- 1Analysis and Test Center, Guangdong Ocean University, Zhanjiang, Guangdong, China
- 2College of Chemistry and Environment, Guangdong Ocean University, Zhanjiang, Guangdong, China
Estuarine sediments serve as significant reservoirs for mercury (Hg) and methylmercury(MeHg), which can also interconvert in the external environment. The release of Hg in response to human activities raises concerns about its potential ecological and human health effects. Sediment samples were collected in December 2021 from four locations (sites), and Hg cycling by measuring the concentrations of, and controls on, the spatial distribution of total Hg (THg) and methylmercury (MeHg) in high-tidal zone (HTZ) and mid-tidal zone (MTZ) sediments of a mangrove forest (MF) and oyster farm (OF) was examined in northwestern Zhanjiang Bay, including simultaneous determination of sediment particle size, oxidation-reduction potential (Eh), pH, total organic carbon (TOC), sulfide concentration (S2-), and sulfate reducing bacteria (SRB). The research results indicated that concentrations of both THg and MeHg ranged between 20.0–104.0 ng/g and 0.011–0.277 ng/g in the sediments, respectively. The highest methylation potentials within the MF and OF were in sediments located approximately 10–15 cm below the surface. MeHg in the HTZ of the OF was likely derived from exogenous inputs as Hg methylation appears limited, and the formation of MeHg depended not only on the amount of inorganic mercury available for methylation in SRB, but also on the TOC, pH, Eh and S2- content in the sediment. A risk assessment of MeHg during the anthropogenic disturbance of this estuaries conducted on individuals eating oysters demonstrated that health risks are low.
1 Introduction
Mercury (Hg) is a persistent, priority pollutant on a global scale due, in part, to is toxic effects on human and ecosystem health, its ability to be easily transported in the Earth’s near surface environment. Natural sources of Hg include volcanic eruptions, hydrothermal activity, forest fires, earthquakes, and sediment (Gonzalez-Raymat et al., 2017), whereas anthropogenic sources include fossil fuel power plants, chlor-alkali plants, and Hg amalgamation mining activities, to mention a few (Liu et al., 2021). Once released to the Earth’s surface, its biogeochemical cycling is highly complex. Elemental (Hg0) can, for example, exist as a stable gaseous species that may be converted by a wide range of redox, phase, and biologically mediated transformations into other chemical forms, including oxidized mercury (HgII), methylmercury (MeHg), dimethylmercury (DMeHg), and ethylmercury (EtHg). MeHg, an organic form of Hg, is of particular concern because of its ability to be readily absorbed and bio-accumulated in biota, where it may subsequently be bio-magnified up the food chain. In fact, the predominant pathway of human exposure in many areas is the consumption of contaminated biota, particular sea food. Once ingested, MeHg is highly toxic and can damage the central nervous system (Rice et al., 2010; Li and Cai, 2013).
MeHg is produced by the conversion of HgII to MeHg in anaerobic environments by methylating bacteria, including sulfate-reducing bacteria (SRB) and, to a much lesser degree, iron-reducing bacteria and methanogens (Regnell and Watras, 2018; Villar et al., 2020; Eckley et al., 2021). The processes of methylation are not well understood, but it is influenced by the amount of inorganic and dissolved organic matter as well as sulfur compounds in the water and sediments (Eckley et al., 2021). MeHg demethylation is a reverse process of Hg methylation. It simultaneously occurs in the sites (sediment, periphyton, and water) that methylation takes place. Within aquatic environments, some methylation may occur in anoxic waters where the water column is stratified, but the overwhelming majority of methylation takes place in anoxic sediments, particularly within the first few centimeters of the water-sediment interface, where there tends to be a relative abundance of methylating bacteria. Similar to methylation of Hg, biotic process was suggested to be the dominant pathway of Hg demethylation in sediment and periphyton, however this process has not been confirmed in natural environments. But methylation typically overrides demethylation and determines the net production of MeHg in sediment and periphyton (Li and Cai, 2013).
Due to the possible local increase in mercury input in estuaries and general coastal environments in recent years, the level of methylation in estuarine environments can be especially pronounced because of the abundance of organic matter and sulfur compounds in the underlying sediments as well as the widespread occurrence of anoxic conditions (Crane, 2006). While the cycling of Hg and MeHg production has been extensively studied in estuarine environments, the influence of localized human activities and morphological changes on estuaries and MeHg production, and their results impacts on human health, remain a relatively understudied topic. This is particularly true of mangrove forests (MF), because sediments in MF often serve as an important sink of many heavy metal pollutants (Castro et al., 2021), including MeHg, as they represent depositional environments that possess the conditions conducive to methylation, including high OM and sulfur ion (S2−) concentrations and local anoxic conditions (Morel et al., 1998; Wolfenden et al., 2005; Drott et al., 2007; Fitzgerald et al., 2007). At the same time, mangrove sediments may also serve as a source of MeHg as it can be released from the sediments into the water column as a result of tidal action (Benoit et al., 2009; Seelen et al., 2021). However, investigations pertaining to the contamination of the Bay’s sediments in general, and MeHg in particular, are currently lacking. One issue of particular concern is the potential for human activities to disturb MFs, such that it increases the production of MeHg and/or the remobilization of MeHg from the sediments. For example, increased nitrogen loading in estuaries is associated with increased deposition of organic carbon to the sediments due to the production and sedimentation of detrital particulate organic matter, thereby affecting the production of MeHg (Chen et al., 2021).
The primary objectives of this study were to: (1) characterize the sediments within high- to mid-tidal areas of the Zhanjiang Bay estuary within a MF and an OF in terms of the parameters that are likely to influence Hg speciation and methylation, including particle size, Eh, total organic carbon (TOC) and sulfide concentrations (S2−) as well as SRB; (2) explore the influence of these factors on the concentrations and vertical distribution of Hg and MeHg within the sediments, (3) document the potential for Hg methylation within the MF and OF, and (4) determine the human health risks posed by Hg when oysters raised within an OF are consumed. This study furthers our understanding of the biogeochemical cycling of Hg in estuarine environments, and the potential risks of Hg methylation and MeHg bioaccumulation within oyster farms near mangrove forests in Zhanjiang Bay, and other coastal areas.
2 Material and methods
2.1 Study area
Zhanjiang Bay is located adjacent to the South China Sea and is surrounded by three islands that create a semi-enclosed, shallow bay. A dyke has been built on the west side of Zhanjiang Bay, connecting Donghai Island and the interior of Zhanjiang City (Figure 1B). On both sides of the dyke, there are areas of OF and MF, with an area of approximately 12 square kilometers for OF. OF near mangroves is done through poles inserted in high densities into the sediments (Figure 1C), resulting in severe siltation. Abundant organic matter from oysters’ feces and MF’ litter jointly create anaerobic environment for mercury methylation. However, it is currently unclear which external input or on-site production is the main source of MeHg in the research location.
