizes the necessity for ongoing surveillance and implementation of strategies aimed at mitigating the effects of trace element pollution in aquatic environments.
4.3.1 Potential mitigation strategies for trace elements
To address and mitigate the issue of trace element pollution in the LIR and its impact on aquatic ecosystems and human health, several approaches may be considered: (1) Integration of advanced wastewater treatment facilities in industrial and agricultural areas can significantly reduce the release of trace elements into rivers. Techniques such as membrane filtration, ion exchange, and advanced oxidation processes have demonstrated their efficacy in eliminating pollutants from wastewater before it is discharged into the environment. (2) Enhancing environmental regulations and enforcement mechanisms can help minimize the discharge of harmful substances into the river. Regular monitoring of water quality and sediment characteristics at different locations along the river can facilitate the detection of sources and patterns of contamination, allowing for prompt intervention. (3) Enhancing the resilience of the ecosystem is achievable through the restoration and protection of natural habitats along the river. Riparian buffer zones, wetlands, and vegetated areas can function as inherent filtration systems, capturing and decomposing contaminants prior to their entrance into the primary water source. (4) Encouraging the adoption of sustainable agricultural practices can help reduce the runoff of trace elements into the river. This encompasses the use of natural fertilizers, precise application of pest control substances, and the establishment of buffer zones adjacent to riverbanks to absorb pollutants before they enter the aquatic ecosystem. (5) Educating local communities and stakeholders on the origins and consequences of trace element pollution is of paramount importance. Educational initiatives can raise awareness among the general population regarding the significance of sustainable methodologies and the potential health hazards linked to the consumption of polluted fish. (6) Implementing fishery management strategies that include regular assessment of fish health and population trends is crucial for maintaining sustainable fish populations. Enforcing fishing restrictions during key breeding periods and regulating fish size can effectively prevent overfishing and support the recovery of fish populations. (7) Controlling sediment levels by employing dredging techniques and implementing appropriate disposal methods may lead to a decrease in accumulation of trace elements within the riverbed. This approach helps prevent the re-suspension of pollutants amidst periods of increased flow, thereby mitigating their impact on aquatic ecosystems.
By implementing these strategies, it is possible to significantly reduce trace element contamination in the LIR, thereby protecting aquatic ecosystems and improving public health outcomes.
4.4 Distribution and trace elements in macrophytes
A total of 18 species of macrophytes were identified during PRS and POS. Sampling during MSS was not carried out because of high water flow, and most of the macrophyte habitats were either flooded or disturbed. The identified aquatic plants were categorized into four groups: (i) Free-floating hydrophytes (Pistia stratiotes, Eichhornia crassipes, and Lemna minor), (ii) Rooted-submerged hydrophytes (Vallisneria spiralis, Myriophyllum spicatum, Potamogeton crispus, and Hydrilla verticillate), (iii) Submerged floating hydrophytes (Spirogyra maxima and Chara globularis), and (iv) Amphibious hydrophytes (Rorippa nasturtium-aquaticum, Persicaria barbata, Persicaria glabra, Cyperus digitatus, Juncus articulates, Ranunculus scleratus, Typha latifolia, Phragmites australis, and Brachiaria mutica).
Submerged macrophytes are the primary food source for fish. Seasonal and spatial variations of algal and macrophytes are represented in Figure 8. Two species of macrophytes, namely, S. maxima and L. minor L., were present at all the sampling sites during PRS. However, their spatial distribution was disturbed during MSS, and afterward, they were only found at some sampling sites (Table 5). The results also revealed that macrophytes richness and abundance were much higher at the RB sampling sites than at the left-side sampling sites. The highest macrophyte diversity was observed at the confluence of nullahs and streams with the LIR, whereas the lowest diversity was observed at the fast-flowing segments of the river. The highest macrophyte richness was observed along the RB of the LIR. Except for the S5 and S6 sites, macrophyte diversity was higher in PRS than in POS. This indicates that most macrophyte habitats are disturbed during high-flow MSS, and it takes time for them to regain their original diversity.
