- 1Department of Earth and Environmental Sciences, Boston College, Chestnut Hill, MA, United States
- 2Department of Biology, Boston University, Boston, MA, United States
- 3Department of Earth and Planetary Sciences, McGill University, Montreal, QC, Canada
- 4School of Ocean and Earth Science and Technology, University of Hawai’i at Mānoa, Mānoa, HI, United States
The resilience of coral reefs in oligotrophic, (sub)tropical oceans is largely due to the symbiotic relationship between scleractinian corals and Symbiodiniaceae algae, which enables efficient internal nutrient recycling. Investigating the history of this coral symbiosis can provide insights into its role in sustaining the health of both present and future coral reefs. The isotopic composition of organic nitrogen (15N/14N or δ15N) bound within coral skeletons has been utilized to trace the existence of symbiosis in fossil corals, suggesting that coral symbiosis dates back to at least 210 million years ago. The basis of this proxy is that symbiotic corals are expected to exhibit lower δ15N compared to their non-symbiotic (aposymbiotic) counterparts within the same environments, owing to internal nitrogen recycling between the coral host and algal symbiont, and reduced leakage of low-δ15N ammonium into seawater. However, this hypothesis has not been adequately tested in contemporary settings. In a laboratory experiment, we examined the δ15N differences between the symbiotic and aposymbiotic branches within the same genetic backgrounds of the facultatively symbiotic coral Oculina arbuscula under well-fed conditions. Across five different genotypes in two separate experiments, symbiotic branches consistently showed lower δ15N than their aposymbiotic counterparts. These findings corroborate the use of δ15N as a proxy for identifying coral symbiosis in the past, particularly when multiple species of corals coexisted in the same environments.
1 Introduction
Coral reef habitats predominantly exist in oligotrophic (low nutrient) environments in tropical and subtropical oceans (Capone et al., 2008; Dubinsky and Stambler, 2011). These habitats comprise a diverse array of organisms, with scleractinian (stony) corals serving as the primary reef builders. These ecosystems formed by corals are biodiversity hotspots, offering a range of ecosystem services including serving as nursery habitats for juvenile fish, facilitating carbon sequestration, and providing coastal protection against storms (Rosenberg et al., 2007; Woodhead et al., 2019). Remarkably, coral reef tourism alone generates up to $35.8 billion globally per year (Woodhead et al., 2019). However, these vital organisms and the ecosystems they support are under severe threats from rising sea surface temperatures and ocean acidification due to anthropogenic CO2 emissions (Goldberg and Wilkinson, 2004; Dubinsky and Stambler, 2011; Hughes et al., 2018). In addition, local anthropogenic stressors, such as nitrogen (N) pollution, further imperil coral reefs and their ecosystem services by adversely affecting coral health, including increased disease susceptibility and bleaching, loss of coral diversity, and high mortality rates (Rosenberg et al., 2007; D’Angelo and Wiedenmann, 2014; Burkepile et al., 2020). Over the past few decades, coral coverage in tropical and subtropical waters has decreased by approximately 50% (Eddy et al., 2021).
The success of tropical and subtropical coral reefs in oligotrophic waters hinges on their symbiosis with Symbiodiniaceae algae within their gastrodermal cells. The recent decline of coral reefs is largely attributed to the loss of Symbiodiniaceae under conditions of rising water temperatures and other adverse factors, leading to “coral bleaching” (Li et al., 2023). In healthy symbiotic conditions, this symbiosis facilitates the transfer of photosynthetic products from the algae to the coral (Rädecker et al., 2015; Gustafsson et al., 2013; Houlbrèque and Ferrier-Pagès, 2009). In return, the algae assimilates metabolic waste (e.g., N) excreted by the coral, preventing its loss to the ambient ocean (Cui et al., 2019). This symbiosis fosters an “internal nutrient recycling” mechanism, enabling corals to conserve nutrients and thrive in oligotrophic waters. In the absence of these algal symbionts, corals would struggle to thrive due to nutrient scarcity (Grover et al., 2008; Yellowlees et al., 2008; Falkowski et al., 1984; D’Elia et al., 1983). As a result, the once-healthy symbiotic relationship deteriorates, resulting in reduced coral growth rates, increased mortality rates, and ultimately, coral reef degradation.
Numerous studies have investigated the interactions between the host coral and their algal symbionts (Davy et al., 2012; Kopp et al., 2013; Weis et al., 2008; Yellowlees et al., 2008), though most have focused on present-day coral symbioses. The response of coral symbiosis to a warmer climate in the past, however, might also provide important insights into the future of coral symbiosis under global warming (Frankowiak et al., 2016; Weis et al., 2008; Pochon et al., 2006). While corals leave behind skeletal fossils composed of calcium carbonate, Symbiodiniaceae do not, complicating the study of historical coral symbiosis. The molecular clock technique has been used to study the origin of symbiosis in contemporary scleractinian corals, placing it between approximately 140 Ma to 200 Ma (LaJeunesse et al., 2018). However, this method is based on assumptions regarding genetic mutation rates and can lack precision without corroboration from fossil evidence. Moreover, this late origin of symbiosis in stony corals is at odds with the rapid expansion of ancient coral reefs around 200–251 Ma during the Triassic period (Stanley, 2003).
In addition to the molecular clock approach, indirect “proxies” derived from coral skeleton records have also been employed to infer the origin and evolution of the coral-algal symbiosis. These proxies include skeletal microstructures and the isotopic composition of carbon (C), oxygen (O), and nitrogen (N) (Stanley and Swart, 1995; Frankowiak et al., 2016). Notably, the N isotopic composition (15N/14N or δ15N) of skeleton-bound organic matter has been recently utilized to suggest the presence of symbiosis in fossil corals dating back to approximately 210 million years (Frankowiak et al., 2016; Tornabene et al., 2017). These studies have also suggested that these corals lived in oligotrophic water and that symbiosis with photosynthetic algae was crucial for the evolution and expansion of scleractinian corals (Frankowiak et al., 2016).
The coral δ15N symbiosis proxy is predicated on the differences in skeletal δ15N values between symbiotic and aposymbiotic corals within identical environmental settings (Frankowiak et al., 2016). Nitrogen has two stable isotopes, 14N and 15N. 14N constitutes ~99.63% of naturally occurring N, while 15N accounts for the remaining ~0.37% (Reddy and DeLaune, 2008). The ratio of 15N to 14N in a sample is expressed with the δ15N notation, defined as δ15N = [(15N/14Nsample)/(15N/14Nair)-1] × 1,000‰. In a typical ecosystem, a trophic enrichment of approximately ~3‰ in δ15N is observed at each successive trophic level, which is due to the preferential breakdown of 14N-rich proteins during catabolism and the excretion of 14N-rich ammonium or urea (Deniro and Epstein, 1981; Minagawa and Wada, 1984). In symbiotic corals, however, the 14N-rich ammonium is recycled within the symbiotic system, reducing its excretion to the surrounding seawater and consequently limiting isotopic fractionation. As a result, when residing in identical environments, symbiotic corals are expected to exhibit lower δ15N values compared to their non-symbiotic counterparts. However, this hypothesis has not been sufficiently tested in contemporary settings using extant coral species as most coral δ15N studies have focused on its use as a trophic level indicator in modern corals (Conti-Jerpe et al., 2020; Wiedenmann et al., 2023).
In this study, we investigate the impact of symbiosis on coral δ15N using a facultatively symbiotic coral species, Oculina arbuscula. Unlike most extant symbiotic corals that harbor obligate symbionts, facultatively symbiotic corals exhibit a range of symbiotic states—from hosting high to low densities of algal symbionts—and can even be manipulated to achieve aposymbiosis (functionally devoid of symbionts) in laboratory settings similar to other closely related cnidarian clades (Madin et al., 2016; Matthews et al., 2016). This flexibility allows for a detailed examination of the differences between symbiotic states. We explored the δ15N differences between aposymbiotic and symbiotic branches of Oculina arbuscula under consistent laboratory conditions. Our experimental design focuses on a single species while also considering the influence of genetic background on δ15N, thereby isolating the presence or absence of symbionts as the sole variable. The results could enhance our understanding of δ15N as a proxy for symbiosis in fossil corals and provide valuable information on how contemporary corals may respond to global warming.
2 Materials and methods
2.1 Sample collection
All facultatively symbiotic coral colonies, Oculina arbuscula Agassiz, 1864, were collected on May 25, 2018 from Radio Island, North Carolina (34° 42.520′ N, 76° 40.796′ W; Supplementary Figure 1) from depths of 4.5–6 m using a hammer and chisel (NC Division of Marine Fisheries Permit #1627488; Rivera and Davies, 2021). Five genetically distinct parent colonies (genets) of O. arbuscula were collected and transported overnight to the Davies Marine Population Genomics Lab at Boston University. Parent colonies were used to generate two nubbins per colony for each experiment, creating genotypic replicates, and resulting in a total of 8 nubbins used in Experiment 1 and 10 nubbins used in Experiment 2. These coral fragments were then attached to ceramic tiles and acclimated in a common tank until used for the experiments. Although O. arbuscula used in our experiment does not inhabit the same environmental conditions as tropical reef-building corals, they are able to calcify (Rivera and Davies, 2021), making an effective model species for groundtruthing the use of δ15N as a proxy for symbiosis in extant corals.