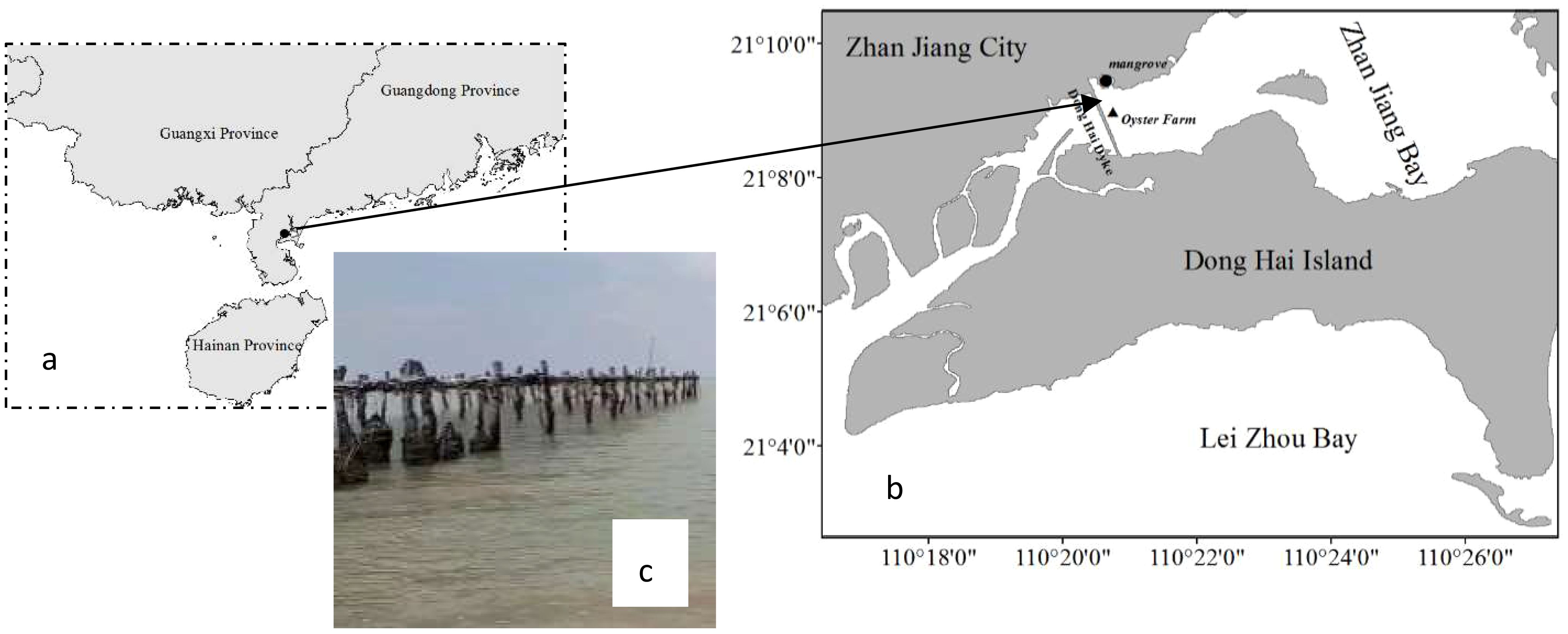
Figure 1. (A) Location of Zhanjiang Bay in China; (B) location of sampling sites within the Bay; (C) photograph of oyster cultivation conducted by inserting into the sediments.
2.2 Sample collection and analysis
Sediment samples were collected in December 2021 from four locations (sites), two in the high-tidal zone (HTZ) and two in the mid-tidal zone (MTZ) of each “cover type”, including sites HTZ-MF, MTZ-MF, HTZ-OF and MTZ-OF (Figure 1). The reason for choosing these four regions was because the HTZ was more influenced by land and human activities, while the geological and environmental types of the mid tide zone and low tide zone were similar but sampling in the mid tide zone was safer and more convenient. A stainless-steel sampling tube was used to collect a sediment profile to a depth of 25 cm. Once collected, the sediment cores (profiles) were subdivided into samples at 5 cm intervals. Eh of the pore waters was measured using a redox potentiometer (Nanjing Dropper Instrument and Equipment Co., LTD. FJA-6). The remaining samples were placed in glass bottles, and transported to the laboratory where fresh and wet samples were analyzed for sulfide (S2−) content, particle size distribution, and SRB (as described below). The remaining portion of the samples were freeze-dried, ground, and passed through a 0.15 mm nylon sieve, before they were analyzed for TOC, THg, and MeHg. Six oysters of different sizes collected on-site in the OFs were randomly selected from numerous individuals about to enter the consumer market and transported to the laboratory. The oysters were washed with ultrapure water, and the size and weight of the oysters were recorded.
2.2.1 Analysis of the sediments
The particle size distribution of the sediment samples was analyzed using a Mastersizer 2000 (Malvern, UK) laser particle size analyzer. TOC concentrations were determined using the K2Cr2O7 OX-RE-VL based, Walkley-Black (WB) titration method as described by Gaudette et al. (1974). The analysis of S2− in the sediments was determined using a Sulfur Ion Selective Electrode (PXSJ-226, Shanghai Instrument and Electricity Scientific Instrument Co., Ltd.), and was carried out following the method of Wildish et al. (1999). The occurrence of SRB in the sediments was determined using a multitube fermentation technique via incubation at 29 ± 1°C for 21 d. The presence of SRB was indicated by the production of a black precipitate in the tube with a hydrogen sulfide odor. A most probably number (MPN) method was used to count the SRB in the analyzed samples. Sample blanks were also analyzed. If a blank appeared positive, the assay had been contaminated and the data were considered invalid.
2.2.2 Analysis of THg in the sediment and oyster samples
THg was analyzed using an Atomic Fluorescence Spectrometer (SK-AFS, Jinsuokun, Beijing, China) with a standard curve correlation coefficient (R2) of 0.999. Diluted aqua regia (50%, HCl: HNO3 = 3:1 v/v) was added to the tubes to digest the sediments (Ding et al., 2011). The quality control standard concentration for sediment was 0.048 µg/g; the analyzed value was 0.043 μg/g, with a recovery of 90.0%. Dried oyster samples, along with quality control samples, were digested using a microwave digestion system (Qiu et al., 2006). The analyzed biotic standard consisted of shrimp (GBW10050; GSB-28) with a control concentration of 0.049 μg/g; the measured value was 0.050 μg/g, with a recovery of 101.9%. Sediment and biotic samples are expressed on a dry-weight basis.
2.2.3 Analysis of MeHg in sediments and oysters
MeHg in sediments and oysters were measured using an Automated Alkyl Mercury Analyzer (Tekran 2700, Canada) (Qiu et al., 2009). Dry sediment was weighed into a 50 mL centrifuge tube, to which 1.0 ml of 2 mol/LCuSO4, 5.0 ml of an acidic KBr solution and 10 ml of CH2Cl2 were added to extract MeHg. The MeHg in the sample solution was then ethylated using NaBEt4 to form gaseous diethyl-mercury, and DMeHg was converted into gaseous methyl-ethyl-mercury. The method’s detection limit was 1.0 pg. Quality control and verification were carried out at the same time using a method blank, parallel sample and a certified reference material. MeHg recovery from the sediments ranged from 90% to 110%.
Similarly, six oysters were accurately weighed and placed into their respective Teflon bottles, to which 5 ml of 250 g/L KOH-CH3OH solution was added, and then allowed to digest in a water bath at 75°C for 3 h. The bottles were shaken every 30 minutes to ensure that the digestion solution fully reacted with the sample. After digestion, the Teflon bottle was removed from the water bath and cooled to room temperature, after which 3 ml of concentrated HCl was added. The remainder of the procedure followed the steps described above for the analysis of MeHg in sediments. MeHg recovery for the utilized fish standard (tormart-3, DORM-4) was between 90% and 110% (Qiu et al., 2009).
2.2.4 Hg and MeHg exposure risk assessment for the consumption of oysters
An exposure risk assessment quantifies risk by combining hazard identification and dose effects to assess the probability that the contaminant will result in a health hazard for human (Feng et al., 2013). The needed parameters were calculated as follows.
where PDIs from Equation 1 is the Probable Daily Intake, defined as the average daily intake of a contaminant by means of the food to be ingested; the average daily intake is expresses per body weight, bw, (μg·kg−1·d−1); bw was assumed here to be 60 kg; C is the concentration of Hg and MeHg in oysters (ng·g−1); IR is the intake reference, defined as the average daily intake of food (kg·d−1); here, it was assumed to be 5.5 g/d of oysters, which is based on the daily consumption of fish; HRI from Equation 2 is the Human Health Risk Index, and RfD is the reference dose, which is the amount that can be safely consumed (µg·kg−1·d−1). HRI is obtained from the ratio of PDIs and the Joint FAO/WHO Expert Committee on Food Additives recommended reference dose (RfD) for inorganic Hg and MeHg (Liang et al., 2015). The RfD for inorganic mercury is 0.57 μg/(kg·d) and for MeHg is 0.23 μg/(kg·d); the maximum allowable exposure set by the US EPA for MeHg is 0.1 μg·kg−1·d−1. If the HRI is >1.0, the population possesses a high health risk.
