- 1Ministry of Education and Jiangxi Key Laboratory of Crop Physiology, Ecology and Genetic Breeding, Jiangxi Agricultural University, Nanchang, China
- 2College of Agronomy, Jiangxi Agricultural University, Nanchang, China
- 3Department of Physiology and Biochemistry, Cholistan University of Veterinary and Animal Sciences (CUVAS), Bahawalpur, Pakistan
- 4Institute of Biochemistry and Molecular Biology, College of Life and Health Sciences, Northeastern University, Shenyang, China
Diabetes mellitus (DM), a major global health concern, is a chronic metabolic disorder. Bioactive compounds sourced from numerous marine natural products recently have drawn attention as novel therapeutic approaches. Considering these chemicals and their role in cellular redox modulation by involving the nuclear factor erythroid 2-related factor 2 (Nrf2)/heme oxygenase 1 (HO-1) pathway, the current study attempts to highlight their anti-diabetic effects and the molecular mechanisms involved. Reactive oxygen species (ROS)-mediated oxidative stress, inflammation, and cellular damage are linked to most human pathologies specifically DM. The Nrf2/HO-1 pathway is a key defense mechanism developed by the cells to combat ROS burst. Marine natural compounds have strong pharmacological potential in triggering cellular antioxidant defense mechanisms by declining oxidative damage and inflammation linked to DM. How marine natural products potentially alleviate DM specifically type 2 diabetes (T2D) and its related issues is especially focused on. The literature was thoroughly analyzed to open a discussion about specific marine compounds and their well-established anti-diabetic effects to elucidate possible therapeutic applications. Furthermore, opportunities and the pros and cons of using these marine bioactive compounds as complementary treatment for DM are also discussed. The diverse characteristics of marine natural products, specifically with regard to redox control, offer promising opportunities for drug discovery and therapeutic interventions in clinical trials.
1 Introduction
The World Health Organization (WHO) declared diabetes mellitus (DM) as a major problem alongside chronic respiratory illnesses, cancer, and cardiovascular diseases (CVDs). The rising DM cases have impacted the human development, health, and economical situations of countries (Roglic, 2016). The WHO approved the global framework for non-communicable diseases (NCDs) in 2013 composed of multi-target goals for 2025. DM and its main risk factors include reduction of poor diets and physical inactivity, minimizing the rise in DM prevalence, improving treatment accessibility, and reducing early age deaths (Beaglehole et al., 2011; World Health Organization (WHO), 2018).
DM is caused by insufficient insulin secretion by pancreatic β cells or by an immune system resistant to insulin (Khan et al., 2022; Maestas et al., 2024). Type 1 diabetes (T1D) is a genetic disorder where the pancreas does not produce insulin at all (Galicia-Garcia et al., 2020; Roep et al., 2021). In type 2 diabetes (T2D), body cells develop insulin resistance that causes a progressive decrease in insulin production, and gestational diabetes during pregnancy results in complications during pregnancy and birth (Galicia-Garcia et al., 2020; Choi et al., 2024; Maestas et al., 2024). T2D risk is increased by gestational diabetes and later on by poor lifestyle (Eizirik et al., 2020; Elliott et al., 2024). Two intermediate stages between normal and diabetic blood glucose levels are impaired fasting glucose (IFG) and impaired fasting glycemia (IGT), and individuals suffering from both of these conditions have a higher risk of developing CVD than a normal person (Ahmadizar et al., 2021; Zuo et al., 2021; Poznyak et al., 2022).
Complementary and alternative medicine (CAM) improves overall health and follows global trends for better life standards. The US National Center for Complementary and Alternative Medicine (NCCAM) defines CAM as a group of medical and healthcare systems, practices, and products that are not presently considered part of conventional medicine. Alternative medicine is used in place of conventional treatment, whereas complementary medicine is used in addition to it (Tangkiatkumjai et al., 2020). Because these marine bioactive compounds have been reported to exert no or little adverse responses than already known drugs, they became an attractive option (Hassan et al., 2022). Along with herbal medicines, massage therapy, acupuncture, faith healing, hypnosis, and music therapies are also included in CAM (Kumar et al., 2006). Medicinal plants cure multiple diseases, and usage of herbal treatments has increased by multi-fold in the USA during the past decade (Vuksan et al., 2000); over three million Americans use CAM therapies against DM (Ranasinghe et al., 2012). In-depth investigation of the underlying molecular mechanisms on how these bioactives work could provide important novel insights for the proper cure of DM. However, the exploration of novel anti-diabetic drugs would be enhanced by the application of certain computational tools (Wang et al., 2020).
DM is treated with continuous insulin dosage or chemical agents such as acarbose, pioglitazone, and miglitol that block digestive enzymes, or oral medication like sulfonylureas and biguanides. Preventing CVD is a major goal during diabetes treatment, and a key concept in this therapy system is to get the patient to have optimal disease control, which includes managing their blood pressure, cholesterol, and glucose levels (ElSayed et al., 2022; Li et al., 2023). Chronic high blood glucose level generates ROS burst, which develops diabetes, diabetes-induced retinal impairment, nephropathy, neuropathy, and CVD (Yuan et al., 2019; Caturano et al., 2023). Consequently, reducing carbohydrate absorption postprandial may be a good way to keep blood glucose levels within the permissible range (Olaokun et al., 2013; Nasab et al., 2020). Synthetic or chemically modified drugs to cure diabetes and associated pathologies are expensive and may have side effects (Groop and Pociot, 2014). The quest for novel and cheaper natural bioactive compounds as alternative drug molecules is a vital component of contemporary pharmaceuticals (Mayer et al., 2010; Yuan et al., 2019; Gagare et al., 2024). Natural resources in oceans are deemed for proper entry into new pharmaceuticals and nutritional supplements (Beygmoradi and Homaei, 2017; Banday et al., 2024). Herein, we highlighted that products derived from marine life could promisingly be the next authentic DM treatment options.
The possible medicinal uses of phytoplanktons and related marine life are not much known. Diseases such as diabetes, Parkinson’s disease (PD), Alzheimer’s disease (AD), and many others can be treated by inhibiting certain enzymes at multiple cellular events (Nowell et al., 2023). The enzymes aldose reductase (AR), protein tyrosine phosphatase, and α-glucosidase are deleterious to T2D and associated disorders. Biologically active insulin, fucoxanthin, dysidine, benzene acetamide, 2-piperidione, n-hexadecanoic acid, and hundreds of other compounds have been isolated from the red or brown algae, diatoms, ascidians, and corals (Bhattacharjee et al., 2014; Pathak et al., 2022). Marine natural products are equipped with certain specialized functional groups on BACs, indicating that the origin of certain specialized enzymes in the cells of those organisms may be of potential therapeutic applications. Moreover, the presence of plenty of reducing sites, –OH, and structural diversity make marine BACs superior to already known plant BACs. Other marine substances are notable examples of inhibitors of these enzymes and have antioxidant potential (Bhattacharjee et al., 2014). Activation of the GLUT-4 receptor improves peripheral glucose consumption and may also control diabetes. Fucoxanthin inhibits the activities of insulin resistance causing protein factors including adipokines, tumor necrosis factor-α (TNF-α), monocyte chemoattractant protein-1 (MCP-1), and interleukin-6 (IL-6). Biological insulin mimicking human insulin has been isolated from spotted dogfish and hammerhead sharks (Sphyrna lewini), which is shown to have a potent receptor binding capability and is a promising new bio-insulin (Bhattacharjee et al., 2014).