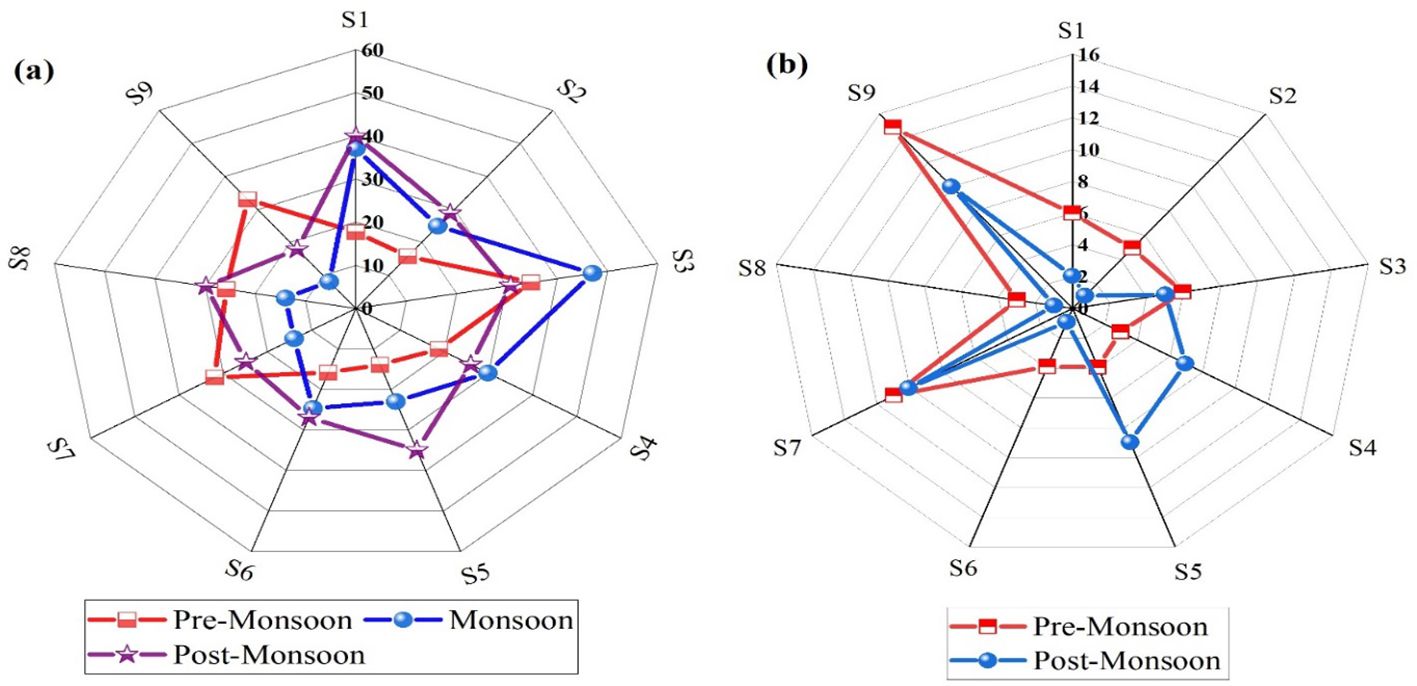
Figure 8. Seasonal and spatial variations in nine sites: (A) algal diversity and (B) macrophyte diversity.
Aquatic macrophytes have been commonly used to determine the state of aquatic ecosystems due to their fast growth, significant bioaccumulation ability, and resilience to trace elements (Ankit et al., 2021). The median CTEs (mg/kg dry wt) were observed as follows: As value for PRS and MSS: 0.70 (U: ± 0.13), 1.23 (U: ± 0.20); Hg value for PRS and MSS: 0.045 (U: ± 0.02), 0.07 (U: ± 0.03); and Cd value for PRS and MSS: 0.33 (U: ± 0.09), 0.34 (U: ± 0.09) in the study reach. It was observed that the Cd median remained constant during the PRS and MSS. In the PRS, As, Hg, and Cd levels were low, with higher medians found below the S6 site (As: 0.69, U: ± 0.13) mg/kg dry wt. In the MSS, the median value of Pb content increased from 2.14 to 4.98 (U: ± 0.22 to ±0.50), respectively. The CIF for the PRS and MSS for various CTEs in macrophyte samples is shown in Figure 9.