2.3 Statistical analysis
Maps of sampling stations were generated using ArcGIS 10.2. The Pearson’s correlation analyses between MeHg, THg and selected environmental factors in sediments were performed using SPSS 24.0. Statistical significance of the Spearman correlations was set at a level of 0.05 (p<0.05) and/or 0.01 (p<0.01). Data plots were constructed using Origin 9.0 software.
3 Results and discussion
The environment change of water bodies caused by aquaculture not only leads to an increase in organic matter, but also causes changes in many physical and chemical parameters of water bodies, including a decrease in dissolved oxygen in water, an increase in organic matter and sulfate in sediments, hydrogen sulfide, a decrease in pH values, and the destruction of biological and microbial communities, thereby affecting the generation of MeHg (Yan and Feng, 2012). The above factors will also change with the increase of sediment depth and further affect the vertical distribution of Hg and MeHg.
The concentrations and spatial distribution of both THg and MeHg depend on the sedimentologic, geochemical, and compositional nature of the estuarine sediments. Here, we explore the sedimentologic characteristics of the sediments that are of most importance in controlling Hg concentrations at a site and compare how they differ as a function of their position within the tidal zone and between the MF and the OF. All of the collected parameter data are presented as a function of depth below the water-sediment interface. This has been done because vertical variations in these parameters, as well as in THg and MeHg, provide insights into the rates and location of methylation and the potential sources of MeHg.
3.1 Sedimentological and geochemical characteristics of the MF and OF sediments
As shown in Figure 2, sediments in the study area were dominated by silt and sand-slit sediments. Sediments of the MF possess higher clay-sized particle contents than sediments of the OF (Table 1).The sedimentology of the bottom sediments within the MF and OF differs, in part, because of the surface cover’s ability to trap sediment. For example, the complex root system of mangrove trees tend to capture suspended particulate matter, resulting in the formation of sandy bottom sediments in MFs (Bouillon et al., 2004; Kristensen et al., 2008). However, sediments within the OF, devoid of mangrove trees, simultaneously receive suspended particles from tidal processes and oyster excrement. The grain size distribution of the sediments, particularly the abundance of silt- and clay-sized particles, is an especially important parameter controlling the sequestration of Hg in the deposits. This follows because of the high surface area and general reactivity of fine particles, that enhances their relative ability to sorb metals.
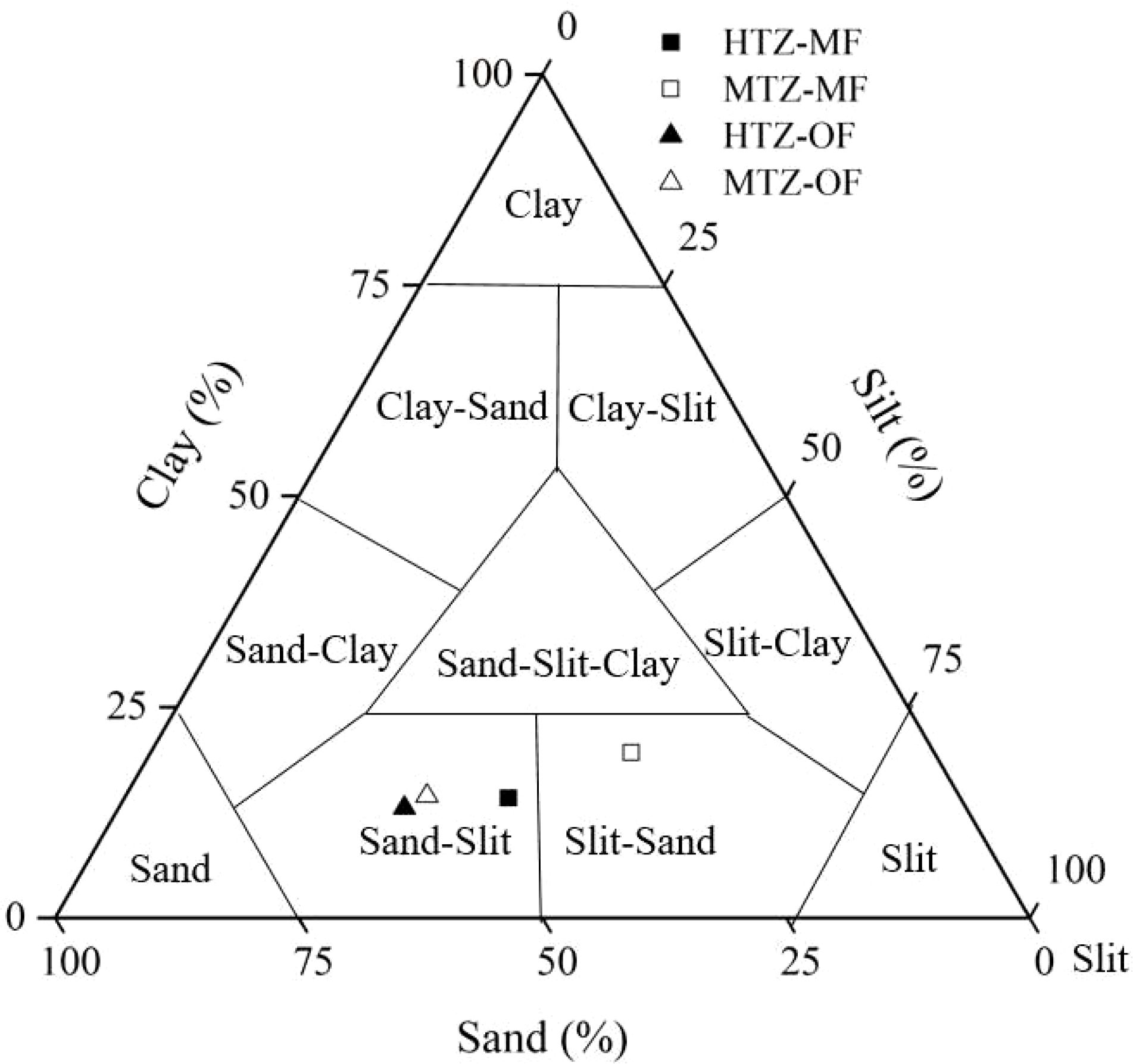
Figure 2. Texture of the sediments sampled within the study area. The position of each symbol in the triangle diagram is determined by the average value of the granularity.
