- College of Life Science and Engineering, Southwest University of Science and Technology, Mianyang, Sichuan, China
Introduction: In recent years, cadmium pollution has increasingly serious impacts on aquatic environments, directly threatening the health and growth of freshwater fish and causing significant economic losses to the aquaculture industry. However, there is limited research on the effects of cadmium on the gut-liver axis and hepatotoxicity in freshwater fish. Therefore, this study investigated the potential toxic effects of cadmium induction through the gut-liver axis on largemouth bass.
Methods: This experiment was divided into four groups, each with different concentrations of cadmium solution added to the water (0.00 mg/L, 1.024 mg/L, 1.537 mg/L, 2.306 mg/L), with three replicates per group, and a feeding period of 42 days.
Results and discussion: The research findings indicate a significant decline in the growth performance of largemouth bass under cadmium stress (P<0.05). Cadmium-induced oxidative stress inhibited the activity of antioxidant enzymes, activated the Nrf2-Keap1 antioxidant pathway, resulting in increased levels of MDA and ROS, and decreased activities of CAT, GSH-PX, and SOD antioxidant enzymes, as well as related gene expressions (P<0.05). Additionally, cadmium down-regulated the expression of IL-10 and up-regulated the expression of IL-15, IL-8, IL-1β, TNF-α, and MT, indicating an inflammatory response in the liver (P<0.05). Tissue section observations after cadmium stress revealed hepatocyte nuclear condensation, cell degeneration, necrosis, and vacuolization, as well as shortened intestinal villi and intestinal epithelial cell metaplasia. Furthermore, cadmium down-regulated the expression of intestinal barrier-related proteins ZO-1 and Occludin (P<0.05), reducing intestinal microbial diversity. Correlation analysis revealed a close relationship between intestinal microbiota and hepatic immune factors. In summary, cadmium stress can disrupt the intestinal barrier, alter the structure of intestinal microbiota, and the gut-liver axis may potentially play a role in the toxicity of intestinal microbiota and liver.
1 Introduction
Largemouth bass, originating from North America and introduced to China in 1983, has become one of the important freshwater economic fishes (Junjie and Shengjie, 2019; Andersen et al., 2021). Largemouth bass is a carnivorous fish characterized by a fast growth rate, tasty meat, and rich nutrition (He et al., 2022; Hou et al., 2023). However, with the rapid development of industries, wastewater generated directly or indirectly from anthropogenic, industrial, and agricultural activities can flow into the surrounding waters, resulting in cadmium ions in the water exceeding safety standards (Khan et al., 2023). Cadmium ion content has exceeded 1 mg/L in some areas, and cadmium pollution has become a major environmental problem (Qi et al., 2023; Zhang et al., 2023). Cadmium ions in water pose a serious threat to the healthy farming of freshwater fish (Vinanthi Rajalakshmi et al., 2023).
In recent years, numerous scholars have delved into the toxic effects of cadmium on the liver and intestinal tract of aquatic animals. The liver emerges as the primary target organ for cadmium toxicity, inducing tissue damage primarily through mechanisms involving inflammation and oxidative stress (Cui et al., 2021). The liver effectively utilizes the active sites of antioxidant enzymes [such as catalase (CAT), glutathione peroxidase (GSH-PX), and superoxide dismutase (SOD)] to displace divalent cations, thereby disrupting cellular redox homeostasis and causing an imbalance in ROS generation (Li et al., 2023). Within hepatocytes, cadmium forms complexes with small peptides and proteins, including glutathione (GSH) or metallothionein (MT), via sulfhydryl groups (Dai et al., 2020). Cadmium largely instigates pathological changes in liver tissues, such as the loss of the normal structure of parenchymal tissues, cytoplasmic vacuolization, cellular degeneration, and vascular congestion (Baş et al., 2021). Research has demonstrated that cadmium induces cellular inflammation in animals and modulates the expression levels of pro-inflammatory and anti-inflammatory factors (Sivaprakasam and Nachiappan, 2016). Meanwhile, cadmium can harm intestinal tissues, increase intestinal permeability, and inhibit the expression of intestinal epithelial tight junction proteins like Occludin and ZO-1 (Meng et al., 2018; He et al., 2020). Heavy metals may impact the intestinal flora, reducing its diversity and leading to an imbalance in the intestinal microbiota (Liu et al., 2023). Furthermore, the disruption of homeostasis in the intestinal environment further facilitates the entry of inflammatory factors into the liver via the gut–liver circulation through the portal vein, resulting in liver damage (Chen et al., 2021). However, research in this area is still nascent in aquatic animals, and whether heavy metals affect the intestinal flora of fish and what their adverse effects are on the organism remain largely unknown, necessitating further study.
The aim of this study is to explore the mechanisms underlying cadmium-induced intestinal and hepatic damage in largemouth bass, as well as its impact on the intestinal microbiota, to better understand the potential mechanisms of cadmium-induced liver and intestinal toxicity in largemouth bass and to provide necessary theoretical foundations.
2 Materials and methods
2.1 Fish and chemicals
The fish were purchased from Meishan, Sichuan, China, with an average weight of approximately 8 g. Prior to the commencement of the experiment, the fish underwent a 2-week acclimatization period during which they were fed twice daily at 9:00 a.m. and 5:00 p.m., with each feeding not exceeding 3%–5% of their body weight. The chemical used in the experiment was CdCl2×2.5H2O, sourced from Chengdu Cologne Chemical Company Limited, China. CdCl2×2.5H2O was dissolved in distilled water to prepare a stock solution, which was further diluted into working solutions of various concentrations by adding distilled water.
2.2 Experimental design and treatment
In the pre-experimental phase, the 96-h median lethal concentration (LC50) of CdCl2×2.5H2O for largemouth bass was determined to be 23.057 mg/L. Largemouth bass were subjected to a 24-h fasting period prior to the formal experiment. A total of 360 uniformly sized fish were randomly allocated into 12 tanks, with each tank containing 30 fish. For the formal experiment, concentrations were based on a 1.5-fold isocratic gradient relative to the 96-h LC50, with the highest concentration being set at 1/10 of the LC50. Four treatment groups were established: control (0.00 mg/L), Cd1 (1.024 mg/L), Cd2 (1.537 mg/L), and Cd3 (2.306 mg/L), each with three replicates. The experimental duration spanned 42 days, during which fish were fed twice daily at 9:00 a.m. and 5:00 p.m., ensuring no residual feed remained in the water after feeding. Water parameters were maintained within optimal ranges, with a temperature of 26°C ± 0.5°C, dissolved oxygen levels exceeding 5 mg/L, and pH maintained at 7.0 ± 0.5.
2.3 Sample collection
At the end of the experiment, fasting was performed for 24 h, followed by anesthesia using 130 mg/L of MS-222 (Topic Popovic et al., 2012). The weight and length of the fish were determined using electronic scales and vernier calipers and weighed after dissection, and the weights of the viscera and liver were recorded. The liver and intestines of 10 fish were collected and preserved in 4% paraformaldehyde for the preparation of tissue sections, while the remaining samples were preserved at −80°C.
2.4 Determination of growth performance
The growth performance indicators relevant to this study were calculated using the following formula:
2.5 Determination of liver biochemical indices
2.5.1 Determination of antioxidant status
A 10% tissue homogenate (liver:saline = 1:9) was prepared and centrifuged for 10 min to obtain the supernatant for further use. The levels of SOD, CAT, GSH-PX, total antioxidant capacity (T-AOC), and malondialdehyde (MDA) were assessed using kits from Nanjing Jiancheng Biotechnology Research Institute, following the protocols provided with the respective kits.
2.5.2 Fluorescent probe detection
The determination of reactive oxygen species (ROS) requires the use of fresh tissue. After the suspension is prepared, it is centrifuged at 2,000 r/min for 5 min, and the cell precipitate is collected. The assay method refers to the instructions of the ROS assay kit from Nanjing Jianjian Bioengineering Research Institute, with product number E004-1-1. Meanwhile, a portion of the suspension is taken for protein determination, and the results are expressed as fluorescence intensity values per milligram of protein. Due to the addition of the DCFH-DA fluorescence probe in the samples, the operation process needs to be conducted in the dark.
2.5.3 Enzyme-linked immunosorbent assay
Using the ELISA assay kit (JI-ICHI, China) and employing the double antibody sandwich method enzyme-linked immunosorbent assay (ELISA), samples and different concentrations of standard solutions were added to corresponding wells. After incubation at 37°C for 60 min, the wells were thoroughly washed five times. Subsequently, the chromogenic substrate TMB was added. TMB is converted into a blue color in the presence of peroxidase and then into the final yellow color under acidic conditions. Finally, the absorbance values of each well were measured at 450 nm, and the concentration of HSP70 was calculated by plotting a standard curve.
2.6 Liver histologic analysis
The livers were sequentially dehydrated by anhydrous ethanol at concentrations of 75%, 85%, 95%, 100% I, and 100% II, transparent with xylene, and soaked in a 1:1 mixture of paraffin and xylene for paraffin embedding (frozen overnight at −20°C).
2.7 Real-time PCR analysis
The total RNA extraction from liver and intestinal tissues was conducted using RNAiso Plus reagent, following the extraction protocol provided by TaKaRa (Code No. 9109). The concentration and purity of the total RNA were assessed using a NanoDrop ND-2000 microspectrophotometer. First-strand cDNA synthesis was carried out using a reverse transcription reagent (TaKaRa), and the reverse transcription reaction was performed on a thermal cycler. The resulting product was stored at −20°C upon completion of the reaction. Real-time fluorescence quantitative PCR was employed to measure the relative expression of liver-related genes in largemouth bass. Relative expression levels were calculated using the 2−ΔΔCt method, normalized to β-actin. The primer sequences for fluorescence quantitative PCR are listed in Table 1.
2.8 Analysis of the intestinal microbiota
The library was subjected to double-end sequencing (paired-end) based on the Illumina NovaSeq sequencing platform. The amount of sequencing data is 50,000 tags. While the total data volume is satisfied, the data volume of each sample is not less than 90% of the target data volume. To study the species composition of each treatment group, the OTU clustering was performed based on the 97% sequence similarity principle.
