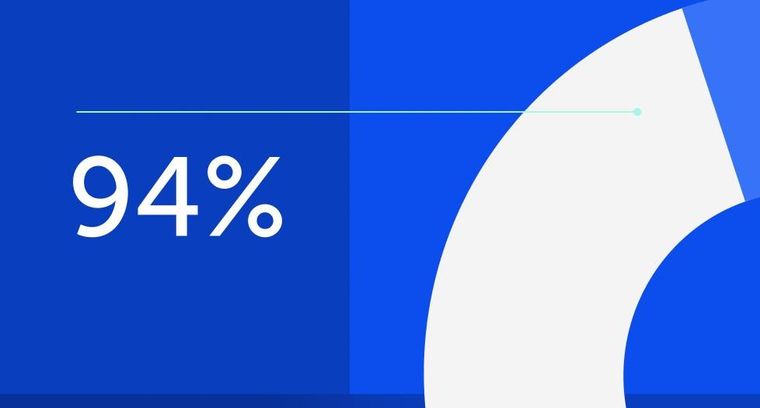
94% of researchers rate our articles as excellent or good
Learn more about the work of our research integrity team to safeguard the quality of each article we publish.
Find out more
ORIGINAL RESEARCH article
Front. Mar. Sci., 19 July 2024
Sec. Global Change and the Future Ocean
Volume 11 - 2024 | https://doi.org/10.3389/fmars.2024.1440734
In recent years, the periodic outbreak of green tides in the coastal areas of China, caused by the combined effects of environmental changes and human activities, has been attracting extensive attention due to the serious negative impacts on the coastal marine ecosystem. In the study, the samples of Ulva linza, a green tide species, were cultivated under two light intensities (LL: 80 μmol photons m-2 s-1; HL: 300 μmol photons m-2 s-1) and three stocking densities (LD: 0.2 g L-1; MD:1 g L-1; HD:2 g L-1) to explore the photosynthetic physiological responses and nutrients absorption capacity. The results showed that high light and low density significantly increased the growth rate of U. linza. Under the HLLD, the maximum growth rate of U. linza was 43.13% day-1 and the energy captured per unit reaction center for electron transfer (ET0/RC) was the highest. The higher density significantly decreased the maximum relative electron transfer rate (rETRmax) of U. linza, especially among groups subjected to high-light condition. Under HL condition, HD also significantly decreased light utilization efficiency (α) in U. linza. The contents of chlorophyll a, b and carotenoids of U. linza were significantly lower in HLLD group compared to other treatment groups. The P uptake of U. linza was prominently inhibited by higher density, and the maximum P uptake and minimum P uptake was 17.94 μM g-1 FW day-1 in LLLD group and 2.74 μM g-1 FW day-1 in LLHD group, respectively. Lower density improved N uptake of U. linza, but high light had no effect on it. These results suggest that high light and lower density synergistically promote the growth of U. linza, which is likely due to enhanced photosynthetic efficiency and nutrient uptake. And the inhibitory effects of higher densities on growth, particularly under high-light conditions, may be due to increased competition for light and nutrients. In the late stage of the green tides outbreak, an increase in accumulation density could help to suppress the sustained outbreak of the green tides, particularly in high-light condition.
Under the combined influence of various factors such as environmental changes and human activities, the coastal areas of China have been experiencing the worldwide large-scale green tides since 2007 (Li et al., 2022). Among them, the Southern Yellow Sea of China has been particularly affected, with successive years of Ulva tide disaster. The green tide drifted in a south to north direction, covering an area of 13,000-30,000km2 between 2007 and 2019 (Zhang et al., 2019; Sun et al., 2022). In 2022, the maximum distribution area reached 18,002 km2, with the maximum coverage areas exceeding 135 km2 (Xia et al., 2024). The biomass of attached Ulva species on Pyropia aquaculture rafts in the Yellow Sea was ranged from 4000 to 6500 tons (Liu et al., 2021). Green tide outbreaks seriously affected the near-shore landscape environment and damaged to the marine ecosystem (Gao et al., 2016), because of high frequency outbreaks, wide distribution, long duration, and deep harm (Zhang et al., 2015). Moreover, it was also a major ecological disaster which troubled the livelihoods of coastal communities, resulting in substantial economic losses and attracting extensive attention and high priority globally (Wang et al., 2019).
In many coastal areas, macroalgal blooms are often dominated by a single green algal species out of several co-occurring ones. Ulva has frequently been identified as the predominant species responsible for green tides and marine fouling (Luo et al., 2012). Ulva linza is a common green macroalgae in China and is also one of important species contributing to the early outbreaks of the green tides (Kang et al., 2016). It exhibits a wide range of tolerance for temperature and salinity, characterized by fast and versatile reproduction (Li et al., 2008). With better environmental adaptability, strong nutrient uptake capacity, and strong resistance to adversity, U. linza plays a significant role in dynamics of green tide outbreaks. The outbreaks of green tide were influenced not only by the intrinsic physical and chemical properties of the algae, but also affected by the environmental factors at the sites of outbreak, such as temperature, nutrients, light and so on (Fan et al., 2020).
Among these factors, light serve as the main source of energy for photosynthesis in algae, significantly influencing their growth rate and photosynthetic responses (Wu et al., 2018). The light intensity requirements of seaweeds exhibit evident species variations. In general, the optimal range of individual light intensity lies between compensated and saturated light intensities (Singh and Singh, 2015). At low light intensities, the light energy utilization rate and the electron transport rate of algae were slower, leading to weakened photosynthetic activity and reduced growth rates (Wang et al., 2021). However, low-light intensity can promote the synthesis of photosynthetic pigments in species such as Ulva prolifera (He et al., 2020), Caulerpa lentillifera (Guo et al., 2015) and Gracilaria lemaneiformis (Wu et al., 2015), serving as a compensatory response to the reduced efficiency in light energy utilization. The light intensity can be appropriately increased within a specific range to enhance the utilization rate of light energy by algae, as well as to augment the energy utilized by photosystem II (PSII) for electron transport, leading to increased photosynthetic activity and growth rates (Yan et al., 2022). However, excessive light intensity can lead to photoinhibition, leading to a deceleration in electron transport rate and a subsequent decrease in photosynthetic activity (Murata et al., 2007).