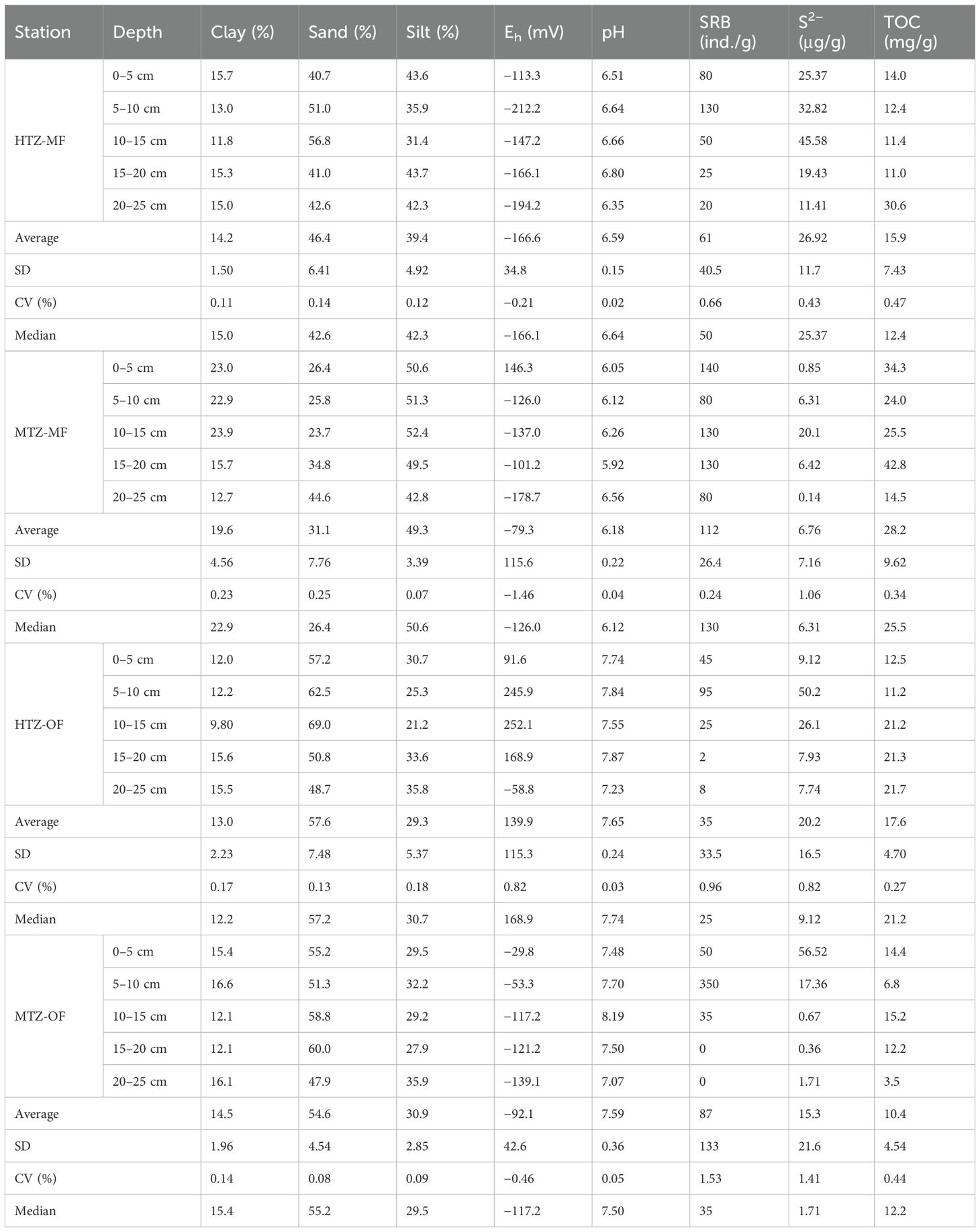
Table 1. Sediment characteristics of the MF and OF samples within the intertidal zone of Zhanjiang Bay, including their particle size distribution (i.e., percent clay, silt and sand), Eh, SRB, S2− and TOC.
The composition of the sediments not only influences the reactivity of the materials, and their ability to sequester Hg, but is believed to affect its Eh. Related studies had shown that the Eh of estuarine surface sediments typically ranges from −213 mV to 512 mV (Sunderland, 2003). In this study, except for site HTZ-OF, dominated by areas of bare sediment, the average Eh of the sediments at sites HTZ-MF, MTZ-MF and MTZ-OF averaged −166.6 mV, −79.3 mV and −92.1 mV, respectively (Table 1). The Eh values observed at site HTZ-OF were strongly positive, except for the interval between 20–25 cm. As noted in the introduction, reducing conditions are required for the significant production of MeHg by SRB. Therefore, the reducing environment of sediments in the study area met one of the conditions for MeHg formation, but whether this process was also influenced by other factors needed further investigation.
Acidic substances in MF plant litter, including tannins, resins, and low molecular weight organic acids acidify the sediments. These effects can be especially pronounced in areas characterized by high organic matter contents (Chai et al., 2020; Shi et al., 2020). The pH of MF sediments in the study area generally ranged between 6 and 6.8, although values as low as 5.92 were recorded at stie MTZ-MF. In contrast, the pH of sediments within the OF were typically above 7.00, which is similar to the pH of nearshore water bodies. Sediment pH has a significant impact on the availability, migration, and transformation of Hg by influencing its solubility, and by regulating the types and activities of microorganisms in the sediments (Correia and Guimarães, 2016, 2017). To some extent, the lower pH in the MF sediments promoted the desorption of mercury ions from the adsorption phase and further participation in methylation reactions.
3.2 Relations between S2-, TOC, and SRB in sediments
Previous studies have demonstrated that TOC combined with sulfur plays an important role in controlling the production of MeHg in sediments (Shen et al., 2020). In this study area, the median TOC contents in sediments of the HTZ-MF and MTZ-MF were 12.4 and 25.5 mg/g. The differences presumably reflect a relatively high cover of vegetation and biological activity within the MF, including at site MTZ-MF. The vertical variations in TOC contents at site MTZ-MF were smaller than at site HTZ-MF, as illustrated by its coefficient of variation (Table 1). However, site MTZ-OF is affected by more significant tidal exchanges and human activities. Thus, within sediments from the OF, the vertical variations in TOC observed in the HTZ-MF were smaller than those in the MTZ-OF (see coefficient of variation for TOC, Table 1).
The cycling of sulfur can affect MeHg production by restricting the migration of Hg within the sediments (Padalkar et al., 2019). In this study, we considered S2− concentrations in the sediment pore waters because (1) it can directly influence the formation of Hg sulfides, and (2) it is, in some cases, related to the magnitude of MeHg production. Table 1 shows that the average S2− content of the sediments from both the MF and OF were higher in the high-tide zone than in the mid-tide zone. In general, sediment in the HTZ-MF contained more S2−, and the correlation coefficient was smaller than that in other areas. The elevated levels of S2− within sediments of the HTZ may be due to the enhanced exchange of sediment pore waters with seawater within the MTZ, as this process led to higher concentrations of sulfate and dissolved oxygen (Wilms et al., 2007). In addition, the breeding density of oysters within the HTZ-OF and the MTZ-OF is likely to have affected the S2− content of the sediment, which, in combination with the deposition of organic matter associated with oyster feces, is more likely to form a reducing environment.
SRB can promote the methylation process of mercury, which had been confirmed by many studies (Azaroff et al., 2020). The highest SRB count (130 ind./g) was observed in sediments between a depth of 5–10 cm at site HTZ-MF. Compared with that of the OF, the higher TOC and clay-sized particle contents in the sediments of the MF create more widespread anoxic conditions that are suitable for the establishment and growth of SRB. Therefore, the median abundance of SRB in sediments from the MF was higher than in the OF.
3.3 Spatial distribution of THg in the MF and OF sediments
Concentrations of inorganic Hg exert a significant control on MeHg production. Given that most of the Hg in the sediments is inorganic, THg concentrations provide insights into the amount of inorganic Hg that is available for methylation. As shown in Figure 3, the THg content at site HTZ-MF decreased slightly with increasing depth, ranging from about 35.5 to 20.0 ng/g. Mangrove leaves absorb Hg through stomatal gas exchange, and leaf fall transfers Hg to the soil, which may exceed the direct input of Hg to the sediments by atmospheric wet deposition. Due to dense vegetation and rich biological diversity in the MTZ-MF, THg concentrations ranged from 76.0 to ~104.0 ng/g at stie MTZ-MF. Vertically, concentrations increased with depth, reaching a maximum concentration of 104.0 ng/g at a depth of 15–20 cm and a minimum concentration of 76.0 ng/g in the surface layer (0–5 cm). These values are lower than those measured for THg in MF sediments in Guangdong, Guangxi, Fujian, Zhejiang and Hainan provinces in southern China, which ranged from 50–200 ng/g (Duan et al., 2021). The lower values within Zhanjiang Bay may be due to the limited industrial development in Zhanjiang and the implementation of strict environmental protection measures. The observed trend in THg with depth may result from bioturbation that physically mixes “fresh” sediments at the surface with older sediments (Tseng et al., 2001; Benoit et al., 2009).