5 Conclusions
The present findings improved our understanding of the CTEs in water, sediment, macrophyte, and fish tissues in the LIR. Despite this, the research has shed light on the need for future studies, emphasizing the importance of increasing the sampling quantity and continuity to gain a better understanding of geochemical aspects in the study region. This research found that water and sediment samples are contaminated, exceeding the WHO guidelines, primarily due to the contribution of industrial sewage and wastewater contributing nullahs. Correlation analysis between water and sediment illustrates a strong positive correlation for Cr p-value (0.944) and Cu p-value (~0.972) in water, and Ag has relatively lower values and strong correlation in sediment in Cd (p-value ~0.915) and Ni (p-value ~0.90) in the study reach. Higher CTEs were typically associated with fish tissues such as the liver and gills, while concentration in edible muscles was lower than the permissible limits of hazardous elements for local inhabitants. Furthermore, no correlation was observed between fish size and CTEs, and no direct correlation between tissue components and sampling sites was found, probably due to the high migration rate of species. The higher accumulation of trace elements, especially As, was found in the consumable part of fish samples. Historically, a strong correlation has existed between the river species and climate change, needing the assessment and restoration of the natural environment to prevent channel deterioration. It is, therefore, necessary to focus on CTEs within the framework of climatic change to further investigate the threats they pose to marine organisms. Future studies should prioritize expanding the scope and consistency of sampling, employing advanced analytical techniques to enhance the detection of trace elements, and conducting long-term investigations to observe variations over time. Additionally, research should scrutinize the impacts of climate change on the mobilization of trace elements, perform meticulous concentration evaluations, and carry out assessments of human health risks, particularly focusing on vulnerable communities. Moreover, investigating and enhancing efficient mitigation and remediation strategies, such as phytoremediation and advanced wastewater treatment, will play a pivotal role in minimizing trace element pollution in the LIR. This is essential for safeguarding both the ecosystem and human health.
Data availability statement
The raw data supporting the conclusions of this article will be made available by the authors, without undue reservation.
Author contributions
MB: Writing – review & editing, Writing – original draft, Methodology, Formal analysis, Data curation. S-E-HS: Writing – original draft, Methodology, Conceptualization. HX: Writing – review & editing, Supervision, Resources, Project administration, Funding acquisition. YQ: Writing – review & editing, Supervision, Resources. MI: Writing – review & editing, Visualization, Formal analysis, Data curation. AY: Writing – review & editing, Formal analysis, Data curation.
Funding
The author(s) declare financial support was received for the research, authorship, and/or publication of this article. This work was supported by the Xinyang Academy of Ecological Research Open Foundation (2023XYMS06).
Acknowledgments
The authors are very grateful to Haoming Xia for providing all resources and facilities during the research.
Conflict of interest
The authors declare that the research was conducted in the absence of any commercial or financial relationships that could be construed as a potential conflict of interest.
Publisher’s note
All claims expressed in this article are solely those of the authors and do not necessarily represent those of their affiliated organizations, or those of the publisher, the editors and the reviewers. Any product that may be evaluated in this article, or claim that may be made by its manufacturer, is not guaranteed or endorsed by the publisher.
Abbreviations
BAFs, Bioaccumulation factors; BWAF, Biota-water accumulation factor; BSAF, Biota-sediment accumulation factor; BDL, Below the detection limit; BOD, Biological oxygen demand; COD, Chemical oxygen demand; CTEs, Concentration of trace elements; CIF, Control impact factor; ETAAS, Electrothermal atomic absorption spectrometry; FIAS, Flow injection appraisal system; HF, High flow; LOD, Limit of detection; LIR, Lower Indus River; PTFE, Polytetrafluoroethylene tubes; RB, Right bank; THQ, Target hazard quotient; TTHQ, Total THQ; WHO, World Health Organization; As, Arsenic; Ag, Silver; Cd, Cadmium; Cr, Chromium; Cu, Copper; Ni, Nickel; Pb, Lead; Hg, Mercury; Zn, Zinc; D/S, Downstream.