2.9 Statistical analysis
The statistical analysis was conducted using SPSS 26.0 software, and the data results were presented as mean ± standard error of the mean (mean ± SEM). Prior to statistical analysis, normality and variance homogeneity of the data were assessed. One-way ANOVA and Tukey’s multiple comparisons were employed to evaluate differences among treatment groups, with significance set at P<0.05. Correlation analysis was performed using Spearman’s rank test, and visualization images were created by using GraphPad Prism 9.5.
3 Results
3.1 Growth performance
The effects of cadmium on the growth performance of largemouth bass are shown in Table 2. Compared with the control group, WGR, SGR, and SR were significantly decreased (P< 0.05). The SR of the Cd3 group was the lowest (P< 0.05). The HSI and VSI of the Cd groups increased with the increase in cadmium concentration, with the highest HSI and VSI in the Cd3 group (P< 0.05). Compared with the control group, FCR significantly increased in the Cd groups (P< 0.05).
3.2 Liver antioxidant capacity
The influence of cadmium on the antioxidant capacity of largemouth bass liver is presented in Table 3. With increasing cadmium concentration (P< 0.05), the activities of MDA and ROS in the Cd groups were significantly higher than those in the control group. Furthermore, compared to the control group, the activities of CAT, GSH-PX, and SOD were significantly lower, while there was no significant difference in SOD activity among the Cd groups (P > 0.05). The T-AOC activity of the Cd groups was notably lower than that of the control group, with the Cd3 group exhibiting the lowest activity among the Cd groups (Table 3). These findings suggest that cadmium reduces the activity of CAT, SOD, and GSH-PX while promoting an increase in the concentration of MDA and ROS in the liver of largemouth bass.
3.3 Effects of the Nrf2–Keap1 antioxidant pathway on the liver
The effect of cadmium on the Nrf2–Keap1 pathway in the liver is depicted in Figure 1. Relative to the control group, the expression levels of the GPX, SOD, and CAT genes were significantly downregulated in the Cd-exposed groups. Moreover, the expression levels of GPX and CAT genes exhibited a decrease with increasing Cd concentration, whereas the expression of the SOD gene showed no significant difference in the Cd groups. Overall, the activities of GPX, SOD, and CAT were markedly lower compared to those in the control group, indicating that cadmium can inhibit the activity of antioxidant enzymes (P< 0.05). Within the Nrf2–Keap1 antioxidant pathway, the expression level of Nrf2 decreased in the Cd groups, while the expression level of Keap1 increased (P< 0.05). These results suggest that the Nrf2–Keap1 antioxidant pathway may play a role in regulating the antioxidant response under cadmium stress, consistent with the trend observed in the antioxidant capacity index.
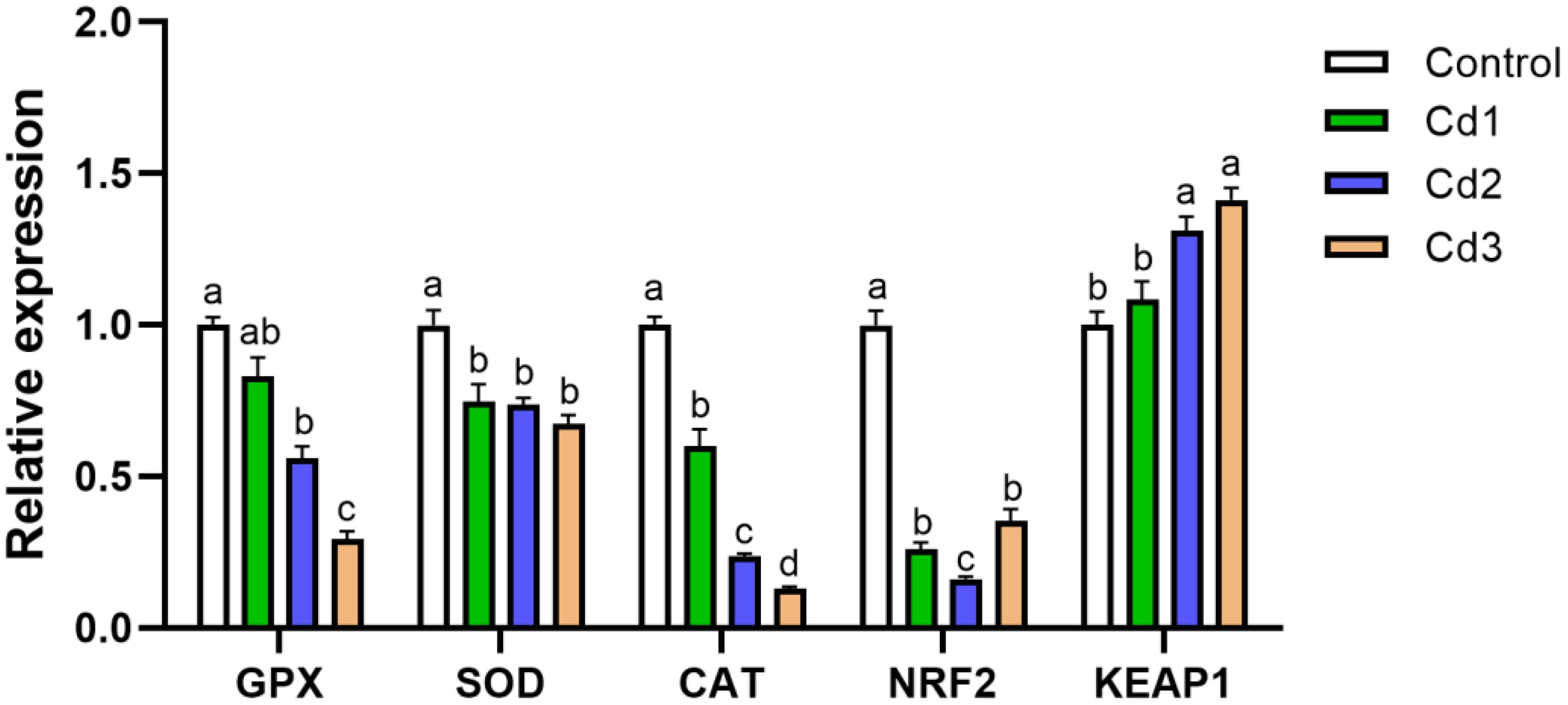
Figure 1. The impact of cadmium stress on liver Nrf2–Keap1 in largemouth bass. Lowercase letters with different numerical superscripts indicate statistically significant differences (P< 0.05), while the same letters indicate no significant differences (P > 0.05). Nrf2, nuclear factor erythroid 2-related factor; Keap1, kelch-like ECH-associated protein 1.
3.4 Histopathology
To examine the impact of cadmium on the liver and intestine of largemouth bass, tissue slices were stained with eosin. The liver cell structure of the control group (Figure 2A) remained intact, while that of the Cd groups exhibited lesions. Compared with the control group, liver cells in Cd1 (Figure 2B) showed hypertrophy, karyopyknotic, and cell degeneration. The nucleus was displaced toward the cell periphery, and the cytoplasm showed exfoliation and vacuolization; however, the overall cell structure remained relatively intact. Similar lesions were observed in both Cd2 (Figure 2C) and Cd3 (Figure 2D), but some liver cells in Cd2 and Cd3 were necrotic with an incomplete cell structure. Liver cell necrosis and cytoplasm shedding were more severe in Cd3 than in Cd2 (Figure 2). In comparison to the intestinal tract of the control group (Figure 2a), intestinal villi in the Cd groups appeared thicker and shorter, with an incomplete villi structure indicating varying degrees of damage, and intestinal metaplasia was especially evident in Cd3 (Figure 2d).
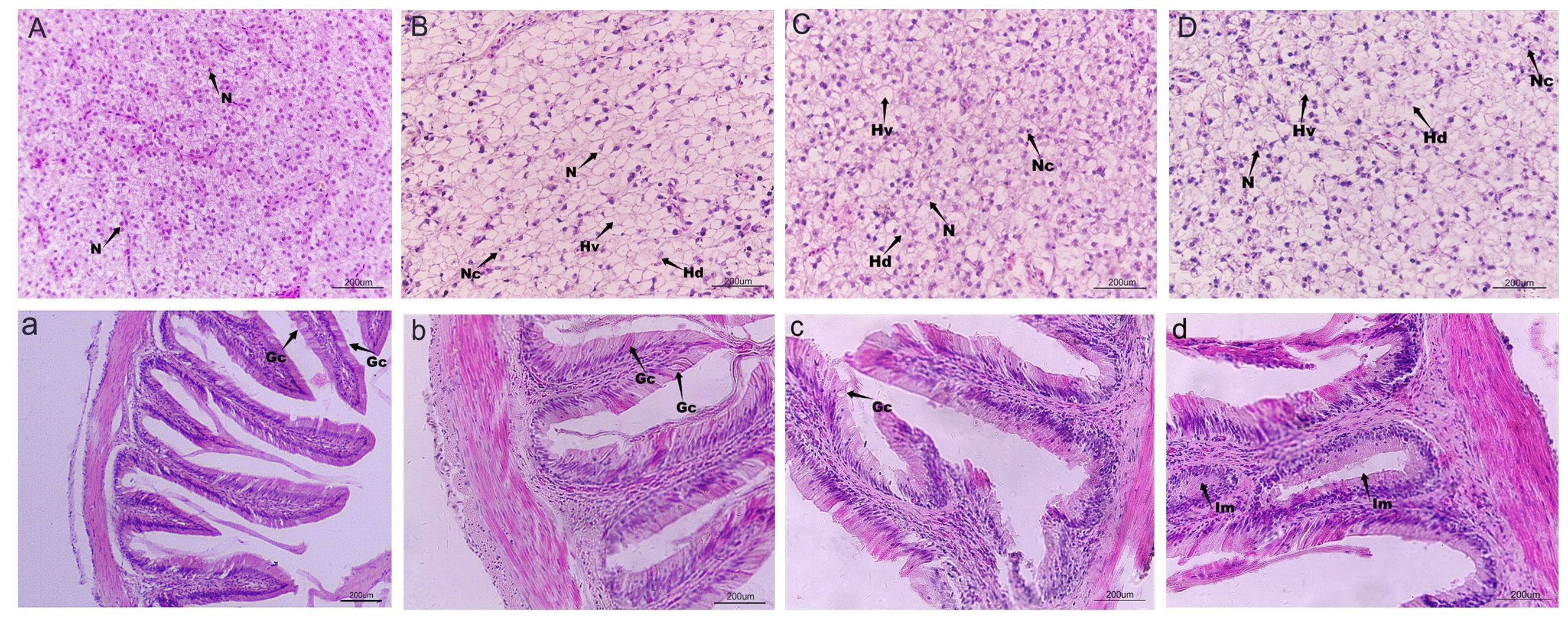
Figure 2. Effects of cadmium stress on the liver and intestinal injury of largemouth bass. HE staining, magnification of 400 times, except (a). (A, a) Control; (B, b) 1.024 mg/L; (C, c) 1.537mg/L; (D, d) 2.306 mg/L. N, nucleus; Hv, hepatocyte vacuolization; Hn, hepatocyte necrosis; Hd, hepatocyte degeneration; Nc, nuclei condensation; Im, intestinal metaplasia; Gc, Goblet cell.
