- 1College of Fisheries, Southwest University, Chongqing, China
- 2Integrative Science Center of Germplasm Creation in Western China (CHONGQING) Science City, Southwest University, Chongqing, China
Gymnocypris eckloni (G. eckloni), a cold-water economic fish, is widely cultivated in southwestern China. The increase in extreme summer weather conditions owing to global warming can significantly affect their survival and health. The fish intestine and its microbiota are closely associated with fish feeding and growth, nutritional metabolism, and immune defense. However, the mechanisms underlying the changes in the G. eckloni intestine and its microbiota under acute heat stress remain unknown. In this study, we investigated the effects of acute heat stress on the G. eckloni intestine employing histology, plasma biochemical indices, transcriptomics, and 16S rDNA sequencing. Histological analysis showed that acute heat stress induced significant morphological damage to the intestine, with microvilli detachment and mitochondrial abnormalities in the ultrastructure. Biochemical indicators associated with stress (reactive oxygen species and catalase), inflammation (interleukin-1β and tumor necrosis factor-α), and intestinal permeability (diamine oxidase and lipopolysaccharide) were significantly elevated after acute heat stress, indicating an intestinal inflammatory response and disruption of barrier function. Many DEGs were mined by transcriptomic analysis, with tfrc, pfkp, egln1 enriched in the HlF-1 signaling pathway, hsp70, hsp90aa1 and hspa4 enriched in the Antigen processing and presentation pathway, pmm1, pfkfb3 and hk1 enriched in the Fructose and mannose metabolism patyway. The HIF-1 signaling pathway is a crucial regulatory pathway during acute heat stress in the G. eckloni intestine, while significant downregulation of genes associated with adaptive immunity (mica, hla-dpa1, hla-dpb1, and hla-dqb2) suggested impaired immune function. Additionally, the composition of the intestinal microbiota was dominated by Aeromonas, Citrobacter, and Acinetobacter in the control group; but there was a significant decrease in the abundance of Citrobacter and Acinetobacter, and a significantly increased in Shewanella and Hafnia-Obesumbacterium after acute heat stress. Correlation analyses revealed that changes in the abundance of Hafnia-Obesumbacterium, Buttiauxella, and Pseudomonas were closely associated with changes in gene expression associated with stress, inflammation, and immunity. These results comprehensively demonstrate the adaptive mechanisms of the G. eckloni intestine in response to acutely high temperatures and provide a theoretical basis for the future advancement of artificial culture of cold-water fish.
1 Introduction
Temperature is a crucial environmental factor that influences the survival and growth of animals, significantly impacting their metabolism, physiology, and behavior (Polsky and von Keyserlingk, 2017; Farag and Alagawany, 2018; Ern et al., 2023). In recent years, rising temperatures have become an increasing threat to freshwater ecosystems, exposing aquatic organisms to more frequent climate extremes (Barbarossa et al., 2021). Fish, which cannot maintain a constant body temperature, are more vulnerable to these temperature fluctuations (GusChina and Harwood, 2006). High temperatures can induce heat stress in fish, with acute heat stress from short-term temperature spikes causing significant disruptions to their physiological homeostasis, thereby threatening their health and survival (Stewart et al., 2019; Alfonso et al., 2021). To predict the adaptive capacity of fish in response to climate change, understanding the mechanisms through which acute temperature changes affect their physiology and adaptation is essential.
The intestine is an extremely complex ecosystem composed of the gastrointestinal epithelium, a mucus layer, immune cells, and resident microbiota (Cornick et al., 2015). It is a critical digestive and absorptive organ for fish and also influences their immunity, endocrinology, and defense mechanisms (Zhu et al., 2013). The intestine is sensitive to various stressors, including heat stress, hypoxia, and transport (Sundh et al., 2010; Cao et al., 2021; Zheng et al., 2022; Liu et al., 2024). Stress can alter intestinal function by affecting the brain-intestine axis, potentially leading to inflammation or intestinal disease (Zhou et al., 2022). Numerous studies have shown that heat stress not only affects feeding and growth processes in fish (Wade et al., 2019; Chen et al., 2022), but also influences intestinal barrier function, alters the intestinal microbiota, and disrupts homeostasis (Sundh et al., 2010; Huyben et al., 2019; Sepulveda and Moeller, 2020). Intestinal microbiota are crucial for teleost fish in terms of nutrient supply, disease resistance, and immune maintenance (Nayak, 2010; Llewellyn et al., 2014; Wang et al., 2018). Research on the effects of temperature on intestinal microbiology have been conducted in various fish species, such as sturgeons (Yang et al., 2022b), Seriola lalandi (Soriano et al., 2018), and rainbow trout (Zhou et al., 2022), demonstrating that high temperatures severely affect intestinal microbiota. Intestinal microbiota play an essential role in stress response and help the host adapt to temperature changes (Sekirov et al., 2010; Kokou et al., 2018). However, previous studies have primarily focused on observations of intestinal histology or changes in intestinal function due to heat stress (Olsen et al., 2005; Golovanova et al., 2013; Huyben et al., 2019), or preliminary analyses of the intestinal microbiome (Yang et al., 2022b). Few studies have been conducted on the interaction between heat stress, fish intestinal microbiota, and transcriptional profiles. Integrating intestinal transcriptomics and microbiome analyses can better explore regulatory mechanisms in fish under adverse environmental conditions (Czech et al., 2022; Yang et al., 2022c).
Gymnocypris eckloni, a schizothoracine fish endemic to the Qinghai-Tibetan Plateau, is a significant aquatic biological resource. It is widely distributed in the Golmud River, isolated lakes, and the upper reaches of the Yellow River. G. eckloni serves as a crucial aquatic wildlife germplasm resource in Qinghai Province and a component of biodiversity in the upper waters of the Yellow River, playing an important role in the freshwater ecosystem of the plateau (Wu and Wu, 1992; Wang et al., 2022a). In addition, it has a high economic exploitation value due to its delicious taste and rich nutrition, and has been cultured on a large scale in Southwest China (Yang et al., 2017). Artificial breeding studies have shown that G. eckloni exhibits strong sensitivity to high temperatures, with optimal growth rates at water temperatures between 16°C and 18°C, with growth rates decreasing above 20°C (Jian et al., 2020). Consequently, the species is susceptible to acute heat stress caused by increased climatic extremes of high temperature. Current research on G. eckloni has focused on genome assembly (Wang et al., 2022a), phylogeny (Wanghe et al., 2022), biological characteristics (Yang et al., 2017), and artificial breeding (Dong et al., 2016), but studies on the effects of high temperatures remain relatively scarce (Li et al., 2024b). Thus, in this study, we integrated histology, biochemical indicators, transcriptomics, and 16S rDNA analysis to examine the effects of acute heat stress on the G. eckloni intestine. This comprehensive approach provided a better understanding of how temperature affects fish intestinal physiology and microbiota, providing a theoretical basis for future advancements in the artificial culture of cold-water fish.
2 Materials and methods
2.1 Fish and experimental design
A total of 90 healthy G. eckloni (body weight 262 ± 19.8 g) were acquired from Chuanze Fisheries Co. Ltd. (Sichuan, China), and transported to the indoor laboratory at the Aquaculture Base of Southwest University in November 2023 for subsequent experiment. They were equally distributed among six glass tanks (15 fish per tank), and the size of each tank was approximately 0.18 m3 (110 cm×40 cm ×40 cm) with ~ 0.15 m3 water, and equipped with electric heaters and thermometers. After two weeks of acclimatization, the six tanks were divided into two groups: the control (CO) group and the acute heat stress (AH) group. During the experiment, the CO group was maintained at a water temperature of 16 ± 0.5°C, while the water temperature of the AH group was increased from 16°C to 28°C at a rate of 2°C/h and maintained for 12 h (Pérez-Casanova et al., 2008). The fish were fed with commercial feed (Rongchuan Feed Co. Ltd., Sichuan, China) twice daily at 9:00 and 18:00 during the experiment. Water quality parameters were measured using the water Quality tester AZ86031 (AZ Instrument Corp., Guangdong, China). They were as follows: pH 7.6-8.5, dissolved oxygen > 6.0 mg/L, ammonia nitrogen and nitrite concentration ≤ 0. 5 mg/L. The study protocol was approved by the Institutional Animal Care of the Southwest University, Chongqing, China, and was performed in compliance with the guidelines for the care and use of laboratory animals.
2.2 Sample collection
Nine fish (three per tank) were randomly selected from each experimental group and anesthetized with 40 mg/L MS-222 (Sigma, Shanghai, China) to collect blood and intestinal samples. Blood samples were collected using disposable syringes containing ethylenediaminetetraacetic acid (EDTA), centrifuged at 5,000 rpm for 15 min, and the supernatant was preserved as plasma for the detection of biochemical indicators. Based on the study of the digestive tract of G. eckloni (Yang et al., 2017), the intact intestine was removed after dissection and divided into the three sections (foregut, midgut, and hindgut). Since foregut has more microvilli and mitochondria and is more suitable for histological observations, two small segments of foregut were collected from each fish and preserved separately in 4% paraformaldehyde and 2.5% glutaraldehyde for histological and ultrastructural observations. Following the collection methods of previous studies (Zhu et al., 2022; Zhou et al., 2024), the midgut of nine fish from each experimental group was collected, divided into three replicates, and preserved in liquid nitrogen for transcriptome sequencing. The hindgut contents of the nine fish were carefully squeezed into centrifuge tubes, thoroughly mixed, divided into six replicate samples, and stored in liquid nitrogen for subsequent microbial DNA extraction.
2.3 Histological analysis
The obtained foregut was fixed in 4% paraformaldehyde for 24 h. The samples were dehydrated in a series of ethanol solutions (70%, 80%, 90% and 100%), embedded in paraffin, and sectioned to a thickness of 5 µm. These sections were stained with hematoxylin and eosin (H&E) according to the procedures outlined in previous studies (Novelli et al., 2015). Observation and photography were then conducted using a microscope (Olympus, Tokyo, Japan) equipped with a microscope imager (Leica, Wetzlar, Germany). Additionally, a total of 10 microscopic fields at 40 × magnification were randomly selected to determine the morphological index, including villus length, villus width, and muscle thickness, using ImageJ software (National Institutes of Health, Bethesda, USA).
Tissue sections for transmission electron microscopy (TEM) were prepared as previously described (He and Woods, 2004; Wu et al., 2008). Foregut samples were fixed in 2.5% glutaraldehyde for 4 h and in 4% osmium tetroxide for 1 h. They were then washed in PBS and dehydrated using a series of alcohol concentrations. The tissue was embedded in resin, and ultrathin sections were obtained using an ultrathin sectioning machine. These sections were stained with uranyl acetate and lead citrate and subsequently observed using a Hitachi H7650 TEM.
2.4 Detection and analysis of plasma biochemical indicators
The collected plasma samples were used to detect biochemical indicators of various reactions. The levels of the stress indicator cortisol, the intestinal permeability indicators diamine oxidase (DAO) and lipopolysaccharide (LPS), as well as the inflammatory factors interleukin 1β (IL-1β) and tumor necrosis factor-α (TNF-α), were measured using an enzyme labeling analyzer (Rayto RT-6100) and enzyme-linked immunosorbent assay (ELISA) kits (Jiancheng Bioengineering Institute, Nanjing, China).
Oxidative stress indicators, including superoxide dismutase (SOD), catalase (CAT), and glutathione peroxidase (GSH-Px), as well as metabolic indicators such as glucose (GLU), triacylglycerol (TG), and cholesterol (CHO), were detected using kits procured from ZCIBIO Technology Co., Ltd., China (A001-1, A007-1, A005, S03039, A110–1, and A111–1-1). Reactive oxygen species (ROS) were identified using fluorescent probe assay kits (ZC-A4108, ZCIBIO, Shanghai, China). All biochemical indicators were measured strictly in accordance with the operating instructions.
2.5 Transcriptome data and analysis
Total RNA was extracted from three replicate samples of the CO and AH groups using TRIzol reagent, following the instructions. The integrity, purity, and quality of the RNA were assessed using agarose gel electrophoresis, a Nanodrop microspectrophotometer assay (NanoDrop Technologies, Wilmington, USA), and an Agilent 2100 assay (Agilent Technologies, CA, USA). Six cDNA libraries were constructed using the Hieff NGS® Ultima Dual-mode mRNA Library Prep Kit (12309ES, Yeasen, Shanghai, China), and the library quality was evaluated with the DNA 1000 Assay Kit (5067-1504, Agilent Technologies, Beijing, China). The cDNA libraries were sequenced using the Illumina NovaSeq 6000 by Gene Denovo Biotechnology Co. (Guangzhou, China). After sequencing, the raw reads were quality controlled using Fastp (version 0.18.0) to filter low-quality data and obtain clean reads. The paired-end sequences were aligned to the reference genome (GenBank accession:JAMHKY000000000, https://www.ncbi.nlm.nih.gov/datasets/taxonomy/334712/) using HISAT 2 (Wang et al., 2022a). The expression level of each transcript was calculated using the reads per kilobase of exon per million mapped reads (RPKM) method using RSEM software. Differentially expressed genes (DEGs) were identified with a false discovery rate (FDR) < 0.05 and an absolute fold change ≥ 2 using DESeq2 software. In addition, functional enrichment analysis (GO and KEGG analysis) of the DEGs was performed, and terms or pathways with a corrected P value < 0.05 were considered significantly enriched. Pathway diagram is drawn by Figdraw.