Density is a crucial environmental factor affecting the photosynthesis and growth of macroalgae (Richards et al., 2011; Li et al., 2020). The density of algae is relatively low in the early stage of green tides; however, as it continues to grow and reproduce, biomass accumulates and density increases. The increase in algal density results in reductions of the electron transport rate and light energy utilization rate of algae, thereby limiting photosynthesis (Jiang et al., 2019). Furthermore, the elevation of stocking density leads to a closer aggregation of algae, hindering the water flow inside the algae and resulting in a slower exchange rate of CO2 and nutrients required for photosynthesis. The competition for nutrients and space within algae species becomes more intense as the density increases, leading to a decrease in the relative growth rate (Hurd, 2000). This phenomenon has been observed in macroalgae such as Undaria pinnatifida (Richards et al., 2011), Sargassum fusiforme, and Ulva lactuca (Cao et al., 2022). However, there is limited research examining how density affects different stages of Ulva outbreaks.
The effects of light and density on the photosynthesis and growth of algae are indisputable, and these two factors are closely interconnected. Higher stocking densities lead to a reduction in light transmittance due to mutual occlusion among algae, resulting in weakened light intensity. Conversely, low stocking densities result in relatively stronger light intensity (Hurd, 2000). While numerous experts and scholars have conducted extensive research on the photosynthetic responses of algae to varying light intensity and stocking density, there is a lack of reports regarding the combined impacts of these two factors on Ulva outbreaks.
The present study aims to investigate the photosynthetic and nutrients absorption responses of U. linza under different light intensities and stocking densities, encompassing low and high light levels as well as low, medium, and high stocking densities. These findings provide additional perspectives on understanding the outbreaking mechanism behind different stages of green tide outbreaks.
The samples of Ulva linza were collected on March 12, 2023 from Yueliang Bay (37°53’N,121°43’E) in Yantai City, China. The collected samples were placed in a temperature-controlled collection box (4°C) and promptly transported to the laboratory within 2 hours. After being washed with filtered and sterilized seawater, healthy U. linza samples were carefully selected and inoculated with 5 L sterilized seawater enriched with VSE medium (Ott, 1965) for 2 days in a controlled environment incubator (GXZ-380D, Ningbo Jiangnan Instruments, China). The cultured temperature was maintained at 15°C, the light intensity was set to 80 ± 10 μmol photons m-2 s-1 with a light and dark period of 12:12 hours, and continuous aeration was provided for 24 hours. After pre-culture, 0.1, 0.5 and 1g fresh weight (FW) of healthy U. linza with similar size (about 4-5 cm) were separately weighed and placed in conical flasks containing 500 mL sterilized seawater with VSE medium. The experiment involved three different stocking densities: low density (LD: 0.2 g L-1), medium density (MD: 1 g L-1) and high density (HD: 2 g L-1), and the density of 1 g L-1 was considered as the reference point for the outbreak of green tides (Gao et al., 2019). At the same time, low light intensity (LL: 80 μmol photons m-2 s-1) and high light intensity (HL: 300 μmol photons m-2 s-1) were set respectively, and they were divided into 6 groups (with 3 parallel groups in each treatment). Among them, the optimal light intensity for the growth of Ulva was about 80 μmol photons m-2 s-1, with a surface light intensity of seawater ranging from 300−400 μmol photons m-2 s-1 during the early stage of the outbreak of Ulva blooms (Jiang et al., 2020). The temperature was set to 20°C with 24-hour uninterrupted aeration and the cultured medium was replaced every two days. All the parameters were measured at the end of experiment.
The relative growth rate of the U. linza was obtained by measuring the fresh weight every two days with an electronic balance (FB244, Shanghai Shunyuhengping Scientific Instrument, China) when cultured medium was changed. During the measurement process, the exposure time of the algae in the air would better be minimized to mitigate potential damage to its activity, and subsequently, the initial density of the algae was restored upon completion of the measurement (Wu et al., 2010). The relative growth rate (RGR, % day-1) was calculated following Equation (1).
Where t is the number of days; Wt is the fresh weight (g) at days t; W0 is the initial fresh weight (g) of algae.
The photosynthetic efficiency of U. linza was assessed using a handheld chlorophyll fluorometer (AquaPen AP-C100, Photon Systems Instruments, Czech Republic). The algal samples were acclimated in darkness for 20 minutes prior to the measurement, and the rapid light response curves (RLC) were recorded using 8 photosynthetic active radiation (PAR) gradients of 0, 10, 20, 50, 100, 300, 500 and 1000 μmol photons m-2 s-1 for a duration of 10 seconds per light intensity. According to the equations proposed by Eilers and Peeters (Eilers and Peeters, 1988), the maximum relative electron transport rate (rETRmax), light energy utilization efficiency (α) and saturated light intensity (Ik) can be calculated using the Equations 2–5.
Where a, b and c in the formulas are fitting parameters.
The parameters were measured using a double-modulation chlorophyll fluorometer (FL6000, Photon Systems Instruments, Czech Republic) after subjecting an appropriate quantity of well-cultivated algae to a 15-minute period of dark adaptation. The fluorescence signals were recorded with a scanning time that ranged from 10 μs to 2 s and were labeled as O, J (at about 2 ms), I (at about 30 ms), and P. When plotting the curve, the logarithmic form of the horizontal coordinate was utilized to enhance visualization of the OJIP phase, thereby facilitating direct observation of its J and I points and inferring the state of the PSII reaction center. The relevant parameters of the OJIP curve were calculated following Equations 6–12 (Strasser et al., 2000).
Where the OJIP curve provided information on the light energy absorbed per unit reaction center (ABS/RC), the light energy dissipated (DI0/RC), the energy captured per unit reaction center for the reduction of QA (TR0/RC), the energy captured per unit reaction center for electron transfer (ET0/RC), the degree of closure of active reaction centers at 2 ms (VJ), the initial slope (M0), and the degree of opening of active reaction centers at 2 ms (Ψ0).
On the last day of the experiment, approximately 0.02 g (FW) of U. linza thalli was collected from each conical bottle and transferred into a centrifugal tube with 5 mL of anhydrous methanol, and followed by extraction in a dark environment at a low temperature of 4°C for 24 hours. The supernatant was transferred to a quartz colorimeter, and the absorbance at 470 nm, 653 nm, and 666 nm were measured using an ultraviolet spectrophotometer (UH5300, Hitachi High-tech Science, Japan). The contents of Chlorophyll a, Chlorophyll b and Carotenoid in U. linza were calculated based on the equation reported by Wellburn (Wellburn, 1994).