References
Ali K. F., De Boer D. H. (2007). Spatial patterns and variation of suspended sediment yield in the upper Indus River basin, northern Pakistan. J. Hydrol. 334, 368–387. doi: 10.1016/j.jhydrol.2006.10.013
An Y.-J., Kampbell D. H. (2003). Total, dissolved, and bioavailable metals at Lake Texoma marinas. Environ. pollut. 122, 253–259. doi: 10.1016/S0269-7491(02)00291-9
Ankit Y., Muneer W., Lahajnar N., Gaye B., Misra S., Jehangir A., et al (2021). Long term natural and anthropogenic forcing on aquatic system-evidence based on biogeochemical and pollen proxies from lake sediments in Kashmir Himalaya. Applied Geochemistry 131, 105046. doi: 10.1016/j.jhydrol.2006.10.013
Arain M. B., Kazi T. G., Jamali M. K., Jalbani N., Afridi H. I., Shah A. (2008). Total dissolved and bioavailable elements in water and sediment samples and their accumulation in Oreochromis mossambicus of polluted Manchar Lake. Chemosphere 70, 1845–1856. doi: 10.1016/j.chemosphere.2007.08.005
Association A. P. H. (1995). Standard Methods for the Examination of Water and Wastewater, twentieth ed (Washington, DC: American Public Health Association).
Avigliano E., Monferrán M. V., Sánchez S., Wunderlin D. A., Gastaminza J., Volpedo A. V. (2019). Distribution and bioaccumulation of 12 trace elements in water, sediment and tissues of the main fishery from different environments of the La Plata basin (South America): Risk assessment for human consumption. Chemosphere 236, 124394. doi: 10.1016/j.chemosphere.2019.124394
Azizullah A., Taimur N., Khan S., Häder D.-P. (2021). Heavy metals pollution in surface waters of Pakistan. Anthropog. pollut. Aquat. Ecosyst., 271–312. doi: 10.1007/978-3-030-75602-4_13
Barone G., Storelli A., Garofalo R., Busco V., Quaglia N. C., Centrone G., et al. (2015). Assessment of mercury and cadmium via seafood consumption in Italy: estimated dietary intake (EWI) and target hazard quotient (THQ). Food Addit. Contam. Part A. 32, 1277–1286. doi: 10.1080/19440049.2015.1055594
Bazrafshan E., Mostafapour F. K., Esmaelnejad M., Ebrahimzadeh G. R., Mahvi A. H. (2016). Concentration of heavy metals in surface water and sediments of Chah Nimeh water reservoir in Sistan and Baluchestan province, Iran. Desalin. Water Treat. 57, 9332–9342. doi: 10.1080/19443994.2015.1027958
Bhat S. U., Bhat A. A., Jehangir A., Hamid A., Sabha I., Qayoom U. (2021). Water quality characterization of Marusudar River in Chenab Sub-Basin of North-Western Himalaya using multivariate statistical methods. Water. Air. Soil pollut. 232, 1–22. doi: 10.1007/s11270-021-05394-8
Boota M. W., Xia H., Qin Y., Kakakhel M. A., Yan C., Weiran L., et al. (2024). Distribution and bioaccumulation of trace elements in two Cyprinidae fish species in the Indus river, Pakistan, including the impact of hydraulic structure on macroinvertebrates’ biodiversity. Environ. Res. 252, 118882. doi: 10.1016/j.envres.2024.118882
Boota M. W., Yan C., Idrees M. B., Li Z., Soomro S., Dou M., et al. (2021). Assessment of the morphological trends and sediment dynamics in the Indus River, Pakistan. J. Water Clim. Chang. 12, 3082–3098. doi: 10.2166/wcc.2021.125