To verify the reliability of the transcriptome data, we randomly selected seven DEGs—the heat shock protein 90 alpha family class A member 1 (hsp90aa1), the heat shock protein 70 (hsp70), the phosphoenolpyruvate carboxykinase 1 (pck1), the cold shock domain-containing protein C2-like (csdc2), the GTPase IMAP Family Member 7 (gimap7), the jumonji and AT-rich interaction domain containing 2 (jarid), and the (glutamate-ammonia ligase) glul—for quantitative reverse-transcription polymerase chain reaction (qRT-PCR) to obtain their expression profiles. The total RNA used was consistent with that used for RNA sequencing. cDNA synthesis and qRT-PCR were performed following the manufacturer’s protocols, utilizing the HiScript III All-in-one RT SuperMix Perfect Kit for qPCR and ChamQ Universal SYBR qPCR Master Mix (Vazyme, Nanjing, China). All reactions were performed in three biological replicates and three technical replicates, with β-actin serving as the reference gene. Primer sequences for the seven DEGs and the reference gene are detailed in Supplementary Table 1. Relative expression in the qPCR results was calculated using the 2-ΔΔCt method, and statistical significance was determined by one-way analysis of variance (ANOVA) (Livak and Schmittgen, 2001).
2.6 Intestinal microbiological data and analysis
Six content samples (n = 6) were collected from each experimental group, and DNA was extracted using HiPure Stool DNA Kits (Magen, Guangzhou, China). The purity and integrity of nucleic acids were verified using a NanoDrop microspectrophotometer (Thermo, Massachusetts, USA) and agarose gel electrophoresis (Liuyi Biotechnology Co. Ltd., Beijing, China). The V3 to V4 regions of the 16S rDNA gene were amplified, purified, and sequenced on the NovaSeq 6000 platform after quality assessment. The primers used were 341F (5′-CCTAYGGGRBGCASCAG-3′) and 806R (3′-GACTACNNGGGGTATCTAAT-5′). Raw data underwent quality control and chimera filtering, and operational taxonomic units (OTUs) were obtained using UPARSE (version 9.2.64) clustering. The representative OTU sequences were classified into organisms using a naive Bayesian model, and the species composition was analyzed at the phylum and genus levels. A Venn diagram was used to identify unique and shared OTUs between the CO and AH groups. Alpha diversity analyses were performed using QIIME2 software (version 1.9.1) to calculate Chao1, ACE, Shannon, and Simpson indices. Based on OTUs and species abundance tables, the Bray–Curtis algorithm was used to evaluate the beta diversity of all samples, including principal component analysis (PCA) based on out, unweighted Unifrac Distance-based Principal Coordinate analysis (PCoA), and Binary-Jaccard-based non-metric multidimensional scaling (NMDS) analysis. Functional prediction of OTUs and KEGG pathway analysis were performed using Tax4Fun (version 1.0). Biomarker features in each group were identified using LEfSe software (version 1.0).
2.7 Integrated analysis of the microbiome and transcriptome
Pearson correlation analyses were performed to examine the relationship between the intestinal microbiota and DEGs. On the basis of the transcriptome results, we focused on the major DEGs in the hypoxia-inducible factor-1 (HIF-1) signaling pathway, as well as immune-related DEGs. Heat maps and correlation network diagrams were generated using the Omicsmart platform (http://www.omicsmart.com).
2.8 Statistical analysis
All data are presented as mean ± SD. Data were processed and plotted using SPSS 26.0 (SPSS Inc., Chicago, USA) and GraphPad Prism 9.5 (GraphPad Software Inc., CA, USA). Differences between the CO and AH groups were determined using a t-test. A significance level of P < 0.05 was considered statistically significant, P < 0.01 was considered highly significant, and P < 0.001 was considered extremely significant.
3 Results
3.1 Effects of acute heat stress on intestinal organization and structure
The histology and ultrastructure of the foregut demonstrated changes in intestinal organization and structure following acute heat stress. In the CO group, the intestinal histology of G. eckloni appeared normal, with an intact mucosal layer and goblet cells (Figure 1A). After acute heat stress (Figure 1B), some obvious pathological symptoms indicative of intestinal damage were observed, including numerous swollen goblet cells, vacuoles in the lamina propria, localized necrosis of the mucosal layer, and detached cells in the intestinal cavity. Measurements of the midgut’s morphological indices revealed a significant decrease in villus length and width, along with a significant increase in muscle thickness in the AH group (Figure 1C). The ultrastructure of the intestine in the CO group showed well-arranged microvilli and clearly visible, structurally intact mitochondria (Figures 2A, B). However, in the AH group (Figures 2C, D), the microvilli structure was ruptured, leading to breakage and detachment. Moreover, the mitochondria exhibited notable swelling, cristae dissolution, and even vacuolization, resulting in mitochondrial abnormalities. Therefore, both histological and ultrastructure analyses indicated that the intestinal organization and structure of G. eckloni were damaged following acute heat stress.
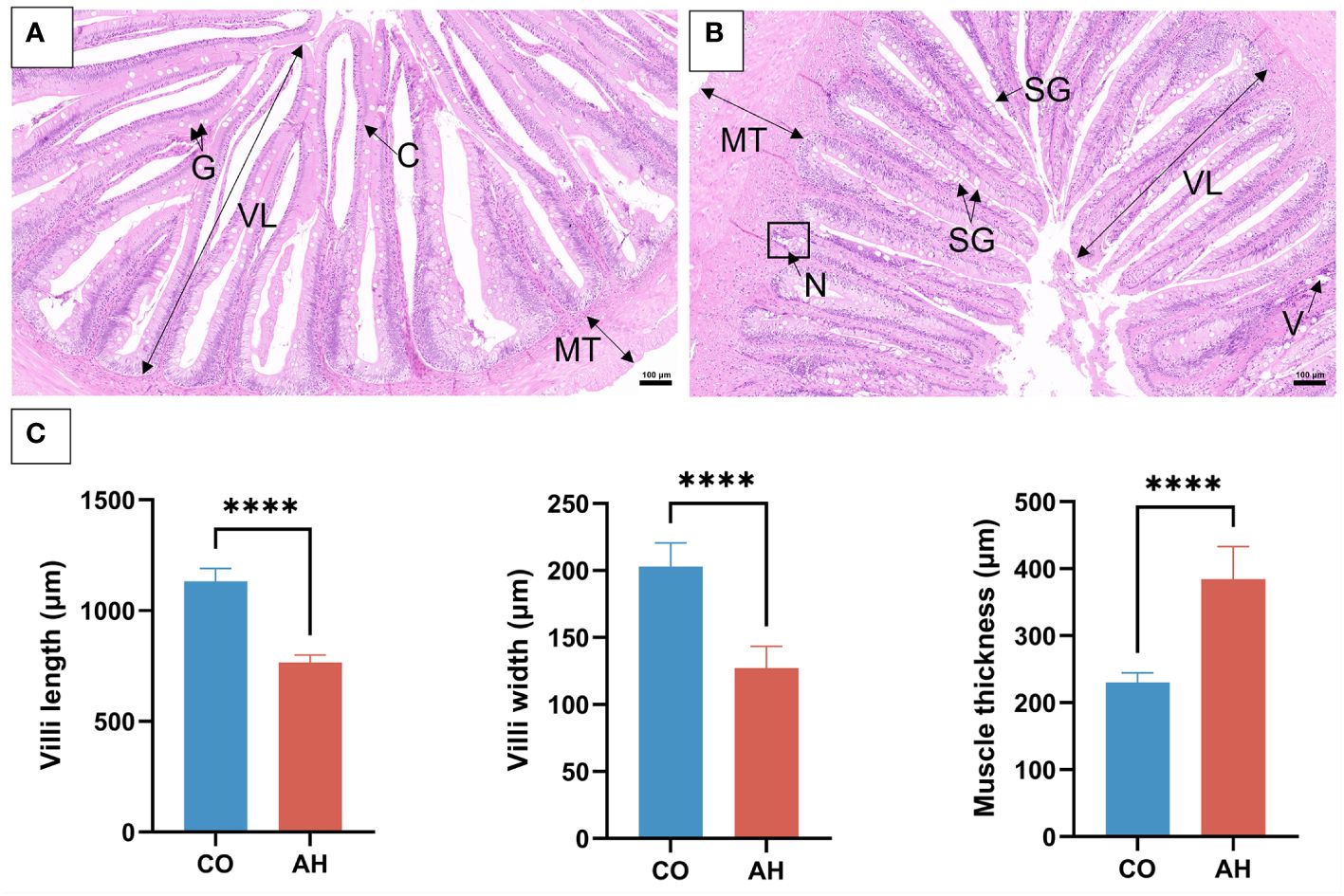
Figure 1 Histological changes in the intestine after acute heat stress. (A): CO group. (B): AH group. (C): The intestine morphological index measurement. G, goblet cell; C, columnar cells; N, necrosis; V, vacuolization; SG, swollen goblet cell; lV, villi length; MT, muscle thickness. Scale bar: 100 μm. “****” represents P<0.0001.
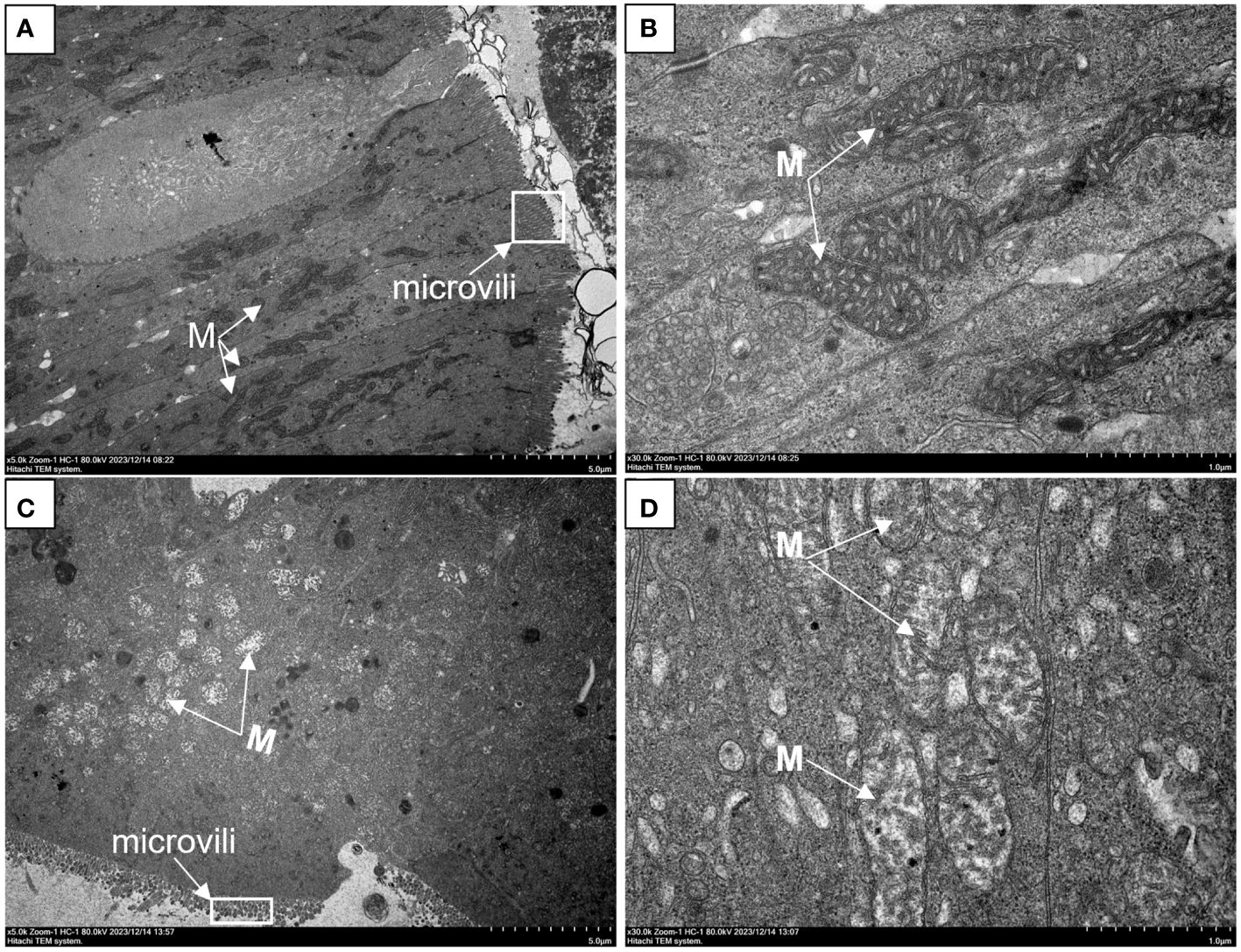
Figure 2 Changes in the intestinal ultrastructure of G eckloni in the CO group compared to the AH group. (A, B): CO group. (C, D): AH group. M: mitochondria.
3.2 Effects of acute heat stress on plasma biochemical indicators
The effect of acute heat stress on the plasma biochemical indices of G. eckloni is illustrated in Figure 3. The levels of ROS, a marker of oxidative damage, were significantly higher after heat stress compared to the CO group (P < 0.05). Among the several antioxidant enzymes studied, a highly significant increase was observed in the activity of SOD (P < 0.01), a highly significant decrease in the activity of CAT (P < 0.01), and no significant change in the activity of GSH-Px.