2.2 Laboratory experiments
Corals were maintained under common garden conditions, with a temperature of 25 ± 0.17°C, salinity of 34.6 ± 0.76, and pH of 8.0 ± 0.08 for 3–4 years leading up to and throughout the sampling period. Three months prior to each sampling date, one nubbin from each genet was menthol-bleached for 7 days in a 20% weight/volume menthol solution (Matthews et al., 2016). Following menthol bleaching, aposymbiotic fragments were housed in a separate tank from symbiotic fragments (Figure 1; Matthews et al., 2016; Wang et al., 2012). Each experimental tank was equipped with a powerhead (1321 gallons hr-1) to ensure water circulation within the tank. Additionally, a large pump was used to recirculate water (4000 gallons hr-1) among all experimental tanks. Water changes were conducted every other week, during which turf algal growth on the tank walls was scrubbed, and about 1/3 of the total tank volume was replaced. Two experiments, each lasting approximately 3 months, were conducted. Aposymbiotic and symbiotic fragments from the same genet were paired for multiple coral colonies (Experiment 1: 4 genets; Experiment 2: 5 genets). In both experiments, corals were fed the same diet (combined Artemia nauplii and commercial coral food) three times a week for the duration of each experiment.
2.3 Host/symbiont separation
Three months following menthol bleaching of aposymbiotic fragments, tissue samples were taken from a symbiotic and aposymbiotic nubbin from each genet using the same process outlined below (n=8 coral fragments for Experiment 1 and n=10 coral fragments for Experiment 2). Briefly, a section of coral was detached and the surface of the coral tissue was removed via airbrushing using 0.2 micron filter sterilized artificial seawater (FSW, Instant Ocean) (Figure 1; Rivera et al., 2023; Aichelman et al., 2021). The tissue slurry was homogenized using a handheld tissue homogenizer for 3 minutes (Tissue-Tearor Model 985370, BioSpec Products, Inc.). A subsample of the slurry was preserved at -20°C as the ‘Host + Symbiont’ sample, which included the animal and algal symbiont cells for further analyses (detailed below). The remaining slurry underwent centrifugation at 3300 rpm for 10 minutes to separate the animal cells ‘Host’ (supernatant) from the Symbiodiniaceae ‘Symbiont’ (pellet) components. The ‘Host’ fraction was decanted, further purified via centrifugation at 12,000 rpm at 4°C for 10 minutes, transferred to a new tube, and stored at -80°C. The ‘Symbiont’ fraction was treated with 2 mL of 2N HCl to dissolve calcium carbonate particles. This pellet was then rinsed three times with FSW, each time followed by centrifugation at 3000 rpm for 3 minutes. After the final rinse, the supernatant was discarded, and the pellet was resuspended in 1.5 mL FSW and sieved through a 40-micron mesh. The filtrate was subjected to a final centrifugation at 12,000 rpm at 4 °C for 10 minutes. After this, the supernatant was discarded, and the remaining pellet was stored at -80°C.
2.4 Symbiont density analyses
The ‘Host + Symbiont’ aliquots were used to determine symbiont cell densities (cells mm-2). Three replicates per coral genet and symbiosis type were measured using 10 μL of tissue slurry aliquots with a hemocytometer under a light microscope (Rodrigues and Grottoli, 2007). Counts were averaged, extrapolated to the total slurry volume, and normalized to coral tissue surface area (as measured by an Einscan-SE scanner and MeshLab software) to quantify symbiont densities for each coral fragment.
2.5 δ15N analysis
All coral tissue samples were freeze-dried overnight (Figure 1). Samples were then homogenized and crushed to form a powder. These powdered samples were weighed into tin capsules (Kolasinski et al., 2008). Their nitrogen isotopic composition was analyzed on an isotope ratio mass spectrometer interfaced with a FlashSmart™ Elemental Analyzer (EA-IRMS; ThermoFisher Scientific; Figure 1). Although coral samples were separated into ‘Host’, ‘Host + Symbiont’, and ‘Symbiont’ fractions, only the ‘Host’ and ‘Host + Symbiont’ samples yielded sufficient mass for precise isotopic data. The ‘Symbiont’ fraction produced a peak intensity on the EA-IRMS that was only marginally higher than the blanks, rendering the data unusable. Consequently, we report only the δ15N values of the ‘Host’ and ‘Host + Symbiont’ fractions in this paper. The precision of the method, based on replicates and standards, was approximately 0.2‰. The δ15N of the food mixture was also measured alongside the coral samples.
2.6 Nitrate concentration and δ15N analysis
To analyze the nitrate (NO3-) concentrations and δ15N values of the water, samples were collected from the tank at the onset and conclusion of Experiment 2. Both NO3- concentration and δ15N were determined using the denitrifier method (Sigman et al., 2001). Briefly, NO3- in the water sample was converted into N2O using Pseudomonas aureofaciens in pre-purged 20 mL gas-tight vials. The concentration and δ15N of the resulting N2O were then analyzed on a customized Gas Bench coupled to an IRMS (GB-IRMS). NO3- isotope standards with known concentrations (IAEA-NO3 and USGS 34) were used to calibrate the NO3- δ15N and concentrations of the samples. The precision of this method for NO3- δ15N and concentration are better than 0.2‰ and 2%, respectively.
2.7 Statistical analysis
δ15N for the ‘Host’ and ‘Host + Symbiont’ were assessed using a series of linear mixed effects models (lmer) for each (including fixed effects of experiment and symbiosis type) using a forward model selection method as described in Aichelman et al. (2021) using R Version 1.3.959 (R Core Team, 2017). A random effect of genet was included in all models to account for variation across genets. Assumptions of normality were assessed using a Shapiro–Wilk Test. Assumptions for δ15N for the ‘Host’ and ‘Host + Symbiont’ were both met. The best fit model was derived by starting with the intercept-only model and then using forward-selection to incorporate additional parameters, starting with the most significant parameter, until further addition of parameters did not significantly improve the model fit. Additional parameters were retained in the model if they were significant (p<0.05) and produced smaller AIC values (Akaike, 1974). Comparisons between the δ15N averages of the aposymbiotic ‘Host’ and the symbiotic ‘Host’ and ‘Host + Symbiont’ were performed using a Tukey’s HSD post-hoc pairwise comparison for each experiment (lsmeans). A one-way ANOVA with a Tukey’s HSD posthoc pairwise comparison were performed for significant effects from the linear mixed model for each genet in Prism GraphPad Version 10.2.3.
3 Results
3.1 Cell density
Symbiont densities were highest in symbiotic coral and lowest for aposymbiotic coral for both experiments, which was expected. Three abnormal symbiont densities were observed in aposymbiotic corals across all experiments, where the density was not the expected 0 cells mm-2. In Experiment 1, the aposymbiotic coral for genet A showed a density of 65 cells mm-2. In Experiment 2, the aposymbiotic corals for genet A and F had a cell density of 48 cells mm-2 and 124 cells mm-2, respectively (Table 1). The cell densities for genet D and E in symbiotic corals in Experiment 2 were also lower, registering at 3,125 cells mm-2 and 5,626 cells mm-2, respectively, compared to other symbiotic coral for all experiments that had an average cell density of 13,286 cells mm-2 (Table 1).

Table 1. Aposymbiotic and symbiotic coral tissue symbiont count (cells mm-2) for all genets in Experiments 1 and 2.
3.2 Nitrogen isotopes
Overall, both the δ15N of the ‘Host’ and ‘Host + Symbiont’ for the corals exhibited distinct differences between the two types (i.e., symbiotic coral and aposymbiotic coral) in the first experiment (p1, Apo ‘Host’ – Sym ‘Host’ = 0.014, p1, Apo ‘Host’ – Sym ‘Host+Sym’ = 0.0001; Supplementary Table 1). Experiment 2 revealed a significant difference only between the aposymbiotic ‘Host’ and symbiotic ‘Host + Symbiont’ (p2, Apo ‘Host’ – Sym ‘Host+Sym’ = 0.0014; Supplementary Table 1) and no differences between the aposymbiotic ‘Host’ and symbiotic ‘Host’ (p2, Apo ‘Host’ – Sym ‘Host’ = 0.36; Supplementary Table 1). In each experiment, the δ15N values of the symbiotic branches were consistently lower than those of the corresponding aposymbiotic branches (Figure 2). Between the two experiments (1 and 2), significant differences were observed for the ‘Host’ and ‘Host + Symbiont’ δ15N (p Apo ‘Host’ – Sym ‘Host’ = 0.0023, p Apo ‘Host’ – Sym ‘Host+Sym’ < 0.0001; Table 2).