The THg content at site MTZ-OF ranged from 27.0 to 89.0 ng/g, whereas the MeHg content ranged from 0.031 to 0.202 ng/g. The THg concentrations in the MTZ-OF exhibited trends similar to those in the HTZ-OF. The 5–10 cm and 15–20 cm layers of sediment had relatively more silty sand than the other sediments, which may have resulted in a loss of THg and MeHg by means of in situ dissolution. In general, THg concentrations are elevated within the upper 10 cm of the sediment, and subsequently, decrease with depth. The enrichment of THg in the surface sediments may be associated with the sorption of Hg to organic matter (OM) within the water column, including oyster fecal excreta, metabolites, bait residues and marine plankton carcasses, which settles on the surface of the sediments.
3.4 Controls on the distribution of MeHg in the MF and OF sediments
Concentrations of MeHg ranged from 0.011~0.128 ng/g. Spatially, MeHg at site HTZ-MF exhibited a vertical trend similar to that of THg, with the exception of a peak in concentration at a depth of 10–15 cm (Figure 3). Concentrations of MeHg above the peak are slightly higher than those below the peak. A predominance of MeHg in the surface sediment is consistent with the results of Sunderland et al. (2006) who found that MeHg production occurred mainly in the approximately 15 cm thick, active surface layer (Sunderland et al., 2006).MeHg concentrations in the MTZ-MF ranged from 0.05 to 0.277 ng/g; the maximum concentrations being more than twice as high as was found at site HTZ-MF. However, the maximum MeHg concentration at site MTZ-MF was found at a depth of 10–15 cm, as it was at site HTZ-MF. Presumably, this depth interval at both sites provided the most suitable conditions for SRB to participate in methylation reactions (Benoit et al., 1999). As shown in Figure 3, the MeHg content ranged from 0.168–0.211 ng/g at site HTZ-OF. The maximum concentrations of MeHg are about twice as high as those observed at site HTZ-MF.
3.5 Spatial distribution of methylation potential in MF and OF sediments
The ratio of MeHg to THg (%MeHg) is often used as an indicator of the Hg methylation potential (Liem-Nguyen et al., 2022). As shown in Figure 3, the methylation potential of Hg in MF and OF sediments varied from 0.05% to 0.75%, which is comparable to that estimated for nearshore sediments in the East China Sea (0.02% to 0.64%) (Zhao et al., 2019) and the estuarine sediments in New England (0.4%) (Taylor et al., 2012). It is lower, however, than that in the Southern Baltic sea (0.12–1.05%) (Bełdowski et al., 2014) and aquatic sediments across western North America (0.7%) (Fleck et al., 2016). The Hg methylation potential at site HTZ-OF increased with depth between 0 and 20 cm, reaching a maximum of 0.75% between 15–20 cm (Figure 3). Usually, only a small fraction (< 1–10%) of Hg in coastal sediments typically occurs in the form of MeHg, the majority of which is produced in the sediments. However, sediments at site HTZ-OF possessed limited quantities of SRB, and the lack of favorable conductions for Hg methylation suggests that the existing MeHg originated primarily from exogenous inputs. At site MTZ-OF, the THg contents and the quantity of SRB in some sediment layers were high. However, sediments at the site also exhibited high S2− concentrations and low TOC levels, suggesting that the methylation potential, which represents the net production of MeHg, was low in some sediment layers at site MTZ-OF.
In fact, the HTZ-MF had a maximum methylation potential (an indicator of net MeHg production) of 0.37% at a depth interval of 10–15 cm; the methylation potential in the sediments at depths of 0–5 cm and 10–15 cm was relatively high, 0.33% and 0.29%, respectively. In addition to the quantity of inorganic Hg that SRB can be used for methylation, the amount of MeHg formation depends on (1) the OM, sulfate, and S2− contents of the sediment, and (2) the local Eh (Ullrich et al., 2001; Benoit et al., 2003; Chen et al., 2008). Therefore, the relationship between the above factors and mercury methylation in the study area needs further confirmation.
3.6 Environmental factors controlling the vertical distribution of THg and MeHg
Table 2 shows that THg concentrations are significantly negatively correlated with TOC (r = −0.950, p<0.05) in sediments at site HTZ-OF, and significantly positively correlated with Eh (r = 0.912, p<0.05) at site MTZ-OF. THg of the MF showed no significant correlations with any of the selected environmental parameters; correlation coefficients varied across the study areas, even yielding opposing values. This finding is consistent with the work of Shi et al. (2020). They found no significant correlations between Hg contents and TOC, pH, salinity, and the amount of clay, fine chalk, coarse chalk and sand in mangrove sediments. OM often controls the spatial distribution of Hg in aquatic sediments (Li et al., 2015; Xiao et al., 2017). It is not surprising, then, that sediment THg and TOC are often significantly positively correlated (Xue et al., 2019; Zhao et al., 2019). However, we observed a significant negative correlation between THg and TOC in sediments of the OF, suggesting that OM, which contains both Hg-binding and hydrophilic groups, was combined with Hg and facilitated the transfer of Hg from the solid phase to the water column rather than the opposite process.
As shown in Table 3, MeHg in the HTZ-OF was positively correlated with sand (r = 0.930, p < 0.05) and negatively correlated with the amount of sandy, silt-sized (r = −0.902; p < 0.05) and clay-sized (r=−0.947; p < 0.05) sediments. The above results are contrary to the research of Haris et al. (2017) and Castro et al. (2021) who found that sediments with small particle sizes were significantly positively correlated with MeHg. High concentrations of fine sediments suggest a slow rate of water movement through the relatively stable bottom sediments, which allowed epiphytes (a complex consisting mainly of algal, protozoan, fungal and bacterial strains) to grow in the sediments and methylate Hg under low Eh conditions (Yáñez et al., 2013). The lack of an influence of particle size on MeHg within the study area also suggests that the MeHg at site HTZ-OF is mainly exogenous.
In the HTZ-MF, MeHg was positively correlated with S2− (r = 0.880, p<0.05), and TOC was weakly or negatively correlated with MeHg contents at sites MTZ-MF and MTZ-OF. These relationships show that TOC is not a major factor influencing the methylation of Hg in estuarine wetland sediments. The poor correlation may also be due to the presence of high concentrations of other metals that compete with Hg for binding or adsorption sites on TOC (Xu et al., 2014). The oxidizing conditions induced by aquatic plants tended to cause Cd, Pb, and Zn, rather than Hg, to be bound to the Fe-Mn oxide fraction. Alternatively, it is possible that TOC provides energy and electron donors to demethylating bacteria and facilitates the demethylation of Hg. Of course, further experiments are needed to verify the above speculation. The distribution of MeHg in sediments can often be predicted by THg concentrations (Yu et al., 2021), but THg is not the only important factor determining MeHg abundance in sediments (Petranich et al., 2018). Similarly, no association had been found between THg and MeHg in the current research area. In fact, THg and MeHg were even found to be negatively correlated in the freshwater sediments of Scottish canals (Cavoura et al., 2017).
3.7 Correlation of methylation potential and various environmental factors
We further explored the influence of selected environmental factors on the distribution of the methylation potential in the sediment profiles. Table 4 shows that the correlation between THg and methylation potential is weakly negative. The highest percentages of MeHg in sediments at sites HTZ-OF and MTZ-OF have the lowest THg concentrations. This phenomenon has been recognized in other areas, and is known as the “Hg accumulation paradox” (Haris et al., 2017; Mondal et al., 2018). This paradox may be related to microbial demethylation that is induced by high THg concentrations, such that the rate of demethylation increases with increasing THg concentrations (Schaefer et al., 2004; Haris et al., 2017). The methylation potential was significantly positively correlated with MeHg (r=0.619, p<0.01), positively correlated with Eh (r=0.455, p<0.05), and weakly negatively correlated with THg, TOC, S2−, and SRB. The positive correlation of methylation potential with MeHg and Eh occurs because methylation is enhanced at the interface between aerobic and anoxic zones, as these regions are most favorable to SRB activity. This interface also be used to undergo adsorption-desorption of Hg between the particulate and water phases and redox reactions (King et al., 2001; Jiang et al., 2015; Zhu et al., 2018). For example, inputs of degradable OM promoted sulfate reduction to inorganic sulfur that combined with Hg ions to form a Hg precipitate, which reduces the bioavailability of Hg ions for methylating microorganisms (Wang et al., 2022). Previous studies have shown that the highest SRB activity occurs at a redox potential of −200 mV (Wildish et al., 1999; Sunderland, 2003), which is consistent with the results of the this study in which higher methylation potentials at site MTZ-OF occurred at Eh values of −117.2 mV and −139.1 mV, respectively. However, it is unclear whether the increase of MeHg in sediments during hypoxic events, which result from the decomposition of algae by bacteria following red tides, is caused by the redox environment at the sediment–water interface that created favorable conditions for methylating bacteria, or to the release of MeHg from interstitial water within the sediments (Sunderland et al., 2006; Merritt and Amirbahman, 2008). Distinguishing these specific factors is difficult through simple statistical analysis. Nonetheless, the results demonstrate that the amount of MeHg accumulated in the study area through methylation was limited.