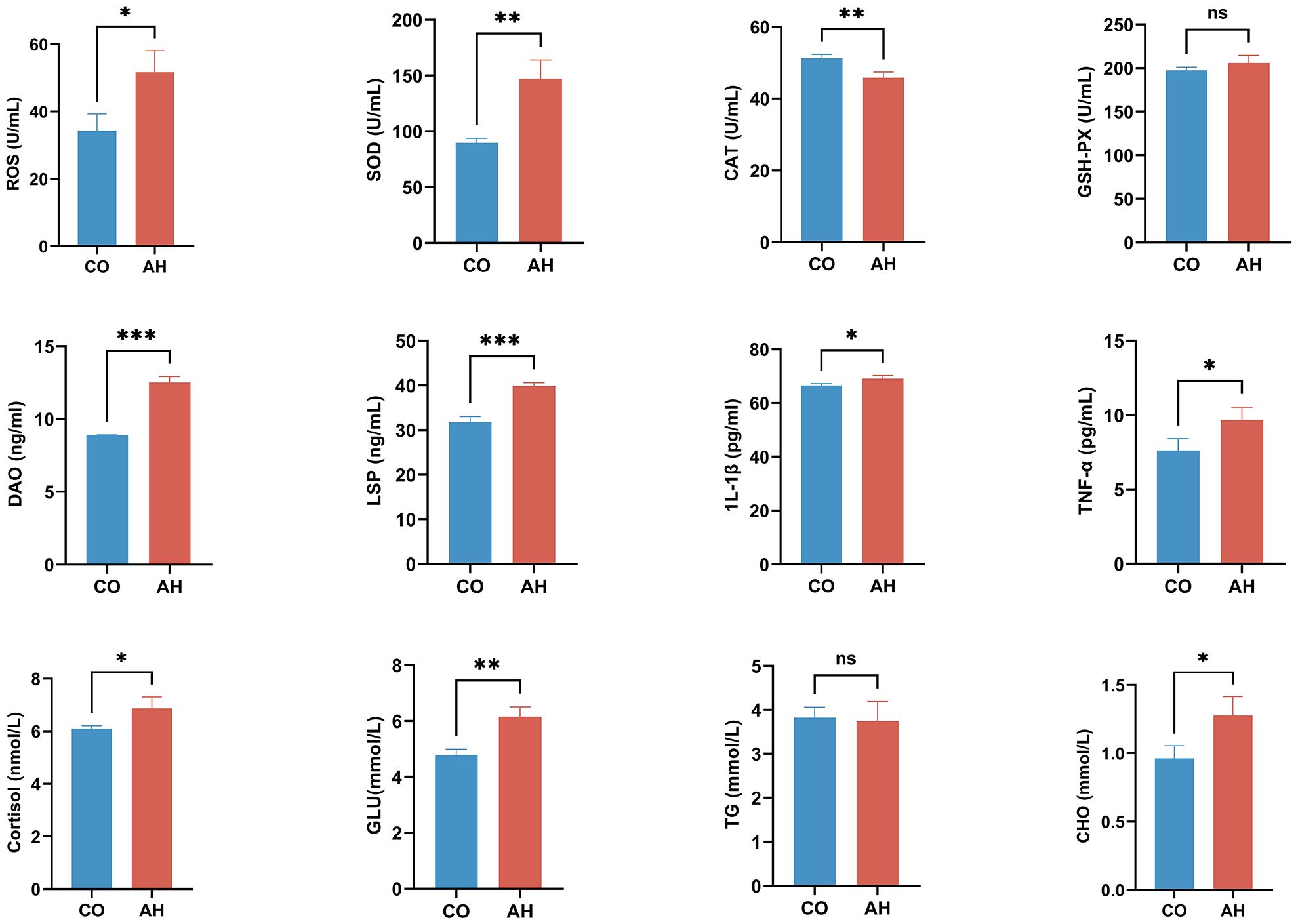
Figure 3 Effect of acute heat stress on plasma biochemical indicators. Statistical significance was defined as *P < 0.05, **P < 0.01, and ***P < 0.001. “ns”, not significant.
Compared with those in the CO group, plasma DAO and LPS levels were markedly increased (P < 0.001), indicating heightened intestinal permeability following acute heat stress. The levels of pro-inflammatory factors (IL-1β and TNF-α) and the stress indicator cortisol were also significantly elevated (P < 0.05), suggesting an inflammatory response in the intestine of G. eckloni owing to increased stress under acute heat stress. In terms of energy metabolism, a highly significant increase was observed in plasma glucose levels (P < 0.01), as well as a significant increase in cholesterol (P < 0.05), while triglyceride levels showed no significant change.
3.3 Transcriptome data and analysis
The CO and AH groups assembled six transcriptome libraries and obtained a total of 24,250,5574 raw reads. Following low-quality filtering, a total of 24,111,06070 clean reads were obtained, ranging from 4,780,859,426–8,961,080,914. The Q20 and Q30 values were above 97.00% and 94.00%, respectively, indicating the viability of the sequencing data (Supplementary Table 2). Differential expression analysis revealed a total of 786 DEGs after acute heat stress compared to controls, with 535 DEGs upregulated and 251 DEGs downregulated (Figures 4A, B).
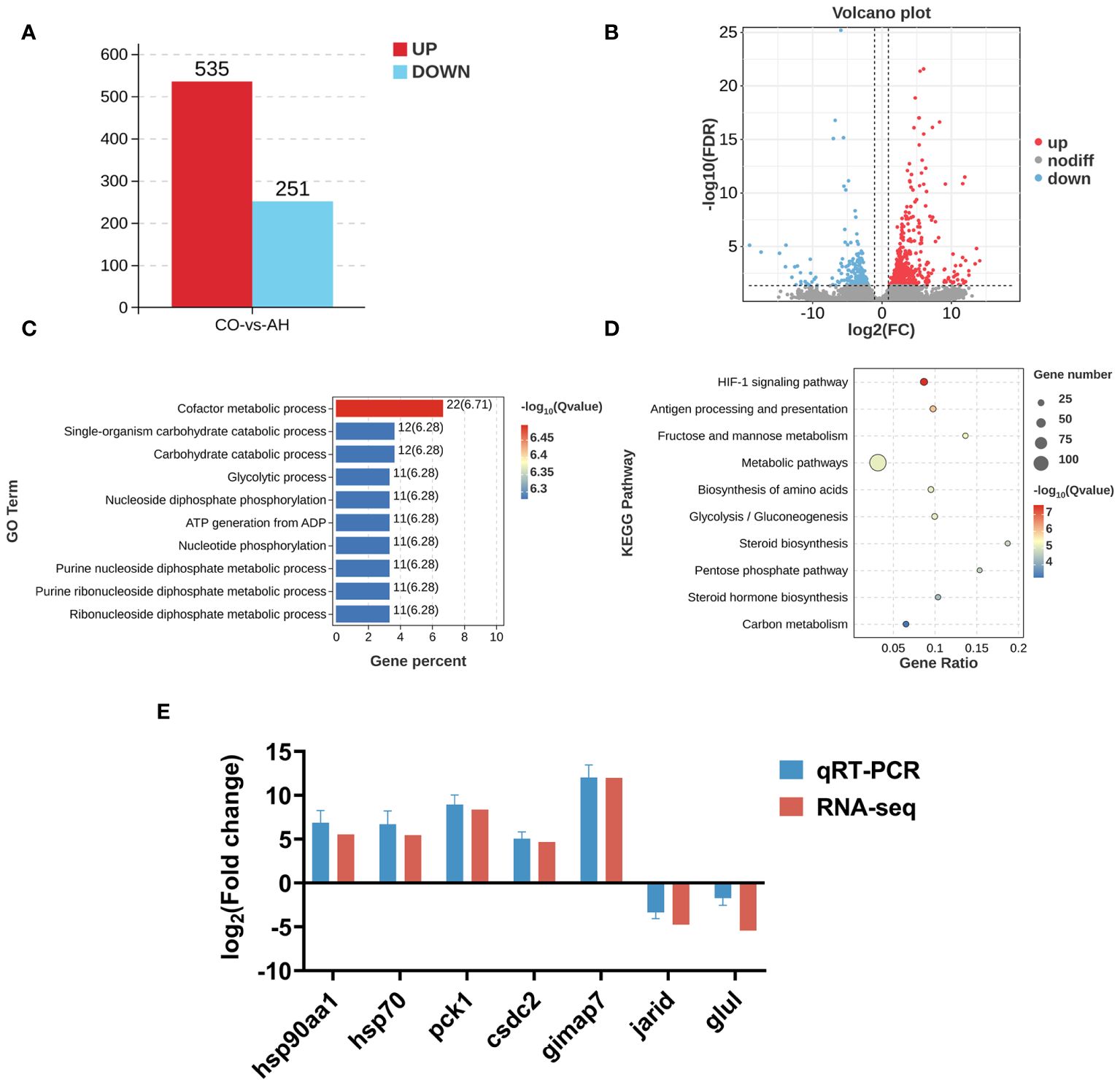
Figure 4 Effect of acute heat stress on the transcriptome. (A): The numbers of DEGs between the CO and AH groups. (B): A volcano map. (C): A bar chart of the top 10 significantly enriched GO terms. (D): A bubble diagram of the top 10 significantly enriched KEGG pathways. (E): Validation of qPCR.
The detailed GO terms and the top 10 significantly enriched GO terms are shown in Supplementary Figure 1; Figure 4C. A total of 41 GO terms were enriched in the comparison between the CO and AH groups. Within the molecular function category, most DEGs were enriched for binding and catalytic activity. Regarding biological processes, cellular processes and metabolic processes were the most represented. The top 10 significantly enriched terms were associated with metabolic processes, including cofactor metabolic processes, carbohydrate catabolic processes, glycolytic processes, nucleoside diphosphate phosphorylation, nucleotide phosphorylation, and purine nucleoside diphosphate metabolic processes.
KEGG enrichment analysis revealed that DEGs in the “CG vs. CH” comparison were significantly enriched in 25 pathways (q < 0.05). The top 10 pathways included the HIF-1 signaling pathway, antigen processing and presentation, fructose and mannose metabolism, metabolic pathways, biosynthesis of amino acids, glycolysis/gluconeogenesis, steroid biosynthesis, pentose phosphate pathway, steroid hormone biosynthesis, and carbon metabolism (Figure 4D). Changes of DEGs in the HIF-1 signaling pathway were shown in Figure 5.
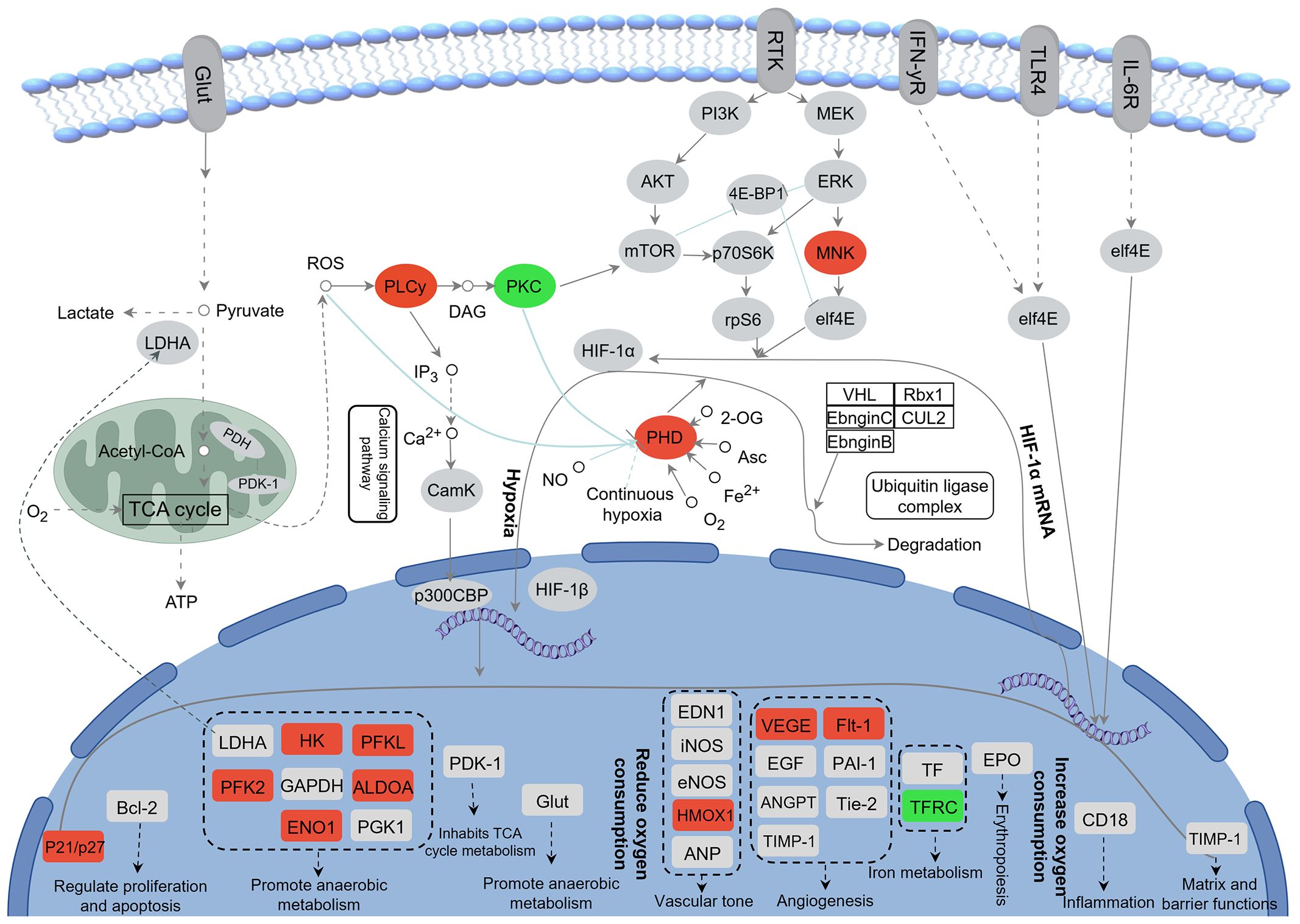
Figure 5 Changes of DEGs in HIF-1 signaling pathway after acute heat stress. Red color represents up-regulation of gene expression and green color represents down-regulation of gene expression.