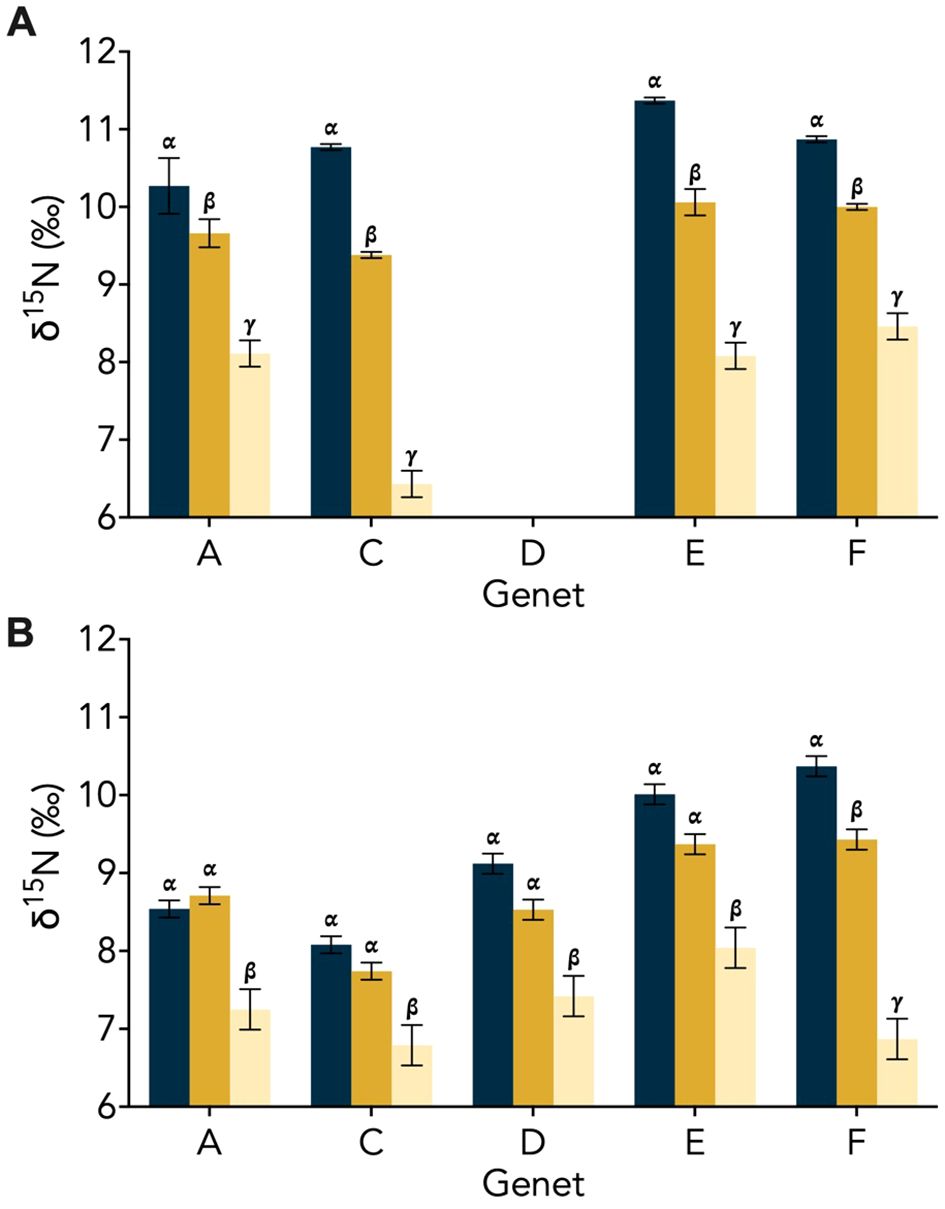
Figure 2. Genet δ15N (± SD) for the aposymbiotic ‘Host’ (blue), symbiotic ‘Host’ (dark yellow), and symbiotic ‘Host + Symbiont’ (light yellow) for the Oculina arbuscula corals from (A) Experiment 1 and (B) Experiment 2. Different Greek letters (α, β, γ) indicate a significant difference (p < 0.05). For all variables with the same Greek letter, the difference between the means is not statistically significant.
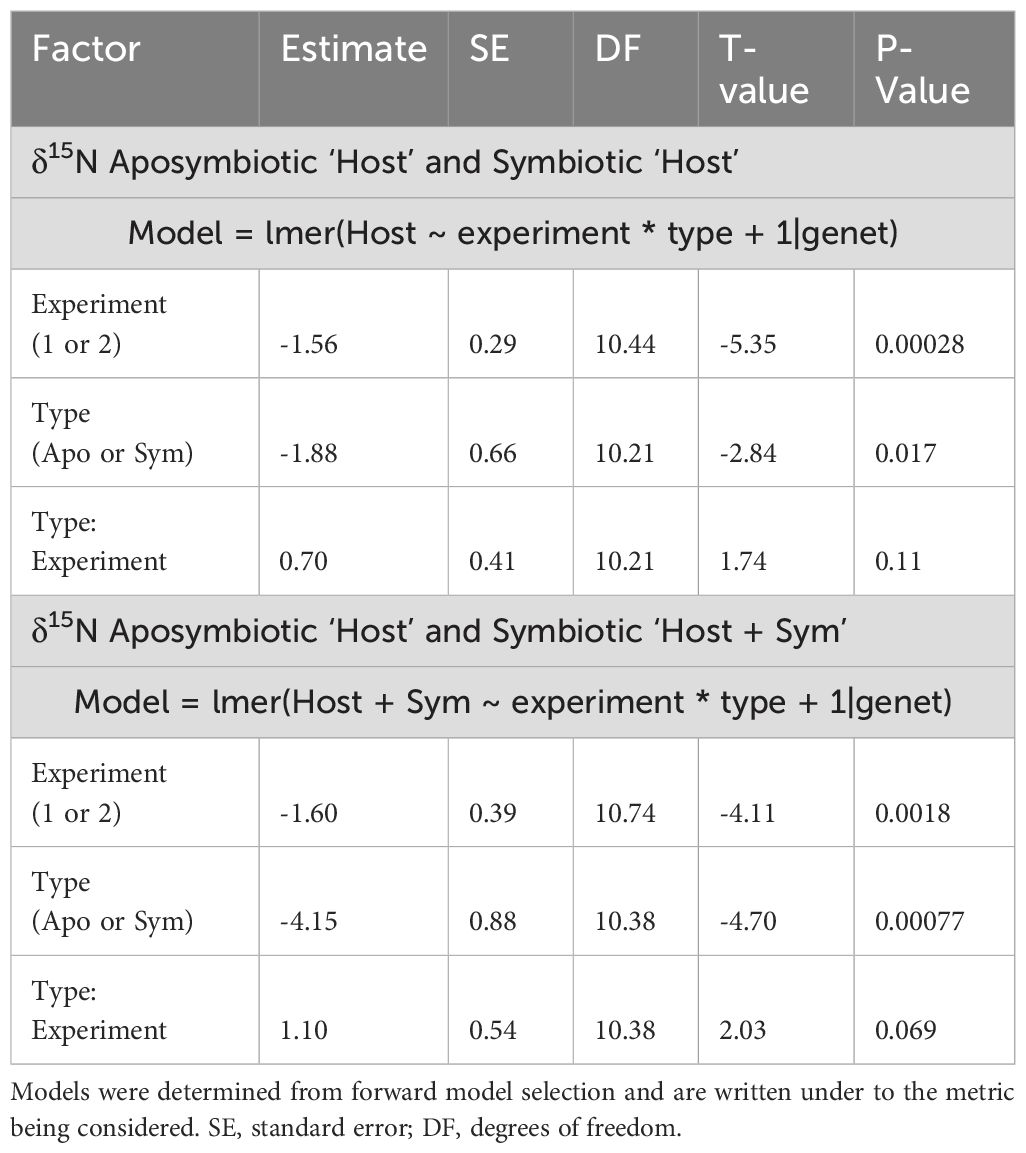
Table 2. Model results for δ15N for the aposymbiotic and symbiotic ‘Host’ as well as aposymbiotic ‘Host’ and symbiotic ‘Host + Symbiont’ (experiment and type).