3.8 Risk assessment of eating MeHg contaminated oysters
Approximately 70–90% of Hg in seafood is in the form of MeHg (Canuel et al., 2006). In the research area, oysters had an annual output value of 7 billion yuan, with an annual production of up to 400000 tons, accounting for 10% of the country’s total. Therefore, oysters could be used as a representative food for human consumption of seafood. The high levels of industrial and social activity in coastal areas, combined with the influx of Hg from sewage and dry and wet atmospheric deposition, result in high Hg inputs to estuaries. Part of this Hg may be transformed into MeHg, and accumulated by biota, thereby posing a risk to coastal dwellers eating sea food. Therefore, Hg monitoring and the assessment of potential ecological and human health risks of MeHg exposure are needed (Wiener et al., 2007).
The oyster, a shellfish that feeds on OM in water, can be used as a sentinel organism due to its nutritional value and significant, widespread consumption. As presented in Table 5, the THg and MeHg contents of six oysters were found to range from 128.74 to 187.04 ng/g and 0.71 to 6.63 ng/g, respectively. Using formulas (1) and (2) provided in the Methods section, the HRI values of THg and MeHg obtained from consuming oysters in the study area were much lower than 1.0, there was no correlation between the MeHg, and the potential health risk of Hg. However, there are still potential flaws in the calculation results of mercury exposure risk. Especially, it should be taken into account that the underrepresentation caused by the small number of experimental individuals, as well as the fact that oysters may have different mercury concentrations due to their different life states, size classes, and other environmental factors. Therefore, it is necessary to further study the potential factors mentioned above in the future to correct possible errors in the results.
4 Conclusion
MeHg contents were generally higher in OF sediment than sediments of the MF. Neither THg or MeHg significantly correlated with the environmental factors (except S2−) within the MF that were suspected to have controlled their concentrations in sediments. A correlation analysis indicates that the amount of MeHg accumulated through methylation was limited in the study area. Thus, we conclude that MeHg in the HTZ-OF was likely to be derived from exogenous inputs. Although the risk assessment based on existing research results indicates that the potential health risks associated with eating oyster containing MeHg in this area is low, attention should be paid to the individual differences in mercury content in oysters caused by external inputs in OF, changes in sedimentary environment, and other factors leading to additional ingestion.
Data availability statement
The original contributions presented in the study are included in the article/supplementary material. Further inquiries can be directed to the corresponding author.
Author contributions
ZZ: Writing – original draft. CC: Writing – review & editing. MF: Data curation, Methodology, Writing – original draft.
Funding
The author(s) declare financial support was received for the research, authorship, and/or publication of this article. This work was supported by the Science and Technology Special Project of Zhanjiang City (2019B01009) and Innovation and Entrepreneurship Training Program for College Students of Guangdong Ocean University (CXXL2022180).
Acknowledgments
We thank LetPub (www.letpub.com) for linguistic assistance and pre-submission expert review. The authors would like to thank all volunteers for their help with fieldwork and the technical staff for their fantastic support.
Conflict of interest
The authors declare that the research was conducted in the absence of any commercial or financial relationships that could be construed as a potential conflict of interest.
Publisher’s note
All claims expressed in this article are solely those of the authors and do not necessarily represent those of their affiliated organizations, or those of the publisher, the editors and the reviewers. Any product that may be evaluated in this article, or claim that may be made by its manufacturer, is not guaranteed or endorsed by the publisher.
References
Azaroff A., Goñi Urriza M., Gassie C., Monperrus M., Guyoneaud R. (2020). Marine mercury-methylating microbial communities from coastal to Capbreton Canyon sediments (North Atlantic Ocean). Environ. Pollut. 262, 114333. doi: 10.1016/j.envpol.2020.114333
Bełdowski J., Miotk M., Bełdowska M., Pempkowiak J. (2014). Total, methyl and organic mercury in sediments of the Southern Baltic Sea. Mar. Pollut. Bull. 87, 388–395. doi: 10.1016/j.marpolbul.2014.07.001
Benoit J. M., Gilmour C. C., Heyes A., Mason R. P., Miller C. L. (2003). Geochemical and biological controls over methylmercury production and degradation in aquatic ecosystems, in: Biogeochemistry of Environmentally Important Trace Elements, ACS Symposium Series. Am. Chem. Soc. 19, 262–297. doi: 10.1021/bk-2003-0835.ch019
Benoit J. M., Gilmour C. C., Mason R. P., Heyes A. (1999). Sulfide controls on mercury speciation and bioavailability to methylating bacteria in sediment pore waters. Environ. Sci. Technol. 33, 951–957. doi: 10.1021/es9808200
Benoit J. M., Shull D. H., Harvey R. M., Beal S. A. (2009). Effect of bioirrigation on sediment-water exchange of methylmercury in Boston Harbor, Massachusetts. Environ. Sci. Technol. 43, 3669–3674. doi: 10.1021/es803552q
Bouillon S., Moens T., Dehairs F. (2004). Carbon sources supporting benthic mineralization in mangrove and adjacent seagrass sediments (Gazi Bay, Kenya). Biogeosciences 1, 71–78. doi: 10.5194/bg-1-71-2004
Canuel R., de Grosbois S. B., Atikessé L., Lucotte M., Arp P., Ritchie C., et al. (2006). New evidence on variations of human body burden of methylmercury from fish consumption. Environ. Health Perspect. 114, 302–306. doi: 10.1289/ehp.7857
Castro S., Luiz-Silva W., MaChado W., Valezio E. (2021). Mangrove sediments as long-term mercury sinks: Evidence from millennial to decadal time scales. Mar. Pollut. Bull. 173, 113031. doi: 10.1016/j.marpolbul.2021.113031
Cavoura O., Brombach C. C., Cortis R., Davidson C. M., Gajdosechova Z., Keenan H. E., et al. (2017). Mercury alkylation in freshwater sediments from scottish canals. Chemosphere 183, 27–35. doi: 10.1016/j.chemosphere.2017.05.077
Chai M., Li R., Qiu Z., Niu Z., Shen X. (2020). Mercury distribution and transfer in sediment-mangrove system in urban mangroves of fast-developing coastal region, Southern China. Estuarine Coast. Shelf Sci. 240, 106770. doi: 10.1016/j.ecss.2020.106770
Chen C., Amirbahman A., Fisher N., Harding G., Lamborg C., Nacci D., et al. (2008). Methylmercury in marine ecosystems: Spatial patterns and processes of production, bioaccumulation, and biomagnification. EcoHealth 5, 399–408. doi: 10.1007/s10393-008-0201-1
Chen C. Y., Buckman K. L., Shaw A., Curtis A., Taylor M., Montesdeoca M., et al. (2021). The influence of nutrient loading on methylmercury availability in Long Island estuaries. Environ. Pollut. 268, 115510. doi: 10.1016/j.envpol.2020.115510
Correia R. R. S., Guimarães J. R. D. (2016). Impacts of crab bioturbation and local pollution on sulfate reduction, Hg distribution and methylation in mangrove sediments, Rio de Janeiro, Brazil. Mar. Pollut. Bull. 109, 453–460. doi: 10.1016/j.marpolbul.2016.05.028
Correia R. R. S., Guimarães J. R. D. (2017). Mercury methylation and sulfate reduction rates in mangrove sediments, Rio de Janeiro, Brazil: The role of different microorganism consortia. Chemosphere 167, 438–443. doi: 10.1016/j.chemosphere.2016.09.153
Crane J. L. (2006). Phase iv gis-based sediment quality database for the St. Louis riverarea of concern-wisconsin focus. Overview of sediment quality conditions in the St. Louis river area of concern. (St. Paul, MN: Minnesota Pollution Control Agency, Environmentalanalysis and Outcomes Division). Available online at: http://www.pca.state.mn.us/publications/tdr-fg06-04a.pdf (Accessed January 19, 2020).