2 Redox modulation in diabetes
Marine natural products may stabilize and modulate the production of ROS, and activate or inhibit enzymatic activities to reduce insulin resistance altogether by provoking the Nrf2/HO-1 redox responsive pathway (Figure 1). Imbalance in antioxidants and ROS levels may lead to heavy cellular oxidative stress and chronic conditions may lead to pathologies like diabetes (West, 2000). After macrophages and dendritic cells (DCs) enter the islets, T cells in T1D autoimmune destroy pancreatic β cells. In contrast, NF-κB and other redox-dependent transcription factors activate DCs and macrophages by generating intracellular ROS as a result of interactions between receptors and ligands (Figure 1), or ROS from neighboring pancreatic phagocytes. The pancreatic lymph nodes receive β-cell antigens transported by activated DCs and macrophages, which enhance ROS production, proinflammatory cytokines, and synapses with reactive T cells (Sun et al., 2023). Innate and adaptive immune responses rely solely on ROS generation for their development and are the primary culprits in islet cell autoimmunity (Delmastro and Piganelli, 2011; Waldron-Lynch and Herold, 2011). There are several uses for transient receptor potential (TRP) channels in normal and disease conditions. Redox TRPs, a subset of these channels, are recently recognized as important in identifying any changes in cellular redox homeostasis to respond quickly. Hyperglycemia is a major cause of diabetes and its associated pathologies. Variations in the oxidative state of cells can affect the activity of many redox TRP channels (TRPA1, TRPC5, TRPMs, and TRPV1). By focusing on these redox TRPs, a new therapeutic approach to treat diabetes is possible. Natural drugs that modulated redox TRP activation may serve as novel therapeutic targets for the efficient treatment of hyperglycemia-induced alterations in cellular redox states, diabetes, and associated diseases (Adhya and Sharma, 2019). When ROS activate stress-sensitive serine/threonine kinases, i.e., c-Jun N-terminal kinase (JNK) and other kinases, the insulin receptor substrate (IRS) protein is phosphorylated at serine residues. Tissues are normally sensitive to insulin, and phosphorylation impairs insulin signaling to induce insulin resistance (Han, 2016). A high-fat diet (HFD) has also been shown to increase the formation of ROS in mitochondria, which in vivo causes insulin resistance. Many insulin resistance models, including those brought on by glucocorticoids and inflammation, solely rely on ROS (Geer et al., 2014).
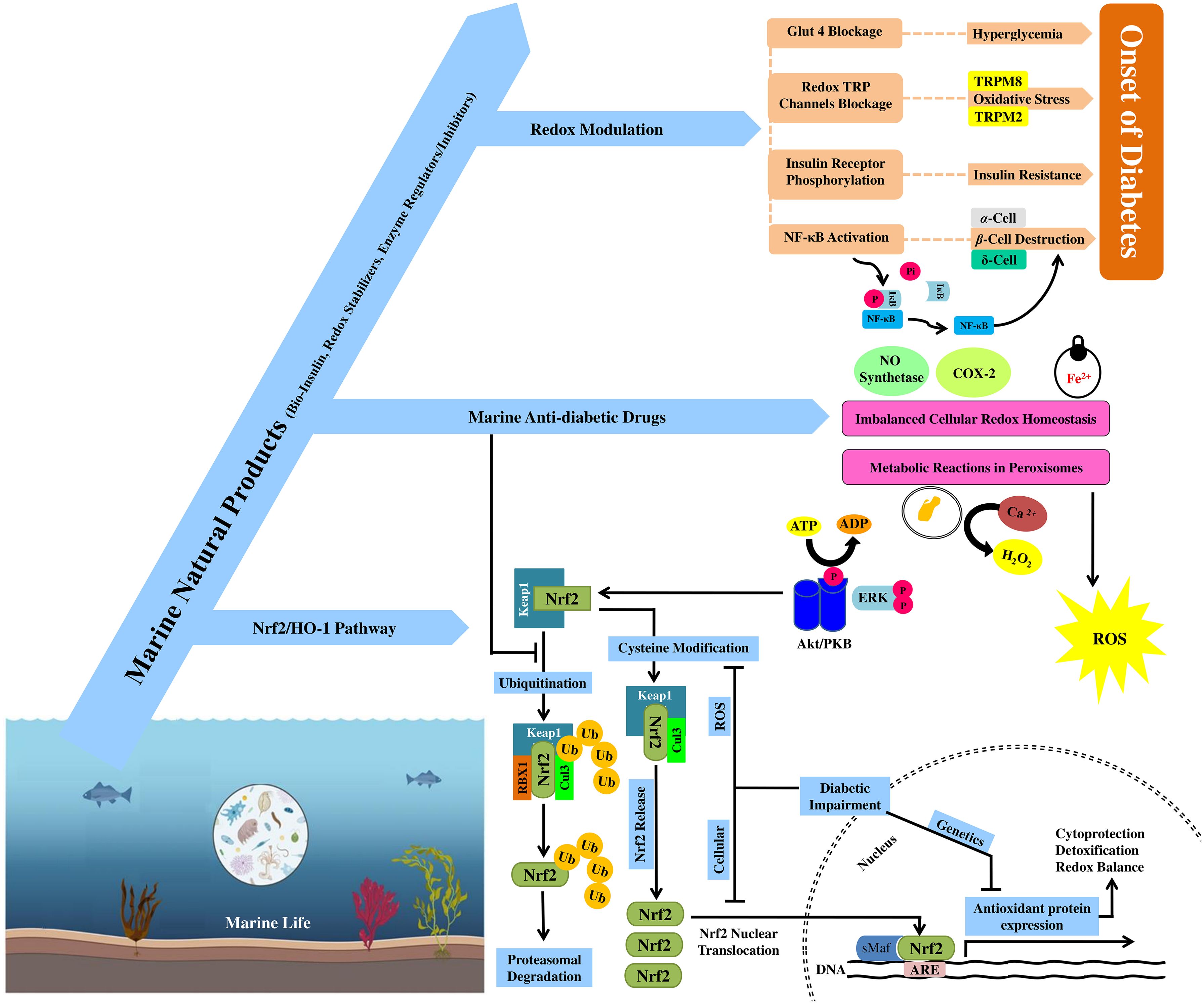
Figure 1. Anti-diabetic effects of marine natural products through redox modulation and activation of the Nrf2/HO-1 cytoprotective pathway. Interplay between marine natural products, redox modulation, and the Nrf2/HO-1 pathway to pose anti-diabetic effects. The mechanism of ubiquitination of Nrf2 and its nuclear movement after releasing from its inhibitor Keap1 or (INrf2) in the cytoplasm. Role of redox modulation in diabetes through multiple signaling cascades to overcome the onset of diabetes (GLUT-4 and redox TRP channel blockage, phosphorylation of IκB and insulin receptors).
3 Nrf2/HO-1 redox responsive cytoprotective pathways in diabetes
An important transcription factor controlling the expression of antioxidant proteins and phase II detoxifying enzymes is Nrf2 (Figure 1) (He et al., 2020). Normally, Nrf2 is inhibited by the cytosolic repressor protein Kelch-like ECH-associated protein 1 (Keap1) and causes its ubiquitination and proteasomal destruction (Cuadrado et al., 2019). Nrf2 is activated and released from the Keap1–Nrf2 complex upon oxidative stress-mediated damage to Keap1 cysteine residues (Cuadrado et al., 2019). After translocation into the nucleus and being firmly attached onto antioxidant response elements (AREs), Nrf2 activates target gene transcription (Baird and Yamamoto, 2020). Additionally, upon transgenic Nrf2 deletion, mice showed reduced levels of antioxidant-encoding genes at both basal and inducible levels, making them very susceptible to develop oxidative stress, diabetes, inflammation, and nephropathy. Activation of Nrf2 is a promising target that could reduce the severity of diabetic kidney injury caused by chronic oxidative stress (Tanase et al., 2022).
Cellular ROS burst dissociates Nrf2 from its inhibitor Keap1 (INrf2)/Cul3–Rbx1 complex and translocates into the nucleus (Kaspar and Jaiswal, 2010; Iso et al., 2016; Baird and Yamamoto, 2020), where it attaches to ARE and activates the expression of downstream antioxidant hemeoxygenase-1 (HO-1) (Figure 1) (Loboda et al., 2016; Hammad et al., 2023). HO-1 is essential in the early stages of homeostatic adaptive responses because it removes excess heme by decomposing into biliverdin, which quickly reduced to bilirubin, iron ions, and carbon monoxide (CO) that reduces oxidative stress-induced tissue damage, inflammation, and apoptosis (Figure 1) (Loboda et al., 2016; Mancuso, 2023). Nrf2 and HO-1 both provide essential cytoprotective responses against any slight change in cellular redox homeostasis (Figure 1). The development of diabetes is predominantly linked to the downregulation of the Nrf2/HO-1 pathway, and their absence contributes to increased oxidative stress, inflammation, and immunological dysregulation (Loboda et al., 2016; Mancuso, 2023).