3.5 Relative expression of hepatic inflammatory factors
Cadmium stress induced an inflammatory response in largemouth bass. As illustrated in Figure 3, the mRNA expression of the anti-inflammatory factor IL-10 significantly decreased compared to the control group (P< 0.05). Furthermore, the relative expression levels of pro-inflammatory factors IL-8, IL-15, IL-1β, TNF-α, and TGF-β1 in the Cd3 group were significantly higher than those in the control group (P< 0.05).
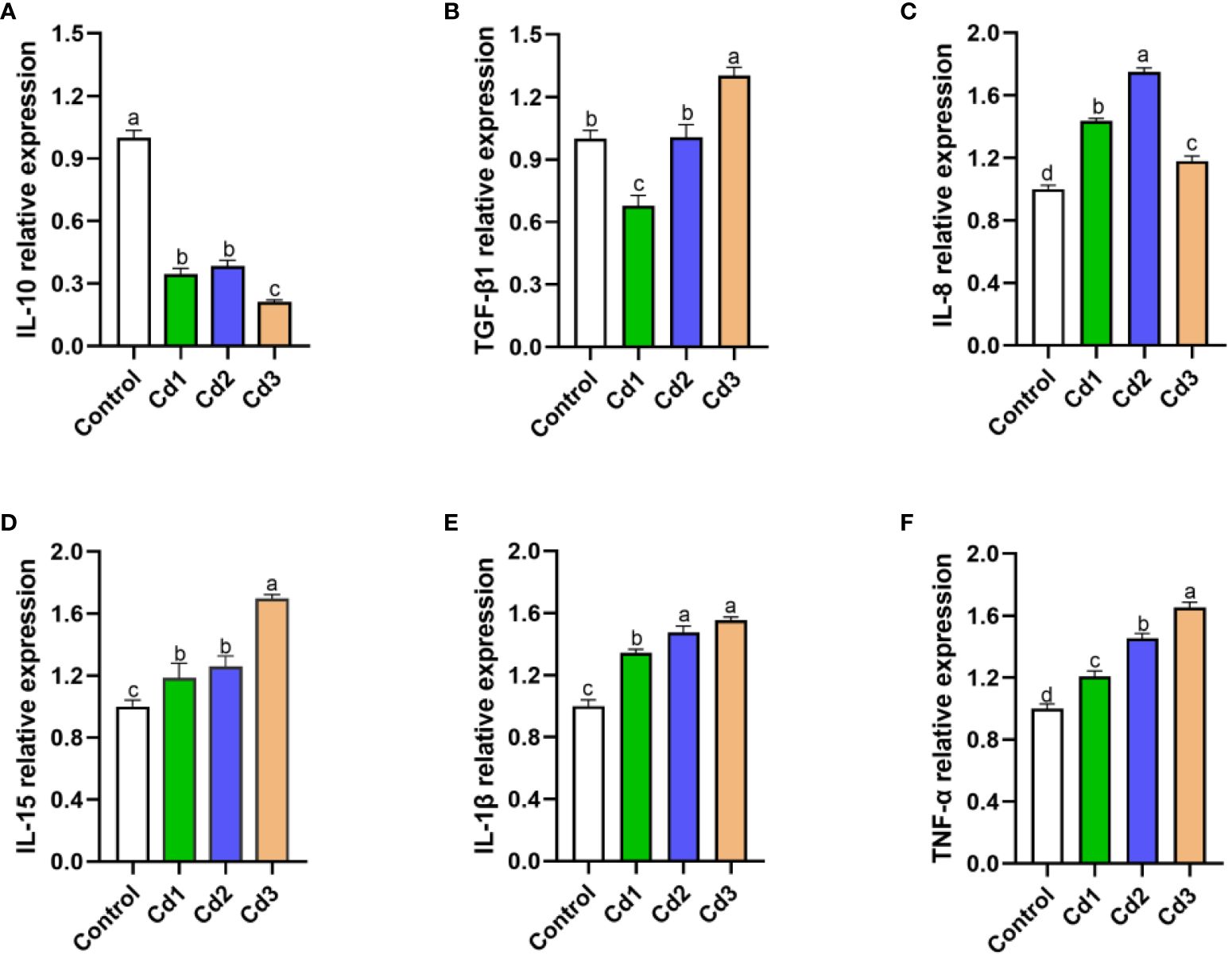
Figure 3. (A–F) Effects of cadmium stress on liver inflammatory factors of largemouth bass larvae. Lowercase letters with different numerical superscripts in peers indicate significant differences (P< 0.05), while the same letters indicate no significant differences (P > 0.05). IL-10, interleukin-10; TGF-β1, transforming growth factor-β: IL-8, interleukin-8; IL-15, interleukin-15; IL-1β, interleukin-1b; TNF-a, tumor necrosis factor-α.
3.6 Relative expression of MT and HSP70 genes in the liver
As displayed in Figure 4, the levels of MT and HSP70 in the liver significantly increased under cadmium stress compared to the control group (P< 0.05). Spearman’s correlation analysis was employed to confirm the relationship between MT and HSP70, revealing a bilateral correlation coefficient of 0.862. MT levels were found to increase with the rise of HSP70, indicating a significant positive correlation between the two factors.
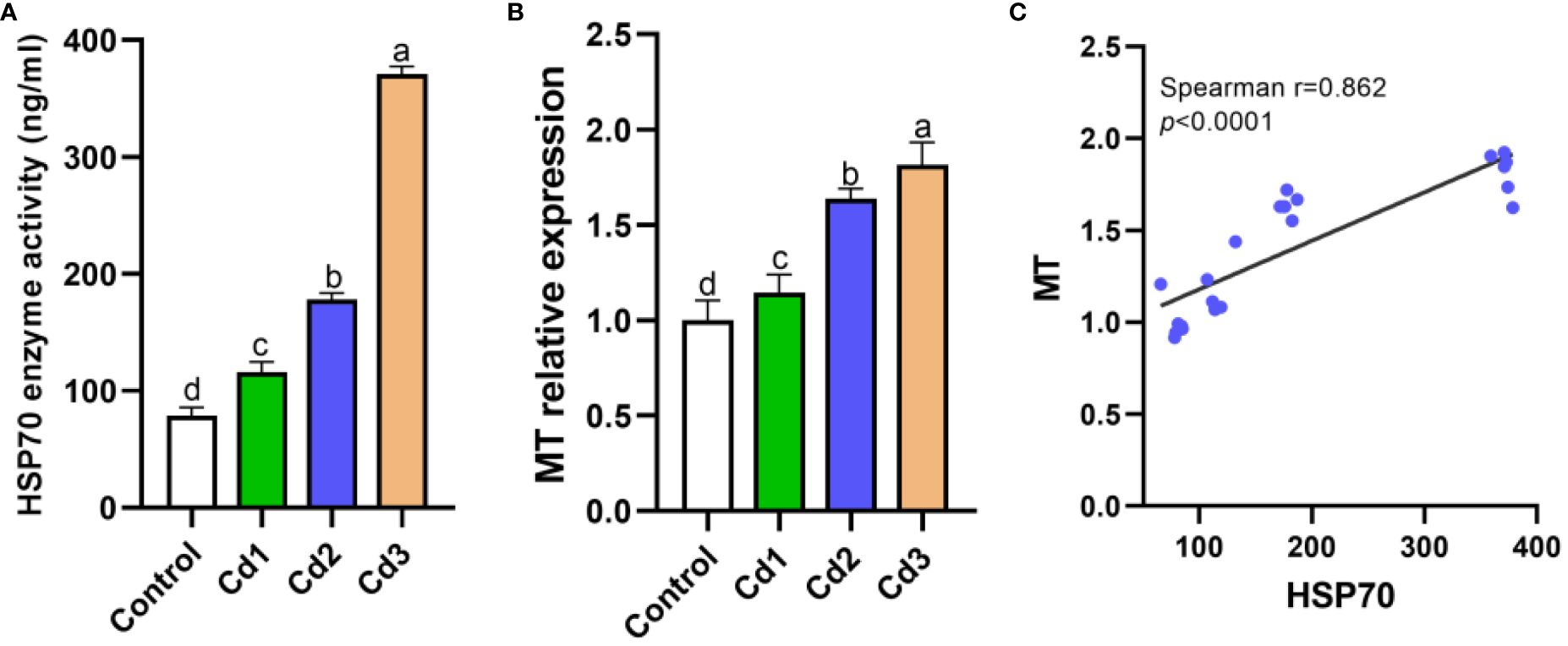
Figure 4. (A–C) Expression levels of MT and HSP70. Lowercase letters with different numerical superscripts in peers indicate significant differences (P< 0.05), while the same letters indicate no significant differences (P > 0.05). MT, metallothionein; HSP70, heat shock protein 70.
3.7 Intestinal barrier
As depicted in Figure 5, compared to the control group, the expression levels of Occludin and ZO-1 in the Cd groups were significantly decreased. Specifically, the expression of Occludin decreased with the increase of cadmium concentration, with the most severe damage observed in the Cd3 group. However, there was no significant difference in ZO-1 expression among the Cd groups (P< 0.05).