The soluble protein (SP) content in U. linza was determined using the Coomassie Brilliant Blue G-250 method reported by Bradford (Bradford, 1976). Under each cultured condition, 0.05g FW of algal thalli was taken, adding 1mL of 0.1mol/L phosphate buffer (pH=7.0), and was fully ground and extracted by GeneReady UltraCool (BSH-C2, Hangzhou Suizhen Biotechnology Co, China). The samples were then subjected to centrifugation at 6000 r/min for 15 minutes by centrifuge (Microfuge 20R, Germany). The supernatants (0.05mL) was diluted to ten times and mixed with G-250 dyeing reagent (1.5mL). After mixing, the absorbance at a wavelength of 595 nm was measured using a Full-wavelength Enzyme Labeler (INFINITE 200 PRO, Austria) to determine the SP content.
At the ending of the experiment, algal samples were collected from each treatment and their tissue contents (C, N) were quantified. The samples were dried at 60°C to constant weight, and then ground into powder by GeneReady UltraCool (BSH-C2, Hangzhou Suizhen Biotechnology Co., LTD., China). The contents of C and N elements in the algae were determined by Elemental Analyzer (MACRO, Almonta Trading (Shanghai) Co., LTD., China).
Phosphorus content in seawater was determined using Phosphorus Molybdenum Blue Spectrophotometry (Shyla et al., 2010). The nitrogen content in seawater was assessed using 220nm ultraviolet spectrophotometry (Collos et al., 1999). The nutrients uptake rate (NUR) of algae was evaluated based on the changes in nutrient levels before and after a 2-day period. The formula of NUR was Equation 13.
Where N0 represents the initial concentration of nitrogen or phosphorus; Nt denotes the concentration of nitrogen or phosphorus after a 2-day period; V represents the volume of cultured algae; FW indicates the fresh weight of algae; the variable t stands for cultured time.
Results were analyzed using Microsoft Excel and presented as the mean ± standard deviation (X+SD) (n=3). IBM SPSS Statistics 27.0 was employed for data processing and analysis. GraphPad Prism 8 and Origin 2021 were utilized to plot the data. Two-way ANOVA was conducted to analyze the effects of light intensity and stocking density on the growth, photosynthetic physiological parameters and nutrient absorption of U. linza. Post hoc examination was performed using Duncan’s multiple comparison test, with a significance level of P<0.05.
The results of Two-way ANOVA (Duncan) revealed that both light intensity and stocking density had significant effects on the relative growth rate (RGR) of U. linza (P<0.05), with a significant interaction between them (P<0.05) (Supplementary Table S1). Specifically, under identical light condition, the RGR decreased with increasing density. The RGR of the HLLD and LLLD groups was 43.13% day-1 and 18.85% day-1, respectively (Figure 1). Moreover, compared to the HLLD treatment group, the RGR of U. linza in both MD treatment group and HD treatment group decreased by 37.69% (HLMD) and 57.34% (HLHD) under HL condition, respectively; while under LL condition, it decreased by 43.36% (LLMD) (P<0.05) and 56.31% (LLHD) (P<0.05), respectively, and there was no significant difference was observed between LLMD and LLHD groups (P>0.05) (Figure 1). Additionally, RGR was significantly higher in the HL treatment group compared to LL treatment group overall at the same stocking density (P<0.05) (Figure 1).
Figure 1 The relative growth rate (RGR) of U. linza grown under three stocking densities (LD: 0.2 g L-1; MD: 1 g L-1; HD: 2 g L-1) and tow light intensities(LL: 80 μmol photons m-2 s-1; HL: 300 μmol photons m-2 s-1)treatments. Data are reported as means ± SD (n = 3). Different lowercase letters above the error bars represent significant differences between the treatments (P< 0.05).
The photosynthetic properties of U. linza under different treatment conditions were shown (Figure 2; Table 1). The rETRmax of U. linza was significantly influenced by light intensity and stocking density, and their interaction (P<0.05). Light intensity and stocking density didn’t have a significant effect on α and Ik (P>0.05), and their interaction only had a significant effect on α (P<0.05) (Supplementary Table S1). Under HL condition, the rETRmax was significantly reduced only in the HD (46.00 μmol e- m-2 s -1) treatment group (P<0.05); Under LL condition, both the MD (47.26 and 45.24 μmol e- m-2 s -1) and HD treatment groups showed a significant decrease in rETRmax(P<0.05), with no significant difference between them (P>0.05) (Table 1). At the same density condition, the HL treatment group exhibited a significantly higher rETRmax than the LL treatment group under LD and MD conditions (P<0.05) (Table 1). The difference in α and Ik were not statistically significant between the HL group and LL group at the same density(P>0.05) (Table 1). Under HL condition, α in the HD group was significantly lower than that in the LD and MD groups (P<0.05); Under LL condition, density had no significant effect on α (P>0.05) (Table 1). Density didn’t have a significant effect on Ik under HL condition (P>0.05) (Table 1). However, under LL condition, there was a progressive decrease in Ik with increasing density, and a significant difference was observed between HD (160 μmol photons m-2 s-1) group and LD (135 μmol photons m-2 s-1) group (P<0.05) (Table 1).
Figure 2 Rapid light curves (RLCs) of U. linza grown under different treatments (HLLD: 300 μmol photons m-2 s-1, 0.2 g L-1; HLMD: 300 μmol photons m-2 s-1, 1 g L-1; HLHD: 300 μmol photons m-2 s-1, 2 g L-1; LLLD: 80 μmol photons m-2 s-1, 0.2 g L-1; LLMD: 80 μmol photons m-2 s-1, 1 g L-1; LLHD: 80 μmol photons m-2 s-1, 2 g L-1). Data are reported as means ± SD (n = 3). Different lowercase letters above the error bars represent significant differences between the treatments (P< 0.05).
Table 1 The best fitting parameters of rapid light response curve of U. linza grown under different treatments (HLLD: 300 μmol photons m-2 s-1, 0.2 g L-1; HLMD: 300 μmol photons m-2 s-1, 1 g L-1; HLHD: 300 μmol photons m-2 s-1, 2 g L-1; LLLD: 80 μmol photons m-2 s-1, 0.2 g L-1; LLMD: 80 μmol photons m-2 s-1, 1 g L-1; LLHD: 80 μmol photons m-2 s-1, 2 g L-1). rETRmax is the maximum relative electron transport rate; α is light energy utilization efficiency; Ik is saturated light intensity.