Furthermore, to validate the accuracy of the transcriptome data, we randomly selected seven genes for qPCR, including hsp90aa1, hsp70, pck1, csdc2, gimap7, jarid, and glul. The qPCR data exhibited a high degree of consistency with the transcriptomic profiles (Figure 4E), indicating that the transcriptome results are reliable and credible.
3.4 Intestinal microbiological data and analysis
A total of 850,182 high-quality clean reads were obtained from 16S rDNA amplicon gene sequencing in all intestinal content samples, with a mean number of clean reads per sample of 70,849. Venn diagram analysis elucidated a total of 1,690 OTUs in the CO and AH groups, of which 601 were CO group-specific OTUs and 824 were AH group-specific OTUs (Figure 6C). Species composition analysis showed that the major bacterial phylum in all groups was Proteobacteria, followed by Fusobacteriota and Firmicutes (Figure 6A). At the genus level, Aeromonas, Shewanella, Citrobacter, Acinetobacter, and Hafnia-Obesumbacterium were the dominant species in the intestinal contents of G. eckloni (Figure 6B). Following acute heat stress, the percentage of Aeromonas increased from 45.69% to 46.86%, Shewanella increased from 1.31% to 17.16%, Citrobacter decreased from 23.43% to 1.73%, Acinetobacter decreased from 16.99% to 4.79%, and Hafnia-Obesumbacterium increased from 0.52% to 10.08%.
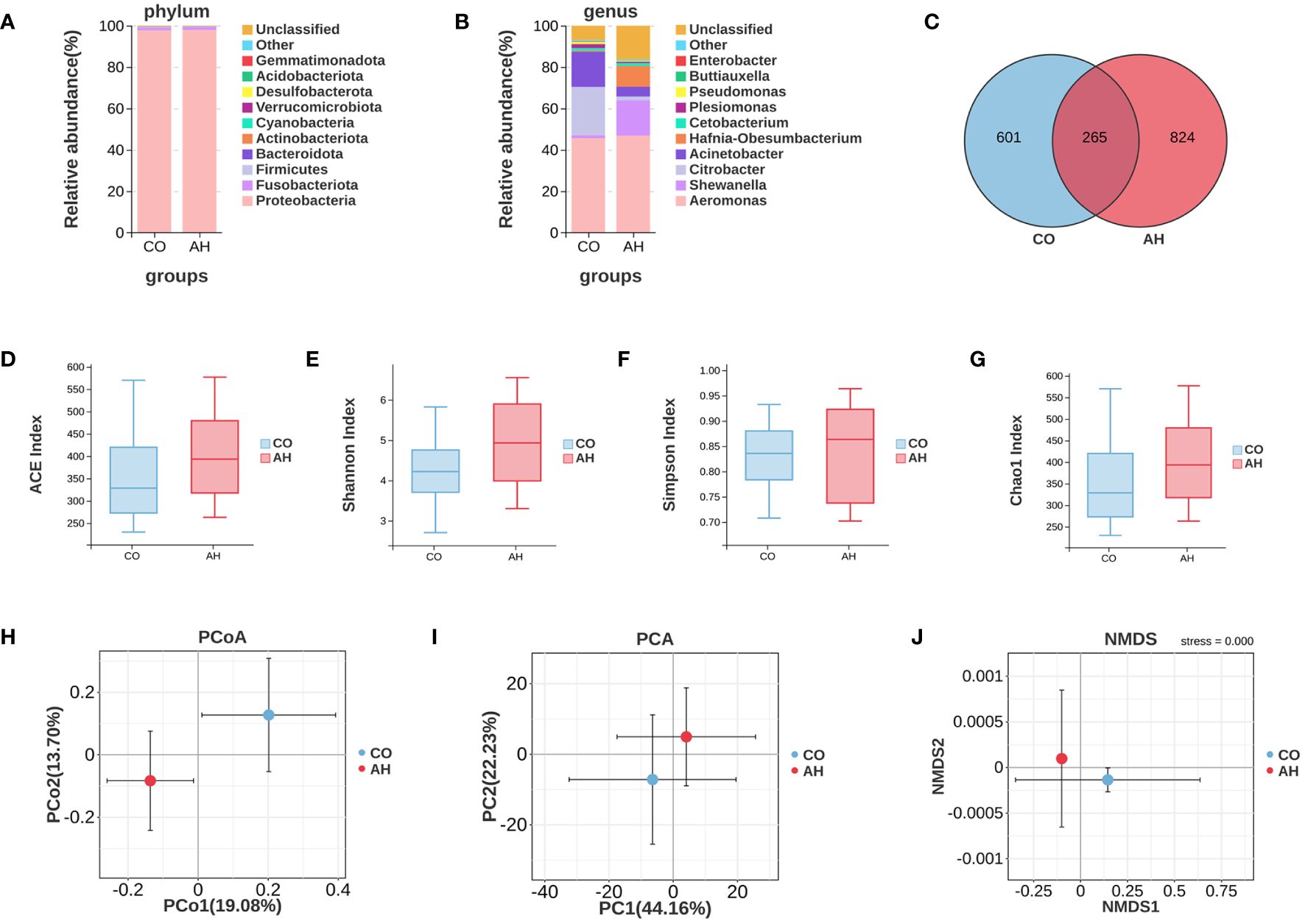
Figure 6 Effects of acute heat stress on the composition and diversity of the intestinal microbiota. (A): A Venn diagram depicting OTUs. (B, C): The average relative abundances of intestinal microbiota at phylum and genus levels. (D–G): Analysis of alpha diversity of the intestinal microbiota. (H-J): Analysis of beta diversity of the intestinal microbiota.
The alpha diversity analysis (Figures 6D-G) revealed that the differences in diversity indices, including Chao1, Shannon, Simpson, and ACE, between the CO and AH groups were not statistically significant, indicating that acute heat stress had a minimal effect on the diversity of the intestinal microbiota in G. eckloni. In contrast, the beta diversity analysis (Figures 6H-J) showed clear separation between the CO and AH groups on the two-dimensional coordinate plots of PCoA, PCA, and NMDS, suggesting that acute heat stress significantly affected the composition of the intestinal microbiota in G. eckloni.
Tax4Fun was employed for predictive analysis of the intestinal microbiota’s function (Figure 7A). The results indicated that the functions of the intestinal microbiota of G. eckloni were primarily focused on carbohydrate metabolism, membrane transport, amino acid metabolism, and signal transduction. LEfSe analysis elucidated biomarkers with significant differences between the two groups (Figures 7B, C). In total, 11 strains of differential microflora were identified in the CO and AH groups, with Shewanella sp., Lachnospira multipara, and Peptostreptococcaceae serving as biomarkers in the AH group.
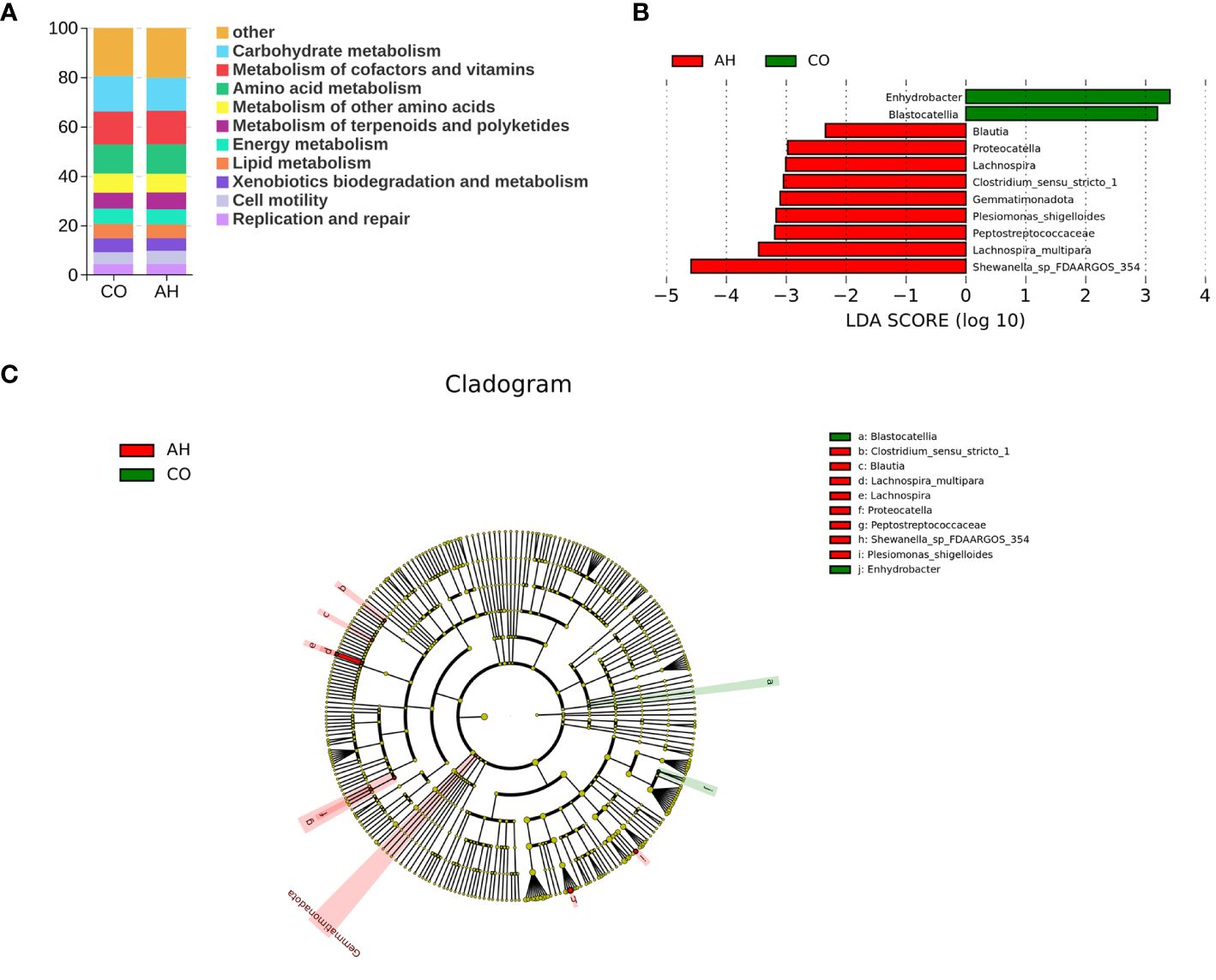
Figure 7 Intergroup variation in the relative abundance of the intestinal microbial communities. (A): Function prediction analysis of the intestinal microbiota using PICRUSt2. (B): LDA score at the genus level from Lefse-PICRUSt. (C): Cladogram from Lefse.
3.5 Integrated analysis of the microbiome and transcriptome
The potential relationship between the intestinal microbiota and DEGs was analyzed using the Pearson correlation method. On the basis of the transcriptome results, we focused on the major DEGs in the HIF-1 signaling pathway and immune-related DEGs. The heatmaps and network diagrams depicting the correlation between gene expression and the intestinal microbiota are presented in Figure 8. The results indicated that the heat shock protein family (hsp70, hsp90aa1, and hspa4) showed a strong positive correlation with the abundance of Hafnia-Obesumbacterium, Buttiauxella, and Cetobacterium. In the HIF-1 signaling pathway, the expression of the transferrin receptor (trfc) and the protein kinase C, beta b (prkcbb) was positively correlated with Pseudomonas abundance, while the expression of the phosphofructokinase, platelet (pfkp) was negatively correlated. In the antigen presentation and processing pathway of the immune system, changes in the expression of the major histocompatibility complex, class II, DP alpha 1 (hla_dpa1), the major histocompatibility complex, class II, DP beta 1 (hla_dpb1), the major histocompatibility complex, class II, DQ beta 2 (hla_dqb2), and mica were positively correlated with the abundance of Pseudomonas, Streptococcus, or Plesiomonas. Conversely, the expression of IL1β was negatively correlated with Pseudomonas abundance.