4 Marine natural products with anti-diabetic effects
Two primary treatments for diabetes are insulin injection and oral anti-diabetic medications, but long-term medication with synthetic drugs leads to slower recovery and a plethora of side effects. Marine natural drugs seem a potential source of anti-diabetic medication due to their strong antioxidant nature and bioactivity (Figure 1) (Unnikrishnan and Jayasri, 2018). The medicinal and pharmacological potential of biologically active compounds from marine plants or non-plant sources has not been focused on until early this century (De la Calle, 2009; Karthikeyan et al., 2022). Pathogenicity in T2D and its associated disorders can be caused by dysregulation in enzyme activities such as α-glucosidase, protein tyrosine phosphatase (PTP), and AR, which can be regulated by marine natural products (Hansen, 2002; Magwaza and Islam, 2023; Banday et al., 2024).
The biologically active insulin, methyl-ethyl ketone derivatives, fucoxanthin, dysidine, 2-piperidione, benzene acetamide, n-hexadecenoic acid, and many other related compounds from brown or red algae, diatoms, ascidians, and corals from the ocean could be novel anti-diabetic drugs that may target enzyme modulation and higher antioxidant activity (Debbab et al., 2010; Bhattacharjee et al., 2014). Activation of the GLUT-4 receptor, which improves peripheral glucose utilization, is another possible anti-diabetic mechanism (Dugani et al., 2008). Fucoxanthin mediates insulin resistance by lowering the expression of adipokines, TNF-α, IL-6, and MCP-1 (Maeda et al., 2007; Maeda et al., 2008). Human insulin and biologically active insulin derived from hammerhead sharks and spotted dogfish are comparable and showed equivalent receptor binding capabilities (Anderson et al., 2002).
A range of bioactive substances such as dietary fibers, omega-3 fatty acids, pigments, sulfated polysaccharides, phlorotannins, polyphenols, and essential amino acids are found in brown algae, all of which pose strong anti-diabetic effects. PTP, α-glucosidase, and α-amylase are among the enzymes that are specifically inhibited by phenols, while GLUT-4 and AMPK signaling cascades are activated to enhance peripheral glucose absorption. Phlorotannin-rich extracts of seaweeds are a strong inhibitor of α-glucosidase and α-amylase (Lee et al., 2009; Lee and Jeon, 2013). Marine algal compounds control blood glucose levels by blocking enzymes that hydrolyze proteins and carbohydrates, enhancing insulin sensitivity, increasing glucose absorption, and averting problems associated with diabetes (Unnikrishnan and Jayasri, 2018).
Numerous aquatic creatures including bacteria, micro- and macroalgae, sea grasses, sponges, corals, sea anemones, fish, salmon skin, a shark fusion single-domain protein, and by-products from fish and shellfish have been investigated for their possible anti-diabetic properties. The anti-hyperglycemic and anti-diabetic characteristics of marine creatures have been studied using both in vitro and in vivo studies (Lauritano and Ianora, 2016; Rayapu et al., 2021). Out of 500 cyanobacteria from freshwater and marine environments, only 38 species showed putative α-glucosidase and α-amylase modulating activity (Cannell et al., 1987). Investigation of drug molecules that block β-glucosidase in bacteria is ongoing due to the enzyme function in the breakdown of polysaccharides and the digestion of glycoproteins and glycolipids, which make it a viable target for the treatment of diabetes and obesity. Many glucosidase inhibitors have been discovered from microbes connected to marine sponges (Aka coralliphaga) (Pandey et al., 2013). Actinomycete Streptomyces sp. produces bioactive pyrostatins A and B that inhibit and regulate the expression levels of N-acetyl-glycosaminidase. At 100 m ocean depth, another strain of Streptomyces corchorusii subsp. Rhodomarinus isolated from Otsuchi Bay showed notable α-amylase inhibition (Imada, 2005).
Different marine fungi have also been reported for their anti-diabetic, anti-inflammatory, and anti-cancer effects (Debbab et al., 2010; Shams Ul Hassan et al., 2021). Aquastatin A showed a strong inhibitory impact on the PTP1B enzyme in a study on the culture broth of the marine fungus Cosmospora sp. SF-5060, which was isolated from intertidal silt at Gejae Island (Korea) (Debbab et al., 2010).
5 Interplay between marine natural products, redox modulation, and the Nrf2/HO-1 pathway in diabetes
Oxidative stress prevents Nrf2 from being ubiquitinated and degraded under basal levels, which phosphorylates and translocates it into the nucleus to attach to ARE at promoter regions of target genes (HO-1) for their transcriptional activation (Bryan et al., 2013; Dinkova-Kostova and Abramov, 2015). Upon its expression, HO-1 catabolizes heme into bilirubin, Fe2+, and CO. Bilirubin exceptionally scavenges intracellular ROS burst to lower down oxidative stress (Aayadi et al., 2017). Various marine natural products provide protection by activating protein kinase B (PKB, Akt) and extracellular signal-regulated kinase (ERK). Under oxidative stress, ERK and the PKB/Akt pathway also facilitate Nrf2 nuclear translocation and hence improve the transcriptional control of the HO-1 gene to protect cells against oxidative damage (Kim and Jang, 2014; Han et al., 2017). Marine drug molecules also protect cells from ultraviolet B (UVB) and H2O2-induced oxidative damage, but they also raise the levels of HO-1 and Nrf2 by phosphorylation of ERK and activation of Akt. The prevention and treatment of oxidative damage and related disorders are involved with the proper modulation and activation of the Nrf2-Keap1 signaling pathway to mitigate the cell/tissue damage (Kang et al., 2004).
The natural pigment echinochrome A from sea urchins has therapeutic properties due to its capacity to efficiently scavenge water-soluble radical ions especially O2•−. Echinochrome A exhibits exceptional ability to scavenge peroxy radicals and bind Fe2+ ions. Free radical chelating activity of hydroxyl groups on echinochrome A neutralize ROS in order to maintain cellular redox homeostasis (Lebedev et al., 2008; Popov and Krivoshapko, 2013). H2O2 is produced when this polyhydroxynaphthoquinone is exposed to ROS in the presence of Ca2+ ions. One hypothesis states that physiological H2O2 levels could function as a second messenger in intracellular and intercellular signal transduction. The optimum quantity of echinochrome A neutralized O2•− to less toxic H2O2, in order to control gene expression and production of stress- and antioxidant-related enzymes (Lebedev et al., 2005; Popov and Krivoshapko, 2013).
Marine substances are also known to impede the enzymes producing eicosanoids, which lowers the production of pro-inflammatory molecules such as prostaglandins and leukotrienes. These substances might prevent the activation of transcription factors controlling the expression of genes linked to inflammation and oxidation such as cyclooxygenase-2 (COX-2), inducible NO synthase, TNF-α, IL-1β, and IL-6 (Kim et al., 2004; Popov and Krivoshapko, 2013).
The redox regulation potential of marine natural compounds via the Nrf2/HO-1 cytoprotective pathways is highlighted by their anti-diabetic actions primarily due to their antioxidant potential to diminish oxidative damage to β cells. Through the specialized targeting of protective pathways and maintenance of cellular redox balance, these mechanisms highlight the therapeutic potential of compounds originating from marine environments in the treatment of diabetes (Dong et al., 2021).
6 Preclinical and clinical studies
The anti-diabetic effects of the various compounds reported in preclinical and clinical studies have extensively been reviewed herein (Table 1).