Under different treatments, U. linza showed typical OJIP curves, encompassing phases O, J, I and P. The light intensity exerted significant effects on VJ, ψ0, ABS/RC, TR0/RC, ET0/RC and DI0/RC (P<0.05). Density only had a significant effect on ABS/RC (P<0.05), while the interaction effects between light intensity and density was observed in VJ, ψ0, and ET0/RC (P<0.05) (Supplementary Table S1). The VJ in the HL treatment group was significantly lower than that in the LL treatment group under identical density condition (P<0.05) (Figure 3). The ψ0 of the HL treatment group was only significantly higher than that of the LL treatment group under LD and MD conditions (P<0.05) (Figure 3). Density had no significant effect on ψ0 under HL condition (P>0.05), while ψ0 in the LD treatment group was significantly lower than that in the HD treatment group under LL condition (P<0.05) (Figure 3).
Figure 3 OJIP curves of U. linza grown under LL treatment (A), HL treatment (B). And the energy absorbed per unit reaction center (ABS/RC), the energy dissipated per unit reaction center (DI0/RC), the energy captured per unit reaction center for the reduction of QA (TR0/RC), the energy captured per unit reaction center for electron transfer (ET0/RC), relative variable fluorescence (VJ), initial slope of the OJIP fluorescence induction curve (M0), and the degree of opening of active reaction centers (Ψ0) of U. linza grown under different treatments (C) (HLLD: 300 μmol photons m-2 s-1, 0.2 g L-1; HLMD: 300 μmol photons m-2 s-1, 1 g L-1; HLHD: 300 μmol photons m-2 s-1, 2 g L-1; LLLD: 80 μmol photons m-2 s-1, 0.2 g L-1; LLMD: 80 μmol photons m-2 s-1, 1 g L-1; LLHD: 80 μmol photons m-2 s-1, 2 g L-1).
For ABS/RC, the HL treatment group exhibited a 12.73%, 10.78% and 15.08% higher response compared to the LL treatment group with increasing density, respectively (P>0.05) (Figure 3). Under the same light intensity, the LD treatment group showed a higher response than the MD and HD treatment groups (P>0.05) (Figure 3). In terms of TR0/RC, the HL treatment group exhibited higher values compared to the LL treatment group at equivalent densities (P>0.05) (Figure 3). Under identical light intensity conditions, the LD treatment group demonstrated higher values than both the MD and HD treatment groups without statistically significant differences (P>0.05) (Figure 3). The ET0/RC of the HL treatment group was only significantly higher than that of the LL treatment group under LD and MD conditions (P<0.05) (Figure 3). Under HL condition, the ET0/RC in the LD treatment group was significantly higher than that in the MD and HD treatment groups (P<0.05) (Figure 3). For DI0/RC, the HL treatment groups showed higher values compared to the LL treatment groups at the same density; however, no statistically significant difference among them were observed (P>0.05) (Figure 3).
The interaction of light intensity and density, light intensity had significant effects on the pigment contents (Chl a, Chl b, Car.) of U. linza (P<0.05), but density had no significant effect on them (P>0.05) (Supplementary Table S1). The contents of Chl a, Chl b and Car. in HLLD treatment group were (1.886 ± 0.176) mg/g, (1.160 ± 0.115) mg/g and (0.457 ± 0.036) mg/g, respectively, which were significantly lower than those in the other treatment groups (P<0.05) (Figures 4A–C). No significant differences were observed in the pigment contents among the other treatment groups (P>0.05) (Figures 4A–C). The content of soluble protein in U. linza wasn’t significantly affected by light intensity, density or their interaction (P>0.05) (Supplementary Table S1). There were no significant differences observed among the different treatment groups (P>0.05) (Figure 4D).
Figure 4 The Chlorophyll a (A), Chlorophyll b (B), Carotenoid (C) and Soluble protein (D) contents of U. linza grown under different treatments (LD: 0.2 g L-1; MD: 1 g L-1; HD: 2 g L-1; LL: 80 μmol photons m-2 s-1; HL: 300 μmol photons m-2 s-1). Data are reported as means ± SD (n = 3). Different lowercase letters above the error bars represent significant differences between the treatments (P< 0.05).
The light intensity, density and their interaction didn’t have a significant impact on the Tissue C, Tissue N and C: N of U. linza (P>0.05) (Supplementary Table S1). The carbon content of dry weight in all treatment groups averaged approximately 30%, nitrogen content ranged from 3.23% to 3.54%, and C:N was about 9 (Figure 5).
Figure 5 Tissue carbon (A), nitrogen (B) and C:N ratio (C) of U. linza grown under different treatments (LD: 0.2 g L-1; MD: 1 g L-1; HD: 2 g L-1; LL: 80 μmol photons m-2 s-1; HL: 300 μmol photons m-2 s-1). Data are reported as means ± SD (n = 3). Different lowercase letters above the error bars represent significant differences between the treatments (P< 0.05).
The density had a significant impact on the N uptake rate (P<0.05), while the light intensity and their interaction didn’t have a significant effect on it (P>0.05) (Supplementary Table S1). With increasing density, under HL condition, both MD and HD treatment groups showed a significant decrease of 53.32% and 70.57% respectively, compared to the LD treatment group (P<0.05) (Figure 6A). Similarly, under LL condition, both MD and HD treatment groups exhibited a significant decrease of 67.45% and 81.03% respectively, compared to the LD treatment group (P<0.05) (Figure 6A). There was no significant difference between the MD and HD treatment groups (P>0.5) (Figure 6A).
Figure 6 N uptake rate (A) and P uptake rate (B) of U. linza grown under different treatments (LD: 0.2 g L-1; MD: 1 g L-1; HD: 2 g L-1; LL: 80 μmol photons m-2 s-1; HL: 300 μmol photons m-2 s-1). Data are reported as means ± SD (n = 3). Different lowercase letters above the error bars represent significant differences between the treatments (P< 0.05).