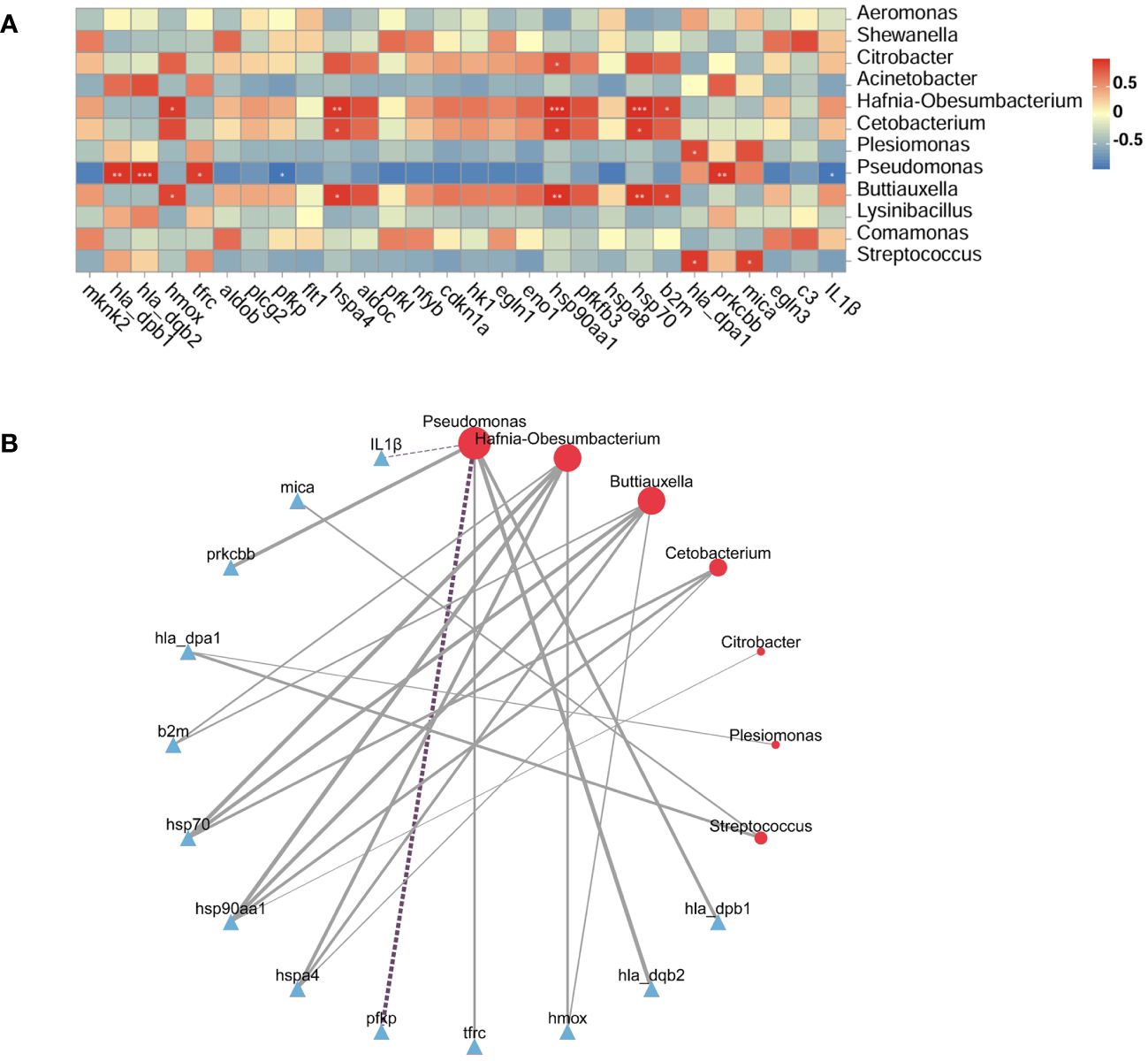
Figure 8 Correlation between the intestinal microbiota and DEGs. (A): Correlation heatmap. The X-axis represents the selected DEGs, the Y-axis represents the microbiota, and the color indicates the strength of the correlation. Statistical significance was defined as *P < 0.05, **P < 0.01, and ***P < 0.001. (B): Network diagram: Full lines denote positive correlations and dotted lines denote negative correlations. Line thickness represents the strength of the correlation.
4 Discussion
4.1 Effects of acute heat stress on intestinal organization and structure of G. eckloni
The intestine is the central organ for digestion and absorption, and it plays a crucial barrier function against the environment (Vancamelbeke and Vermeire, 2017). Previous studies have found significant structural changes in the intestine under stressful conditions, particularly in the villi and goblet cells, in species such as Oncorhynchus mykiss (Li et al., 2023a), Crassostrea gigas (Zhao et al., 2023), and Nile tilapia (Ran et al., 2016). Villus length influences the body’s ability to absorb nutrients by increasing the surface area of the villi (Wei et al., 2024). Goblet cells secrete mucus for lubrication, protect the intestine, and resist bacteria (Johansson and Hansson, 2016). In addition, muscular thickness is an important indicator of intestinal health, with increased thickness promoting intestinal motility (Sanders et al., 2012). In our study, we observed shortening of villus length, swelling of goblet cells, and increased thickness of the muscularis propria in the intestinal histology of G. eckloni after heat stress, indicating that acute heat stress impaired the intestinal health of G. eckloni and caused barrier damage, similar to the observations in Oxygymnocypris stewarti (Zhu et al., 2022). Following heat stress, the intestinal ultrastructure of G. eckloni exhibited notable damage, including microvilli detachment and mitochondrial structural abnormalities. Microvilli are the final site for digestion and absorption of nutrients, and defects in microvilli can affect intestinal function and cause diseases (Pavelka and Roth, 2010; Orbach and Su, 2020). Following high-temperature stress in oysters, the shortening and shedding of microvilli impede intestinal nutrient absorption (Zhao et al., 2023). Mitochondria, as the main organelles providing energy, are also the primary site of oxidative stress (Munro and Treberg, 2017). We observed significant swelling, cristae lysis, and even vacuolization of mitochondria, suggesting that intestinal damage in G. eckloni is closely associated with oxidative stress and that mitochondrial damage may result from a lack of adequate energy supply (Zhou et al., 2023). Thus, acute heat stress can cause damage to the intestinal morphology and structure of G. eckloni, subsequently affecting its digestion and absorption abilities.
4.2 Effects of acute heat stress on the physiological responses of G. eckloni
Generally, high temperatures induce oxidative stress in body tissues (Blier, 2014). To avoid oxidative damage caused by excess ROS generated by oxidative stress, the activation of antioxidant enzymes in the antioxidant defense system plays a dominant role (Cheng et al., 2018). In this study, following acute heat stress, a significant increase in the plasma level of ROS was observed in G. eckloni, potentially inducing changes in antioxidant enzymes. We examined the changes in several antioxidant enzymes, including CAT, SOD, and GSH-Px, and found that only the level of SOD increased significantly. This indicates that under acute heat stress, G. eckloni mitigates the harmful effects of ROS and alleviates oxidative damage by increasing the activity of SOD, similar to Labeo rohita (Kumar et al., 2011) and Litopenaeus vannamei (Al-Masqari et al., 2022). The level of CAT was significantly reduced, which may be associated with excess ROS exceeding the threshold of antioxidant enzymes, leading to enzyme degradation or inactivation (Zhang et al., 2008; Chen et al., 2021). No significant changes in GSH-Px were observed, which we speculated was due to the low sensitivity of GSH-Px to oxidative damage (Li et al., 2022). Previous studies have provided a rationale for these phenomena, noting that the duration of heat stress, fish species, or compensatory mechanisms can all lead to differences in the activation of antioxidant enzyme systems (Wu et al., 2021). In addition, with disruption in the balance between the oxidative-antioxidant system and inflammatory cytokines, oxidative damage may further affect fish immunity, leading to an inflammatory response (Slimen et al., 2014; Dang et al., 2022). This study showed that the pro-inflammatory cytokines IL-1β and TNF-α were significantly increased, indicating that there may be inflammation in the intestine of G. eckloni after acute heat stress, and that the changes in inflammatory cytokines are associated with intestinal mucosal injury (Konturek et al., 2011). Intestinal inflammation is often accompanied by permeability dysfunction (Lambert, 2009). Plasma LPS and DAO levels were extremely significantly elevated in the AH group, suggesting that intestinal permeability increases after acute heat stress, making it easier for harmful substances to infiltrate and cause systemic inflammation (Chelakkot et al., 2018). Therefore, this study suggests that acute heat stress affects intestinal homeostasis and barrier function in G. eckloni.
The metabolic demands of fish increase in response to stress (Przepiura et al., 2019). Stress activates the hypothalamic-pituitary-adrenal axis, leading to an increase in cortisol, an indicator of the stress response, which is accompanied by an increase in energy expenditure (Montero et al., 1999; Faheem et al., 2022). In Wuchang bream (Ming et al., 2012), Lateolabrax maculatus (Qin et al., 2023), and Epinephelus akaara (Cho et al., 2021), elevated cortisol levels enhance gluconeogenesis and glycogenolysis to meet the heightened energy demand due to heat stress. In this study, plasma cortisol and glucose levels were significantly increased in G. eckloni following acute heat stress, suggesting that gluconeogenesis accelerates in response to the increased metabolic demand to resist heat stress. In addition, CHO content was significantly increased in the AH group, suggesting the promotion of lipid metabolism to fulfill the energy demand following heat stress in G. eckloni, similar to the response observed in rainbow trout (Lu et al., 2022).
4.3 Effects of acute heat stress on the intestinal transcriptome of G. eckloni
Transcriptome sequencing revealed that regulatory pathways and key genes are affected by acute heat stress. In this study, DEGs were predominantly and significantly enriched in the HIF-1 signaling pathway in the comparison between the CO and AH groups. The HIF signaling pathway is a crucial metabolic regulatory pathway for maintaining organismal stability under hypoxic conditions. This pathway is involved in vascular development, the transcription of genes associated with vascular development, glucose metabolism, immune function, and cell growth and survival (He et al., 2019; Lin et al., 2021). Previous studies have found that the activation of the HIF-1 pathway may be associated with oxidative stress (Zhang et al., 2023), and it has been validated to play a vital role in the heat adaptation mechanism (Treinin et al., 2003). In addition, compared with other organs, the oxygen concentration in the intestine is lower. The increase in oxygen consumption and blood redistribution after heat stress reduces the energy and oxygen supply to intestinal epithelial cells, which may lead to pathological hypoxia in the intestine (Albenberg et al., 2014; Taylor and Colgan, 2017; Li et al., 2024a). The HIF-1 pathway determines the stability of HIFs during hypoxia by influencing the activity of proline hydroxylase (Egln) through various factors and can regulate downstream genes to help cells adapt to hypoxia (Luo et al., 2022; Strocchi et al., 2022). Meanwhile, excess ROS production induces phospholipase Cγ (PLCγ), leading to inflammation (Tsai et al., 2022). Protein kinase C (PKC) is recognized as a potential mediator of gene expression induced by inflammatory stimulation. The inhibition of PKC may play a role in HS-induced myocardial protection and the prevention of intestinal epithelial dysfunction (Wu et al., 2003; Arnaud et al., 2004; Yang et al., 2007). In our experiments, the expression of egln, plcg1, and plcg2 was significantly upregulated, while the expression of PKC-encoded genes (prkac, prkcb, and prkcg) was significantly downregulated. We hypothesize that hypoxia occurs in the intestine of G. eckloni, inducing inflammation after heat stress, and that the HIF-1 pathway is activated to protect the intestine. Activation of the HIF-1 pathway also regulates its downstream target genes, affecting oxygen delivery (by regulating angiogenesis and remodeling) and oxygen utilization (by regulating glucose metabolism and oxidation-reduction) (Baptista et al., 2016). Following acute heat stress, the expression of glycolysis-related genes (hk, pfka, aldo, eno, and pfkfb3) is upregulated in the intestine of G. eckloni, increasing the rate of glycolysis to sustain energy production and reducing hypoxia-induced ROS production (Deng et al., 2022). Additionally, the upregulation of vascular endothelial growth factor A (vegfa) and its receptor flt1 induces angiogenesis, enhancing oxygen transport (Kim et al., 2021). Haem oxygenase 1 (hmox1) is considered an antioxidant with many anti-inflammatory properties (Campbell et al., 2021), and its expression is also upregulated in response to oxidative stress and inflammation. Thus, the HIF-1 signaling pathway is an important regulatory mechanism during acute heat stress in the intestine of G. eckloni.
Additionally, we found that many genes were significantly enriched in immune system pathways, including antigen processing and presentation, the IL-17 signaling pathway, complement and coagulation cascades, and the hematopoietic cell lineage. The immune system is mainly divided into innate and adaptive immunity (Furuta and Eguchi, 2021).The primary role of heat shock proteins (HSPs) under heat stress conditions is to prevent protein misfolding and accumulation. This function contributes to both peptide-specific (adaptive) and non-specific (innate) components, which can trigger the activation of the immune system and stimulate immune responses (Srivastava, 2002; Park et al., 2021). In our experiments, the expression of hsp90aa1 and hsp70 was significantly upregulated in antigen presentation and processing, suggesting that these proteins may induce adaptive immunity through antigen presentation (Xu et al., 2018). Two major classes of glycoproteins responsible for antigen presentation, MHC I and MHC II, are essential for T cell-mediated adaptive immunity (Cruz-Tapias et al., 2013). However, we found that the expression of genes encoded by MHC I and MHC II (mica, hla-dpa1, hla-dpb1, and hla-dqb2) was downregulated after acute heat stress. This downregulation may be due to suppression by inflammation, similar to findings in the study of Micropterus salmoides (Yang et al., 2022a). This finding is also supported by the upregulation of proinflammatory cytokine expression, including IL-1B, and the increased enzymatic activity of IL-1β and TNF-α. The complement system is the primary line of defense in innate immunity and serves as a crucial barrier against pathogens (Parra-Medina et al., 2013). C3 is central to multiple complement pathways and is known as an evolutionarily conserved building block of immunology, coordinating immune responses through the detection and elimination of hazards (Ricklin et al., 2016). C3 expression following inflammation in zebrafish has been found to regulate the quality and magnitude of innate immune responses to various inflammatory stimuli (Dodds and Matsushita, 2007). In this study, the expression of c3 in the intestine of G. eckloni was upregulated, indicating that G. eckloni can respond to heat stress by upregulating c3. Thus, we found that acute heat stress significantly induces intestinal inflammation and affects immune function in G. eckloni.