Ding Z., Wu H., Feng X., Liu J., Liu Y., Yuan Y., et al. (2011). Distribution of Hg in mangrove trees and its implication for Hg enrichment in the mangrove ecosystem. Appl. Geochem. 26, 205–212. doi: 10.1016/j.apgeochem.2010.11.020
Drott A., Lambertsson L., Björn E., Skyllberg U. (2007). Importance of dissolved neutral mercury sulfides for methyl mercury production in contaminated sediments. Environ. Sci. Technol. 41, 2270–2276. doi: 10.1021/es061724z
Duan D., Lei P., Lan W., Li T., Zhang H., Zhong H., et al. (2021). Litterfall-derived organic matter enhances mercury methylation in mangrove sediments of South China. Sci. Total Environ. 765, 142763. doi: 10.1016/j.scitotenv.2020.142763
Eckley C. S., Luxton T. P., Knightes C. D., Shah V. (2021). Methylmercury production and degradation under light and dark conditions in the water column of the Hells Canyon reservoirs, USA. Environ. Toxicol. Chem. 40, 1827–1837. doi: 10.1002/etc.5041
Feng X. B., Qiu G. L., Wang S. F., Li P., Meng B. (2013). Risk assessments and exposure pathways of inorganic mercury and methylmercury to populations from mercury mining regions in China. Geochimica 42, 205–211. doi: 10.3969/j.issn.0379-1726.2013.03.002
Fitzgerald W. F., Lamborg C. H., Hammerschmidt C. R. (2007). Marine biogeochemical cycling of mercury. Chem. Rev. 107, 641–662. doi: 10.1021/cr050353m
Fleck J. A., Marvin-DiPasquale M., Eagles-Smith C. A., Ackerman J. T., Lutz M. A., Tate M., et al. (2016). Mercury and methylmercury in aquatic sediment across western North America. Sci. Total Environ. 568, 727–738. doi: 10.1016/j.scitotenv.2016.03.044
Gaudette H. E., Flight W. R., Toner L., Folger D. W. (1974). An inexpensive titration method for the determination of organic carbon in recent sediments. J. Sediment. Res. 44, 249–253. doi: 10.1306/74D729D7-2B21-11D7-8648000102C1865D
Gonzalez-Raymat H., Liu G., Liriano C., Li Y., Yin Y., Shi J., et al. (2017). Elemental mercury: Its unique properties affect its behavior and fate in the environment. Environ. Pollut. 229, 69–86. doi: 10.1016/j.envpol.2017.04.101
Haris H., Aris A. Z., bin Mokhtar M. (2017). Mercury and methylmercury distribution in the intertidal surface sediment of a heavily anthrophogenically impacted saltwater-mangrove-sediment interplay zone. Chemosphere 166, 323–333. doi: 10.1016/j.chemosphere.2016.09.045
Jiang T., Skyllberg U., Wei S., Wang D., Lu S., Jiang Z., et al. (2015). Modeling of the structure-specific kinetics of abiotic, dark reduction of Hg(II) complexed by O/N and S functional groups in humic acids while accounting for time-dependent structural rearrangement. Geochimica Cosmochimica Acta 154, 151–167. doi: 10.1016/j.gca.2015.01.011
King J. K., Kostka J. E., Frischer M. E., Saunders F. M., Jahnke R. A. (2001). A quantitative relationship that demonstrates mercury methylation rates in marine sediments are based on the community composition and activity of sulfate-reducing bacteria. Environ. Sci. Technol. 35, 2491–2496. doi: 10.1021/es001813q
Kristensen E., Bouillon S., Dittmar T., Marchand C. (2008). Organic carbon dynamics in mangrove ecosystems: A review. Aquat. Bot. 89, 201–219. doi: 10.1016/j.aquabot.2007.12.005
Li Y., Cai Y. (2013). Progress in the study of mercury methylation and demethylation in aquatic environments. Chin. Sci. Bull. 58, 177–185. doi: 10.1007/s11434-012-5416-4
Li Y., Duan Z., Liu G., Kalla P., Scheidt D., Cai Y. (2015). Evaluation of the possible sources and controlling factors of toxic metals/metalloids in the Florida Everglades and their potential risk of exposure. Environ. Sci. Technol. 49, 9714–9723. doi: 10.1021/acs.est.5b01638
Liang P., Feng X.-B., Zhang C., Zhang J., Cao Y., You Q.-Z., et al. (2015). Human exposure to mercury in a compact fluorescent lamp manufacturing area: By food (rice and fish) consumption and occupational exposure. Environ. Pollut. 198, 126–132. doi: 10.1016/j.envpol.2014.12.036
Liem-Nguyen V., Wild B., Gustafsson Ö., Semiletov I., Dudarev O., Jonsson S. (2022). Spatial patterns and distributional controls of total and methylated mercury off the Lena River in the Laptev Sea sediments. Mar. Chem. 238, 104052. doi: 10.1016/j.marchem.2021.104052
Liu M., Zhang Q., Maavara T., Liu S., Wang X., Raymond P. A. (2021). Rivers as the largest source of mercury to coastal oceans worldwide. Nat. Geosci. 14, 672–677. doi: 10.1038/s41561-021-00793-2
Merritt K. A., Amirbahman A. (2008). Methylmercury cycling in estuarine sediment pore waters (Penobscot River estuary, Maine, USA). Limnol. Oceanogr. 53, 1064–1075. doi: 10.4319/lo.2008.53.3.1064
Mondal P., de Alcântara Mendes R., Jonathan M. P., Biswas J. K., Murugan K., Sarkar S. K. (2018). Seasonal assessment of trace element contamination in intertidal sediments of the meso-macrotidal Hooghly (Ganges) River Estuary with a note on mercury speciation. Mar. Pollut. Bull. 127, 117–130. doi: 10.1016/j.marpolbul.2017.11.041
Morel F. M. M., Kraepiel A. M. L., Amyot M. (1998). The chemical cycle and bioaccumulation of mercury. Annu. Rev. Ecol. System. 29, 543–566. doi: 10.1146/annurev.ecolsys.29.1.543
Padalkar P. P., Chakraborty P., Chennuri K., Jayachandran S., Sitlhou L., Nanajkar M., et al. (2019). Molecular characteristics of sedimentary organic matter in controlling mercury (Hg) and elemental mercury (Hg0) distribution in tropical estuarine sediments. Sci. Total Environ. 668, 592–601. doi: 10.1016/j.scitotenv.2019.02.353
Petranich E., Covelli S., Acquavita A., Faganeli J., Horvat M., Contin M. (2018). Evaluation of mercury biogeochemical cycling at the sediment–water interface in anthropogenically modified lagoon environments. J. Environ. Sci. 68, 5–23. doi: 10.1016/j.jes.2017.11.014
Qiu G., Feng X., Wang S., Fu X., Shang L. (2009). Mercury distribution and speciation in water and fish from abandoned Hg mines in Wanshan, Guizhou province, China. Sci. Total Environ. 407, 5162–5168. doi: 10.1016/j.scitotenv.2009.06.007
Qiu G., Feng X., Wang S., Xiao T. (2006). Mercury contaminations from historic mining to water, soil and vegetation in Lanmuchang, Guizhou, Southwestern China. Sci. Total Environ. 368, 56–68. doi: 10.1016/j.scitotenv.2005.09.030
Regnell O., Watras C. J. (2018). Microbial mercury methylation in aquatic environments: A critical review of published field and laboratory studies. Environ. Sci. Technol. 53, 4–19. doi: 10.1021/acs.est.8b02709
Rice G. E., Hammitt J. K., Evans J. S. (2010). A probabilistic characterization of the health benefits of reducing methyl mercury intake in the United States. Environ. Sci. Technol. 44, 5216–5224. doi: 10.1021/es903359u
Schaefer J. K., Yagi J., Reinfelder J. R., Cardona T., Ellickson K. M., Tel-Or S., et al. (2004). Role of the bacterial organomercury lyase (MerB) in controlling methylmercury accumulation in mercury-contaminated natural waters. Environ. Sci. Technol. 38, 4304–4311. doi: 10.1021/es049895w
Seelen E. A., Chen C. Y., Balcom P. H., Buckman K. L., Taylor V. F., Mason R. P. (2021). Historic contamination alters mercury sources and cycling in temperate estuaries relative to uncontaminated sites. Water Res. 190, 116684. doi: 10.1016/j.watres.2020.116684
Shen J., Feng Q., Algeo T. J., Liu J., Zhou C., Wei W., et al. (2020). Sedimentary host phases of mercury (Hg) and implications for use of Hg as a volcanic proxy. Earth Planet. Sci. Lett. 543, 116333. doi: 10.1016/j.epsl.2020.116333
Shi C., Yu L., Chai M., Niu Z., Li R. (2020). The distribution and risk of mercury in Shenzhen mangroves, representative urban mangroves affected by human activities in China. Mar. Pollut. Bull. 151, 110866. doi: 10.1016/j.marpolbul.2019.110866
Sunderland E. M. (2003). Development of a marine mercury cycling model for Passamaquoddy Bay, New Brunswick. Simon Fraser University, Burnaby, British Columbia.