The light intensity didn’t have a significant impact on the P uptake rate (P>0.05), whereas density and their interaction had significant effects on it (P<0.05) (Supplementary Table S1). Under the same light condition, U. linza exhibited a significant decrease in P uptake rate with increasing density (P<0.05), transitioning from HLLD (15.00 μMg-1 FW day-1) to HLMD (6.80 μMg-1 FW day-1), and finally to HLHD (4.12 μMg-1 FW day-1) (Figure 6B). Furthermore, under LD condition, the LL treatment group showed significantly higher P uptake rate at17.94 μMg-1 FW day-1 compared to the HL treatment group at 15.00 μMg-1 FW day-1 (P<0.05); Under MD condition, the HL treatment group demonstrated a significantly higher P uptake rate than the LL treatment group at 5.03 μMg-1 FW day-1 (P<0.05) (Figure 6B). Under HD condition, there was no significant difference between the HL treatment group and LL treatment group (P>0.05) (Figure 6B).
This study attempted to determine the combined effects of light intensity and stocking density on photosynthesis and nutrients uptake of U. linza (a bloom-forming green alga). Overall, the growth of U. linza was influenced by both light intensity and stocking density. The most important finding of this investigation was that high light and low density synergistically promote the growth of U. linza, and the inhibitory effects of higher densities on Ulva’s growth are more pronounced under high-light condition.
The growth and photosynthesis of macroalgae are influenced by multiple factors (Xue et al., 2023), including light intensity, density, nutrients, temperature etc. Light serves as the fundamental energy source for assimilatory power during the process of algal photosynthesis, and appropriate light conditions are crucial for their rapid growth and reproduction (Xiao et al., 2016). Studies have demonstrated that the light reaction stage of algal photosynthesis was impeded under low-light condition (light-restricted state), resulting in insufficient production of H+ and ATP to support the dark reaction stage, consequently leading to slow growth due to inadequate accumulation of photosynthetic organic matter (Meyer et al., 2015). However, optimal high-light condition has been shown to significantly enhance the relative electron transport rate, net photosynthetic rate and growth of U. prolifera (Wu et al., 2018), S. horneri (Yan et al., 2022) and P. umbilicalis (Green and Neefus, 2016), which aligns with our findings. In this study, both the relative electron transport rate and growth rate of U. linza were notably higher in the HL treatment groups compared to those in the LL treatment groups. The chlorophylls, as the most crucial pigment molecule in photosynthesis, play a vital role in the absorption and transmission of light energy and the primary photochemical reaction, which is the foundation of algal photosynthesis (Logan et al., 2023). The results of our study demonstrated that the contents of Chl a, Chl b, and Car. in the HLLD treatment group were significantly lower than those in other treatment groups. This observation suggested that algae can enhance their production of photosynthetic pigments under low-light condition to optimize light energy absorption for maintaining normal growth, and reduce pigments synthesis under higher light conditions to save energy towards other biosynthesis and metabolic pathways, which was a mechanism for algae to actively respond to changes in the external environment (Zhukova and Yakovleva, 2021; Zhou et al., 2022). This negative correlation between pigment and light intensity was also commonly observed in other types of algae such as U. prolifera (Zhao et al., 2016).
In addition, chlorophyll fluorescence can also quickly, accurately and harmlessly detect the state of plant photosynthesis, which reflected the influence of environment on plants through the changes of fluorescence parameters (Kalaji et al., 2012). The OJIP curve demonstrated that ψ0 was consistently higher under HL condition compared to the LL treatment group, indicating that high light condition facilitated electron transport on the PSII acceptor side of U. linza. Furthermore, the confirmation was further supported by the observed increase in ET0/RC under high-light condition and the elevated relative variable fluorescence at the J-point (the impeded electron transport from QA to secondary quinone receptor QB on the PSII side) under low-light condition. These findings collectively supported strong photosynthetic capacity and rapid growth rate of U. linza under high light condition. The rapid growth and large-scale outbreaks of U. linza can be attributed to its high capacity for nutrients absorption (Fan et al., 2020), with light intensity playing a crucial role in facilitating this process. Appropriate light intensity promoted the absorption of N and P by algae, and increased with the increase of light intensity within a certain range (Shi et al., 2015). However, the results of this study showed that light intensity had no significant effect on N absorption, while significantly affected P absorption. This difference may be due to the varying effects of high-light intensity on N and P absorption, potentially caused by differences in photosynthetic demand. Compared to HLLD treatment, The increased absorption rate of P under LLLD could compensate for energy and carbon source deficiencies, thus supporting the photosynthesis and growth of algae (Li et al., 2016).
The changes in growth density will result in alterations in environmental factors, including light intensity, CO2 concentration, seawater pH and nutrients within seaweed populations. Consequently, these changes will impact the growth and photosynthetic capacity of macroalgae. Regarding the impact of density on algae, Li et al. reported that high density led to a reduction in light energy utilization efficiency, maximum photosynthetic oxygen releasing rate and dark respiration rate in U. conglobata, thereby influencing the physiological response of algae to environmental changes (Li et al., 2020). Additionally, high density also decreased the relative electron transport rate and relative growth rate of U. lactuca (Cao et al., 2022). Consistent with these findings, regardless of high or low light conditions, the relative electron transport rate and relative growth rate of U. linza were consistently the lowest in the HD treatment groups. In the natural environment, as the algae grew and developed, their densities gradually increased. Consequently, the algae adaptively decreased their light harvesting efficiency and photosynthetic efficiency (Jiang et al., 2017), thereby further inhibiting their growth.
Under the condition where high density affected light intensity, the algae underwent physiological adjustments to adapt to lower light levels and enhanced pigment accumulation to mitigate the issue of reduced light intensity caused by high density (Zou, 2005). In this experiment, as density increased, the regulatory effect under high light conditions became more pronounced. Under medium and high densities, U. linza exhibited an increase in pigment content to facilitate the utilization of light energy by photosynthetic pigments. Additionally, as the stocking density increased, so did the biomass and the consumption of nutrients. This can lead to carbon or nutrient restrictions in macroalgae under high density conditions. Kang et al. showed that the nutrients uptake at thallus stocking densities of 5 and 10 g FW L–1 were significantly higher than that at 20 g FW L–1 (Kang et al., 2014). The results of this study also demonstrated that low density facilitated the absorption rate of N and P simultaneously, while under low-light condition the absorption rate of P was further enhanced. Under low-density condition the algae faced minimal competition, enabling them to efficiently absorb nutrients such as nitrogen and phosphorus from seawater for algal growth. Excess nutrients can be stored as an energy reservoir (Xu and Gao, 2012). However, in the presence of low light and low density, U. linza experienced inhibition of photosynthetic efficiency. The acquisition of more P may contribute to the synthesis of ATP, proteins and other biological macromolecules, thereby promoting the photosynthesis and growth of U. linza (Li et al., 2016).