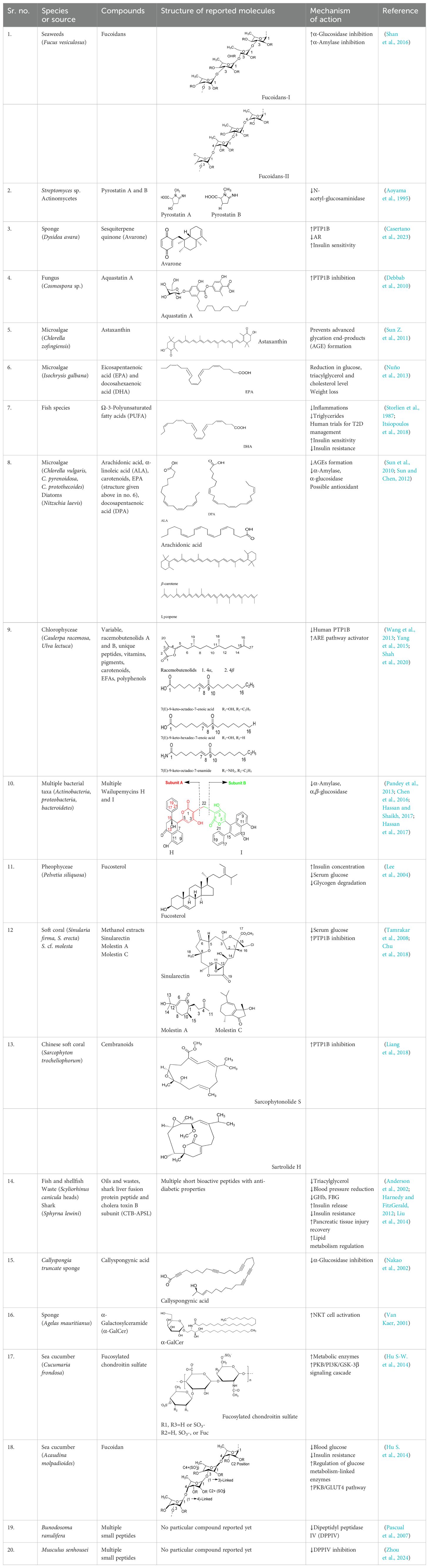
Table 1. Screening of bioactive chemicals in microorganisms and investigating their mechanism of action concerning their potential anti-diabetic effects.
7 Comparative analysis
Various differentiating factors exist between T1D and T2D. T1D, also known as insulin-dependent diabetes mellitus (IDDM), is characterized by insufficient pancreatic insulin production. In order to cure T1D, insulin must be imported and administered intravenously to those with T1D in order to control blood sugar levels (Forouhi and Wareham, 2019). On the other hand, high blood glucose levels are a hallmark of T2D, commonly referred to as non-insulin-dependent diabetes mellitus (NIDDM), with insufficient insulin secretion. Oral anti-diabetic drugs that lower blood glucose levels such as biguanides and sulfonylureas are treatment options for T2D. Chemical medications that work by blocking digestive enzymes and are available to treat T2D include acarbose, pioglitazone, and miglitol. Diabetes is a major public health concern that can lead to retinopathy, nephropathy, and neuropathy (Jain and Saraf, 2010). Furthermore, most of the synthetic or modified drugs might have negative side effects that get worse if a patient used them for a long time (Groop and Pociot, 2014).
Diabetes treatments are costly, and scientists continue to look for better, less expensive alternatives with no side effects. Alternative natural medicines are being considered by the majority of people in developing nations (Hassan et al., 2010). Natural resources provide the basis of many clinical treatments; furthermore, the majority of contemporary pharmaceutics are produced from compounds derived from marine natural resources (Mayer et al., 2010). These marine natural resources have yielded a wide variety of potent drug molecules, several of which are in clinical trials. Recently, certain novel biologically active molecules have been isolated for pharmaceuticals and nutritional supplements (Mayer et al., 2010; Sun H-H. et al., 2011; Beygmoradi and Homaei, 2017).
Diabetes has a complex array of symptoms that make management and treatment more difficult (Borse et al., 2021). The abundance of bioactive molecules from marine resources offers a viable way to tackle this complexity (Lauritano and Ianora, 2016; Cotas et al., 2024). Fish, invertebrates, marine grasses, algae, sponges, mushrooms, bacteria, and microalgae can be further explored as novel diabetic medicines (Anjum et al., 2016). Dietary fiber and complex polysaccharides in seaweed help lower blood sugar and enhance insulin sensitivity (Cotas et al., 2024). The high fatty acid content in seaweeds minimizes inflammation linked to obesity and high blood sugar (Popov and Krivoshapko, 2013). In order to treat hyperglycemia and hyperlipidemia, seaweed-derived phenolic compounds have the capacity to inhibit PPD-4 and subsequently suppress α-glucosidase and α-amylase activity, initiate signaling via AMPK and Akt, stop AGE production, and improve insulin sensitivity (Unnikrishnan and Jayasri, 2018). Moreover, marine bioactive chemicals can also manage obesity; protect pancreatic β cells; inhibit AR, PTP1B, and PPD-4; and enhance glucose uptake. There is encouraging prospect for the management of diabetes and its aftereffects through the oral and pharmaceutical supplementation of biological components derived from marine resources (Cotas et al., 2024).
8 Conclusion
A unique strategy for the treatment of diabetes has been revealed by the investigation of the anti-diabetic effects of marine natural products. Aquatic species that have been studied for their possible anti-diabetic properties include bacteria, microalgae, macroalgae, seagrass, sponges, corals, sea anemones, fish, salmon skin, a shark fusion protein, shellfish, and fish wastes. The study focused on the redox regulation potential of marine natural products via the Nrf2/HO-1 cytoprotective pathways. The relationship between oxidative stress, inflammation, and cellular damage in diabetes emphasized focusing on the Nrf2/HO-1 pathway as a novel methodology by these marine bioactives. Marine bioactive compounds have significant antioxidant, anti-inflammatory, and cytoprotective capabilities due to their complex physical structures and functional groups. This review demonstrated that micro- and macroorganisms found in the ocean contain biologically active drug molecules as potential anti-diabetic agents.
Author contributions
MN: Conceptualization, Writing – original draft, Writing – review & editing. ML: Visualization, Writing – review & editing. JX: Writing – original draft, Writing – review & editing. CW: Conceptualization, Visualization, Writing – original draft, Writing – review & editing.
Funding
The author(s) declare that no financial support was received for the research, authorship, and/or publication of this article.
Acknowledgments
I acknowledge Dr. Muhammad Rafiq, Assistant Professor, Department of Physiology and Biochemistry, Cholistan University of Veterinary and Animal Sciences (CUVAS), Bahawalpur, for giving useful suggestions that helped to improve the draft.
Conflict of interest
The authors declare that the research was conducted in the absence of any commercial or financial relationships that could be construed as a potential conflict of interest.
Publisher’s note
All claims expressed in this article are solely those of the authors and do not necessarily represent those of their affiliated organizations, or those of the publisher, the editors and the reviewers. Any product that may be evaluated in this article, or claim that may be made by its manufacturer, is not guaranteed or endorsed by the publisher.
Abbreviations
ARE, Antioxidant response element; CAM, Complementary and alternative medicine; CVD, Cardiovascular disease; DCs, Dendritic cells; DM, Diabetes mellitus; DPP4, Dipeptidyl-peptidase 4; ERK, Signal-regulated kinase; FBG, Fasting blood glucose; GHb, Glycosylated hemoglobin; GLUT4, Glucose transporter protein type 4; HFD, High-fat diet; HO-1, Heme oxygenase-1; IDDM, Insulin-dependent diabetes mellitus; IGG, Impaired fasting glucose; IL-6, Interleukin-6; IκB, Inhibitor of kappa B; iNKT, Invariant natural killer T; IRS, Insulin receptor substrate; JNK, Jun N-terminal kinase; MCP-1, Monocyte chemoattractant protein-1; NCCAM, National Center for Complementary and Alternative Medicine; NCDs, Noncommunicable diseases; PKB, Protein kinase B; PTP1B, Protein tyrosine phosphatase 1B; ROS, Reactive oxygen species; T1D, Type 1 diabetes; TNF-α, Tumor necrosis factor-α; TRP, Transient receptor potential.