4.4 Changes in the intestinal microbiota of G. eckloni under acute heat stress
The intestinal microbiota plays a crucial role in maintaining host health and regulating various physiological functions, particularly nutrient metabolism and immune defense (Jin et al., 2017). A healthy and stable intestinal microbiota produces beneficial components that help the host resist pathogen infections and maintain the structural integrity of the intestinal barrier (Song et al., 2024). However, under deleterious conditions, disturbances in the intestinal microbiota can lead to intestinal diseases and reduced immune function (Zhang et al., 2020). Intestinal microbiota may also serve as biomarkers of stress response (Llewellyn et al., 2014). Previous studies have shown that heat stress can significantly affect the composition and abundance of microflora, disrupting intestinal homeostasis and immune barriers in aquatic animals such as Eriocheir sinensis (Li et al., 2023c), Penaeus vannamei (Song et al., 2024), Micropterus salmoides (Yu et al., 2023), and Crassostrea gigas (Li et al., 2023b). The intestinal microbiota of G. eckloni changed significantly at the genus level after acute heat stress. In Proteobacteria, the abundance of Shewanella, Hafnia-Obesumbacterium, and Buttiauxella increased after acute heat stress, whereas the abundance of Citrobacter, Acinetobacter, Plesiomonas, Pseudomonas, and Enterobacter decreased. In Oncorhynchus mykiss (Zhou et al., 2022), a decrease in the abundance of multiple microbiota under acute heat stress can affect the diversity of intestinal microbes and even disrupt homeostasis. Shewanella, which is composed of Gram-negative Proteobacteria, increases significantly as a pathogen under harmful conditions, causing intestinal infection or disease in fish or crustaceans (Lemaire et al., 2020; Yu et al., 2022), such as Cyprinus rubrofuscus, Procambarus clarkii and Nile tilapia (Ruan et al., 2022; Reda et al., 2024; Rich and Naguib, 2024). Meanwhile, Hafnia-Obesumbacterium plays an important role in enteritis and intestinal infections (Janda and Abbott, 2006). Citrobacter assists in the clearance of intestinal pathogens and stimulates host immune defense (Sonnenburg et al., 2006; Buffie and Pamer, 2013; Guo et al., 2021). In this study, acute heat stress significantly altered the intestinal microbiota of G. eckloni, with a significant increase in the abundance of Shewanella and Hafnia-Obesumbacterium and a decrease in the abundance of Citrobacter. This is similar to a recent study in which chronic heat stress in G. eckloni resulted in a severe dysbiosis of the intestinal flora, characterized by an increase in Aeromonas and a decrease in Citrobacter (Zhou et al., 2024). Based on these findings, it is reasonable to hypothesize that acute heat stress leads to ecological imbalance of intestinal microbiota and intestinal inflammation in G. eckloni. We also observed an increasing trend in the abundance of Cetobacterium in Fusobacteriota. This increase can promote inflammatory pathways and is associated with immunosuppression (Huang et al., 2022). Therefore, acute heat stress may induce inflammation in the intestine of G. eckloni by altering the intestinal microbiota, adversely affecting immune function.
A robust interdependence exists between the intestinal microbiota and DEGs, with gene expression possibly mediating the link between microbial communities and host functions (Wang et al., 2022b; Liu et al., 2023). To further elucidate the host-microbiota relationship, we focused on the HIF-1 signaling pathway and immune-related DEGs in relation to the intestinal microbiota, using Pearson correlation analysis based on transcriptome results. We found that Hafnia-Obesumbacterium, Buttiauxella, and Cetobacterium exhibited robust positive correlations with heat shock proteins (hsp90aa1, hsp70, and hspa4). Additionally, Pseudomonas was associated with downstream target genes (prkcbb, tfrc, hmox, and pfkp) in the HIF-1 signaling pathway. These findings reveal significant interactions between the intestinal transcriptome and microbiota and suggest that the HIF-1 signaling pathway is an important regulatory pathway in the intestine of G. eckloni under acute heat stress. Pseudomonas, as a beneficial bacterium, helps balance the intestinal microbiota and immune system (Ruan et al., 2022). Significant correlations between Pseudomonas and immune DEGs (IL1β, hla_dqb2, and hla_dqb1) suggest that changes in Pseudomonas abundance significantly impact intestinal immune function in G. eckloni after acute heat stress. In this study, both Pseudomonas abundance and adaptive immunity-related genes (hla_dqb2 and hla_dqb1) were reduced, leading us to hypothesize that acute heat stress affects adaptive immunity in the intestines of G. eckloni and reduces immune function.
5 Conclusion
In this study, we evaluated the effects of acute heat stress on the intestine of G. eckloni using histological analysis, plasma biochemical indicators, transcriptomics, and 16S rDNA methods. The results indicated that acute heat stress induces damage to the intestinal morphology and structure of G. eckloni. Furthermore, biochemical indicators indicated that acute heat stress induced significant oxidative stress and inflammatory responses, affecting intestinal permeability and barrier function. Transcriptome analysis revealed that the HIF-1 signaling pathway is a crucial regulatory pathway during acute heat stress in the intestine of G. eckloni, while the expression of adaptive immunity genes (mica, hla-dpa1, hla-dpb1, and hla-dqb2) was significantly downregulated. This further suggests that acute heat stress causes intestinal inflammation and affects immune defense function. Furthermore, the composition of the intestinal microbiota was significantly altered at the genus level, affecting intestinal homeostasis. Changes in the abundance of the intestinal microbiota were strongly associated with changes in gene expression in response to stress, inflammation, and immunity. These findings provide novel avenues for ameliorating high-temperature stress in the artificial culture of G. eckloni.
Data availability statement
The datasets presented in this study can be found in online repositories. The names of the repository/repositories and accession number(s) can be found below: https://www.ncbi.nlm.nih.gov/genbank/, PRJNA1121983.
Ethics statement
The animal study was approved by Institutional Animal Care of the Southwest University, Chongqing, China. The study was conducted in accordance with the local legislation and institutional requirements.
Author contributions
YD: Conceptualization, Data curation, Writing – original draft. HeL: Conceptualization, Software, Writing – original draft. JL: Conceptualization, Writing – review & editing. SB: Investigation, Writing – original draft. SF: Resources, Writing – original draft. YZ: Resources, Writing – original draft. SL: Software, Validation, Writing – original draft. RL: Software, Validation, Writing – original draft. HaL: Resources, Writing – review & editing. CZ: Conceptualization, Funding acquisition, Supervision, Writing – review & editing. LL: Conceptualization, Funding acquisition, Supervision, Writing – review & editing.
Funding
The author(s) declare financial support was received for the research, authorship, and/or publication of this article. This research was supported by the National Natural Science Foundation of China (32160864); the Natural Science Foundation of Chongqing Province of China (CSTB2022NSCQ-MSX0566); the “Special Fund for Youth team of the Southwest University” (SWU-XJPY202302); the Fundamental Research Funds for the Central Universities (SWU-KQ24008); and the “National Talent Research Grant for 2023” (5330500953).
Conflict of interest
The authors declare that the research was conducted in the absence of any commercial or financial relationships that could be construed as a potential conflict of interest.
Publisher’s note
All claims expressed in this article are solely those of the authors and do not necessarily represent those of their affiliated organizations, or those of the publisher, the editors and the reviewers. Any product that may be evaluated in this article, or claim that may be made by its manufacturer, is not guaranteed or endorsed by the publisher.
Supplementary material
The Supplementary Material for this article can be found online at: https://www.frontiersin.org/articles/10.3389/fmars.2024.1448313/full#supplementary-material
References
Albenberg L., Esipova T. V., Judge C. P., Bittinger K., Chen J., Laughlin A., et al. (2014). Correlation between intraluminal oxygen gradient and radial partitioning of intestinal microbiota. Gastroenterology 147, 1055–1063.e8. doi: 10.1053/j.gastro.2014.07.020
Alfonso S., Gesto M., Sadoul B. (2021). Temperature increase and its effects on fish stress physiology in the context of global warming. J. Fish Biol. 98, 1496–1508. doi: 10.1111/jfb.14599
Al-Masqari Z. A., Guo H., Wang R., Yan H., Dong P., Wang G., et al. (2022). Effects of high temperature on water quality, growth performance, enzyme activity and the gut bacterial community of shrimp (Litopenaeus vannamei). Aquacult. Res. 53, 3283–3296. doi: 10.1111/are.15836
Arnaud C., Joyeux-Faure M., Bottari S., Godin-Ribuot D., Ribuot C. (2004). New insight into the signalling pathways of heat stress-induced myocardial preconditioning: protein kinase C-ϵ translocation and heat shock protein 27 phosphorylation. Clin. Exp. Pharmacol. Physiol. 31, 129–133. doi: 10.1111/j.1440-1681.2004.03966.x
Baptista R. B., Souza-Castro N., Almeida-Val V. M. F. (2016). Acute hypoxia up-regulates HIF-1α and VEGF mRNA levels in Amazon hypoxia-tolerant Oscar (Astronotus ocellatus). Fish Physiol. Biochem. 42, 1307–1318. doi: 10.1007/s10695-016-0219-1
Barbarossa V., Bosmans J., Wanders N., King H., Bierkens M. F. P., Huijbregts M. A. J., et al. (2021). Threats of global warming to the world’s freshwater fishes. Nat. Commun. 12, 1701. doi: 10.1038/s41467-021-21655-w
Blier P. (2014). Fish health: an oxidative stress perspective. Fish. Aquacult. J. 05, e105. doi: 10.4172/2150-3508.1000e105
Buffie C. G., Pamer E. G. (2013). Microbiota-mediated colonization resistance against intestinal pathogens. Nat. Rev. Immunol. 13, 790–801. doi: 10.1038/nri3535
Campbell N. K., Fitzgerald H. K., Dunne A. (2021). Regulation of inflammation by the antioxidant haem oxygenase 1. Nat. Rev. Immunol. 21, 411–425. doi: 10.1038/s41577-020-00491-x
Cao C., Chowdhury V. S., Cline M. A., Gilbert E. R. (2021). The microbiota-gut-brain axis during heat stress in chickens: A review. Front. Physiol. 12. doi: 10.3389/fphys.2021.752265
Chelakkot C., Ghim J., Ryu S. H. (2018). Mechanisms regulating intestinal barrier integrity and its pathological implications. Exp. Mol. Med. 50, 1–9. doi: 10.1038/s12276-018-0126-x
Chen C.-Z., Li P., Wang W.-B., Li Z.-H. (2022). Response of growth performance, serum biochemical parameters, antioxidant capacity, and digestive enzyme activity to different feeding strategies in common carp (Cyprinus carpio) under high-temperature stress. Aquaculture 548, 737636. doi: 10.1016/j.aquaculture.2021.737636
Chen Y., Liu E., Li C., Pan C., Zhao X., Wang Y., et al. (2021). Effects of heat stress on histopathology, antioxidant enzymes, and transcriptomic profiles in gills of pikeperch Sander lucioperca. Aquaculture 534, 736277. doi: 10.1016/j.aquaculture.2020.736277
Cheng C.-H., Guo Z.-X., Luo S.-W., Wang A.-L. (2018). Effects of high temperature on biochemical parameters, oxidative stress, DNA damage and apoptosis of pufferfish (Takifugu obscurus). Ecotoxicol. Environ. Saf. 150, 190–198. doi: 10.1016/j.ecoenv.2017.12.045
Cho Y. S., Jeong T. H., Choi M.-J., Kim J.-M., Lim H. K. (2021). Heat shock protein 70 gene expression and stress response of red-spotted (Epinephelus akaara) and hybrid (E. akaara female × E. lanceolatus male) groupers to heat and cold shock exposure. Fish Physiol. Biochem. 47, 2067–2080. doi: 10.1007/s10695-021-00966-1
Cornick S., Tawiah A., Chadee K. (2015). Roles and regulation of the mucus barrier in the gut. Tissue Barriers 3, e982426. doi: 10.4161/21688370.2014.982426
Cruz-Tapias P., Castiblanco J., Anaya J.-M. (2013).Major histocompatibility complex: Antigen processing and presentation. In: Autoimmunity: From Bench to Bedside (El Rosario University Press). Available online at: https://www.ncbi.nlm.nih.gov/books/NBK459467/ (Accessed May 12, 2024).