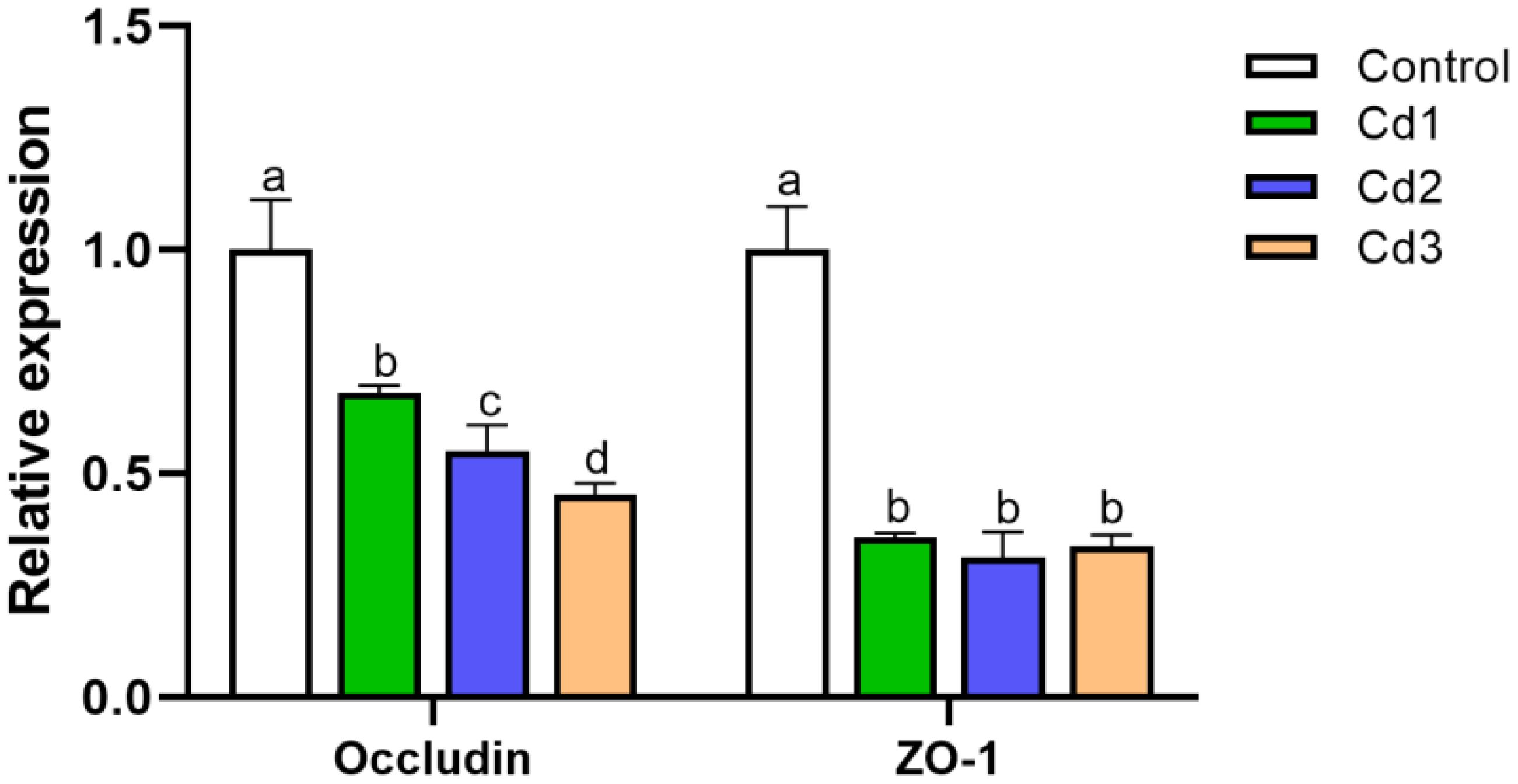
Figure 5. Effect of cadmium on intestinal barrier-related protein expression in largemouth bass. Lowercase letters with different numerical superscripts in peers indicate significant differences (P< 0.05), while the same letters indicate no significant differences (P > 0.05).
3.8 Gut microorganism
3.8.1 Analysis of intestinal microbial diversity
In the experiment, a total of 2,018 operational taxonomic units (OTUs) were identified across the four treatment groups. Among these, the control group had 20 unique OTUs, the Cd1 group had 85 unique OTUs, the Cd2 group had 18 unique OTUs, and the Cd3 group had 925 unique OTUs (Figure 6A). α-Diversity serves as an indicator of microbial community richness and diversity within each group. In this study, there was a significant difference in the Shannon index between the Cd2 and Cd3 groups in comparison to the control group. However, no significant differences were observed in the ACE and Chao1 indices among the control group and the Cd groups (Figures 6B–E). β-Diversity reflects variations in microbial communities among different groups. The principal component analysis (PCA) results revealed that the interpretation of the PCA1 axis was 43.88%, while the PCA2 axis accounted for 28.66% (Figure 6F). Compared with the control group, noticeable alterations were observed in microbial flora composition within the Cd groups, with the highest biodiversity seen in the Cd2 group.
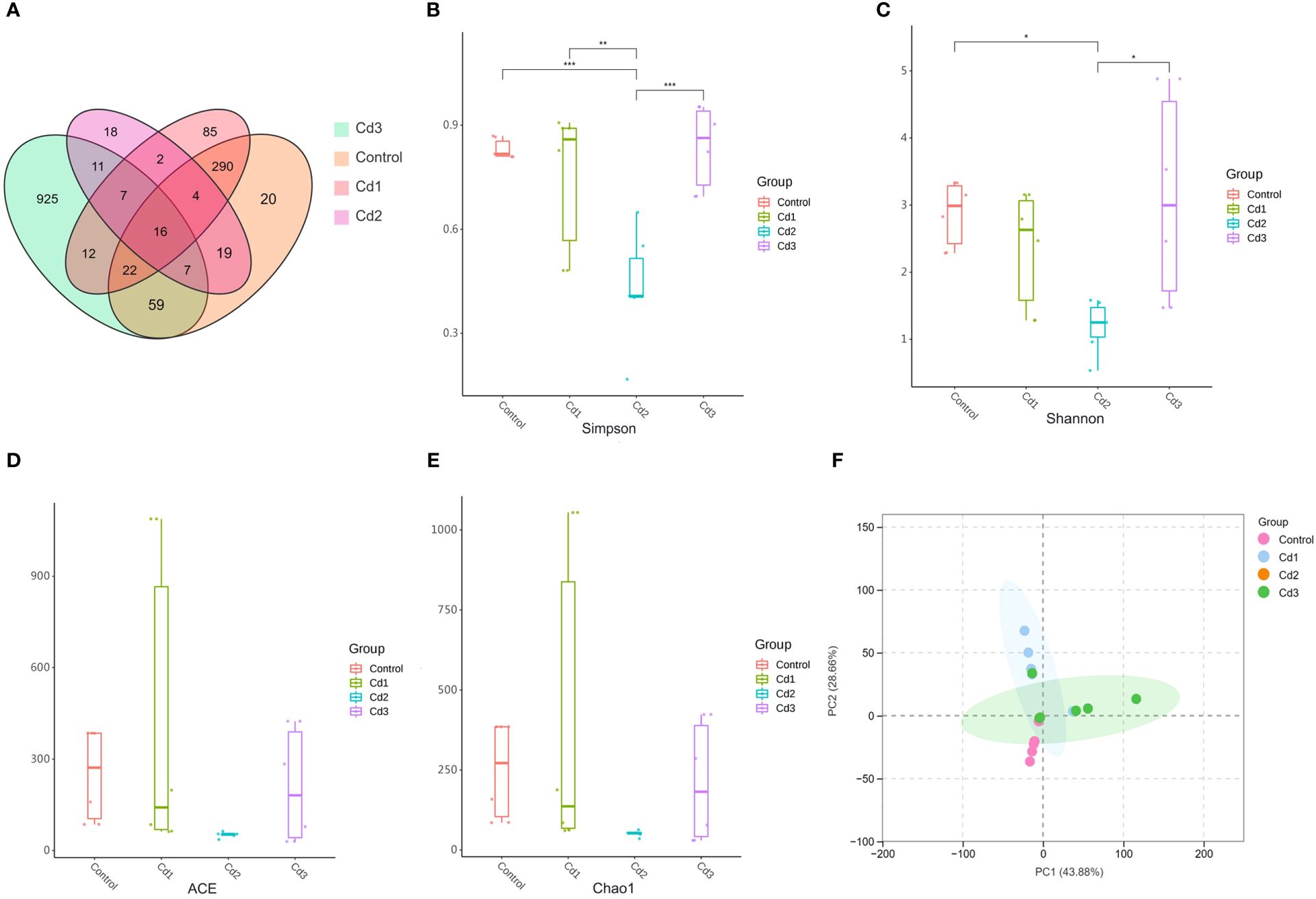
Figure 6. (A-F) Effects of cadmium on intestinal microbial diversity (Venn diagram), a-diversity, and b-diversity of largemouth bass. the symbol * shows significant differences among the groups (*P< 0.05, **P< 0.01, ***P< 0.001).
3.8.2 Analysis of intestinal microbial structure at different levels
Proteobacteria and Firmicutes are the two most predominant phyla, followed by Fusobacteria and Bacteroidetes. The relative richness of Proteobacteria increased compared to the control group but decreased with increasing cadmium concentration. Additionally, the relative richness of Fusobacteria in the Cd3 group also decreased (Figure 7A). At the genus level, Rolstonia, Acetobacter, and Mycoplasma were the three genera with the highest proportion in the control group. In contrast, Acetobacter, Paromonas, and Pseudomonas were dominant in the Cd1 group, while Rolstonia, Paromonas, and Mycoplasma dominated in the Cd2 group. The Cd3 group consisted of Rolstonia, Mycoplasma, and Oligotrophomonas (Figure 7B).