Sunderland E. M., Gobas F. A. P. C., Branfireun B. A., Heyes A. (2006). Environmental controls on the speciation and distribution of mercury in coastal sediments. Mar. Chem. 102, 111–123. doi: 10.1016/j.marchem.2005.09.019
Taylor D. L., Linehan J. C., Murray D. W., Prell W. L. (2012). Indicators of sediment and biotic mercury contamination in a southern New England estuary. Mar. Pollut. Bull. 64, 807–819. doi: 10.1016/j.marpolbul.2012.01.013
Tseng C. M., Amouroux D., Abril G., Tessier E., Etcheber H., Donard O. F. X. (2001). Speciation of mercury in a fluid mud profile of a highly turbid macrotidal estuary (Gironde, France). Environ. Sci. Technol. 35, 2627–2633. doi: 10.1021/es001750b
Ullrich S. M., Tanton T. W., Abdrashitova S. A. (2001). Mercury in the aquatic environment: A review of factors affecting methylation. Crit. Rev. Environ. Sci. Technol. 31, 241–293. doi: 10.1080/20016491089226
Villar E., Cabrol L., Heimbürger-Boavida L.-E. (2020). Widespread microbial mercury methylation genes in the global ocean. Environ. Microbiol. Rep. 12, 277–287. doi: 10.1111/1758-2229.12829
Wang Y., Wang Z., Zheng X., Zhou L. (2022). Influence of Spartina alterniflora invasion on mercury storage and methylation in the sediments of Yangtze River estuarine wetlands. Estuarine Coast. Shelf Sci. 265, 107717. doi: 10.1016/j.ecss.2021.107717
Wiener J., Bodaly R., Brown S., Lucotte M., Newman M., Porcella D., et al. (2007). “Monitoring and evaluating trends in methylmercury accumulation in aquatic biota,” Ecosystem Responses to Mercury Contamination (CRC Press), 87–122. doi: 10.1201/9780849388897.ch4
Wildish D. J., Akagi H. M., Hamilton N., Hargrave B. T. (1999). A recommended method for monitoring sediments to detect organic enrichment from mariculture in the Bay of Fundy. Canadian technical report of fisheries and aquatic sciences/Rapport technique canadien des sciences halieutiques et aquatiques. Imprint varies, 2286, p.34.
Wilms R., Sass H., Köpke B., Cypionka H., Engelen B. (2007). Methane and sulfate profiles within the subsurface of a tidal flat are reflected by the distribution of sulfate-reducing bacteria and methanogenic archaea. FEMS Microbiol. Ecol. 59, 611–621. doi: 10.1111/j.1574-6941.2006.00225.x
Wolfenden S., Charnock J. M., Hilton J., Livens F. R., Vaughan D. J. (2005). Sulfide species as a sink for mercury in lake sediments. Environ. Sci. Technol. 39, 6644–6648. doi: 10.1021/es048874z
Xiao C., Jian H., Chen L., Liu C., Gao H., Zhang C., et al. (2017). Toxic metal pollution in the Yellow Sea and Bohai Sea, China: distribution, controlling factors and potential risk. Mar. Pollut. Bull. 119, 381–389. doi: 10.1016/j.marpolbul.2017.03.027
Xu J., Kleja D. B., Biester H., Lagerkvist A., Kumpiene J. (2014). Influence of particle size distribution, organic carbon, pH and chlorides on washing of mercury contaminated soil. Chemosphere 109, 99–105. doi: 10.1016/j.chemosphere.2014.02.058
Xue W., Kwon S. Y., Grasby S. E., Sunderland E. M., Pan X., Sun R., et al. (2019). Anthropogenic influences on mercury in Chinese soil and sediment revealed by relationships with total organic carbon. Environ. Pollut. 255, 113186. doi: 10.1016/j.envpol.2019.113186
Yan H., Feng C. (2012). Resesrcher necessity and advances about influence on methlylation of mercury in aquatic ecosystem from the aquaculture. Environ. Chem. 31, 1782–1786.
Yáñez J., Guajardo M., Miranda C., Soto C., Mansilla H. D., Flegal A. R. (2013). New assessment of organic mercury formation in highly polluted sediments in the Lenga estuary, Chile. Mar. Pollut. Bull. 73, 16–23. doi: 10.1016/j.marpolbul.2013.06.015
Yu C., Xiao W., Xu Y., Sun X., Li M., Lin H., et al. (2021). Spatial-temporal characteristics of mercury and methylmercury in marine sediment under the combined influences of river input and coastal currents. Chemosphere 274, 129728. doi: 10.1016/j.chemosphere.2021.129728
Zhao L., Wang R., Zhang C., Yin D., Yang S., Huang X. (2019). Geochemical controls on the distribution of mercury and methylmercury in sediments of the coastal East China Sea. Sci. Total Environ. 667, 133–141. doi: 10.1016/j.scitotenv.2019.02.334
Keywords: Hg, MeHg, methylation potential, mangrove forest, oyster farm, sediment
Citation: Zhao Z, Chen C and Feng M (2024) Impacts of oyster farms on sediment-associated mercury and methylmercury concentrations and health risks in an estuarine, mangrove forest, Zhanjiang Bay, China. Front. Mar. Sci. 11:1447272. doi: 10.3389/fmars.2024.1447272
Received: 11 June 2024; Accepted: 15 August 2024;
Published: 02 September 2024.
Edited by:
Wen-Hong Liu, National Kaohsiung University of Science and Technology, TaiwanReviewed by:
Fabiana Corami, National Research Council (CNR), ItalyMartin Jara-Marini, National Council of Science and Technology (CONACYT), Mexico
Copyright © 2024 Zhao, Chen and Feng. This is an open-access article distributed under the terms of the Creative Commons Attribution License (CC BY). The use, distribution or reproduction in other forums is permitted, provided the original author(s) and the copyright owner(s) are credited and that the original publication in this journal is cited, in accordance with accepted academic practice. No use, distribution or reproduction is permitted which does not comply with these terms.
*Correspondence: Chunliang Chen, MTgyMTgyNTI4MzlAMTM5LmNvbQ==