Boota M. W., Yan C., Soomro S., Li Z., Zohaib M., Ijaz M. W., et al. (2022). Appraisal of hydro-ecology, geomorphology, and sediment behavior during low and high floods in the Lower Indus River Estuary. J. Water Clim. Change 13, 889–907. doi: 10.2166/wcc.2022.367
Cao X., Lu Y., Wang C., Zhang M., Yuan J., Zhang A., et al. (2019). Hydrogeochemistry and quality of surface water and groundwater in the drinking water source area of an urbanizing region. Ecotoxicol. Environ. Saf. 186, 109628. doi: 10.1016/j.ecoenv.2019.109628
Chatha A. M. M., Naz S., Mansouri B., Nawaz A. (2023). Accumulation and human health risk assessment of trace elements in two fish species, Cirrhinus mrigala and Oreochromis niloticus, at Tarukri Drain, District Rahimyar Khan, Punjab, Pakistan. Environ. Sci. pollut. Res. 30, 56522–56533. doi: 10.1007/s11356-023-26337-2
El-Kady A. A., Abdel-Wahhab M. A. (2018). Occurrence of trace metals in foodstuffs and their health impact. Trends Food Sci. Technol. 75, 36–45. doi: 10.1111/oik.07476
Eqani S.A.-M.-A.-S., Malik R. N., Cincinelli A., Zhang G., Mohammad A., Qadir A., et al. (2013). Uptake of organochlorine pesticides (OCPs) and polychlorinated biphenyls (PCBs) by river water fish: the case of River Chenab. Sci. Total. Environ. 450, 83–91. doi: 10.1016/j.scitotenv.2013.01.052
Haider H., Ali W. (2013). Evaluation of water quality management alternatives to control dissolved oxygen and un-ionized ammonia for Ravi River in Pakistan. Environ. Model. Assess. 18, 451–469. doi: 10.1007/s10666-012-9353-z
He Z., Li F., Dominech S., Wen X., Yang S. (2019). Heavy metals of surface sediments in the Changjiang (Yangtze River) Estuary: distribution, speciation and environmental risks. J. Geochem. Explor. 198, 18–28. doi: 10.1016/j.gexplo.2018.12.015
Ijaz M. W., Mahar R. B., Ansari K., Siyal A. A., Anjum M. N. (2020). Integrated assessment of contemporary hydro-geomorphologic evolution of the Indus River Estuary, Pakistan in context to regulated fluvial regimes. Estuar. Coast. Shelf. Sci. 236, 106657. doi: 10.1016/j.ecss.2020.106657
fJia Y., Wang L., Cao J., Li S., Yang Z. (2018). Trace elements in four freshwater fish from a mine-impacted river: spatial distribution, species-specific accumulation, and risk assessment. Environ. Sci. pollut. Res. 25, 8861–8870. doi: 10.1007/s11356-018-1207-z
Kassaye Y. A., Skipperud L., Einset J., Salbu B. (2016). Aquatic macrophytes in Ethiopian Rift Valley lakes; their trace elements concentration and use as pollution indicators. Aquat. Bot. 134, 18–25. doi: 10.1016/j.aquabot.2016.06.004
Kumar L., Kumari R., Kumar A., Tunio I. A., Sassanelli C. (2023). Water quality assessment and monitoring in Pakistan: A comprehensive review. Sustainability 15, 6246. doi: 10.3390/su15076246
La Colla N. S., Botté S. E., Simonetti P., Negrin V. L., Serra A. V., Marcovecchio J. E. (2021). Water, sediments and fishes: First multi compartment assessment of metal pollution in a coastal environment from the SW Atlantic. Chemosphere 282, 131131. doi: 10.1016/j.chemosphere.2021.131131