References
Aayadi H., Mittal S. P., Deshpande A., Gore M., Ghaskadbi S. S. (2017). Cytoprotective effect exerted by geraniin in HepG2 cells is through microRNA mediated regulation of BACH-1 and HO-1. BMB. Rep. 50, 560. doi: 10.5483/BMBRep.2017.50.11.060
Adhya P., Sharma S. S. (2019). Redox TRPs in diabetes and diabetic complications: Mechanisms and pharmacological modulation. Pharmacol. Res. 146, 104271. doi: 10.1016/j.phrs.2019.104271
Ahmadizar F., Wang K., Aribas E., Fani L., Heshmatollah A., Ikram M. K., et al. (2021). Impaired fasting glucose, type 2 diabetes mellitus, and lifetime risk of cardiovascular disease among women and men: the Rotterdam Study. BMJ Open Diabet. Res. Care 9, e002406. doi: 10.1136/bmjdrc-2021-002406
Anderson W. G., Ali M., Einarsdóttir I. E., Schäffer L, Hazon N, Conlon JM, et al. (2002). Purification, characterization, and biological activity of insulins from the spotted dogfish, Scyliorhinus canicula, and the hammerhead shark, Sphyrna lewini. Gen. Comp. Endocrinol. 126, 113–122. doi: 10.1006/gcen.2002.7787
Anjum K., Abbas S. Q., Shah S. A., Akhter N., Batool S., Hassan S. S., et al. (2016). Marine sponges as a drug treasure. Biomol. Ther. (Seoul). 24, 347–362. doi: 10.4062/biomolther.2016.067
Aoyama T., Kojima F., Imada C., Muraoka Y., Naganawa H., Okami Y., et al. (1995). Pyrostatins A and B, new inhibitors of N-acetyl-beta-D-glucosaminidase, produced by Streptomyces sp. SA-3501. J. Enzyme Inhibition. 8, 223–232. doi: 10.3109/14756369509020129
Baird L., Yamamoto M. (2020). The molecular mechanisms regulating the KEAP1-NRF2 pathway. Mol. Cell Biol. 40, e00099-20. doi: 10.1128/MCB.00099-20
Banday A. H., Azha Nu Farooq R., Sheikh S. A., Ganie M. A., Parray M. N., et al. (2024). Exploring the potential of marine natural products in drug development: A comprehensive review. Phytochem. Lett. 59, 124–135. doi: 10.1016/j.phytol.2024.01.001
Beaglehole R., Bonita R., Alleyne G., Horton R., Li L., Lincoln P., et al. (2011). UN high-level meeting on non-communicable diseases: addressing four questions. Lancet 378, 449–455. doi: 10.1016/S0140-6736(11)60879-9
Beygmoradi A., Homaei A. (2017). Marine microbes as a valuable resource for brand new industrial biocatalysts. Biocatalysis. Agric. Biotechnol. 11, 131–152. doi: 10.1016/j.bcab.2017.06.013
Bhattacharjee R., Mitra A., Dey B., Pal A. (2014). Exploration of anti-diabetic potentials amongst marine species-a mini review. Indo. Global J. Pharma. Sci. 4, 65–73. doi: 10.35652/IGJPS.2014.109
Borse S. P., Chhipa A., Sharma V., Singh D. P., Nivsarkar M. (2021). Management of type 2 diabetes: current strategies, unfocussed aspects, challenges, and alternatives. Med. Princ. Pract. 30, 109–121. doi: 10.1159/000511002
Bryan H. K., Olayanju A., Goldring C. E., Park B. K. (2013). The Nrf2 cell defence pathway: Keap1-dependent and-independent mechanisms of regulation. Biochem. Pharmacol. 85, 705–717. doi: 10.1016/j.bcp.2012.11.016
Cannell R. J., Kellam S. J., Owsianka A. M., Walker J. M. (1987). Microalgae and cyanobacteria as a source of glycosidase inhibitors. J. Gen. Microbiol. 133, 1701–1705. doi: 10.1099/00221287-133-7-1701
Casertano M., Genovese M., Santi A., Pranzini E., Balestri F., Piazza L., et al. (2023). Evidence of insulin-sensitizing and mimetic activity of the sesquiterpene quinone avarone, a protein tyrosine phosphatase 1B and aldose reductase dual targeting agent from the marine sponge dysidea avara. Pharmaceutics 15, 528. doi: 10.3390/pharmaceutics15020528
Caturano A., D’Angelo M., Mormone A., Russo V., Mollica M. P., Salvatore T., et al. (2023). Oxidative stress in type 2 diabetes: impacts from pathogenesis to lifestyle modifications. Curr. Iss. Mol. Bio. 45, 6651–6666. doi: 10.3390/cimb45080420
Chen Z., Hao J., Wang L. P., et al. (2016). New α-glucosidase inhibitors from marine algae-derived Streptomyces sp. OUCMDZ-3434. Sci. Rep. 6, 20004. doi: 10.1038/srep20004
Choi J., Lee H., Kuang A., Huerta-Chagoya A., Scholtens D. M., Choi D., et al. (2024). Genome-wide polygenic risk score predicts incident type 2 diabetes in women with history of gestational diabetes. Diabet. Care. 47, 1622-1629. doi: 10.2337/dc24-0022
Chu M. J., Tang X., Han X., Li T., Luo X. C., Jiang M. M., et al. (2018). Metabolites from the Paracel Islands Soft Coral Sinularia cf. molesta. Mar. Drugs 16, 517. doi: 10.3390/md16120517
Cotas J., Lomartire S., Pereira L., Valado A., Marques J. C., Gonçalves A. M. M., et al. (2024). Seaweeds as nutraceutical elements and drugs for diabetes mellitus: future perspectives. Mar. Drugs 22, 168. doi: 10.3390/md22040168
Cuadrado A., Rojo A. I., Wells G., Hayes J. D., Cousin S. P., Rumsey W. L., et al. (2019). Therapeutic targeting of the NRF2 and KEAP1 partnership in chronic diseases. Nat. Rev. Drug Disc. 18, 295–317. doi: 10.1038/s41573-018-0008-x
Debbab A., Aly A. H., Lin W. H., Proksch P. (2010). Bioactive compounds from marine bacteria and fungi. Microbial. Biotechnol. 3, 544–563. doi: 10.1111/j.1751-7915.2010.00179.x
De la Calle F. (2009). Marine genetic resources. A source of new drugs the experience of the biotechnology sector. Int. J. Mar. Coast. Law 24, 209–220. doi: 10.1163/157180809X421743
Delmastro M. M., Piganelli J. D. (2011). Oxidative stress and redox modulation potential in type 1 diabetes. Clinic. Dev. Immunol. 2011, 1–15. doi: 10.1155/2011/593863
Dinkova-Kostova A. T., Abramov A. Y. (2015). The emerging role of Nrf2 in mitochondrial function. Free Radical Biol. Med. 88, 179–188. doi: 10.1016/j.freeradbiomed.2015.04.036
Dong H., Liu M., Wang L., Liu Y., Lu X., Stagos D., et al. (2021). Bromophenol bis (2, 3, 6-tribromo-4, 5-dihydroxybenzyl) ether protects HaCaT skin cells from oxidative damage via Nrf2-mediated pathways. Antioxidants 10, 1436. doi: 10.3390/antiox10091436
Dugani C. B., Randhawa V. K., Cheng A. W., Patel N., Klip A. (2008). Selective regulation of the perinuclear distribution of glucose transporter 4 (GLUT4) by insulin signals in muscle cells. Eur. J. Cell Biol. 87, 337–351. doi: 10.1016/j.ejcb.2008.02.009