In Experiment 1, the δ15N of the aposymbiotic ‘Host’ ranged from 10.3–11.4‰, while that of the symbiotic ‘Host’ ranged from 9.4–10.1‰ and the symbiotic ‘Host + Symbiont’ ranged from 6.4–8.5‰ (Figure 2A). Across all genets in the first experiment, there was a significant difference between the aposymbiotic ‘Host’, symbiotic ‘Host’, and the symbiotic ‘Host + Symbiont’ (Figure 2A; Supplementary Table 2). In Experiment 2, the δ15N of all coral samples were lower than in Experiment 1, with the aposymbiotic ‘Host’ ranging from 8.1–10.4‰, the symbiotic ‘Host’ from 7.7–9.4‰, and the symbiotic ‘Host + Symbiont’ from 6.8–8.0‰ (Figure 2B). For all genets in Experiment 2, there was no significant difference between the aposymbiotic ‘Host’ and the symbiotic ‘Host’ except for genet F (pF, Apo ‘Host’ – Sym ‘Host’ = 0.029; Supplementary Table 2). However, there was a significant difference between the aposymbiotic ‘Host’ and the symbiotic ‘Host + Symbiont’ as well as the symbiotic ‘Host’ and the symbiotic ‘Host + Symbiont’ (Figure 2B; Supplementary Table 2).
In both experiments, the δ15N of the symbiotic ‘Host + Symbiont’ was >2‰ lower than the aposymbiotic ‘Host’. When averaging the δ15N of all genets for each experiment, the offset between the aposymbiotic ‘Host’ and the symbiotic ‘Host + Symbiont’ was 2.9‰ for Experiment 1 and 2.0‰ for Experiment 2. The difference for both experiments was significantly different (Experiment 1: p = 0.0001, t-ratio = 7.6, df = 10.0; Experiment 2: p = 0.0014, t-ratio = 5.4, df = 10.0; Supplementary Table 1; Figure 3). For Experiment 1, there was also a significant difference between the averages of the aposymbiotic ‘Host’ and symbiotic ‘Host’ (p = 0.013, t-ratio = 3.9, df = 10.0; Supplementary Table 1), and between the symbiotic ‘Host’ and symbiotic ‘Host + Symbiont’ (p < 0.0001, t-ratio = 7.9, df = 11.0; Supplementary Table 1). For Experiment 2, there was only an additional significant difference between the symbiotic ‘Host’ and symbiotic ‘Host + Symbiont’ (p < 0.0001, t-ratio = 7.9, df = 11.0; Supplementary Table 1). The δ15N of the food mixture used during the duration of the study averaged to be 8.0 ± 2.0‰. In Experiment 2, water samples were collected at both the start and end for nitrate concentration and δ15N measurements. The nitrate concentration increased from 2.3 ± 0.02 μM in the beginning to 14.2 ± 0.24 μM at the end. Nitrate δ15N decreased from 14.5 ± 0.1‰ to 10.3 ± 0.1‰.
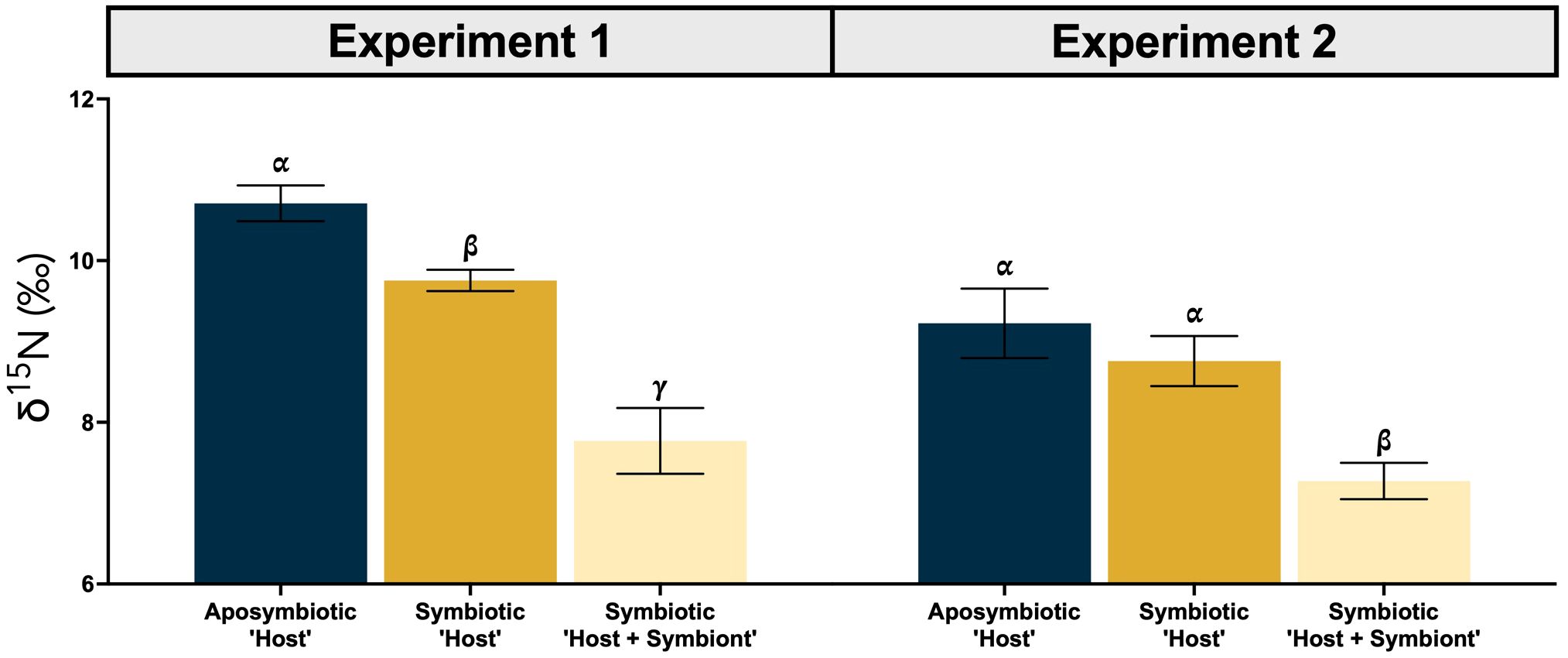
Figure 3. Average δ15N (± SE) for the aposymbiotic ‘Host’ (blue), symbiotic ‘Host’ (dark yellow), and symbiotic ‘Host + Symbiont’ (light yellow) for the Oculina arbuscula corals from Experiment 1 (left) and 2 (right). Different Greek letters (α, β, γ) indicate a significant difference (p < 0.05). For all variables with the same Greek letter, the difference between the means is not statistically significant.
4 Discussion
4.1 Influence of symbiosis on coral δ15N
While coral δ15N has been used as a proxy to determine the presence of coral symbiosis in fossil corals (Frankowiak et al., 2016; Tornabene et al., 2017), its efficacy has not been sufficiently groundtruthed in contemporary settings with extant corals. Using a facultatively symbiotic coral species under well-fed conditions in a controlled laboratory experiment, we observed that the δ15N of the symbiotic fragments were lower than that of the aposymbiotic fragments while controlling for genetic background (Figure 2). This difference can be attributed to the internal N cycling between the coral host and its symbionts (Rädecker et al., 2015; Gustafsson et al., 2013; Houlbrèque and Ferrier-Pagès, 2009; Bednarz et al., 2017), which results in reduced or minimal low-δ15N ammonium excretion and less 15N enrichment in corals. Although the overall average δ15N values were higher in the aposymbiotic ‘Host’ compared to both the symbiotic ‘Host’ and ‘Host + Symbiont’ (Figure 3), the differences between the aposymbiotic ‘Host’ and symbiotic ‘Host’ were not statistically significant in Experiment 2 (Figure 3). This may be attributed to the high frequency of feeding during the experiment, which will be discussed further in Section 4.3.
In the experiments, the average δ15N of coral food was 8.0 ± 2.0‰. Given aposymbiotic corals lack symbionts, their excretion of low-δ15N ammonium would lead to an increase of 3–4‰ in δ15N relative to that of the food source under steady-state conditions (Deniro and Epstein, 1981; Minagawa and Wada, 1984; Glibert et al., 2019). The δ15N differences between the aposymbiotic corals and their food averaged 2.7‰ in Experiment 1 and 1.2‰ in Experiment 2. The smaller offset in Experiment 2 may be attributed to the experimental duration. Specifically, the aposymbiotic corals had been stripped of their algal symbionts about 3 months prior and might require more time to acclimate and exhibit the 3‰ increase.