Our finding demonstrated that the photosynthetic process and nutrient absorption capacity of U. linza were significantly influenced by both light intensity and stocking density. The growth of U. linza can be enhanced by high-light intensity and lower density synergistically in this study, with the highest growth rate observed under HLLD condition. Moreover, high density exerted a more pronounced inhibitory effect on the photosynthesis and growth of U. linza while light intensity was high. However, under low-light condition, an increase in density had a relatively minor impact on their photosynthesis and growth. Simultaneously, high density hindered the absorption of N and P by U. linza. Due to the climate change, such as global warming, there has been an increase in light intensity on the mixed layer in recent years. During the late stage of green tide outbreaks, there is a notable increase in the accumulation density of Ulva. This suggests that the interaction between light availability and Ulva’s stocking density plays a significant role in shaping the persistence and scale of green tides events. Considering the annual variations and increasing distribution areas since 2007, understanding these interactions are crucial for grasping the broader ecological implications of green tide phenomena in marine ecosystems.
The original contributions presented in the study are included in the article/Supplementary Material. Further inquiries can be directed to the corresponding authors.
MB: Conceptualization, Funding acquisition, Writing – review & editing. QZ: Conceptualization, Writing – original draft. ZHX: Formal analysis, Writing – review & editing. BL: Investigation, Writing – review & editing. HW: Funding acquisition, Writing – review & editing. SZ: Methodology, Writing – review & editing. FY: Data curation, Writing – review & editing. ZGX: Conceptualization, Funding acquisition, Project administration, Writing – review & editing. NL: Conceptualization, Project administration, Resources, Supervision, Writing – review & editing.
The author(s) declare financial support was received for the research, authorship, and/or publication of this article. This study was supported by the Shandong Provincial National Science Foundation of China (ZR2023QD091) and the National Natural Science Foundation of China (42376107; 42276119).
The authors declare that the research was conducted in the absence of any commercial or financial relationships that could be construed as a potential conflict of interest.
All claims expressed in this article are solely those of the authors and do not necessarily represent those of their affiliated organizations, or those of the publisher, the editors and the reviewers. Any product that may be evaluated in this article, or claim that may be made by its manufacturer, is not guaranteed or endorsed by the publisher.
The Supplementary Material for this article can be found online at: https://www.frontiersin.org/articles/10.3389/fmars.2024.1440734/full#supplementary-material
Bradford M. M. (1976). A rapid and sensitive method for the quantitation of microgram quantities of protein utilizing the principle of protein-dye binding. Anal. Biochem. 72, 248–254. doi: 10.1006/abio.1976.9999
Cao C., Zhang T. T., Wu M. J., Chen B. B., Ma Z. L. (2022). Differential growth and physiological responses of Sargassum fusiforme and epiphytic Ulva lactuca to culture densities and interspecific competition. Reg. Stud. Mar. Sci. 56, 102671. doi: 10.1016/j.rsma.2022.102671
Collos Y., Mornet F., Sciandra A., Waser N., Larson A., Harrison P. J. (1999). An optical method for the rapid measurement of micromolar concentrations of nitrate in marine phytoplankton cultures. J. Appl. Phycol. 11, 179–184. doi: 10.1023/A:1008046023487
Eilers P. H. C., Peeters J. C. H. (1988). A model for the relationship between light intensity and the rate of photosynthesis in phytoplankton. Ecol. Model. 42, 199–215. doi: 10.1016/0304-3800(88)90057-9
Fan X., Xu D., Wang D. S., Wang Y. T., Zhang X. W., Ye N. H. (2020). Nutrient uptake and transporter gene expression of ammonium, nitrate, and phosphorus in Ulva linza: adaption to variable concentrations and temperatures. J. Appl. Phycol. 32, 1311–1322. doi: 10.1007/s10811-020-02050-2
Gao G., Fu Q. Q., Beardall J., Wu M., Xu J. T. (2019). Combination of ocean acidification and warming enhances the competitive advantage of Skeletonema costatum over a green tide alga, Ulva linza. Harmful Algae 85, 101698. doi: 10.1016/j.hal.2019.101698
Gao G., Zhong Z. H., Zhou X. H., Xu J. T. (2016). Changes in morphological plasticity of Ulva prolifera under different environmental conditions: A laboratory experiment. Harmful Algae 59, 51–58. doi: 10.1016/j.hal.2016.09.004
Green L. A., Neefus C. D. (2016). Effects of temperature, light level, and photoperiod on the physiology of Porphyra umbilicalis Kutzing from the Northwest Atlantic, a candidate for aquaculture. J. Appl. Phycol. 28, 1815–1826. doi: 10.1007/s10811-015-0702-6
Guo H., Yao J. T., Sun Z. M., Duan D. L. (2015). Effect of temperature, irradiance on the growth of the green alga Caulerpa lentillifera (Bryopsidophyceae, Chlorophyta). J. Appl. Phycol. 27, 879–885. doi: 10.1007/s10811-014-0358-7