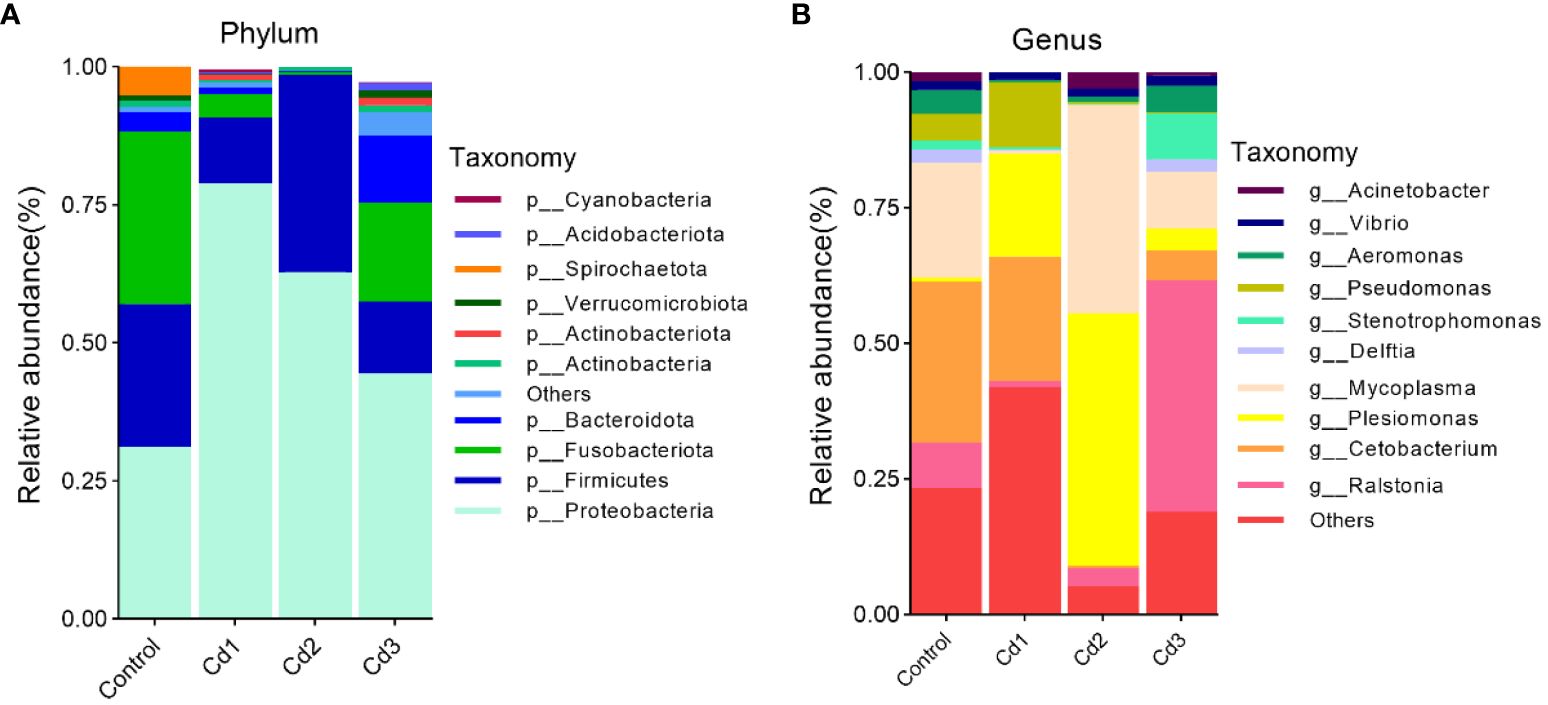
Figure 7. Characteristics of microbial composition in the gut microflora of largemouth bass and identification of different species between groups. The top 10 abundant bacterial community composition and intergroup species differences at the phylum level (A) and genus level (B), respectively.
3.8.3 Analysis of differences in microbial communities between groups
At the phylum level, the richness of cyanobacteria in the Cd1 group was significantly higher than that in the Cd2 group, while the richness of Fusobacteria and Bacteroidetes in the Cd3 group was also significantly higher than that in the Cd2 group. Additionally, the richness of Fusobacteria, Bacteroidetes, and cyanobacteria in the control group was significantly higher than that in the Cd2 group, whereas the richness of Proteobacteria was significantly lower than that in the Cd2 group (P< 0.01) (Figure 8A). At the genus level, Acetobacter and Pseudomonas in the Cd1 group were larger than those in the Cd2 group, but Aeromonas, Rolstonia, Acinetobacter, and Mycoplasma in the Cd1 group were smaller than those in the Cd2 group. The richness of Pseudomonas in the Cd1 group was higher than that in the control group, and those in Acinetobacter, Delfordia, Aeromonas, Oligotrophomonas, Mycoplasma, and Rolstonia were all lower than those in the Cd3 group. Acinetobacter, Delfordia, Aeromonas, Rolstonia, and Mycoplasma in the control group were all larger than those in the Cd1 group. (Figure 8B) In general, cadmium can reduce the richness of gut microbes and lead to microbial imbalance.
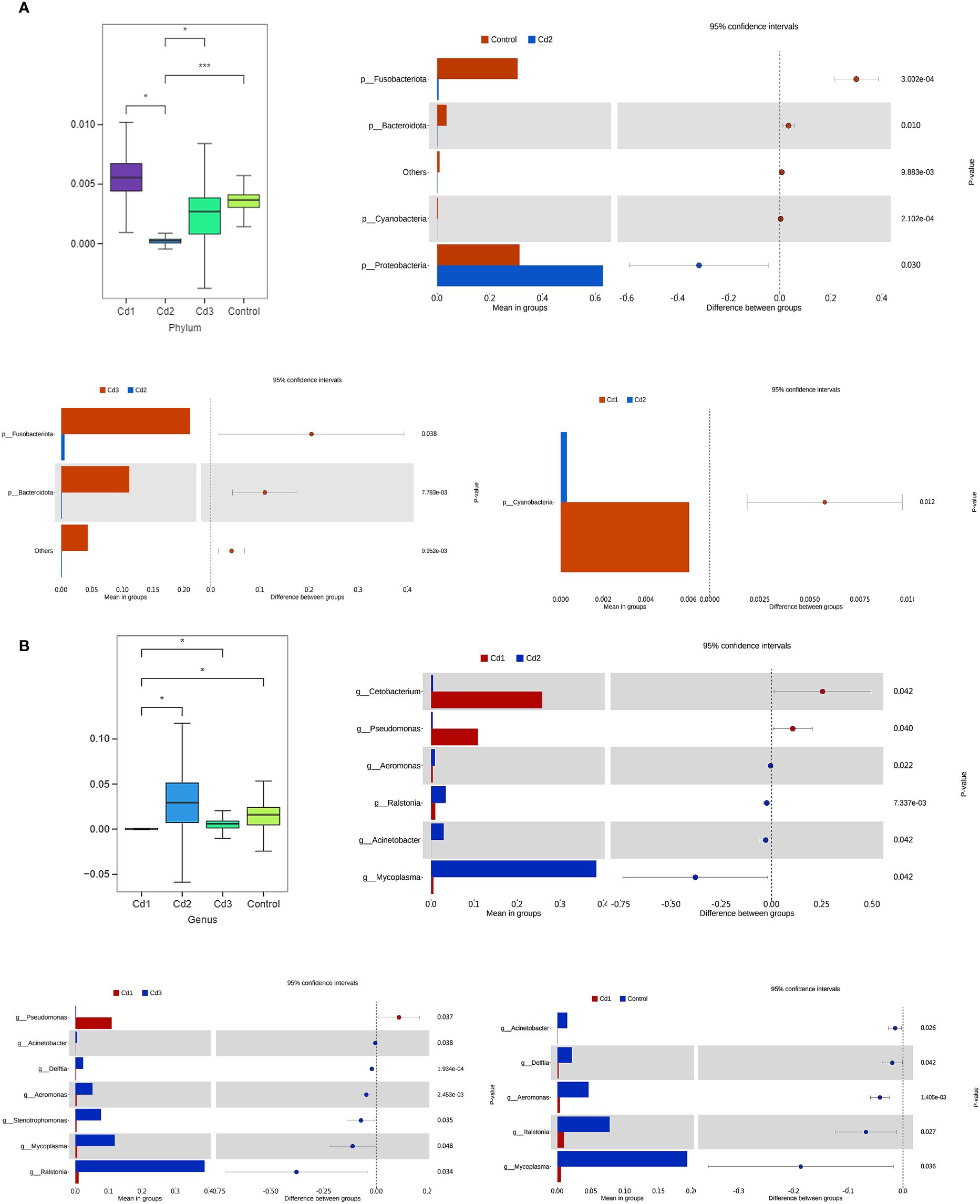
Figure 8. Effects of cadmium on microbial communities of largemouth bass among different treatment groups. The diversity among the top 10 abundant microbial communities at the phylum level (A) and genus level (B), respectively. The symbol * shows significant differences among the groups (*P< 0.05, **P< 0.01, ***P< 0.001).
3.9 Correlation analysis of intestinal microbiome and liver immune index
Spearman correlation analysis revealed potential associations between the intestinal microbiome composition under cadmium stress and liver immune markers. As depicted in Figure 9, the correlations were more significant at the genus level, focusing mainly on Pseudomonas and Cetobacterium. The experiment observed a significant positive correlation between Pseudomonas and Cetobacterium with IL-10, GPX, and CAT, as well as a negative correlation with MT, HSP70, MDA, TNF-α, and IL-1β (P< 0.01). Furthermore, Pseudomonas showed a negative correlation with IL-15, while Cephalosporin exhibited a positive correlation with Nrf2 and a negative correlation with Keap1 (P< 0.001). Overall, a strong association between gut microbes and liver immunity can be concluded.