Eizirik D. L., Pasquali L., Cnop M. (2020). Pancreatic β-cells in type 1 and type 2 diabetes mellitus: different pathways to failure. Nat. Rev. Endocrinol. 16, 349–362. doi: 10.1038/s41574-020-0355-7
Elliott A., Walters R. K., Pirinen M., Kurki M., Junna N., Goldstein J. I., et al. (2024). Distinct and shared genetic architectures of gestational diabetes mellitus and type 2 diabetes. Nat. Genet. 56, 377–382. doi: 10.1038/s41588-023-01607-4
ElSayed N. A., Aleppo G., Aroda V. R., Bannuru R. R., Brown F. M., Bruemmer D., et al. (2022). Pharmacologic approaches to glycemic treatment: standards of care in diabetes—2023. Diabet. Care 46, S140–S157. doi: 10.2337/dc23-S009
Forouhi N. G., Wareham N. J. (2019). Epidemiology of diabetes. Medicine 47, 22–27. doi: 10.1016/j.mpmed.2018.10.004
Gagare S., Patil P., Jain A. (2024). Natural product-inspired strategies towards the discovery of novel bioactive molecules. Future J. Pharmac. Sci. 10, 55. doi: 10.1186/s43094-024-00627-z
Galicia-Garcia U., Benito-Vicente A., Jebari S., Larrea-Sebal A., Siddiqi H., Uribe K. B., et al. (2020). Pathophysiology of type 2 diabetes mellitus. Int. J. Mol. Sci. 21, 6275. doi: 10.3390/ijms21176275
Geer E. B., Islam J., Buettner C. (2014). Mechanisms of glucocorticoid-induced insulin resistance: focus on adipose tissue function and lipid metabolism. Endocrinol. Metab. Clinics 43, 75–102. doi: 10.1016/j.ecl.2013.10.005
Groop L., Pociot F. (2014). Genetics of diabetes–are we missing the genes or the disease? Mol. Cell. Endocrinol. 382, 726–739. doi: 10.1016/j.mce.2013.04.002
Hammad M., Raftari M., Cesário R., Salma R., Godoy P., Emami S. N., et al. (2023). Roles of oxidative stress and nrf2 signaling in pathogenic and non-pathogenic cells: A possible general mechanism of resistance to therapy. Antioxidants 12, 1371. doi: 10.3390/antiox12071371
Han C. Y. (2016). Roles of reactive oxygen species on insulin resistance in adipose tissue. Diabetes Metab. J. 40, 272–279. doi: 10.4093/dmj.2016.40.4.272
Han D., Chen W., Gu X., Shan R., Zou J., Liu G., et al. (2017). Cytoprotective effect of chlorogenic acid against hydrogen peroxide-induced oxidative stress in MC3T3-E1 cells through PI3K/Akt-mediated Nrf2/HO-1 signaling pathway. Oncotarget 8, 14680. doi: 10.18632/oncotarget.14747
Hansen T. (2002). Genetics of type 2 diabetes. Curr. Sci. p, 1477–1482. Available at: http://www.jstor.org/stable/24108171.
Harnedy P. A., FitzGerald R. J. (2012). Bioactive peptides from marine processing waste and shellfish: A review. J. Funct. Foods. 4, 6–24. doi: 10.1016/j.jff.2011.09.001
Hassan Z., Yam M. F., Ahmad M., Yusof A. P. M. (2010). Antidiabetic properties and mechanism of action of Gynura procumbens water extract in streptozotocin-induced diabetic rats. Molecules 15, 9008–9023. doi: 10.3390/molecules15129008
Hassan S. S., Anjum K., Abbas S. Q., Akhter N., Shagufta B. I., Shah S. A. A., et al. (2017). Emerging biopharmaceuticals from marine actinobacteria. Environ. Toxicol. Pharmacol. 49, 34–47. doi: 10.1016/j.etap.2016.11.015
Hassan S. S., Abdel-Daim M. M., Behl T., Bungau S. (2022). Natural products for chronic diseases: A ray of hope. Molecules 27, 5573. doi: 10.3390/molecules27175573
Hassan S., Shaikh A. L. (2017). Marine actinobacteria as a drug treasure house. Biomed. Pharmaco 87, 46–57. doi: 10.1016/j.biopha.2016.12.086
He F., Ru X., Wen T. (2020). NRF2, a transcription factor for stress response and beyond. Int. J. Mol. Sci. 21, 4777. doi: 10.3390/ijms21134777
Hu S.-W., Tian Y.-Y., Chang Y.-G., Li Z.-J., Xue C.-H., Wang Y.-M. (2014). Fucosylated chondroitin sulfate from sea cucumber improves glucose metabolism and activates insulin signaling in the liver of insulin-resistant mice. J. Med. Food 17, 749–757. doi: 10.1089/jmf.2013.2924
Hu S., Xia G., Wang J., Wang Y., Li Z., Xue C. (2014). Fucoidan from sea cucumber protects against high-fat high-sucrose diet-induced hyperglycaemia and insulin resistance in mice. J. Funct. Foods. 10, 128–138. doi: 10.1016/j.jff.2014.05.012
Imada C. (2005). Enzyme inhibitors and other bioactive compounds from marine actinomycetes. Antonie. Van. Leeuwenhoek. 87, 59–63. doi: 10.1007/s10482-004-6544-x
Iso T., Suzuki T., Baird L., Yamamoto M. (2016). Absolute amounts and status of the nrf2-keap1-cul3 complex within cells. Mol. Cell. Biol. 36, 3100–3112. doi: 10.1128/MCB.00389-16
Itsiopoulos C., Marx W., Mayr H., Tatucu-Babet O., Dash S., George E., et al. (2018). The role of omega-3 polyunsaturated fatty acid supplementation in the management of type 2 diabetes mellitus: A narrative review. J. Nutr. Intermediary. Metab. 14, 42–51. doi: 10.1016/j.jnim.2018.02.002
Jain S., Saraf S. (2010). Type 2 diabetes mellitus—Its global prevalence and therapeutic strategies. Diabetes Metab. Syndrome.: Clin. Res. Rev. 4, 48–56. doi: 10.1016/j.dsx.2008.04.011
Kang M.-I., Kobayashi A., Wakabayashi N., Kim S.-G., Yamamoto M. 2004). Scaffolding of Keap1 to the actin cytoskeleton controls the function of Nrf2 as key regulator of cytoprotective phase 2 genes. Proc. Natl. Acad. Sci. 101, 2046–2051. doi: 10.1073/pnas.0308347100
Karthikeyan A., Joseph A., Nair B. G. (2022). Promising bioactive compounds from the marine environment and their potential effects on various diseases. J. Genet. Eng. Biotechnol. 20, 14. doi: 10.1186/s43141-021-00290-4
Kaspar J. W., Jaiswal A. K. (2010). An autoregulatory loop between nrf2 and cul3-rbx1 controls their cellular abundance*. J. Biol. Chem. 285, 21349–21358. doi: 10.1074/jbc.M110.121863
Khan N. U., Qazi N. G., Khan A-u., Ali F., Hassan S. S., Bungau S. (2022). Anti-diabetic activity of brucine in streptozotocin-induced rats: in silico, in vitro, and in vivo studies. ACS Omega. 7, 46358–46370. doi: 10.1021/acsomega.2c04977
Kim H. P., Son K. H., Chang H. W., Kang S. S. (2004). Anti-inflammatory plant flavonoids and cellular action mechanisms. J. Pharmacol. Sci. 96, 229–245. doi: 10.1254/jphs.CRJ04003X