He Y., Ye Y. T., Shen S. D. (2020). Effects of light and salinity on carotenoid biosynthesis in Ulva prolifera. Acta Oceanol. Sin. 39, 50–57. doi: 10.1007/s13131-020-1577-1
Hurd C. L. (2000). Water motion, marine macroalgal physiology, and production. J. phycol. 36, 453–472. doi: 10.1046/j.1529-8817.2000.99139.x
Jiang H., Gong J. Y., Lou W. Y., Zou D. H. (2019). Photosynthetic behaviors in response to intertidal zone and algal mat density in Ulva lactuca (Chlorophyta) along the coast of Nan’ao Island, Shantou, China. Environ. Sci. pollut. R. 26, 13346–133453. doi: 10.1007/s11356-019-04775-1
Jiang J. N., Yu Y. N., Zheng M. S., Liu N. N., Li Y. H., Xu N. J. (2020). High light might alleviate inhibitory effects of high temperature on growth and physiological parameters of Ulva prolifera. Aquac. Res. 51, 2062–2070. doi: 10.1111/are.14557
Jiang H., Zou D. H., Chen W. Z., Yang Y. F. (2017). The photosynthetic responses to stocking depth and algal mat density in the farmed seaweed Gracilaria lemaneiformis (Gracilariales, Rhodophyta). Environ. Sci. pollut. R. 24, 25309–25314. doi: 10.1007/s11356-017-0198-5
Kalaji H. M., Carpentier R., Allakhverdiev S. I., Bosa K. (2012). Fluorescence parameters as early indicators of light stress in barley. J. Photoch. Photobio. B. 112, 1–6. doi: 10.1016/j.jphotobiol.2012.03.009
Kang E. J., Kim J., Kim K., Kim K. Y. (2016). Adaptations of a green tide forming Ulva linza (Ulvophyceae, Chlorophyta) to selected salinity and nutrients conditions mimicking representative environments in the Yellow Sea. Phycologia 55, 210–218. doi: 10.2216/15-67.1
Kang Y. H., Kim S., Lee J., Chung I. K., Park S. R. (2014). Nitrogen biofiltration capacities and photosynthetic activity of Emphasis Pyropia yezoensis/Emphasis Ueda (Bangiales, Rhodophyta): groundwork to validate its potential in integrated multi-trophic aquaculture (IMTA). J. Appl. Phycol. 26, 947–955. doi: 10.1007/s10811-013-0214-1
Li G., Qin Z., Zhang J. J., Lin Q., Ni G. Y., Tan Y. H., et al. (2020). Algal density mediates the photosynthetic responses of a marine macroalga Ulva conglobata (Chlorophyta) to temperature and pH changes. Algal Res. 46, 101797–101797. doi: 10.1016/j.algal.2020.101797
Li A. P., Shen S. D., Wang J. W., Yan B. L. (2008). Reproduction diversity of Enteromorpha prolifera. J. Integr. Plant Biol. 50, 622–629. doi: 10.1111/j.1744-7909.2008.00647.x
Li S. X., Yu K. F., Huo Y. Z., Zhang J. H., Wu H. L., Cai C. E., et al. (2016). Effects of nitrogen and phosphorus enrichment on growth and photosynthetic assimilation of carbon in a green tide-forming species (Ulva prolifera) in the Yellow Sea. Hydrobiologia 776, 161–171. doi: 10.1007/s10750-016-2749-z
Li X. S., Zheng Z. T., Xu J. T., Wu S. Q. (2022). Effects of acid treatment on the survival of thallus of Neopyropia yezoensis and Ulva prolifera. J. Appl. Phycol. 34, 3087–3094. doi: 10.1007/s10811-022-02824-w
Liu J. L., Xia J., Zhuang M. M., Zhang J. H., Yu K. F., Zhao S., et al. (2021). Controlling the source of green tides in the Yellow Sea: NaClO treatment of Ulva attached on Pyropia aquaculture rafts. Aquaculture 535, 736378. doi: 10.1016/j.aquaculture.2021.736378
Logan R. D., Torrey M. A., Feijó-Lima R., Colman B. P., Valett H. M., Shaw J. A. (2023). UAV-Based hyperspectral imaging for river algae pigment estimation. Remote Sens. 15, 3148. doi: 10.3390/rs15123148
Luo M. B., Liu F., Xu L. Z. (2012). Growth and nutrient uptake capacity of two co-occurring species, Ulva prolifera and Ulva linza. Aqua. Bot. 100, 18–24. doi: 10.1016/j.aquabot.2012.03.006
Meyer F. W., Vogel N., Teichberg M., Uthicke S., Wild C. (2015). The physiological response of two green calcifying algae from the Great Barrier Reef towards high dissolved inorganic and organic carbon (DIC and DOC) availability. PloS One 10, e0133596. doi: 10.1371/journal.pone.0133596
Murata N., Takahashi S., Nishiyama Y., Allakhverdiev S. I. (2007). Photoinhibition of photosystem II under environmental stress. BBA 1767, 414–421. doi: 10.1016/j.bbabio.2006.11.019
Ott F. D. (1965). Synthetic media and techniques for the xenic cultivation of marine algae and flagellate. Virginia J. Sci. 16, 205–218.
Richards D. K., Hurd C. L., Pritchard D. W., Wing S. R., Hepburn C. D. (2011). Photosynthetic response of monospecific macroalgal stands to density. Aquat. Biol. 13, 41–49. doi: 10.3354/ab00349
Shi P. L., Shen H., Wang W. J., Chen W. J., Xie P. (2015). The relationship between light intensity and nutrient uptake kinetics in six freshwater diatoms. J. Environ. Sci. 34, 28–36. doi: 10.1016/j.jes.2015.03.003
Shyla B., Mahadevaiah, Nagendrappa G. (2010). A simple spectrophotometric method for the determination of phosphate in soil, detergents, water, bone and food samples through the formation of phosphomolybdate complex followed by its reduction with thiourea. Spectrochim. Acta A. 78, 497–502. doi: 10.1016/j.saa.2010.11.017
Singh S. P., Singh P. (2015). Effect of temperature and light on the growth of algae species: A review. Renew. Sust. Energ. Rev. 50, 431–444. doi: 10.1016/j.rser.2015.05.024
Strasser R. J., Srivastava A., Tsimilli-Michael M. (2000). “The fluorescence transient as a tool to characterize and screen photosynthetic samples,” in Probing Photosynthesis Mechanisms Regulation & Adaptation. Eds. Yunus M., Pathre U., Mohanty P. (CRC Press, London, FL), 445–483.