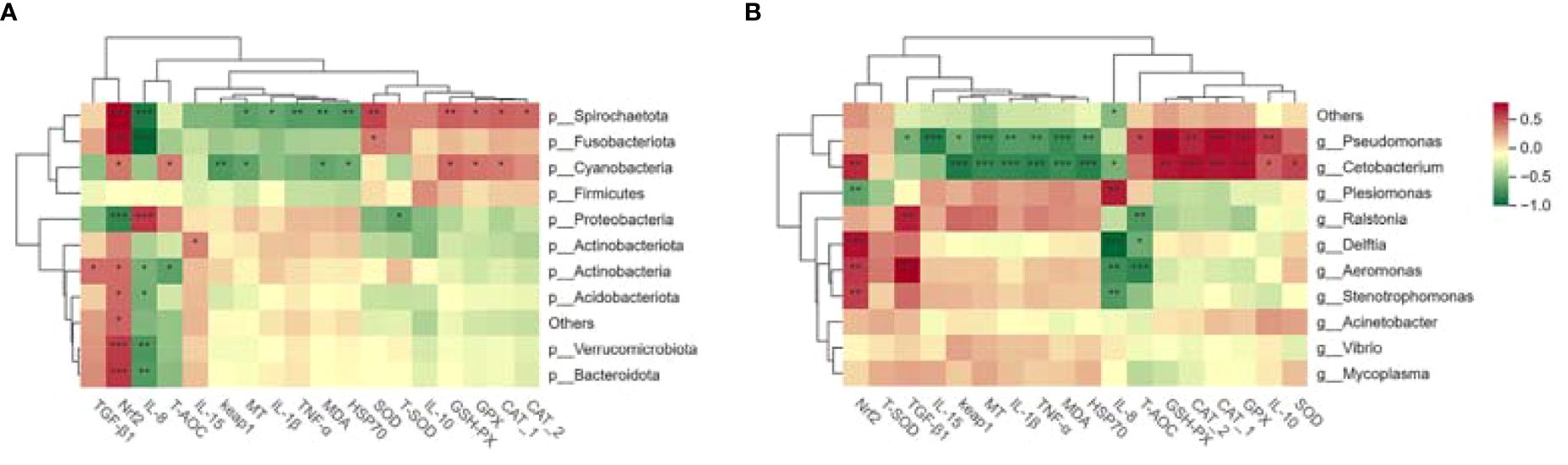
Figure 9. The correlation analysis of cadmium exposure on the intestinal microbiota and liver immune indexes of largemouth bass at the phylum level (A) and genus level (B). Significant differences were observed among the groups (*P< 0.05, **P< 0.01, ***P< 0.001). In the figure, red indicates a positive correlation, while green indicates a negative correlation. CAT-1 indicates enzyme activity, and CAT-2 indicates relative mRNA expression.
4 Discussion
Rapid industrial development has led to heavy cadmium pollution (Wang et al., 2021). Cadmium is significantly toxic and can cause harm to biological health even at a light concentration (Mikhailenko et al., 2020). Many studies have found that cadmium stress can cause liver injury, which in turn triggers inflammatory responses (Honglong et al., 2021). Cadmium can also damage intestinal morphology as well as its barrier (Xiaoya et al., 2022). Many studies have found that the potential effects between the gut and the liver are well connected to the gut–liver axis, but whether the gut–liver axis exists in aquatic animals is uncertain. Previous studies have shown that cadmium-induced tissue damage is primarily attributable to storm-induced oxidative stress (Abarghouei et al., 2021). As cadmium concentration increases, more cadmium ions accumulate in the liver, potentially leading to liver lesions and cell necrosis (Chen et al., 2023). Tissue sections of the present study showed hypertrophy of the liver cells, nuclear consolidation, vacuolization, cellular degeneration, shortening of intestinal villi, intestinal epithelial hyperplasia, and other lesions in the cadmium-treated group. Meanwhile, antioxidant capacity was measured using liver homogenates, which showed a remarkable increase in the levels of MDA and ROS, and a significant decrease in the activities of CAT and GSH-PX, which was most severe in the Cd3 group (Dai et al., 2020; Zhang et al., 2020b). MDA is the end product of lipid oxidative stress and indirectly reflects the degree of cellular damage (Ben et al., 2023). Oxidative stress triggers the production of ROS within cells (Kumar et al., 2018). Consequently, the antioxidant enzymes in the organism’s defense system may be induced, leading to a decrease in enzyme activities, including GSH-PX, SOD, and CAT (Salama et al., 2018; Li et al., 2024). Under Cd stress, fish are exposed to stress that leads to reduced food intake and increased energy expense, thus adversely affecting growth parameters (e.g., WGR, SGR, SR, CF, and FCR) (Jamwal et al., 2018; Xie et al., 2019; Won et al., 2023; Abdel-Tawwab et al., 2024). Furthermore, cadmium can inhibit the activity of digestive enzymes in fish, resulting in increased metabolites, hepatomegaly, and increased HSI (Chen et al., 2020; Luo et al., 2020). Overall, the changes in growth performance observed in this study align with previous research findings, indicating that cadmium stress causes significant damage to liver tissue, resulting in oxidative stress and reduced growth performance of largemouth bass, with a more pronounced effect observed at higher cadmium concentrations.
Nrf2 goes hand in hand with oxidative stress induced by heavy metals (Chen et al., 2018; Lian et al., 2023). To further investigate the effect of cadmium on the Nrf2–Keap1 pathway of largemouth bass, the enzyme activity and the mRNA expression levels of oxidative stress-related genes were analyzed. Keap1 is a regulator of Nrf2, with both acting as a pervasive intracellular defense mechanism to counteract oxidative stress (Bellezza et al., 2018). Under the stimulation of ROS, Nrf2 and Keap1 are uncoupled, which causes Nrf2 to transfer to the nucleus and bind to ARE, inducing the expression of antioxidant enzyme genes to achieve a protective effect on cells (Yang et al., 2018). High concentrations of cadmium inhibited the expression of Nrf2, promoted the expression of Keap1, and thus significantly reduced the activities of SOD, CAT, and GPX, which is the same as the results of the antioxidant capacity of the liver in the present experience. The results of cadmium in the Nrf2–Keap1 signaling pathway in mice had similar results (He et al., 2018). In general, the effect of cadmium on the Nrf2–Keap1 pathway of largemouth bass is still indistinct.
When the liver is stimulated by cadmium, the redox balance of the cells is disrupted, leading to an inflammatory response in the cells (Griffiths et al., 2017). The present results showed that the expression level of IL-10 was significantly downregulated while the expression of IL-8, IL-15, IL-1β, TNF-α, and TGF-β1 was substantially upregulated compared to the control group. Cadmium can inhibit the expression of anti-inflammatory factors IL-10 and TGF-β1 and enhance the expression of pro-inflammatory factors IL-8, IL-15, IL-1β, and TNF-α (Lin et al., 2023; Zhu et al., 2023). One study reported that feeding pigs with 20 mg Cd/kg increased mRNA expression of TGF-β1 (Zhao et al., 2021). In the results of the present study, TGF-α expression decreased and then increased, which may indicate that low concentrations of cadmium were able to inhibit the expression of TGF-β1.
The liver can detoxify and excrete metal ions by inducing MT and HSPs (Paul and Small, 2021). Early findings suggest that MT and HSPs can be used as biomarkers for assessing the toxicity of heavy metals (Aljazzar et al., 2021; Timofeev et al., 2023). HSP70 is abundantly expressed mainly in hepatic tissues, and the synthesis of heat shock proteins is enhanced when stress or pathological injury occurs (Stygar et al., 2019). MT belongs to a family of cysteine-rich metal-binding proteins that play essential roles in maintaining metal homeostasis and detoxification (Yin et al., 2022). In this experiment, the expression of the MT gene was significantly upregulated with the increase of HSP70 enzyme activity. The expression trend of MT genes was consistent with the results of studies on earthworms (Uwizeyimana et al., 2018), South American white shrimp (Chen et al., 2022), dogs, and cats (Nytko et al., 2023), while the expression results of HSP70 did not show significant differences at low concentrations. However, there was a significant difference in the expression results of HSP70 in this experiment, which may indicate that it was due to the higher concentration of cadmium in the present experiment. Overall, cadmium induced a significant upregulation of MT and HSP70 expression, and HSP70 was correlated positively with cadmium. The reason may indicate that hepatocyte stimulation by cadmium activated the enzyme activity of HSP70, which caused MT to bind closely to cadmium and play a protective role in the liver.
Tight junction proteins play a crucial role in intestinal permeability and maintenance of intestinal epithelial integrity, and alterations in gut microbiota may disrupt ZO-1 and Occludin (Chelakkot et al., 2018). The results of this study demonstrate a significant decrease in the levels of ZO-1 and Occludin, indicating cadmium-induced disruption of the intestinal barrier. High-throughput sequencing was also employed to assess the gut microbiota of largemouth bass, which showed a significant decrease in α- and β-diversity in the low-concentration group and a substantial increase in the high-concentration group, suggesting dysbiosis in the gut microbiota of largemouth bass. Studies have confirmed that low concentrations of cadmium can reduce biodiversity (Feng et al., 2019). In this experiment, the Cd2 group showed a significant decrease in biodiversity, while the Cd3 group showed no significant difference in biodiversity compared to the control group. We speculate that this may indicate tolerance of some microbes to cadmium after prolonged exposure. This result is consistent with the effects of 2 mg/L Cd on fish (Chang et al., 2019) and freshwater lobster (Zhang et al., 2020a), with some differences from the effects on mouse gut microbes (He et al., 2020), which may be due to species differences. The results showed that cadmium had a more significant effect on Aspergillus spp. The dominance of Proteobacteria may change the structure and composition of intestinal epithelial tight junction proteins and damage the intestinal barrier (Joeri et al., 2020). In addition, the correlation results showed that intestinal microorganisms had a strong tie with Nrf2 and Keap1, indicating that intestinal microorganisms can regulate liver inflammation through the Nrf2–Keap1 pathway (Chelakkot et al., 2018). However, the mechanism of cadmium’s effect on intestinal microorganisms in freshwater fish is still unclear. The significant correlation between intestinal microorganisms and liver immune indicators can indicate that the intestinal–liver axis theory is feasible in the study of freshwater fish.
5 Conclusion
In summary, cadmium can potentially impair the intestinal barrier of largemouth bass, thereby influencing the composition and structure of intestinal microbes and triggering a liver inflammatory response. Cadmium damages detoxification and immune-related tissues, which suppresses the body’s antioxidant and immune systems. Antioxidant stress, tissue damage, and microbiological imbalances have demonstrated the presence of cadmium toxicity in largemouth bass. This experiment elucidated the effects of cadmium on the liver and enteric toxicity of largemouth bass through the gut–liver axis pathway, laying the groundwork for studying the mechanisms of the gut–liver axis in freshwater fish.
Data availability statement
The original contributions presented in the study are included in the article/supplementary material. Further inquiries can be directed to the corresponding authors.