Czech B., Wang Y., Wang K., Luo H., Hu L., Szyda J. (2022). Host transcriptome and microbiome interactions in Holstein cattle under heat stress condition. Front. Microbiol. 13. doi: 10.3389/fmicb.2022.998093
Dang Y., Sun Y., Zhou Y., Men X., Wang B., Li B., et al. (2022). Effects of probiotics on growth, the toll-like receptor mediated immune response and susceptibility to Aeromonas salmonicida infection in rainbow trout Oncorhynchus mykiss. Aquaculture 561, 738668. doi: 10.1016/j.aquaculture.2022.738668
Deng C.-C., Zhang J.-P., Huo Y.-N., Xue H.-Y., Wang W., Zhang J.-J., et al. (2022). Melatonin alleviates the heat stress-induced impairment of Sertoli cells by reprogramming glucose metabolism. J. Pineal Res. 73, e12819. doi: 10.1111/jpi.12819
Dodds A. W., Matsushita M. (2007). The phylogeny of the complement system and the origins of the classical pathway. Immunobiology 212, 233–243. doi: 10.1016/j.imbio.2006.11.009
Dong Y., Deng S., Xiao W. (2016). Artificial propagation of Gymnocypris eckloni Herzenstein. Anim. Husbandry Feed Sci. 37, 8–10. doi: 10.16003/j.cnki.issn1672-5190.2016.z1.003
Ern R., Andreassen A. H., Jutfelt F. (2023). Physiological mechanisms of acute upper thermal tolerance in fish. Physiol. (Bethesda) 38, 141–158. doi: 10.1152/physiol.00027.2022
Faheem M., Khaliq S., Abbas R. Z., Mansour A. T. (2022). Moringa oleifera alleviated oxidative stress, physiological and molecular disruption induced by acute thermal stress in grass carp, Ctenopharyngodon idella. Fish Physiol. Biochem. 48, 1463–1473. doi: 10.1007/s10695-022-01147-4
Farag M. R., Alagawany M. (2018). Physiological alterations of poultry to the high environmental temperature. J. Therm Biol. 76, 101–106. doi: 10.1016/j.jtherbio.2018.07.012
Furuta K., Eguchi T. (2021). “Roles of HSP on antigen presentation,” in Heat Shock Proteins in Human Diseases. Eds. Asea A. A. A., Kaur P. (Springer International Publishing, Cham), 275–280. doi: 10.1007/7515_2020_5
Golovanova I. L., Golovanov V. K., Smirnov A. K., Pavlov D. D. (2013). Effect of ambient temperature increase on intestinal mucosa amylolytic activity in freshwater fish. Fish Physiol. Biochem. 39, 1497–1504. doi: 10.1007/s10695-013-9803-9
Guo K., Zhao Z., Luo L., Wang S., Zhang R., Xu W., et al. (2021). Immune and intestinal microbiota responses to aerial exposure stress in Chinese mitten crab (Eriocheir sinensis). Aquaculture 541, 736833. doi: 10.1016/j.aquaculture.2021.736833
GusChina I. A., Harwood J. L. (2006). Mechanisms of temperature adaptation in poikilotherms. FEBS Lett. 580, 5477–5483. doi: 10.1016/j.febslet.2006.06.066
He S., Woods L. C. (2004). Changes in motility, ultrastructure, and fertilization capacity of striped bass Morone saxatilis spermatozoa following cryopreservation. Aquaculture 236, 677–686. doi: 10.1016/j.aquaculture.2004.02.029
He J., Yu Y., Qin X.-W., Zeng R.-Y., Wang Y.-Y., Li Z.-M., et al. (2019). Identification and functional analysis of the Mandarin fish (Siniperca chuatsi) hypoxia-inducible factor-1α involved in the immune response. Fish Shellfish Immunol. 92, 141–150. doi: 10.1016/j.fsi.2019.04.298
Huang M., Zhao Q., Yin J., Cao S., Chen H., Duan R. (2022). The toxic effects of chronic atrazine exposure on the intestinal microbiota, metabolism and transcriptome of Pelophylax nigromaculatus larvae. J. Hazard. Mater. 440, 129817. doi: 10.1016/j.jhazmat.2022.129817
Huyben D., Vidakovic A., Sundh H., Sundell K., Kiessling A., Lundh T. (2019). Haematological and intestinal health parameters of rainbow trout are influenced by dietary live yeast and increased water temperature. Fish Shellfish Immunol. 89, 525–536. doi: 10.1016/j.fsi.2019.04.047
Janda J. M., Abbott S. L. (2006). The genus hafnia: from soup to nuts. Clin. Microbiol. Rev. 19, 12–28. doi: 10.1128/CMR.19.1.12-28.2006
Jian S., Yang B., Zhao J., Lv Z., Li K., Guan H., et al. (2020). Technique for raising large-scale seedling of Gymnocypris eckloni in Qinghai. Hebei Fish. 2020 (03), 24–26+49. doi: 10.3969/j.issn.1004-6755.2020.03.007
Jin Y., Wu S., Zeng Z., Fu Z. (2017). Effects of environmental pollutants on gut microbiota. Environ. pollut. 222, 1–9. doi: 10.1016/j.envpol.2016.11.045
Johansson M. E. V., Hansson G. C. (2016). Immunological aspects of intestinal mucus and mucins. Nat. Rev. Immunol. 16, 639–649. doi: 10.1038/nri.2016.88
Kim C.-H., Park C. J., Kim E. J., Nam Y. K. (2021). Transcriptional modulation patterns of abalone Haliotis discus hannai hypoxia inducible factor-1α (HIF-1α) in interdependent crosstalk between hypoxia, infection, and environmental stresses. Aquacult. Rep. 19, 100566. doi: 10.1016/j.aqrep.2020.100566
Kokou F., Sasson G., Nitzan T., Doron-Faigenboim A., Harpaz S., Cnaani A., et al. (2018). Host genetic selection for cold tolerance shapes microbiome composition and modulates its response to temperature. Elife 7, e36398. doi: 10.7554/eLife.36398
Konturek P. C., Brzozowski T., Konturek S. J. (2011). Stress and the gut: pathophysiology, clinical consequences, diagnostic approach and treatment options. J. Physiol. Pharmacol. 62, 591–599.
Kumar S., Sahu N. P., Pal A. K., Subramanian S., Priyadarshi H., Kumar V. (2011). High dietary protein combats the stress of Labeo rohita fingerlings exposed to heat shock. Fish Physiol. Biochem. 37, 1005–1019. doi: 10.1007/s10695-011-9504-1
Lambert G. P. (2009). Stress-induced gastrointestinal barrier dysfunction and its inflammatory effects. J. Anim. Sci. 87, E101–E108. doi: 10.2527/jas.2008-1339
Lemaire O. N., Méjean V., Iobbi-Nivol C. (2020). The Shewanella genus: ubiquitous organisms sustaining and preserving aquatic ecosystems. FEMS Microbiol. Rev. 44, 155–170. doi: 10.1093/femsre/fuz031
Li J., Duan Y., Kong W., Gao H., Fu S., Li H., et al. (2024b). Heat stress affects swimming performance and induces biochemical, structural, and transcriptional changes in the heart of Gymnocypris eckloni. Aquacult. Rep. 35, 101998. doi: 10.1016/j.aqrep.2024.101998
Li L., Liu Z., Quan J., Lu J., Zhao G., Sun J. (2022). Dietary nanoselenium supplementation for heat-stressed rainbow trout: effects on organizational structure, lipid changes, and biochemical parameters as well as heat-shock-protein- and selenoprotein-related gene expression. Fish Physiol. Biochem. 48, 707–722. doi: 10.1007/s10695-022-01084-2
Li L., Liu Z., Quan J., Sun J., Lu J., Zhao G. (2023a). Dietary nano-selenium alleviates heat stress-induced intestinal damage through affecting intestinal antioxidant capacity and microbiota in rainbow trout (Oncorhynchus mykiss). Fish Shellfish Immunol. 133, 108537. doi: 10.1016/j.fsi.2023.108537
Li X., Shi C., Yang B., Li Q., Liu S. (2023b). High temperature aggravates mortalities of the Pacific oyster (Crassostrea gigas) infected with Vibrio: A perspective from homeostasis of digestive microbiota and immune response. Aquaculture 568, 739309. doi: 10.1016/j.aquaculture.2023.739309
Li H., Zhang G., Liu Y., Gao F., Ye X., Lin R., et al. (2024a). Hypoxia-inducible factor 1α inhibits heat stress-induced pig intestinal epithelial cell apoptosis through eif2α/ATF4/CHOP signaling. Sci. Total Environ. 924, 171649. doi: 10.1016/j.scitotenv.2024.171649
Li Z., Zhao Z., Luo L., Wang S., Zhang R., Guo K., et al. (2023c). Immune and intestinal microbiota responses to heat stress in Chinese mitten crab (Eriocheir sinensis). Aquaculture 563, 738965. doi: 10.1016/j.aquaculture.2022.738965
Lin Y., Miao L.-H., Liu B., Xi B.-W., Pan L.-K., Ge X.-P. (2021). Molecular cloning and functional characterization of the hypoxia-inducible factor-1α in bighead carp (Aristichthys nobilis). Fish Physiol. Biochem. 47, 351–364. doi: 10.1007/s10695-020-00917-2
Liu X., Ma Z., Wang Y., Jia H., Wang Z., Zhang L. (2023). Heat stress exposure cause alterations in intestinal microbiota, transcriptome, and metabolome of broilers. Front. Microbiol. 14. doi: 10.3389/fmicb.2023.1244004
Liu Y., Wang J., Ding J., Zhang Y., Hou C., Shen W., et al. (2024). Effects of hypoxia stress on oxidative stress, apoptosis and microorganisms in the intestine of large yellow croaker (Larimichthys crocea). Aquaculture 581, 740444. doi: 10.1016/j.aquaculture.2023.740444
Livak K. J., Schmittgen T. D. (2001). Analysis of relative gene expression data using real-time quantitative PCR and the 2–ΔΔCT method. Methods 25, 402–408. doi: 10.1006/meth.2001.1262
Llewellyn M. S., Boutin S., Hoseinifar S. H., Derome N. (2014). Teleost microbiomes: the state of the art in their characterization, manipulation and importance in aquaculture and fisheries. Front. Microbiol. 5. doi: 10.3389/fmicb.2014.00207
Lu S., Xian T., Wang D., Wang C., Liu Y., Liu H., et al. (2022). Effects of traditional Chinese medicines on biochemical parameters, antioxidant capacity and heat shock protein expression in rainbow trout (Oncorhynchus mykiss) under heat stress. Aquacult. Res. 53, 6148–6157. doi: 10.1111/are.16088
Luo Z., Tian M., Yang G., Tan Q., Chen Y., Li G., et al. (2022). Hypoxia signaling in human health and diseases: implications and prospects for therapeutics. Sig Transduct Target Ther. 7, 1–30. doi: 10.1038/s41392-022-01080-1
Ming J., Xie J., Xu P., Ge X., Liu W., Ye J. (2012). Effects of emodin and vitamin C on growth performance, biochemical parameters and two HSP70s mRNA expression of Wuchang bream (Megalobrama amblycephala Yih) under high temperature stress. Fish Shellfish Immunol. 32, 651–661. doi: 10.1016/j.fsi.2012.01.008
Montero D., Izquierdo M. S., Tort L., Robaina L., Vergara J. M. (1999). High stocking density produces crowding stress altering some physiological and biochemical parameters in gilthead seabream, Sparus aurata, juveniles. Fish Physiol. Biochem. 20, 53–60. doi: 10.1023/A:1007719928905
Munro D., Treberg J. R. (2017). A radical shift in perspective: mitochondria as regulators of reactive oxygen species. J. Exp. Biol. 220, 1170–1180. doi: 10.1242/jeb.132142
Nayak S. K. (2010). Role of gastrointestinal microbiota in fish. Aquacult. Res. 41, 1553–1573. doi: 10.1111/are.2010.41.issue-11
Novelli B., Socorro J. A., Caballero M. J., Otero-Ferrer F., Segade-Botella A., Molina Domínguez L. (2015). Development of seahorse (Hippocampus reidi, Ginsburg 1933): histological and histochemical study. Fish Physiol. Biochem. 41, 1233–1251. doi: 10.1007/s10695-015-0082-5
Olsen R. E., Sundell K., Mayhew T. M., Myklebust R., Ringø E. (2005). Acute stress alters intestinal function of rainbow trout, Oncorhynchus mykiss (Walbaum). Aquaculture 250, 480–495. doi: 10.1016/j.aquaculture.2005.03.014
Orbach R., Su X. (2020). Surfing on membrane waves: microvilli, curved membranes, and immune signaling. Front. Immunol. 11. doi: 10.3389/fimmu.2020.02187
Park D. S., Gu B.-H., Park Y. J., Joo S. S., Lee S.-S., Kim S.-H., et al. (2021). Dynamic changes in blood immune cell composition and function in Holstein and Jersey steers in response to heat stress. Cell Stress Chaperones 26, 705–720. doi: 10.1007/s12192-021-01216-2
Parra-Medina R., Quintero-Ronderos P., Rodríguez É.G. (2013).The complement system. In: Autoimmunity: From Bench to Bedside (El Rosario University Press). Available online at: https://www.ncbi.nlm.nih.gov/books/NBK459482/ (Accessed May 12, 2024).