4.2 N heterotrophy vs. autotrophy in symbiotic corals
Aposymbiotic corals obtain N through heterotrophic means, despite recent research indicating that aposymbiotic coral have the potential to assimilate ammonium at a much lower rate than symbiotic coral in high ambient DIN conditions (Cui et al., 2019). In contrast, symbiotic corals have been reported to obtain N through both heterotrophic feeding on particulate organic N (PON) and plankton (N heterotrophy; Gustafsson et al., 2013; Houlbrèque and Ferrier-Pagès, 2009) and autotrophic assimilation of dissolved inorganic N (DIN) from seawater via their intracellular algae (N autotrophy; Gustafsson et al., 2013; Cui et al., 2019). However, most experiments that explore DIN assimilation are conducted under little to no feeding and/or high DIN concentrations (Bythell, 1990; Hoegh-Guldberg and Williamson, 1999; Grover et al., 2002; 2003; Tanaka et al., 2006; Pernice et al., 2012; Kopp et al., 2013; Tanaka et al., 2015; Bednarz et al., 2017). Discrepancies exist in the literature regarding food availability and its influence on DIN assimilation (DiRoberts et al., 2021), although starvation (i.e., no feeding) could potentially increase DIN assimilation (Szmant-Froelich and Pilson, 1984; Grover et al., 2002). It is still unclear whether symbiotic corals rely on N heterotrophy vs. autotrophy in natural environments.
To minimize potential assimilation of DIN by corals, corals were purposely fed three times per week in our experiments, mimicking highly productive ocean environments where corals have access to plenty of food such as those of the central and eastern equatorial Pacific (Wang et al., 2016). This approach also reflected the natural habitat of O. arbuscula, which thrives in subtropical to temperate regions-environments that are nutrient-richer than that of oligotrophic waters (Gleason et al., 2018). This frequent feeding led to a build-up of nitrate in the tank and turf algae growth due to the degradation of coral food, which increased from 2.3 ± 0.02 μM to 14.0 ± 0.28 μM over the course of Experiment 2, despite periodic water replacement and turf algae removal. Nitrate δ15N decreased from 14.5 ± 0.1‰ to 10.3 ± 0.1‰ in Experiment 2, inconsistent with assimilation of seawater nitrate by coral. In such nutrient-rich environments, it is energetically more favorable for symbionts to utilize ammonium excreted by the coral host rather than assimilating seawater nitrate, which must cross multiple membrane layers before being reduced to ammonium and taken up by the symbionts. Thus, in high-productivity ocean environments, coral N heterotrophy likely dominates over N autotrophy.
4.3 Ammonium leakage in well-fed symbiotic coral and its impact on coral δ15N
Most natural conditions in which corals live are oligotrophic in nature, leading to highly efficient N recycling within symbiotic corals with little to no ammonium leakage (O’Neil and Capone, 2008). As a result, symbiotic corals are expected to exhibit a ~3‰ lower δ15N than co-existing aposymbiotic corals. However, in our experiments, the observed δ15N offset between aposymbiotic coral and symbiotic coral generally falls below this 3‰ (Figures 4A, B), suggesting an underlying mechanism that warrants further investigation.
Previous studies have suggested that well-fed corals may exhibit increased ammonium leakage because the symbionts are unable to fully assimilate the ammonium excreted by the coral host (Szmant-Froelich and Pilson, 1984; Piniak et al., 2003; Piniak and Lipschultz, 2004; Erler et al., 2015; Wang et al., 2015), with the rate of ammonium leakage increasing with the frequency of feeding. This implies that an abundant food supply could lead to a rise in coral δ15N even in symbiotic corals (Erler et al., 2015; Wang et al., 2015), which may explain the observations from our experiments. While aposymbiotic coral will remain consistent in their δ15N regardless of food supply (Figure 5A), symbiotic corals may display a range in their δ15N (Figures 5B, C). Specifically, in Experiment 2, in which both the aposymbiotic ‘Host’ and symbiotic ‘Host’ displayed more similar δ15N values (Figure 4B), suggests ammonium leakage occurred in both coral types. Depending on the extent of this leakage, symbiotic corals could show a range of δ15N elevations relative to their food source (Figure 5B). In scenarios with no leakage (i.e., in oligotrophic waters), coral δ15N might not display any trophic enrichment relative to their food. In high leakage scenario (i.e., in highly productive environments), coral δ15N could be up to ~3‰ higher than the δ15N of their food.
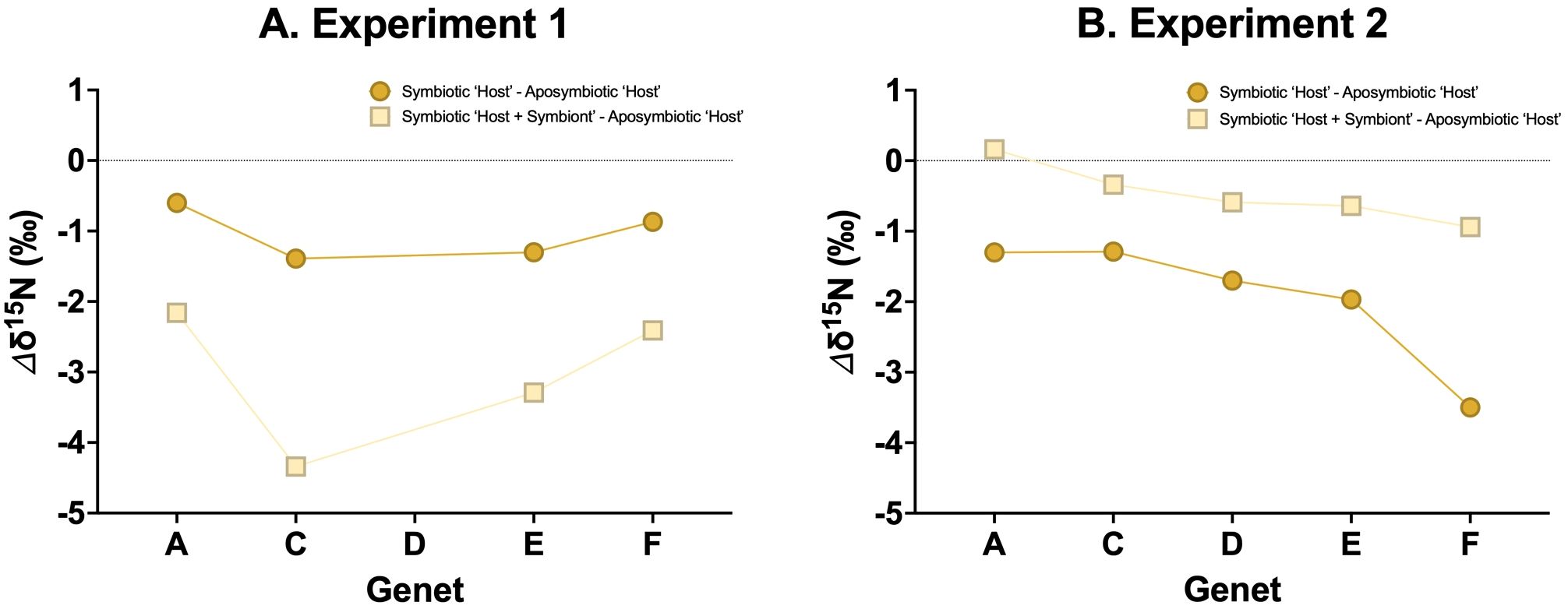
Figure 4. δ15N differences (Δδ15N) between the aposymbiotic ‘Host’ and symbiotic ‘Host’ (dark yellow circles) and the aposymbiotic ‘Host’ and symbiotic ‘Host + Symbiont’ (light yellow squares) for (A) Experiment 1 and (B) Experiment 2.
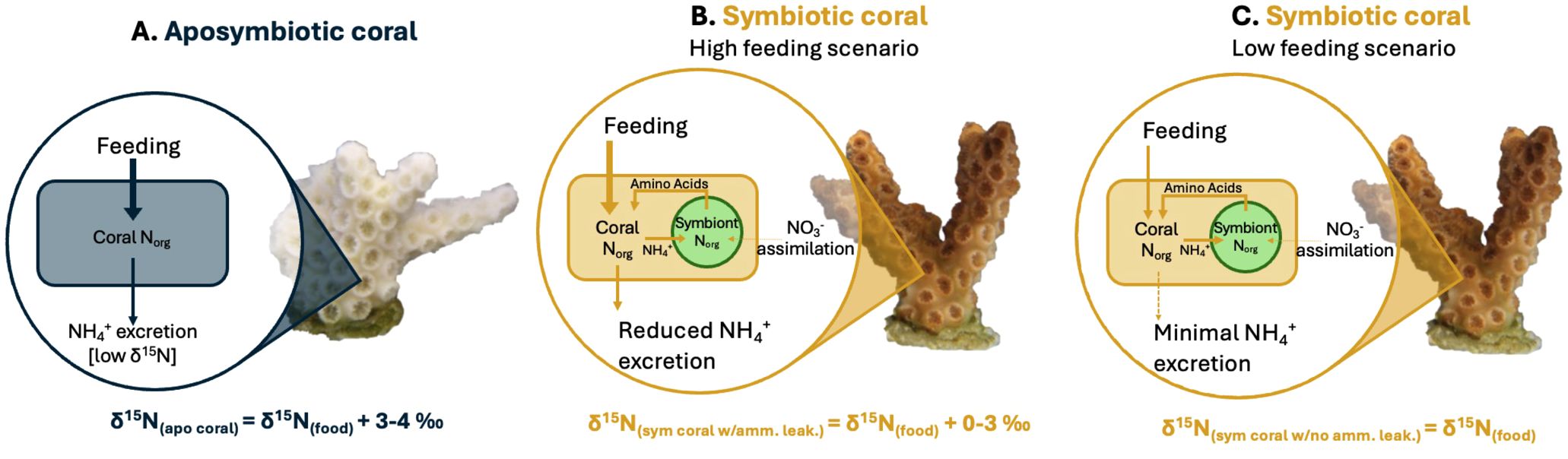
Figure 5. Cartoons showing the causes of δ15N differences between (A) aposymbiotic coral, (B) symbiotic coral with reduced ammonium leakage, and (C) symbiotic coral with minimal ammonium leakage. The δ15N value of a symbiotic coral with ammonium leakage will fall between the δ15N of symbiotic coral with minimal ammonium leakage and the δ15N of aposymbiotic coral. Note: coral growth is not included in this illustration. As most corals inhabit oligotrophic environments, the assimilation of inorganic nitrogen [i.e., nitrate (NO3-) or ammonium (NH4+)] from seawater by the algal symbiont, Symbiodiniaceae, inside the symbiotic coral is considered negligible in this illustration. In all 3 scenarios the input arrow is thicker than the output arrow as a portion of the N is used for coral growth.