Lino A. S., Galvão P. M. A., Longo R. T. L., Azevedo-Silva C. E., Dorneles P. R., Torres J. P. M., et al. (2016). Metal bioaccumulation in consumed marine bivalves in Southeast Brazilian coast. J. Trace Elem. Med. Biol. 34, 50–55. doi: 10.1016/j.jtemb.2015.12.004
Lino A. S., Kasper D., Guida Y. S., Thomaz J. R., Malm O. (2019). Total and methyl mercury distribution in water, sediment, plankton and fish along the Tapajós River basin in the Brazilian Amazon. Chemosphere 235, 690–700. doi: 10.1016/j.chemosphere.2019.06.212
Maurya P. K., Malik D. S. (2018). Bioaccumulation of heavy metals in tissues of selected fish species from Ganga river, India, and risk assessment for human health. Hum. Ecol. Risk Assess. Int. J. 25, 905–923. doi: 10.1080/10807039.2018.1456897
Maurya P. K., Malik D. S., Yadav K. K., Kumar A., Kumar S., Kamyab H. (2019). Bioaccumulation and potential sources of heavy metal contamination in fish species in River Ganga basin: Possible human health risks evaluation. Toxicol. Rep. 6, 472–481. doi: 10.1016/j.toxrep.2019.05.012
Mazej Z., Germ M. (2009). Trace element accumulation and distribution in four aquatic macrophytes. Chemosphere 74, 642–647. doi: 10.1016/j.chemosphere.2008.10.019
Nawab J., Khan S., Xiaoping W. (2018). Ecological and health risk assessment of potentially toxic elements in the major rivers of Pakistan: General population vs. Fishermen. Chemosphere. 202, 154–164. doi: 10.1016/j.chemosphere.2018.03.082
Noor R., Maqsood A., Baig A., Pande C. B., Zahra S. M., Saad A., et al. (2023). A comprehensive review on water pollution, South Asia Region: Pakistan. Urban. Clim. 48, 101413. doi: 10.1016/j.uclim.2023.101413
Paul V., Sankar M. S., Vattikuti S., Dash P., Arslan Z. (2021). Pollution assessment and land use land cover influence on trace metal distribution in sediments from five aquatic systems in southern USA. Chemosphere 263, 128243. doi: 10.1016/j.chemosphere.2020.128243
Puche E., Jordán F., Rodrigo M. A., Rojo C. (2020). Non-trophic key players in aquatic ecosystems: a mesocosm experiment. Oikos 129, 1714–1726. doi: 10.1111/oik.07476
Raman R. K., Talukder A., Mahanty A., Sarkar D. J., Das B. K., Bhowmick S., et al. (2022). Arsenic bioaccumulation and identification of low-arsenic-accumulating food fishes for aquaculture in arsenic-contaminated ponds and associated aquatic ecosystems. Biol. Trace Elem. Res. 200, 2923–2936. doi: 10.1007/s12011-021-02858-0
Rani S., Ahmed M. K., Xiongzhi X., Keliang C., Islam M. S., Habibullah-Al-Mamun M. (2021). Occurrence, spatial distribution and ecological risk assessment of trace elements in surface sediments of rivers and coastal areas of the East Coast of Bangladesh, North-East Bay of Bengal. Sci. Total. Environ. 801, 149782. doi: 10.1016/j.scitotenv.2021.149782
Rice E. W., Bridgewater L., Association A. P. H. (2012). Standard methods for the examination of water and wastewater (Washington, DC: American public health association).