Ethics statement
This trial was approved by the Southwest University of Science and Technology in China, Institutional Animal Care and Use Committee. All of the procedures were performed in accordance with the Declaration of Helsinki and relevant policies in China. The studies were conducted in accordance with the local legislation and institutional requirements. Written informed consent was obtained from the owners for the participation of their animals in this study.
Author contributions
LJ: Data curation, Methodology, Conceptualization, Project administration, Validation, Funding acquisition, Resources, Visualization, Writing – original draft. QW: Conceptualization, Data curation, Formal analysis, Investigation, Methodology, Project administration, Software, Writing – original draft. SB: Formal analysis, Methodology, Writing – original draft, Data curation, Project administration. GF: Conceptualization, Data curation, Formal analysis, Investigation, Methodology, Writing – original draft. ZY: Data curation, Formal analysis, Investigation, Methodology, Writing – original draft. PZ: Data curation, Investigation, Methodology, Writing – original draft. XY: Data curation, Investigation, Methodology, Writing – original draft. XL: Formal analysis, Investigation, Methodology, Writing – original draft. XZ: Conceptualization, Data curation, Formal analysis, Investigation, Methodology, Validation, Writing – original draft. YW: Conceptualization, Funding acquisition, Resources, Supervision, Writing – review & editing.
Funding
The author(s) declare financial support was received for the research, authorship, and/or publication of this article. This work was supported by the Sichuan Province Rural Revitalization Science and Technology Project Program (23zs2105).
Conflict of interest
The authors declare that the research was conducted in the absence of any commercial or financial relationships that could be construed as a potential conflict of interest.
Publisher’s note
All claims expressed in this article are solely those of the authors and do not necessarily represent those of their affiliated organizations, or those of the publisher, the editors and the reviewers. Any product that may be evaluated in this article, or claim that may be made by its manufacturer, is not guaranteed or endorsed by the publisher.
References
Abarghouei S., Hedayati A., Raeisi M., Hadavand B. S., Rezaei H., Abed-Elmdoust A. (2021). Size-dependent effects of microplastic on uptake, immune system, related gene expression and histopathology of goldfish (Carassius auratus). Chemosphere 276, 129977. doi: 10.1016/j.chemosphere.2021.129977
Abdel-Tawwab M., Khalil R. H., Younis N. A., Abo Selema T. A., Saad A. H., et al. (2024). Saccharomyces cerevisiae supplemented diets mitigate the effects of waterborne cadmium toxicity on gilthead seabream (Sparus aurata L.): growth performance, haemato-biochemical, stress biomarkers, and histopathological investigations. Vet. Res. Commun. 48, 69–84. doi: 10.1007/s11259-023-10176-0
Aljazzar A., El-Ghareeb W. R., Darwish W. S., Abdel-Raheem S. M., Ibrahim A. M. (2021). Content of total aflatoxin, lead, and cadmium in the bovine meat and edible offal: study of their human dietary intake, health risk assessment, and molecular biomarkers. Environ. Sci. pollut. 28, 61225–61234. doi: 10.1007/s11356-021-12641-2
Andersen L. K., Abernathy J., Berlinsky D. L., Bolton G., Booker M. M., Borski R. J., et al (2021). The status of striped bass, Morone saxatilis, as a commercially ready species for US marine aquaculture. J World Aquacult Soc. 52, 710–730. doi: 10.1111/jwas.12812
Baş H., Apaydın F. G., Kalender S., Kalender Y. (2021). Lead nitrate and cadmium chloride induced hepatotoxicity and nephrotoxicity: Protective effects of sesamol on biochemical indices and pathological changes. J. Food Biochem. 45, e13769. doi: 10.1111/jfbc.13769
Bellezza I., Giambanco I., Minelli A., Donato R. (2018). Nrf2-Keap1 signaling in oxidative and reductive stress. Biochim. Biophys. Acta (BBA)-Mol. Cell Res. 1865, 721–733. doi: 10.1016/j.bbamcr.2018.02.010
Ben Y., Cheng M., Liu Y., Wang X., Wang L., Yang Q., et al. (2023). Biomarker changes and oxidative damage in living plant cells as new biomonitoring indicators for combined heavy metal stress assessment. Ecol. Indic. 154, 110784. doi: 10.1016/j.ecolind.2023.110784
Chang X., Li H., Feng J., Chen Y., Nie G., Zhang J. (2019). Effects of cadmium exposure on the composition and diversity of the intestinal microbial community of common carp (Cyprinus carpio L.). Ecotox Environ. Safe. 171, 92–98. doi: 10.1016/j.ecoenv.2018.12.066
Chelakkot C., Ghim J., Ryu S. H. (2018). Mechanisms regulating intestinal barrier integrity and its pathological implications. Exp. Mol. Med. 50, 1–9. doi: 10.1038/s12276-018-0126-x
Chen M., Bao X., Yue Y., Yang K., Liu H., Yang Y., et al. (2023). Combined effects of cadmium and nanoplastics on oxidative stress, histopathology, and intestinal microbiota in largemouth bass (Micropterus salmoides). Aquaculture 569, 739363. doi: 10.1016/j.aquaculture.2023.739363
Chen T., Li S., Liang Z., Li L., Guo H. (2022). Effects of copper pyrithione (CuPT) on apoptosis, ROS production, and gene expression in hemocytes of white shrimp Litopenaeus vannamei. Comp. Biochem. Phys. C. 256, 109323. doi: 10.1016/j.cbpc.2022.109323
Chen S.-n., Tan Y., Xiao X.-c., Li Q., Wu Q., Peng Y.-y., et al. (2021). Deletion of TLR4 attenuates lipopolysaccharide-induced acute liver injury by inhibiting inflammation and apoptosis. Acta Pharmacol. Sin. 42, 1610–1619. doi: 10.1038/s41401-020-00597-x
Chen L., Zhang J., Zhu Y., Zhang Y. (2018). Interaction of chromium (III) or chromium (VI) with catalase and its effect on the structure and function of catalase: An in vitro study. Food Chem. 244, 378–385. doi: 10.1016/j.foodchem.2017.10.062
Chen M., Zhou J., Lin J., Tang H., Shan Y., Chang A. K., et al. (2020). Changes in oxidative stress biomarkers in Sinonovacula constricta in response to toxic metal accumulation during growth in an aquaculture farm. Chemosphere 248, 125974. doi: 10.1016/j.chemosphere.2020.125974
Cui J., Yin S., Zhao C., Fan L., Hu H. (2021). Combining Patulin with Cadmium Induces Enhanced Hepatotoxicity and Nephrotoxicity in vitro and in vivo. Toxins 13, 221. doi: 10.3390/toxins13030221
Dai Z., Cheng J., Bao L., Zhu X., Li H., Chen X., et al. (2020). Exposure to waterborne cadmium induce oxidative stress, autophagy and mitochondrial dysfunction in the liver of Procypris merus. Ecotox Environ. Safe. 204, 111051. doi: 10.1016/j.ecoenv.2020.111051
Feng S., Liu Y., Huang Y., Zhao J., Zhang H., Zhai Q., et al. (2019). Influence of oral administration of Akkermansia muciniphila on the tissue distribution and gut microbiota composition of acute and chronic cadmium exposure mice. FEMS Microbiol. Lett. 366, fnz160. doi: 10.1093/femsle/fnz160
Griffiths H. R., Gao D., Pararasa C. (2017). Redox regulation in metabolic programming and inflammation. Redox Biol. 12, 50–57. doi: 10.1016/j.redox.2017.01.023
He L., Li P., Yu L.-H., Li L., Zhang Y., Guo Y., et al. (2018). Protective effects of proanthocyanidins against cadmium-induced testicular injury through the modification of Nrf2-Keap1 signal path in rats. Environ. Toxicol. Phar. 57, 1–8. doi: 10.1016/j.etap.2017.11.002
He X., Qi Z., Hou H., Qian L., Gao J., Zhang X.-X. (2020). Structural and functional alterations of gut microbiome in mice induced by chronic cadmium exposure. Chemosphere 246, 125747. doi: 10.1016/j.chemosphere.2019.125747
He Q., Ye K., Han W., Yekefenhazi D., Sun S., et al. (2022). Mapping sex-determination region and screening DNA markers for genetic sex identification in largemouth bass (Micropterus salmoides). Aquaculture 559, 738450. doi: 10.1016/j.aquaculture.2022.738450
Honglong Z., Jun Y., Ye X., Xuhong C., Junliang L., Chenghui R., et al. (2021). Dual role of cadmium in rat liver: inducing liver injury and inhibiting the progression of early liver cancer. Toxicol. Lett. 355, 62–81. doi: 10.1016/j.toxlet.2021.11.004
Hou H., Ren A., Yu L., Ma Z., Zhang Y., Liu Y. (2023). An environmental impact assessment of largemouth bass (Micropterus salmoides) aquaculture in Hangzhou, China. Sustainability 15, 12368. doi: 10.3390/su151612368
Jamwal A., Lemire D., Driessnack M., Naderi M., Niyogi S. (2018). Interactive effects of chronic dietary selenomethionine and cadmium exposure in rainbow trout (Oncorhynchus mykiss): A preliminary study. Chemosphere 197, 550–559. doi: 10.1016/j.chemosphere.2018.01.087
Joeri T., Glenn V., Jan Van D., Edward G., Bert D., Lien L., et al. (2020). Increased levels of systemic LPS-positive bacterial extracellular vesicles in patients with intestinal barrier dysfunction. Gut 69, 191–1193. doi: 10.1136/gutjnl-2018-317726
Junjie B., Shengjie L. (2019). “Molecular Marker-Assisted Selective Breeding of Largemouth Bass,” in Genetic Breeding and Molecular Marker-Assisted Selective Breeding of Largemouth Bass ed. A. G. Wolff (Beijing, FL: Science Press), 169–298.