4.4 Implications for coral δ15N as a symbiosis proxy
To assess the utility of δ15N as a proxy for coral symbiosis, we calculated the δ15N offset (Δδ15N) between symbiotic and aposymbiotic corals. This offset can be used to determine the symbiotic state of the coral, with the Δδ15N values ranged from -4 to 1‰ (Figure 6A). This range can be attributed to the degree of ammonium leakage, with a Δδ15N close to 0‰ indicating full aposymbiosis and -4‰ suggesting full symbiosis.
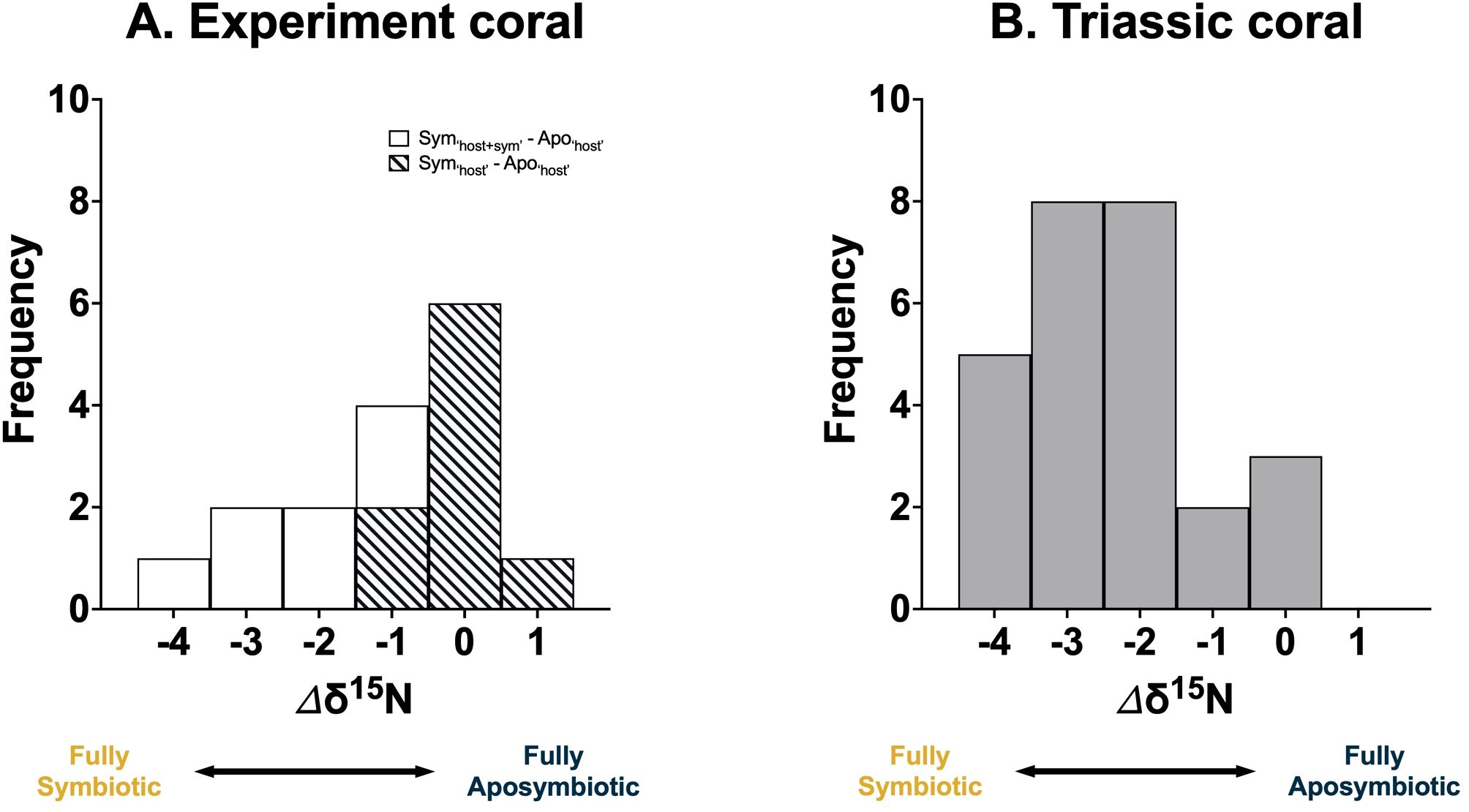
Figure 6. Frequency distribution of the observed coral δ15N offset (Δδ15N) from the (A) ‘Host + Symbiont’ (white) and ‘Host’ (diagonal slash) of the facultatively symbiotic coral Oculina arbuscula under laboratory conditions from this study and (B) Triassic coral (grey) from Antalya, Turkey from a previous study (Frankowiak et al., 2016). Fully aposymbiotic corals release low-δ15N ammonium and exhibit a higher δ15N. In contrast, fully symbiotic corals demonstrate high internal N recycling efficiency, minimal ammonium leakage, and lower δ15N.
Applying this framework to fossil corals requires analyzing multiple coral samples from a specific region. The highest δ15N value within a fossil group can be designated as representing the ‘fully aposymbiotic’ state. The Δδ15N from this baseline then helps determine the symbiotic state of the corals. To validate this framework, δ15N data from a previous study using multiple species of Triassic corals from Antalya, Turkey was used (Frankowiak et al., 2016), which showed a similar Δδ15N range as our experiments (Figure 6B), supporting the conclusion that that some of these fossil corals were indeed symbiotic.
5 Conclusion
As climate change impacts marine ecosystems, corals are increasingly expelling their symbiotic algae, leading to mass bleaching events and elevated mortality rates. Understanding the historical evolution between corals and their intracellular algal symbionts is crucial for the health of both present and future coral reefs. A primary challenge in tracing the evolution of coral symbiosis is that symbiotic algae are not preserved in the fossil record. Recent studies have used the δ15N of fossil-bound organic matter as a proxy for symbiosis in Scleractinian corals as old as 210-million-years-old, uncovering evidence of early symbioses (Tornabene et al., 2017; Frankowiak et al., 2016). In our study, we employed the modern facultatively symbiotic coral, Oculina arbuscula, to validate the δ15N differences between aposymbiotic and symbiotic corals. We found that the δ15N of the aposymbiotic corals was overall higher than that of the symbiotic corals, most likely due to internal N cycling facilitated by the symbiont. These experimental results support the use of δ15N as an indirect proxy for coral symbiosis, applicable to both historical and contemporary settings. However, one limitation of our study is that the corals were maintained under well-fed conditions, which may not accurately represent the nutrient-poor environments typical of most coral reefs. Future research should aim to replicate these experiments under oligotrophic conditions to better simulate natural reef conditions.
Data availability statement
The original contributions presented in the study are included in the article/Supplementary Material. Further inquiries can be directed to the corresponding author.
Ethics statement
The manuscript presents research on animals that do not require ethical approval for their study.