Kim J.-K., Jang H.-D. (2014). Nrf2-mediated HO-1 induction coupled with the ERK signaling pathway contributes to indirect antioxidant capacity of caffeic acid phenethyl ester in HepG2 cells. Int. J. Mol. Sci. 15, 12149–12165. doi: 10.3390/ijms150712149
Kumar D., Bajaj S., Mehrotra R. (2006). Knowledge, attitude and practice of complementary and alternative medicines for diabetes. Public Health 120, 705–711. doi: 10.1016/j.puhe.2006.04.010
Lauritano C., Ianora A. (2016). Marine organisms with anti-diabetes properties. Mar. Drugs 14, 220. doi: 10.3390/md14120220
Lebedev A. V., Ivanova M. V., Levitsky D. O. (2005). Echinochrome, a naturally occurring iron chelator and free radical scavenger in artificial and natural membrane systems. Life Sci. 76, 863–875. doi: 10.1016/j.lfs.2004.10.007
Lebedev A. V., Ivanova M. V., Levitsky D. O. (2008). Iron chelators and free radical scavengers in naturally occurring polyhydroxylated 1, 4-naphthoquinones. Hemoglobin 32, 165–179. doi: 10.1080/03630260701700017
Lee Y. S., Shin K. H., Kim B.-K., Lee S. (2004). Anti-diabetic activities of fucosterol from Pelvetia siliquosa. Arch. pharmacal. Res. 27, 1120–1122. doi: 10.1007/BF02975115
Lee S. H., Karadeniz F., Kim M. M., Kim S. K. (2009). [amp]]alpha;-Glucosidase and α-amylase inhibitory activities of phloroglucinal derivatives from edible marine brown alga, Ecklonia cava. J. Sci. Food Agric. 89, 1552–1558. doi: 10.1002/jsfa.v89:9
Lee S.-H., Jeon Y.-J. (2013). Anti-diabetic effects of brown algae derived phlorotannins, marine polyphenols through diverse mechanisms. Fitoterapia 86, 129–136. doi: 10.1016/j.fitote.2013.02.013
Li Y., Liu Y., Liu S., Gao M., Wang W., Chen K., et al. (2023). Diabetic vascular diseases: molecular mechanisms and therapeutic strategies. Sig. Transduc. Targeting Ther. 8, 152. doi: 10.1038/s41392-023-01400-z
Liang L.-F., Kurtán T., Mándi A., Yao L.-G., Li J., Lan L.-F., et al. (2018). Structural, stereochemical, and bioactive studies of cembranoids from Chinese soft coral Sarcophyton trocheliophorum. Tetrahedron 74, 1933–1941. doi: 10.1016/j.tet.2018.02.059
Liu Y., Gao Z., Guo Q., Wang T., Lu C., Chen Y., et al. (2014). Anti-diabetic effects of CTB-APSL fusion protein in type 2 diabetic mice. Mar. Drugs 12, 1512–1529. doi: 10.3390/md12031512
Loboda A., Damulewicz M., Pyza E., Jozkowicz A., Dulak J. (2016). Role of Nrf2/HO-1 system in development, oxidative stress response and diseases: an evolutionarily conserved mechanism. Cell Mol. Life Sci. 73, 3221–3247. doi: 10.1007/s00018-016-2223-0
Maeda H., Hosokawa M., Sashima T., Miyashita K. (2007). Dietary combination of fucoxanthin and fish oil attenuates the weight gain of white adipose tissue and decreases blood glucose in obese/diabetic KK-Ay mice. J. @ Agric. Food Chem. 55, 7701–7706. doi: 10.1021/jf071569n
Maeda H., Tsukui T., Sashima T., Hosokawa M., Miyashita K. (2008). Seaweed carotenoid, fucoxanthin, as a multi-functional nutrient. Asia. Pac. J. Clinic. Nutr. 17, 196–209.
Maestas M. M., Ishahak M., Augsornworawat P., Veronese-Paniagua D. A., Maxwell K. G., Velazco-Cruz L., et al. (2024). Identification of unique cell type responses in pancreatic islets to stress. Nat. Commun. 15, 5567. doi: 10.1038/s41467-024-49724-w
Magwaza S., Islam M. S. (2023). Roles of marine macroalgae or seaweeds and their bioactive compounds in combating overweight, obesity and diabetes: A comprehensive review. Mar. Drugs 21, 258. doi: 10.3390/md21040258
Mancuso C. (2023). Biliverdin as a disease-modifying agent: An integrated viewpoint. Free Rad. Bio. Med. 207, 133–143. doi: 10.1016/j.freeradbiomed.2023.07.015
Mayer A. M., Glaser K. B., Cuevas C., Jacobs R. S., Kem W., Little R. D., et al. (2010). The odyssey of marine pharmaceuticals: a current pipeline perspective. Trends Pharmacol. Sci. 31, 255–265. doi: 10.1016/j.tips.2010.02.005
Nakao Y., Uehara T., Matunaga S., Fusetani N., Van Soest R. W. (2002). Callyspongynic Acid, a Polyacetylenic Acid Which Inhibits α-Glucosidase, from the Marine Sponge Callyspongia t runcata. J. Natural Products. 65, 922–924. doi: 10.1021/np0106642
Nasab S. B., Homaei A., Pletschke B. I., Salinas-Salazar C., Castillo-Zacarias C., Parra-Saldívar R. (2020). Marine resources effective in controlling and treating diabetes and its associated complications. Process. Biochem. 92, 313–342. doi: 10.1016/j.procbio.2020.01.024
Nowell J., Blunt E., Gupta D., Edison P. (2023). Antidiabetic agents as a novel treatment for Alzheimer’s and Parkinson’s disease. Ageing Res. Rev. 89, 101979. doi: 10.1016/j.arr.2023.101979
Nuño K., Villarruel-López A., Puebla-Pérez A., Romero-Velarde E., Puebla-Mora A., Ascencio F. (2013). Effects of the marine microalgae Isochrysis galbana and Nannochloropsis oculata in diabetic rats. J. Funct. Foods. 5, 106–115. doi: 10.1016/j.jff.2012.08.011
Olaokun O. O., McGaw L. J., Eloff J. N., Naidoo V. (2013). Evaluation of the inhibition of carbohydrate hydrolysing enzymes, antioxidant activity and polyphenolic content of extracts of ten African Ficus species (Moraceae) used traditionally to treat diabetes. BMC Complementary. Altern. Med. 13, 1–10. doi: 10.1186/1472-6882-13-94
Pandey S., Sree A., Dash S. S., Sethi D. P., Chowdhury L. (2013). Diversity of marine bacteria producing beta-glucosidase inhibitors. Microbial. Cell Factories. 12, 1–7. doi: 10.1186/1475-2859-12-35
Pascual I., Lopéz A., Gómez H., Chappé M., Saroyán A., González Y., et al. (2007). Screening of inhibitors of porcine dipeptidyl peptidase IV activity in aqueous extracts from marine organisms. Enzyme Microbial. Technol. 40, 414–419. doi: 10.1016/j.enzmictec.2006.07.012
Pathak K., Gogoi U., Saikia R., Pathak M. P., Das A. (2022). “Chapter 11 - Marine-derived antidiabetic compounds: an insight into their sources, chemistry, SAR, and molecular mechanisms,” in Stud. Nat. Prod. Chem. Ed. Atta ur R. (Cambridge, United States: Elsevier), 467–504.