Sanyal T., Kaviraj A., Saha S. (2015). Deposition of chromium in aquatic ecosystem from effluents of handloom textile industries in Ranaghat–Fulia region of West Bengal, India. J. Adv. Res. 6, 995–1002. doi: 10.1016/j.jare.2014.12.002
Sarker S., Akbor M. A., Nahar A., Hasan M., Islam A. R. M. T., Siddique M. A. B. (2021). Level of pesticides contamination in the major river systems: A review on South Asian countries perspective. Heliyon 7. doi: 10.1016/j.heliyon.2021.e07270
Sedak M., Bilandžić N., Đokić M., Đuras M., Gomerčić T., Benić M. (2022). Body burdens and distribution of mercury and selenium in bottlenose, striped and Risso’s dolphins along the Adriatic coast: A 20-year retrospective. Mar. pollut. Bull. 185, 114298. doi: 10.1016/j.marpolbul.2022.114298
Shannon C. E. (1948). A mathematical theory of communication. Bell. Syst. Tech. J. 27, 379–423. doi: 10.1002/j.1538-7305.1948.tb01338.x
Shi X., Guo J., Jalbani S., Asad M., Anwar M. I., Hu C., et al. (2024). Effects of seasonal temperature regimes: Does Cyprinus carpio act as a health hazard during the construction of Suki Kinari hydropower project on Kunhar River in Pakistan? Sci. Total. Environ. 907, 168023. doi: 10.1016/j.scitotenv.2023.168023
Stankovic S., Kalaba P., Stankovic A. R. (2014). Biota as toxic metal indicators. Environ. Chem. Lett. 12, 63–84. doi: 10.1007/s10311-013-0430-6
Tabezar N., Sadeghi P., Attaran Fariman G. (2023). Monsoon effect on heavy metal and chemical composition in parastromateus Niger of the Oman Sea: health risk assessment of fish consumption. Biol. Trace Elem. Res. 201, 4093–4102. doi: 10.1007/s12011-022-03475-1
Thilakarathne E., Ramawickrama N. W., Bandara T., Maldeniya M. U. S., Egodauyana K., Sewwandi S. W. R., et al. (2024). Abundance and diversity of reef fish species and their relationship with corals along the eastern coast of Sri Lanka. J. Coast. Conserv. 28, 1–10. doi: 10.1007/s11852-024-01040-7
Ullah M. R., Hasan M. M., Alam M. A., Neela J. N., Islam M., Moniruzzaman M., et al. (2023). Diversity of fish species in relation to climatological fluctuations in a coastal river of Bangladesh. J. Mar. Sci. 2023, 6662387. doi: 10.1155/2023/6662387
Van der Oost R., Beyer J., Vermeulen N. P. E. (2003). Fish bioaccumulation and biomarkers in environmental risk assessment: a review. Environ. Toxicol. Pharmacol. 13, 57–149. doi: 10.1016/S1382-6689(02)00126-6
Varol M., Sünbül M. R. (2018). Multiple approaches to assess human health risks from carcinogenic and non-carcinogenic metals via consumption of five fish species from a large reservoir in Turkey. Sci. Total. Environ. 633, 684–694. doi: 10.1016/j.scitotenv.2018.03.218
Washington H. G. (1984). Diversity, biotic and similarity indices: a review with special relevance to aquatic ecosystems. Water Res. 18, 653–694. doi: 10.1016/0043-1354(84)90164-7
World Health Organization. (2002). The world health report 2002: reducing risks, promoting healthy life. (World Health Organization).
Yi Y., Yang Z., Zhang S. (2011). Ecological risk assessment of heavy metals in sediment and human health risk assessment of heavy metals in fishes in the middle and lower reaches of the Yangtze River basin. Environ. pollut. 159, 2575–2585. doi: 10.1016/j.envpol.2011.06.011
Keywords: trace element concentration, Cirrhinus mrigala, bioavailability, health risk assessment, Lower Indus River
Citation: Boota MW, Soomro S-e-h, Xia H, Qin Y, Idrees MB and Yousaf A (2024) Unveiling concentrations of trace elements in the Lower Indus River: risks to aquatic life and human health. Front. Mar. Sci. 11:1449589. doi: 10.3389/fmars.2024.1449589
Received: 15 June 2024; Accepted: 24 September 2024;
Published: 17 December 2024.
Edited by:
Ana Maulvault, Portuguese Institute for Sea and Atmosphere (IPMA), PortugalReviewed by:
Marta Andreia Dias, NOVA University of Lisbon, PortugalMeng Chuan Ong, University of Malaysia Terengganu, Malaysia
Copyright © 2024 Boota, Soomro, Xia, Qin, Idrees and Yousaf. This is an open-access article distributed under the terms of the Creative Commons Attribution License (CC BY). The use, distribution or reproduction in other forums is permitted, provided the original author(s) and the copyright owner(s) are credited and that the original publication in this journal is cited, in accordance with accepted academic practice. No use, distribution or reproduction is permitted which does not comply with these terms.
*Correspondence: Haoming Xia, eGlhaG1AdmlwLmhlbnUuZWR1LmNu; Yaochen Qin, cWlueWNAaGVudS5lZHUuY24=