Author contributions
HD: Data curation, Formal analysis, Investigation, Methodology, Resources, Validation, Visualization, Writing – original draft, Writing – review & editing. MV: Data curation, Formal analysis, Funding acquisition, Investigation, Methodology, Resources, Writing – original draft, Writing – review & editing. ML: Data curation, Formal analysis, Investigation, Methodology, Writing – review & editing. HR: Conceptualization, Data curation, Formal analysis, Investigation, Methodology, Writing – review & editing. CT: Data curation, Formal analysis, Investigation, Methodology, Writing – review & editing. SD: Conceptualization, Data curation, Formal analysis, Funding acquisition, Investigation, Methodology, Project administration, Resources, Supervision, Validation, Writing – review & editing. XW: Conceptualization, Data curation, Formal analysis, Funding acquisition, Investigation, Methodology, Project administration, Resources, Supervision, Validation, Visualization, Writing – review & editing.
Funding
The author(s) declare financial support was received for the research, authorship, and/or publication of this article. Funding was provided by the U.S. Army Engineer Research and Development Center to XW (W912HZ2020061-RA2), Boston College Start-up funds to XW, Boston University Start-up funds to SD, NSF funds to SD (IOS-SDS 1937650), BU URBAN (NSF NRT DGE 1735087), and NSF GRFP to MV.
Acknowledgments
We thank Linh Ta for helping in freeze drying the coral tissue samples.
Conflict of interest
The authors declare that the research was conducted in the absence of any commercial or financial relationships that could be construed as a potential conflict of interest.
Publisher’s note
All claims expressed in this article are solely those of the authors and do not necessarily represent those of their affiliated organizations, or those of the publisher, the editors and the reviewers. Any product that may be evaluated in this article, or claim that may be made by its manufacturer, is not guaranteed or endorsed by the publisher.
Supplementary material
The Supplementary Material for this article can be found online at: https://www.frontiersin.org/articles/10.3389/fmars.2024.1433382/full#supplementary-material
References
Aichelman H. E., Bove C. B., Castillo K. D., Boulton J. M., Knowlton A. C., Nieves O. C., et al. (2021). Exposure duration modulates the response of Caribbean corals to global change stressors. Limnology Oceanography 66, 3100–31155. doi: 10.1002/lno.11863
Akaike H. (1974). A new look at the statistical model identification. IEEE Trans. Automatic Control 19, 716–723. doi: 10.1109/TAC.1974.1100705
Bednarz V. N., Grover R., Maguer J. F., Fine M., Ferrier-Pagès C. (2017). The assimilation of diazotroph-derived nitrogen by scleractinian corals depends on their metabolic status. mBio 8, 105. doi: 10.1128/mbio.02058-16
Burkepile D. E., Shantz A. A., Adam T. C., Munsterman K. S., Speare K. E., Ladd M. C., et al. (2020). Nitrogen identity drives differential impacts of nutrients on coral bleaching and mortality. Ecosystems 23, 798–811. doi: 10.1007/s10021-019-00433-2
Bythell J. C. (1990). Nutrient uptake in the reef-building coral acropora palmata at natural environmental concentrations. Mar. Ecol. Prog. Ser. 68, 65–69. doi: 10.3354/meps068065
Capone D. G., Bronk D. A., Mulholland M. R., Carpenter E. J. (2008). Nitrogen in the Marine Environment. 2nd ed (Burlington, MA: Academic Press).
Conti-Jerpe I. E., Thompson P. D., Wong C. W. M., Oliveira N. L., Duprey N. N., Moynihan M. A., et al. (2020). Trophic strategy and bleaching resistance in reef-building corals. Sci. Adv. 6, eaaz5443. doi: 10.1126/sciadv.aaz5443
Cui G., Liew Y. J., Li Y., Kharbatia N., Zahran N. I., Emwas A.-H., et al. (2019). Host-dependent nitrogen recycling as a mechanism of symbiont control in aiptasia. PloS Genet. 15, e10081895. doi: 10.1371/journal.pgen.1008189
D’Angelo C., Wiedenmann J. (2014). Impacts of nutrient enrichment on coral reefs: new perspectives and implications for coastal management and reef survival. Curr. Opin. Environ. Sustainability 7, 82–93. doi: 10.1016/j.cosust.2013.11.029
D’Elia C. F., Domotor S. L., Webb K. L. (1983). Nutrient uptake kinetics of freshly isolated zooxanthellae. Mar. Biol. 75, 157–167. doi: 10.1007/BF00405998
Davy S. K., Allemand D., Weis V. M. (2012). Cell biology of cnidarian-dinoflagellate symbiosis. Microbiol. Mol. Biol. Rev. 76, 229–615. doi: 10.1128/mmbr.05014-11
Deniro M. J., Epstein S. (1981). Influence of diet on the distribution of nitrogen isotopes in animals. Geochimica Cosmochimica Acta 45, 341–515. doi: 10.1016/0016-7037(81)90244-1
DiRoberts L. E., Dudek A., Ray N. E., Fulweiler R. W., Rotjan R. D. (2021). Testing assumptions of nitrogen cycling between a temperate, model coral host and its facultative symbiont: symbiotic contributions to dissolved inorganic nitrogen assimilation. Mar. Ecol. Prog. Ser. 670, 61–74. doi: 10.3354/meps13731
Dubinsky Z., Stambler N. (Eds.) (2011). Coral Reefs: An Ecosystem in Transition (Dordrecht: Springer Netherlands). doi: 10.1007/978-94-007-0114-4
Eddy T. D., Lam V. W.Y., Reygondeau G., Cisneros-Montemayor A. M., Greer K., Palomares M. L. D., et al. (2021). Global decline in capacity of coral reefs to provide ecosystem services. One Earth 4, 1278–1855. doi: 10.1016/j.oneear.2021.08.016
Erler D. V., Wang X. T., Sigman D. M., Scheffers S. R., Shepherd B. O. (2015). Controls on the nitrogen isotopic composition of shallow water corals across a tropical reef flat transect. Coral Reefs 34, 329–385. doi: 10.1007/s00338-014-1215-5
Falkowski P. G., Dubinsky Z., Muscatine L., Porter J. W. (1984). Light and the bioenergetics of a symbiotic coral. BioScience 34, 705–795. doi: 10.2307/1309663
Frankowiak K., Wang X. T., Sigman D. M., Gothmann A. M., Kitahara M. V., Mazur M., et al. (2016). Photosymbiosis and the expansion of shallow-water corals. Sci. Adv. 2, e16011225. doi: 10.1126/sciadv.1601122
Gleason D. F., Harbin L. R., Divine L. M., Matterson K. O. (2018). The role of larval supply and competition in controlling recruitment of the temperate coral oculina arbuscula. J. Exp. Mar. Biol. Ecol. 506, 107–114. doi: 10.1016/j.jembe.2018.06.006
Glibert P. M., Middelburg J. J., McClelland J. W., Zanden M.J. V. (2019). Stable isotope tracers: enriching our perspectives and questions on sources, fates, rates, and pathways of major elements in aquatic systems. Limnology Oceanography 64, 950–815. doi: 10.1002/lno.11087
Goldberg J., Wilkinson C. (2004). Global threats to coral reefs: coral bleaching, global climate change, disease, predator plagues, and invasive species. Status of coral reefs of the world 26, 67–92.