Popov A. M., Krivoshapko O. N. (2013). Protective effects of polar lipids and redox-active compounds from marine organisms at modeling of hyperlipidemia and diabetes. J. Biomed. Sci. Eng. 6, 543–550. doi: 10.4236/JBISE.2013.65069
Poznyak A. V., Litvinova L., Poggio P., Sukhorukov V. N., Orekhov A. N. (2022). Effect of glucose levels on cardiovascular risk. Cells 11, 3034. doi: 10.3390/cells11193034
Ranasinghe P., Jayawardana R., Galappaththy P., Constantine G., de Vas Gunawardana N., Katulanda P. (2012). Efficacy and safety of ‘true’cinnamon (Cinnamomum zeylanicum) as a pharmaceutical agent in diabetes: a systematic review and meta analysis. Diabet. Med. 29, 1480–1492. doi: 10.1111/j.1464-5491.2012.03718.x
Rayapu L., Chakraborty K., Valluru L. (2021). Marine algae as a potential source for anti-diabetic compounds - A brief review. Curr. Pharm. Des. 27, 789–801. doi: 10.2174/1381612826666200909124526
Roep B. O., Thomaidou S., van Tienhoven R., Zaldumbide A. (2021). Type 1 diabetes mellitus as a disease of the β-cell (do not blame the immune system)? Nat. Rev. Endocrinol. 17, 150–161. doi: 10.1038/s41574-020-00443-4
Roglic G. (2016). WHO Global report on diabetes: A summary. Int. J. Noncommunicable. Dis. 1, 3–8. doi: 10.4103/2468-8827.184853
Shah S. A. A., Hassan S. S., Bungau S., Si Y., Xu H., Rahman M. H., et al. (2020). Chemically diverse and biologically active secondary metabolites from marine phylum chlorophyta. Mar. Drugs 18, 493. doi: 10.3390/md18100493
Shams Ul Hassan S., Ishaq M., Zhang W. D., Jin H. Z. (2021). An overview of the mechanisms of marine fungi-derived anti-inflammatory and anti-tumor agents and their novel role in drug targeting. Curr. Pharm. Des. 27, 2605–2614. doi: 10.2174/1381612826666200728142244
Shan X., Liu X., Hao J., Cai C., Fan F., Dun Y., et al. (2016). In vitro and in vivo hypoglycemic effects of brown algal fucoidans. Int. J. Biol. Macromol. 82, 249–255. doi: 10.1016/j.ijbiomac.2015.11.036
Storlien L. H., Kraegen E. W., Chisholm D. J., Ford G. L., Bruce D. G., Pascoe W. S. (1987). Fish oil prevents insulin resistance induced by high-fat feeding in rats. Science 237, 885–888. doi: 10.1126/science.3303333
Sun Z., Chen F. (2012). Evaluation of the Green Alga Chlorella pyrenoidosa for management of diabetes. J. Food Drug Anal. 20, 28. doi: 10.38212/2224-6614.2094
Sun Z., Peng X., Liu J., Fan K. W., Wang M., Chen F. (2010). Inhibitory effects of microalgal extracts on the formation of advanced glycation endproducts (AGEs). Food Chem. 120, 261–267. doi: 10.1016/j.foodchem.2009.10.018
Sun Z., Liu J., Zeng X., Huangfu J., Jiang Y., Wang M., et al. (2011). Protective actions of microalgae against endogenous and exogenous advanced glycation endproducts (AGEs) in human retinal pigment epithelial cells. Food Funct. 2, 251–258. doi: 10.1039/c1fo10021a
Sun H.-H., Mao W. J., Jiao J. Y., Xu J. C., Li H. Y., Chen Y., et al. (2011). Structural characterization of extracellular polysaccharides produced by the marine fungus Epicoccum nigrum JJY-40 and their antioxidant activities. Mar. Biotechnol. 13, 1048–1055. doi: 10.1007/s10126-011-9368-5
Sun F., Yang C-L., Wang F-X., Rong S-J., Luo J-H., Lu W-Y., et al. (2023). Pancreatic draining lymph nodes (PLNs) serve as a pathogenic hub contributing to the development of type 1 diabetes. Cell Biosc. 13, 156. doi: 10.1186/s13578-023-01110-7
Tamrakar A. K., Tiwari P., Ahmad R., Kumar R., Lakshmi V., Srivastava M. N., et al. (2008). Antihyperglycaemic activity of Sinularia firma and Sinularia erecta in streptozotocin-induced diabetic rats. Med. Chem. Res. 17, 62–73. doi: 10.1007/s00044-007-9037-4
Tanase D. M., Gosav E. M., Anton M. I., Floria M., Seritean Isac P. N., Hurjui L. L., et al. (2022). Oxidative stress and NRF2/KEAP1/ARE pathway in diabetic kidney disease (DKD): new perspectives. Biomolecules 12, 1227. doi: 10.3390/biom12091227
Tangkiatkumjai M., Boardman H., Walker D.-M. (2020). Potential factors that influence usage of complementary and alternative medicine worldwide: a systematic review. BMC Complementary. Med. Therapies. 20, 1–15. doi: 10.1186/s12906-020-03157-2
Unnikrishnan P. S., Jayasri M. A. (2018). Marine algae as a prospective source for antidiabetic compounds–a brief review. Curr. Diabetes Rev. 14, 237–245. doi: 10.2174/1573399812666161229151407
Van Kaer L. (2001). Drugs from the Sea: A marine sponge-derived compound prevents Type 1 diabetes. Sci. World J. 1, 630–632. doi: 10.1100/tsw.2001.357
Vuksan V., Sievenpiper J. L., Koo V. Y., Francis T., Beljan-Zdravkovic U., Xu Z., et al. (2000). American ginseng (Panax quinquefolius L) reduces postprandial glycemia in nondiabetic subjects and subjects with type 2 diabetes mellitus. Arch. Inter. Med. 160, 1009–1013. doi: 10.1001/archinte.160.7.1009
Waldron-Lynch F., Herold K. C. (2011). Immunomodulatory therapy to preserve pancreatic β-cell function in type 1 diabetes. Nat. Rev. Drug Disc. 10, 439–452. doi: 10.1038/nrd3402
Wang Y., Hu B., Feng S., Wang J., Zhang F. (2020). Target recognition and network pharmacology for revealing anti-diabetes mechanisms of natural product. J. Comput. Sci. 45, 101186. doi: 10.1016/j.jocs.2020.101186
Wang R., Paul V., Luesc H. (2013). Seaweed extracts and unsaturated fatty acid constituents from the green alga Ulva lactuca as activators of the cytoprotective Nrf2-ARE pathway. Free Radic. Biol. Med. 57, 141–153. doi: 10.1016/j.freeradbiomed.2012.12.019
West I. C. (2000). Radicals and oxidative stress in diabetes. Diabetic Med. 17, 171–180. doi: 10.1046/j.1464-5491.2000.00259.x
World Health Organization (WHO). (2018). Noncommunicable diseases country profiles 2018. Available online at: https://www.who.int/publications/i/item/9789241514620. (accessed September 24, 2018).
Yang P., Liu D. Q., Liang T. J., Li J., Zhang H. Y., Liu A. H., et al. (2015). Bioactive constituents from the green alga Caulerpa racemosa. Bioorg. Med. Chem. 23, 38–45. doi: 10.1016/j.bmc.2014.11.031
Yuan T., Yang T., Chen H., Fu D., Hu Y., Wang J., et al. (2019). New insights into oxidative stress and inflammation during diabetes mellitus-accelerated atherosclerosis. Redox Biol. 20, 247–260. doi: 10.1016/j.redox.2018.09.025
Zhou L. Y., Xiao C. Q., Gao J., Zhao M. M., Li X. G., Mora L., et al. (2024). Preparation and identification of novel DPP-IV inhibitory peptides from Musculus senhousei: Peptidomic analysis, molecular simulation, and validation. Food Biosci. 59, 103832. doi: 10.1016/j.fbio.2024.103832
Keywords: diabetes mellitus, therapeutics, ROS, marine bioactive compounds, Nrf2, HO-1
Citation: Nisar MF, Li M, Xu J and Wan C (2024) Anti-diabetic effects of marine natural products through redox modulation via Nrf2/HO-1 cytoprotective pathways. Front. Mar. Sci. 11:1438955. doi: 10.3389/fmars.2024.1438955
Received: 27 May 2024; Accepted: 15 October 2024;
Published: 13 November 2024.
Edited by:
Syed Shams ul Hassan, Shanghai Jiao Tong University, ChinaReviewed by:
Syed Qamar Abbas, Sarhad University of Science and Information Technology (SUIT), PakistanChengqian Pan, Jiangsu University, China
Muhammad Ishaq, Shanghai Jiao Tong University, China
Copyright © 2024 Nisar, Li, Xu and Wan. This is an open-access article distributed under the terms of the Creative Commons Attribution License (CC BY). The use, distribution or reproduction in other forums is permitted, provided the original author(s) and the copyright owner(s) are credited and that the original publication in this journal is cited, in accordance with accepted academic practice. No use, distribution or reproduction is permitted which does not comply with these terms.
*Correspondence: Chunpeng Wan, Y2h1bnBlbmd3YW5AanhhdS5lZHUuY24=