Pavelka M., Roth J. (2010). “Small intestine: absorptive cells,” in Functional Ultrastructure: Atlas of Tissue Biology and Pathology. Eds. Pavelka M., Roth J. (Springer, Vienna), 224–225. doi: 10.1007/978-3-211-99390-3_116
Pérez-Casanova J. C., Afonso L. O. B., Johnson S. C., Currie S., Gamperl A. K. (2008). The stress and metabolic responses of juvenile Atlantic cod Gadus morhua L. to an acute thermal challenge. J. Fish Biol. 72, 899–916. doi: 10.1111/j.1095-8649.2007.01763.x
Polsky L., von Keyserlingk M. A. G. (2017). Invited review: Effects of heat stress on dairy cattle welfare. J. Dairy Sci. 100, 8645–8657. doi: 10.3168/jds.2017-12651
Przepiura T., de C. S., Herrerias T., Kandalski P. K., Zaleski T., MaChado C., et al. (2019). Metabolic responses in Antarctic Nototheniidae brains subjected to thermal stress. Brain Res. 1708, 126–137. doi: 10.1016/j.brainres.2018.12.004
Qin H., Long Z., Huang Z., Ma J., Kong L., Lin Y., et al. (2023). A comparison of the physiological responses to heat stress of two sizes of juvenile spotted seabass (Lateolabrax maculatus). Fishes 8, 340. doi: 10.3390/fishes8070340
Ran C., Huang L., Hu J., Tacon P., He S., Li Z., et al. (2016). Effects of dietary live and heat-inactive baker’s yeast on growth, gut health, and disease resistance of Nile tilapia under high rearing density. Fish Shellfish Immunol. 56, 263–271. doi: 10.1016/j.fsi.2016.07.001
Reda R. M., El-Murr A., Abdel-Basset N. A., Metwally M. M. M., Ibrahim R. E. (2024). Infection dynamics of Shewanella spp. in Nile tilapia under varied water temperatures: A hematological, biochemical, antioxidant-immune analysis, and histopathological alterations. Fish Shellfish Immunol. 149, 109588. doi: 10.1016/j.fsi.2024.109588
Rich A. F., Naguib M. (2024). Shewanella xiamenensis-associated ulcerative dermatitis in koi carp (Cyprinus rubrofuscus). J. Fish Dis. 47, e13942. doi: 10.1111/jfd.13942
Ricklin D., Reis E. S., Mastellos D. C., Gros P., Lambris J. D. (2016). Complement component C3 - the “Swiss army knife innate immunity host defense. Immunol. Rev. 274, 33–58. doi: 10.1111/imr.12500
Ruan G., Li S., He N., Fang L., Wang Q. (2022). Short-term adaptability to non-hyperthermal stress: Antioxidant, immune and gut microbial responses in the red swamp crayfish, Procambarus clarkii. Aquaculture 560, 738497. doi: 10.1016/j.aquaculture.2022.738497
Sanders K. M., Koh S. D., Ro S., Ward S. M. (2012). Regulation of gastrointestinal motility—insights from smooth muscle biology. Nat. Rev. Gastroenterol. Hepatol. 9, 633–645. doi: 10.1038/nrgastro.2012.168
Sekirov I., Russell S. L., Antunes L. C. M., Finlay B. B. (2010). Gut microbiota in health and disease. Physiol. Rev. 90, 859–904. doi: 10.1152/physrev.00045.2009
Sepulveda J., Moeller A. H. (2020). The effects of temperature on animal gut microbiomes. Front. Microbiol. 11. doi: 10.3389/fmicb.2020.00384
Slimen I. B., Najar T., Ghram A., Dabbebi H., Ben Mrad M., Abdrabbah M. (2014). Reactive oxygen species, heat stress and oxidative-induced mitochondrial damage. A review. Int. J. Hyperther. 30, 513–523. doi: 10.3109/02656736.2014.971446
Song Z., Li K., Li K. (2024). Acute effects of the environmental probiotics Rhodobacter sphaeroides on intestinal bacteria and transcriptome in shrimp Penaeus vannamei. Fish Shellfish Immunol. 145, 109316. doi: 10.1016/j.fsi.2023.109316
Sonnenburg J. L., Chen C. T. L., Gordon J. I. (2006). Genomic and metabolic studies of the impact of probiotics on a model gut symbiont and host. PloS Biol. 4, e413. doi: 10.1371/journal.pbio.0040413
Soriano E. L., Ramírez D. T., Araujo D. R., Gómez-Gil B., Castro L. I., Sánchez C. G. (2018). Effect of temperature and dietary lipid proportion on gut microbiota in yellowtail kingfish Seriola lalandi juveniles. Aquaculture 497, 269–277. doi: 10.1016/j.aquaculture.2018.07.065
Srivastava P. (2002). Roles of heat-shock proteins in innate and adaptive immunity. Nat. Rev. Immunol. 2, 185–194. doi: 10.1038/nri749
Stewart H. A., Aboagye D. L., Ramee S. W., Allen P. J. (2019). Effects of acute thermal stress on acid–base regulation, haematology, ion-osmoregulation and aerobic metabolism in Channel Catfish (Ictalurus punctatus). Aquacult. Res. 50, 2133–2141. doi: 10.1111/are.14093
Strocchi S., Reggiani F., Gobbi G., Ciarrocchi A., Sancisi V. (2022). The multifaceted role of EGLN family prolyl hydroxylases in cancer: going beyond HIF regulation. Oncogene 41, 3665–3679. doi: 10.1038/s41388-022-02378-8
Sundh H., Kvamme B. O., Fridell F., Olsen R. E., Ellis T., Taranger G. L., et al. (2010). Intestinal barrier function of Atlantic salmon (Salmo salar L.) post smolts is reduced by common sea cage environments and suggested as a possible physiological welfare indicator. BMC Physiol. 10, 22. doi: 10.1186/1472-6793-10-22
Taylor C. T., Colgan S. P. (2017). Regulation of immunity and inflammation by hypoxia in immunological niches. Nat. Rev. Immunol. 17, 774–785. doi: 10.1038/nri.2017.103
Treinin M., Shliar J., Jiang H., Powell-Coffman J. A., Bromberg Z., Horowitz M. (2003). HIF-1 is required for heat acclimation in the nematode Caenorhabditis elegans. Physiol. Genomics 14, 17–24. doi: 10.1152/physiolgenomics.00179.2002
Tsai A. P., Dong C., Lin P. B.-C., Messenger E. J., Casali B. T., Moutinho M., et al. (2022). PLCG2 is associated with the inflammatory response and is induced by amyloid plaques in Alzheimer’s disease. Genome Med. 14, 17. doi: 10.1186/s13073-022-01022-0
Vancamelbeke M., Vermeire S. (2017). The intestinal barrier: a fundamental role in health and disease. Expert Rev. Gastroenterol. Hepatol. 11, 821–834. doi: 10.1080/17474124.2017.1343143
Wade N. M., Clark T. D., Maynard B. T., Atherton S., Wilkinson R. J., Smullen R. P., et al. (2019). Effects of an unprecedented summer heatwave on the growth performance, flesh colour and plasma biochemistry of marine cage-farmed Atlantic salmon (Salmo salar). J. Thermal Biol. 80, 64–74. doi: 10.1016/j.jtherbio.2018.12.021
Wang J., Fan H., Xia S., Shao J., Tang T., Chen L., et al. (2022b). Microbiome, transcriptome, and metabolomic analyses revealed the mechanism of immune response to diarrhea in rabbits fed antibiotic-free diets. Front. Microbiol. 13. doi: 10.3389/fmicb.2022.888984
Wang A. R., Ran C., Ringø E., Zhou Z. G. (2018). Progress in fish gastrointestinal microbiota research. Rev. Aquacult. 10, 626–640. doi: 10.1111/raq.12191
Wang F., Wang L., Liu D., Gao Q., Nie M., Zhu S., et al. (2022a). Chromosome-level assembly of Gymnocypris eckloni genome. Sci. Data 9, 464. doi: 10.1038/s41597-022-01595-w
Wanghe K., Feng C., Tang Y., Qi D., Ahmad S., Nabi G., et al. (2022). Phylogenetic relationship and taxonomic status of Gymnocypris eckloni (Schizothoracinae) based on specific locus amplified fragments sequencing. Front. Ecol. Evol. 10. doi: 10.3389/fevo.2022.933632
Wei M., Zheng T., Lu S., Qiang J., Tao Y.-F., Li Y., et al. (2024). Ammonia-N stress on tissue structure,enzyme activity andintestinal microbiota of Macropterus salmoides. Acta Hydrobiol. Sin. 48, 10–22.
Wu Y., Liu X., Wang Q., Xu Y., Bao Z. (2008). Studies on the ultrastructure of spermiogenesis and spermatozoon of tongue fish, Cynoglossus semilaevis günther. Aquacult. Res. 39, 1467–1474. doi: 10.1111/are.2008.39.issue-14
Wu Y., Wu C. (1992). The fishes of the Qinghai – Xizang plateau (Sichuan, China: Science and Technology Press).
Wu J.-M., Xiao L., Cheng X.-K., Cui L.-X., Wu N.-H., Shen Y.-F. (2003). PKCϵ Is a unique regulator for hsp90β Gene in heat shock response *. J. Biol. Chem. 278, 51143–51149. doi: 10.1074/jbc.M305537200
Wu Y., You X., Sun W., Xiong G., Shi L., Qiao Y., et al. (2021). Insight into acute heat stress on meat qualities of rainbow trout (Oncorhynchus mykiss) during short-time transportation. Aquaculture 543, 737013. doi: 10.1016/j.aquaculture.2021.737013
Xu D., Zhou S., Sun L. (2018). RNA-seq based transcriptional analysis reveals dynamic genes expression profiles and immune-associated regulation under heat stress in Apostichopus japonicus. Fish Shellfish Immunol. 78, 169–176. doi: 10.1016/j.fsi.2018.04.037
Yang C., Dong J., Sun C., Li W., Tian Y., Liu Z., et al. (2022a). Exposure to heat stress causes downregulation of immune response genes and weakens the disease resistance of Micropterus salmoides. Comp. Biochem. Physiol. Part D: Genomics Proteomics 43, 101011. doi: 10.1016/j.cbd.2022.101011
Yang L., Fang J., Peng X., Cui H., He M., Zuo Z., et al. (2017). Study on the morphology, histology and enzymatic activity of the digestive tract of Gymnocypris eckloni Herzenstein. Fish Physiol. Biochem. 43, 1175–1185. doi: 10.1007/s10695-017-0363-2
Yang P.-C., He S.-H., Zheng P.-Y. (2007). Investigation into the signal transduction pathway via which heat stress impairs intestinal epithelial barrier function. J. Gastroenterol. Hepatol. 22, 1823–1831. doi: 10.1111/j.1440-1746.2006.04710.x
Yang S., Zhang C., Xu W., Li D., Feng Y., Wu J., et al. (2022b). Heat stress decreases intestinal physiological function and facilitates the proliferation of harmful intestinal microbiota in sturgeons. Front. Microbiol. 13. doi: 10.3389/fmicb.2022.755369
Yang Y., Zhu X., Huang Y., Zhang H., Liu Y., Xu N., et al. (2022c). RNA-seq and 16S rRNA analysis revealed the effect of deltamethrin on channel catfish in the early stage of acute exposure. Front. Immunol. 13. doi: 10.3389/fimmu.2022.916100
Yu K., Huang Z., Xiao Y., Wang D. (2022). Shewanella infection in humans: Epidemiology, clinical features and pathogenicity. Virulence 13, 1515–1532. doi: 10.1080/21505594.2022.2117831
Yu J., Zhong D., Li S., Zhang Z., Mo H., Wang L. (2023). Acute temperature stresses trigger liver transcriptome and microbial community remodeling in largemouth bass (Micropterus salmoides). Aquaculture 573, 739573. doi: 10.1016/j.aquaculture.2023.739573
Zhang Y., Li Z., Kholodkevich S., Sharov A., Feng Y., Ren N., et al. (2020). Microcystin-LR-induced changes of hepatopancreatic transcriptome, intestinal microbiota, and histopathology of freshwater crayfish (Procambarus clarkii). Sci. Total Environ. 711, 134549. doi: 10.1016/j.scitotenv.2019.134549
Zhang C., Wang S., Hu L., Fang H., Chen G., Ma X., et al. (2023). Analysis of circRNA expression in peripheral blood of Holstein cows in response to heat stress. Int. J. Mol. Sci. 24, 10150. doi: 10.3390/ijms241210150
Zhang X.-D., Zhu Y.-F., Cai L.-S., Wu T.-X. (2008). Effects of fasting on the meat quality and antioxidant defenses of market-size farmed large yellow croaker (Pseudosciaena crocea). Aquaculture 280, 136–139. doi: 10.1016/j.aquaculture.2008.05.010
Zhao J., Zhao B., Kong N., Li F., Liu J., Wang L., et al. (2023). Increased abundances of potential pathogenic bacteria and expressions of inflammatory cytokines in the intestine of oyster Crassostrea gigas after high temperature stress. Dev. Comp. Immunol. 141, 104630. doi: 10.1016/j.dci.2022.104630
Zheng T., Tao Y., Lu S., Qiang J., Xu P. (2022). Integrated Transcriptome and 16S rDNA Analyses Reveal That Transport Stress Induces Oxidative Stress and Immune and Metabolic Disorders in the Intestine of Hybrid Yellow Catfish (Tachysurus fulvidraco♀ × Pseudobagrus vachellii♂). Antioxidants 11, 1737. doi: 10.3390/antiox11091737
Zhou C., Duan Y., Li J., Fu S., Bai S., Zhuang Y., et al. (2024). Decoding the intestinal response to heat stress in Gymnocypris eckloni: Insights from a thorough analysis of microbiome and transcriptome. Aquaculture 591, 741112. doi: 10.1016/j.aquaculture.2024.741112
Zhou C., Gao P., Wang J. (2023). Comprehensive analysis of microbiome, metabolome, and transcriptome revealed the mechanisms of intestinal injury in rainbow trout under heat stress. Int. J. Mol. Sci. 24, 8569. doi: 10.3390/ijms24108569
Zhou C., Yang S., Ka W., Gao P., Li Y., Long R., et al. (2022). Association of gut microbiota with metabolism in rainbow trout under acute heat stress. Front. Microbiol. 13. doi: 10.3389/fmicb.2022.846336
Zhu T., Li X., Wu X., Yang D. (2022). Temperature acclimation alters the thermal tolerance and intestinal heat stress response in a tibetan fish Oxygymnocypris stewarti. Front. Microbiol. 13. doi: 10.3389/fmicb.2022.898145
Keywords: Gymnocypris eckloni, acute heat stress, intestine, transcriptome, 16S rDNA
Citation: Duan Y, Li H, Li J, Bai S, Fu S, Zhou Y, Liu S, Li R, Liu H, Zhou C and Lei L (2024) Integrated transcriptome and 16S rDNA analyses reveal that acute heat stress induces intestinal damage in Gymnocypris eckloni. Front. Mar. Sci. 11:1448313. doi: 10.3389/fmars.2024.1448313
Received: 13 June 2024; Accepted: 04 July 2024;
Published: 18 July 2024.
Edited by:
Yi-Feng Li, Shanghai Ocean University, ChinaReviewed by:
Basanta Kumar Das, Central Inland Fisheries Research Institute (ICAR), IndiaZheng-Yong Wen, Neijiang Normal University, China
Liqin Ji, Chinese Academy of Fishery Sciences, China
Copyright © 2024 Duan, Li, Li, Bai, Fu, Zhou, Liu, Li, Liu, Zhou and Lei. This is an open-access article distributed under the terms of the Creative Commons Attribution License (CC BY). The use, distribution or reproduction in other forums is permitted, provided the original author(s) and the copyright owner(s) are credited and that the original publication in this journal is cited, in accordance with accepted academic practice. No use, distribution or reproduction is permitted which does not comply with these terms.
*Correspondence: Luo Lei, leiluo12311@163.com; Chaowei Zhou, zcwlzq666@163.com
†These authors have contributed equally to this work and share first authorship