Sun Y. Q., Liu J. L., Xa J., Tong Y. C., Li C. X., Zhao S., et al. (2022). Research development on resource utilization of green tide algae from the Southern Yellow Sea. Energy Rep. 8, 295–303. doi: 10.1016/j.egyr.2022.01.168
Wang C. Y., Su R. G., Guo L. D., Yang B., Zhang Y., Zhang L., et al. (2019). Nutrient absorption by Ulva prolifera and the growth mechanism leading to green-tides. Estuar. Coast. Shelf. S. 227, 106329–106329. doi: 10.1016/j.ecss.2019.106329
Wang Y., Zhong Z. H., Qin S., Li J. L., Li J. J., Liu Z. Y. (2021). Effects of temperature and light on growth rate and photosynthetic characteristics of sargassum horneri. J. Ocean U. China 20, 101–110. doi: 10.1007/s11802-021-4507-8
Wellburn A. R. (1994). The spectral determination of chlorophyll a and chlorophyll b, as well as total carotenoids, using various solvents with spectrophotometers of different resolution. J. Plant Physiol. 144, 307–313. doi: 10.1016/S0176-1617(11)81192-2
Wu H. L., Gao G., Zhong Z. H., Li X. S., Xu J. T. (2018). Physiological acclimation of the green tidal alga Ulva prolifera to a fast-changing environment. Mar. Environ. Res. 137, 1–7. doi: 10.1016/j.marenvres.2018.02.018
Wu H. Y., Jiang H., Liu C. X., Deng Y. Y. (2015). Growth, pigment composition, chlorophyll fluorescence and antioxidant defenses in the red alga Gracilaria lemaneiformis (Gracilariales, Rhodophyta) under light stress. S. Afr. J. Bot. 100, 27–32. doi: 10.1016/j.sajb.2015.05.017
Wu Y. P., Gao K. S., Riebesell U. (2010). CO2-induced seawater acidification affects physiological performance of the marine diatom Phaeodactylum tricornutum. Biogeosciences 7, 2915–2923. doi: 10.5194/bg-7-2915-2010
Xia Z. Y., Liu J. L., Zhao S., Sun Y. Q., Cui Q. W., Wu L. J., et al. (2024). Review of the development of the green tide and the process of control in the southern Yellow Sea in 2022. Estuar. Coast. Shelf S. 302, 108772. doi: 10.1016/j.ecss.2024.108772
Xiao J., Zhang X. H., Gao C. L., Jiang M. J., Li R. X., Wang Z. L., et al. (2016). Effect of temperature, salinity and irradiance on growth and photosynthesis of Ulva prolifera. Acta Oceanol. Sin 35, 114–121. doi: 10.1007/s13131-016-0891-0
Xu Z. G., Gao K. S. (2012). NH4+ enrichment and UV radiation interact to affect the photosynthesis and nitrogen uptake of Gracilaria lemaneiformis (Rhodophyta). Mar. pollut. Bull. 64, 99–105. doi: 10.1016/j.marpolbul.2011.10.016
Xue M. Y., Wu M. Q., Zheng L. X., Liu J. Y., Liu L. X., Zhu S., et al. (2023). Multi-factors synthetically contribute to ulva prolifera outbreaks in the south yellow sea of China. Remote Sens-basel. 15, 5151. doi: 10.3390/rs15215151
Yan F., Jiang H. C., Ma Y. Q., Cui C. J., Qin H. W., Liu L. J., et al. (2022). Combined influences of light and nitrogen enrichment on the physiological performance of a golden tide alga (Sargassum horneri). J. Mar. Sci. Eng. 10, 1195–1195. doi: 10.3390/jmse10091195
Zhang Y. Y., He P. M., Li H. M., Li G., Liu J. H., Jiao F. L., et al. (2019). Ulva prolifera green-tide outbreaks and their environmental impact in the Yellow Sea, China. Natl. Sci. Rev. 6, 825–838. doi: 10.1093/nsr/nwz026
Zhang J. H., Liu C. C., Yang L. L., Gao S., Ji X., Huo Y. Z., et al. (2015). The source of the Ulva blooms in the East China Sea by the combination of morphological, molecular and numerical analysis. Estuar. Coast. Shelf. S. 164, 418–424. doi: 10.1016/j.ecss.2015.08.007
Zhao X. Y., Tang X. X., Zhang H. X., Qu T. F., Wang Y. (2016). Photosynthetic adaptation strategy of Ulva prolifera floating on the sea surface to environmental changes. Plant Physiol. Bioch. 107, 116–125. doi: 10.1016/j.plaphy.2016.05.036
Zhou W., Wu H., Huang J. J., Wang J. G., Zhen W., Wang J. W., et al. (2022). Elevated-CO2 and nutrient limitation synergistically reduce the growth and photosynthetic performances of a commercial macroalga Gracilariopsis lemaneiformis. Aquaculture 550, 737878. doi: 10.1016/j.aquaculture.2021.737878
Zhukova N. V., Yakovleva I. M. (2021). Low light acclimation strategy of the brown macroalga Undaria pinnatifida: significance of lipid and fatty acid remodeling for photosynthetic competence. J. phycol. 57, 1792–1804. doi: 10.1111/jpy.13209
Keywords: green tides, light intensity, photosynthesis, nutrients uptake, stocking density, Ulva linza
Citation: Bao M, Zhang Q, Xu Z, Li B, Wu H, Zang S, Yan F, Xu Z and Liu N (2024) High-light pronounced the effects of stocking density on photosynthesis and nutrients uptake of the bloom-forming green alga, Ulva linza. Front. Mar. Sci. 11:1440734. doi: 10.3389/fmars.2024.1440734
Received: 30 May 2024; Accepted: 05 July 2024;
Published: 19 July 2024.
Edited by:
Gang Li, Chinese Academy of Sciences (CAS), ChinaReviewed by:
Yongyu Zhang, Chinese Academy of Sciences (CAS), ChinaCopyright © 2024 Bao, Zhang, Xu, Li, Wu, Zang, Yan, Xu and Liu. This is an open-access article distributed under the terms of the Creative Commons Attribution License (CC BY). The use, distribution or reproduction in other forums is permitted, provided the original author(s) and the copyright owner(s) are credited and that the original publication in this journal is cited, in accordance with accepted academic practice. No use, distribution or reproduction is permitted which does not comply with these terms.
*Correspondence: Zhiguang Xu, Ymlnd2lkZUAxNjMuY29t; Ning Liu, bG5saXVuaW5nbG5AMTI2LmNvbQ==
†These authors have contributed equally to this work and share first authorship
Disclaimer: All claims expressed in this article are solely those of the authors and do not necessarily represent those of their affiliated organizations, or those of the publisher, the editors and the reviewers. Any product that may be evaluated in this article or claim that may be made by its manufacturer is not guaranteed or endorsed by the publisher.
Research integrity at Frontiers
Learn more about the work of our research integrity team to safeguard the quality of each article we publish.