Khan M. B., Setu S., Sultana N., Gautam S., Begum B. A., Salam M. A., et al. (2023). Street dust in the largest urban agglomeration: pollution characteristics, source apportionment and health risk assessment of potentially toxic trace elements. Stoch Env. Res. Risk A. 37, 3305–3324. doi: 10.1007/s00477-023-02432-1
Kumar N., Kumari V., Ram C., Bharath Kumar B. S., Verma S. (2018). Impact of oral cadmium intoxication on levels of different essential trace elements and oxidative stress measures in mice: a response to dose. Environ. Sci. pollut. Res. 25, 5401–5411. doi: 10.1007/s11356-017-0868-3
Li T., Jin M., Huang L., Zhang Y., Zong J., Shan H., et al. (2023). Oxytetracycline-induced oxidative liver damage by disturbed mitochondrial dynamics and impaired enzyme antioxidants in largemouth bass (Micropterus salmoides). Aquat. Toxicol. 261, 106616. doi: 10.1016/j.aquatox.2023.106616
Li X., Wu Q., Chen D., Bai Y., Yang Y., Xu S. (2024). Environment-relevant concentrations of cadmium induces necroptosis and inflammation; baicalein maintains gill homeostasis through suppressing ROS/ER stress signaling in common carps (Cyprinus carpio L.). Environ. pollut. 340, 122805. doi: 10.1016/j.envpol.2023.122805
Lian C.-Y., Chu B.-X., Xia W.-H., Wang Z.-Y., Fan R.-F., Wang L. (2023). Persistent activation of Nrf2 in a p62-dependent non-canonical manner aggravates lead-induced kidney injury by promoting apoptosis and inhibiting autophagy. J. Adv. Res. 46, 87–100. doi: 10.1016/j.jare.2022.04.016
Lin X., Xu Y., Tong T., Zhang J., He H., Yang L., et al. (2023). Cadmium exposure disturbs myocardial lipid signature and induces inflammation in C57BL/6J mice. Ecotox Environ. Safe. 265, 115517. doi: 10.1016/j.ecoenv.2023.115517
Liu X., Zhang J., Si J., Li P., Gao H., Li W., et al. (2023). What happens to gut microorganisms and potential repair mechanisms when meet heavy metal (loid) s. Environ. pollut. 317, 120780. doi: 10.1016/j.envpol.2022.120780
Luo J., Zhu T., Wang X., Cheng X., Yuan Y., Jin M., et al. (2020). Toxicological mechanism of excessive copper supplementation: Effects on coloration, copper bioaccumulation and oxidation resistance in mud crab Scylla paramamosain. J. Hazard Mater. 395, 122600. doi: 10.1016/j.jhazmat.2020.122600
Meng X.-L., Li S., Qin C.-B., Zhu Z.-X., Hu W.-P., Yang L.-P., et al. (2018). Intestinal microbiota and lipid metabolism responses in the common carp (Cyprinus carpio L.) following copper exposure. Ecotox Environ. Safe. 160, 257–264. doi: 10.1016/j.ecoenv.2018.05.050
Mikhailenko A. V., Ruban D. A., Ermolaev V. A., van Loon A. J. (2020). Cadmium pollution in the tourism environment: A literature review. Geosciences 10, 242. doi: 10.3390/geosciences10060242
Nytko K. J., Weyland M. S., Dressel-Böhm S., Scheidegger S., Salvermoser L., Werner C., et al. (2023). Extracellular heat shock protein 70 levels in tumour-bearing dogs and cats treated with radiation therapy and hyperthermia. Vet. Comp. Oncol. 21, 605–615. doi: 10.1111/vco.12923
Paul J. S., Small B. C. (2021). Chronic exposure to environmental cadmium affects growth and survival, cellular stress, and glucose metabolism in juvenile channel catfish (Ictalurus punctatus). Aquat. Toxicol. 230, 105705. doi: 10.1016/j.aquatox.2020.105705
Qi M., Wu Y., Zhang S., Li G., An T. (2023). Pollution profiles, source identification and health risk assessment of heavy metals in soil near a non-ferrous metal smelting plant. Int. J. Environ. Res.Public Health 20, 1004. doi: 10.3390/ijerph20021004
Salama S. A., Arab H. H., Omar H. A., Gad H. S., Abd-Allah G. M., Maghrabi I. A., et al. (2018). L-carnitine mitigates UVA-induced skin tissue injury in rats through downregulation of oxidative stress, p38/c-Fos signaling, and the proinflammatory cytokines. Chem. Biol. Interact. 285, 40–47. doi: 10.1016/j.cbi.2018.02.034
Sivaprakasam C., Nachiappan V. (2016). Modulatory effect of cadmium on the expression of phospholipase A2 and proinflammatory genes in rat testis. Environ. Toxicol. 31, 1176–1184. doi: 10.1002/tox.v31.10
Stygar D., Skrzep-Poloczek B., Romuk E., Poloczek J., Sawczyn T., et al. (2019). The influence of high-fat high-sugar diet and bariatric surgery on HSP70 and HSP90 plasma and liver concentrations in diet-induced obese rats. Cell Stress Chaperon. 24, 427–439. doi: 10.1007/s12192-019-00976-2
Timofeev Y. S., Kiselev A. R., Dzhioeva O. N., Drapkina O. M. (2023). Heat shock proteins (HSPs) and cardiovascular complications of obesity: searching for potential biomarkers. Curr. Issues Mol. Biol. 45, 9378–9389. doi: 10.3390/cimb45120588
Topic Popovic N., Strunjak-Perovic I., Coz-Rakovac R., Barisic J., Jadan M., Persin Berakovic A., et al. (2012). Tricaine methane-sulfonate (MS-222) application in fish anaesthesia. J. Appl. Ichthyol. 28, 553–564. doi: 10.1111/jai.2012.28.issue-4
Uwizeyimana H., Wang M., Chen W., Khan K. (2018). Ecotoxicological effects of binary mixtures of siduron and Cd on mRNA expression in the earthworm Eisenia fetida. Sci. Total Environ. 610, 657–665. doi: 10.1016/j.scitotenv.2017.07.265
Vinanthi Rajalakshmi K. S., Liu W.-C., Balamuralikrishnan B., Meyyazhagan A., Sattanathan G., Pappuswamy M., et al. (2023). Cadmium as an endocrine disruptor that hinders the reproductive and developmental pathways in freshwater fish: A review. Fishes 8, 589. doi: 10.3390/fishes8120589
Wang M., Chen Z., Song W., Hong D., Huang L., Li Y. (2021). A review on cadmium exposure in the population and intervention strategies against cadmium toxicity. Bull. Environ. Contam. Toxicol. 106, 65–74. doi: 10.1007/s00128-020-03088-1
Won T.-J., Yu Y.-B., Kang J.-H., Kim J.-H., Kang J.-C. (2023). Effects on bioaccumulation, growth performance, hematological parameters, plasma components, and antioxidant responses in starry flounder (Platichthys stellatus) exposed to dietary cadmium and ascorbic acid. Antioxid 12, 128. doi: 10.3390/antiox12010128
Xiaoya L., Yi W., Guozhen X., Zhoujin T. (2022). Effects of chronic cadmium exposure on the structure and function of intestinal mucosal flora in mice. Toxin Rev. 41, 904–917. doi: 10.1080/15569543.2021.1955712
Xie D., Li Y., Liu Z., Chen Q. (2019). Inhibitory effect of cadmium exposure on digestive activity, antioxidant capacity and immune defense in the intestine of yellow catfish (Pelteobagrus fulvidraco). Comp. Biochem. Phys. C. 222, 65–73. doi: 10.1080/15569543.2021.1955712
Yang B., Bai Y., Yin C., Qian H., Xing G., Wang S., et al. (2018). Activation of autophagic flux and the Nrf2/ARE signaling pathway by hydrogen sulfide protects against acrylonitrile-induced neurotoxicity in primary rat astrocytes. Arch. Toxicol. 92, 2093–2108. doi: 10.1007/s00204-018-2208-x
Yin Y., Gu Y., Zai X., Li R., Zhu X., Yu R., et al. (2022). A novel built-in adjuvant metallothionein-3 aids protein antigens to induce rapid, robust, and durable immune responses. Front. Immunol. 13. doi: 10.3389/fimmu.2022.1024437
Zhang Y., Li Z., Kholodkevich S., Sharov A., Chen C., Feng Y., et al. (2020a). Effects of cadmium on intestinal histology and microbiota in freshwater crayfish (Procambarus clarkii). Chemosphere 242, 125105. doi: 10.1016/j.chemosphere.2019.125105
Zhang Y., Song B., Zhou Z. (2023). Pollution assessment and source apportionment of heavy metals in soil from lead – Zinc mining areas of south China. J. Environ. 11, 109320. doi: 10.1016/j.jece.2023.109320
Zhang Y., Xie S., Wei H., Zheng L., Liu Z., Fang H., et al. (2020b). High dietary starch impaired growth performance, liver histology and hepatic glucose metabolism of juvenile largemouth bass, Micropterus salmoides. Aquacult. Nutr. 26, 1083–1095. doi: 10.1111/anu.13066
Zhao X., Wang S., Li X., Liu H., Xu S. (2021). Cadmium exposure induces TNF-α-mediated necroptosis via FPR2/TGF-β/NF-κB pathway in swine myocardium. Toxicology 453, 152733. doi: 10.1016/j.tox.2021.152733
Keywords: cadmium, largemouth bass, gut-liver axis, intestinal microbiota, inflammatory response, hepatotoxicity
Citation: Jiang L, Wu Q, Bao S, Fan G, Yang Z, Zhou P, Yang X, Liu X, Zhou X and Wang Y (2024) Cadmium stress induces gut microbiota imbalance and consequent activation of the gut–liver axis leading to liver injury and inflammation response in largemouth bass (Micropterus salmoides). Front. Mar. Sci. 11:1449091. doi: 10.3389/fmars.2024.1449091
Received: 17 June 2024; Accepted: 08 July 2024;
Published: 13 August 2024.
Edited by:
Yafei Duan, South China Sea Fisheries Research Institute, ChinaCopyright © 2024 Jiang, Wu, Bao, Fan, Yang, Zhou, Yang, Liu, Zhou and Wang. This is an open-access article distributed under the terms of the Creative Commons Attribution License (CC BY). The use, distribution or reproduction in other forums is permitted, provided the original author(s) and the copyright owner(s) are credited and that the original publication in this journal is cited, in accordance with accepted academic practice. No use, distribution or reproduction is permitted which does not comply with these terms.
*Correspondence: Yachao Wang, d2FuZ3lhY2hhbzE2ODhAMTI2LmNvbQ==
†These authors have contributed equally to this work and share first authorship