Grover R., Maguer J. F., Allemand D., Ferrier-Pagés C. (2003). Nitrate uptake in the scleractinian coral stylophora pistillata. Limnology Oceanography 48, 2266–2745. doi: 10.4319/lo.2003.48.6.2266
Grover R., Maguer J. F., Allemand D., Ferrier-Pagès C. (2008). Uptake of dissolved free amino acids by the scleractinian coral stylophora pistillata. J. Exp. Biol. 211, 860–655. doi: 10.1242/jeb.012807
Grover R., Maguer J. F., Reynaud-Vaganay S., Ferrier-Pagès C. (2002). Uptake of ammonium by the scleractinian coral stylophora pistillata: effect of feeding, light, and ammonium concentrations. Limnology Oceanography 47, 782–905. doi: 10.4319/lo.2002.47.3.0782
Gustafsson M. S. M., Baird M. E., Ralph P. J. (2013). The interchangeability of autotrophic and heterotrophic nitrogen sources in scleractinian coral symbiotic relationships: A numerical study. Ecol. Model. 250, 183–194. doi: 10.1016/j.ecolmodel.2012.11.003
Hoegh-Guldberg O., Williamson J. (1999). Availability of two forms of dissolved nitrogen to the coral pocillopora damicornis and its symbiotic zooxanthellae. Mar. Biol. 133, 561–570. doi: 10.1007/s002270050496
Houlbrèque F., Ferrier-Pagès C. (2009). Heterotrophy in tropical scleractinian corals. Biol. Rev. 84, 1–175. doi: 10.1111/j.1469-185X.2008.00058.x
Hughes T. P., Kerry J. T., Baird A. H., Connolly S. R., Dietzel A., Eakin C.M., et al. (2018). Global warming transforms coral reef assemblages. Nature 556, 492–496. doi: 10.1038/s41586-018-0041-2
Kolasinski J., Rogers K., Frouin P. (2008). Effects of acidification on carbon and nitrogen stable isotopes of benthic macrofauna from a tropical coral reef. Rapid Commun. Mass Spectrometry 22, 2955–2605. doi: 10.1002/rcm.3694
Kopp C., Pernice M., Domart-Coulon I., Djediat C., Spangenberg J. E., Alexander D. T. L., et al. (2013). Highly dynamic cellular-level response of symbiotic coral to a sudden increase in environmental nitrogen. mBio 4. doi: 10.1128/mbio.00052-13
LaJeunesse T. C., Parkinson J. E., Gabrielson P. W., Jeong H. J., Reimer J. D., Voolstra C. R., et al. (2018). Systematic revision of symbiodiniaceae highlights the antiquity and diversity of coral endosymbionts. Curr. Biol. 28, 2570–25805.e6. doi: 10.1016/j.cub.2018.07.008
Li M., Sheng H.-X., Dai M., Kao S.-J. (2023). Understanding nitrogen dynamics in coral holobionts: comprehensive review of processes, advancements, gaps, and future directions. Front. Mar. Sci. 10 (2023), 1203399. doi: 10.3389/fmars.2023.1203399
Madin J. S., Anderson K. D., Andreasen M. H., Bridge T. C.L., Cairns S. D., Connolly S. R., et al. (2016). The coral trait database, a curated database of trait information for coral species from the global oceans. Sci. Data 3, 160017. doi: 10.1038/sdata.2016.17
Matthews J. L., Sproles A. E., Oakley C. A., Grossman A. R., Weis V. M., Davy S. K. (2016). Menthol-induced bleaching rapidly and effectively provides experimental aposymbiotic sea anemones (Aiptasia sp.) for symbiosis investigations. J. Exp. Biol. 219, 306–105. doi: 10.1242/jeb.128934
Minagawa M., Wada E. (1984). Stepwise enrichment of 15N along food chains: further evidence and the relation between δ15N and animal age. Geochimica Cosmochimica Acta 48, 1135–1405. doi: 10.1016/0016-7037(84)90204-7
O’Neil J. M., Capone D. G. (2008). “Nitrogen cycling in coral reef environments,” in Nitrogen in the Marine Environment (30 Corporate Drive, Suite 400, Burlington, MA 01803, USA; Radarweg 29, PO Box 211, 1000 AE Amsterdam, The Netherlands; 525 B Street, Suite 1900, San Diego, California 92101-4495, USA; 84 Theobald’s Road, London WC1X 8RR, UK: Elsevier), 949–989. doi: 10.1016/B978-0-12-372522-6.00021-9
Pernice M., Meibom A., Heuvel A. V. D., Kopp C., Domart-Coulon I., Hoegh-Guldberg O., et al. (2012). A single-cell view of ammonium assimilation in coral–dinoflagellate symbiosis. ISME J. 6, 1314–1245. doi: 10.1038/ismej.2011.196
Piniak G. A., Lipschultz F. (2004). Effects of nutritional history on nitrogen assimilation in congeneric temperate and tropical scleractinian corals. Mar. Biol. 145, 1085–1965. doi: 10.1007/s00227-004-1410-y
Piniak G. A., Lipschultz F., McClelland J. (2003). Assimilation and partitioning of prey nitrogen within two anthozoans and their endosymbiotic zooxanthellae. Mar. Ecol. Prog. Ser. 262, 125–136. doi: 10.3354/meps262125
Pochon X., Montoya-Burgos J. I., Stadelmann B., Pawlowski J. (2006). Molecular phylogeny, evolutionary rates, and divergence timing of the symbiotic dinoflagellate genus symbiodinium. Mol. Phylogenet. Evol. 38, 20–305. doi: 10.1016/j.ympev.2005.04.028
R Core Team. (2017). “R: A Language and Environment for Statistical Computing.” Vienna, Austria: R Foundation for Statistical Computing. https://www.R-project.org/.
Rädecker N., Pogoreutz C., Voolstra C. R., Wiedenmann J., Wild C. (2015). Nitrogen cycling in corals: the key to understanding holobiont functioning? Trends Microbiol. 23, 490–975. doi: 10.1016/j.tim.2015.03.008
Reddy K. R., DeLaune R. D. (2008). Biogeochemistry of Wetlands: Science and Applications (Boca Raton: CRC Press).
Rivera H. E., Davies S. W. (2021). Symbiosis maintenance in the facultative coral, oculina arbuscula, relies on nitrogen cycling, cell cycle modulation, and immunity. Sci. Rep. 11, 21226. doi: 10.1038/s41598-021-00697-6
Rivera H. E., Tramonte C. A., Samaroo J., Dickerson H., Davies S. W. (2023). Heat challenge elicits stronger physiological and gene expression responses than starvation in symbiotic oculina arbuscula. J. Heredity 114, 312–255. doi: 10.1093/jhered/esac068
Rodrigues L. J., Grottoli A. G. (2007). Energy reserves and metabolism as indicators of coral recovery from bleaching. Limnology Oceanography 52, 1874–1825. doi: 10.4319/lo.2007.52.5.1874
Rosenberg E., Koren O., Reshef L., Efrony R., Zilber-Rosenberg I. (2007). The role of microorganisms in coral health, disease and evolution. Nat. Rev. Microbiol. 5, 355–625. doi: 10.1038/nrmicro1635
Sigman D. M., Casciotti K. L., Andreani M., Barford C., Galanter M., Böhlke J. K. (2001). A bacterial method for the nitrogen isotopic analysis of nitrate in seawater and freshwater. Analytical Chem. 73, 4145–4153. doi: 10.1021/ac010088e
Stanley G. D. (2003). The evolution of modern corals and their early history. Earth-Science Rev. 60, 195–225. doi: 10.1016/S0012-8252(02)00104-6
Stanley G. D., Swart P. K. (1995). Evolution of the coral-zooxanthellae symbiosis during the triassic: A geochemical approach. Paleobiology 21, 179–995. doi: 10.1017/S0094837300013191
Szmant-Froelich A., Pilson M. E. Q. (1984). Effects of feeding frequency and symbiosis with zooxanthellae on nitrogen metabolism and respiration of the coral astrangia danae. Mar. Biol. 81, 153–162. doi: 10.1007/BF00393114
Tanaka Y., Grottoli A. G., Matsui Y., Suzuki A., Sakai K. (2015). Partitioning of nitrogen sources to algal endosymbionts of corals with long-term 15N-labelling and a mixing model. Ecol. Model. 309–310, 163–169. doi: 10.1016/j.ecolmodel.2015.04.017
Tanaka Y., Miyajima T., Koike I., Hayashibara T., Ogawa H. (2006). Translocation and conservation of organic nitrogen within the coral-zooxanthella symbiotic system of acropora pulchra, as demonstrated by dual isotope-labeling techniques. J. Exp. Mar. Biol. Ecol. 336, 110–195. doi: 10.1016/j.jembe.2006.04.011
Tornabene C., Martindale R. C., Wang X. T., Schaller M. F. (2017). Detecting photosymbiosis in fossil scleractinian corals. Sci. Rep. 7, 94655. doi: 10.1038/s41598-017-09008-4
Wang J.-T., Chen Y.-Y., Tew K. S., Meng P.-J., Chen C. A. (2012). Physiological and biochemical performances of menthol-induced aposymbiotic corals. PloS One 7, e464065. doi: 10.1371/journal.pone.0046406
Wang X. T., Sigman D. M., Cohen A. L., Sinclair D. J., Sherrell R. M., Cobb K. M., et al. (2016). Influence of open ocean nitrogen supply on the skeletal δ15N of modern shallow-water scleractinian corals. Earth Planetary Sci. Lett. 8, 125–132. doi: 10.1016/j.epsl.2016.02.032
Wang X. T., Sigman D. M., Cohen A. L., Sinclair D. J., Sherrell R. M., Weigand M. A., et al. (2015). Isotopic composition of skeleton-bound organic nitrogen in reef-building symbiotic corals: A new method and proxy evaluation at Bermuda. Geochimica Cosmochimica Acta 148, 179–190. doi: 10.1016/j.gca.2014.09.017
Weis V. M., Davy S. K., Hoegh-Guldberg O., Rodriguez-Lanetty M., Pringle J. R. (2008). Cell biology in model systems as the key to understanding corals. Trends Ecol. Evol. 23, 369–765. doi: 10.1016/j.tree.2008.03.004
Wiedenmann J., D’Angelo C., Loreto Mardones M., Moore S., Benkwitt C. E., Graham N. A.J., et al. (2023). Reef-building corals farm and feed on their photosynthetic symbionts. Nature 620, 1018–1024. doi: 10.1038/s41586-023-06442-5
Woodhead A. J., Hicks C. C., Norström A. V., Williams G. J., Graham N. A. J. (2019). Coral reef ecosystem services in the anthropocene. Funct. Ecol. 33, 1023–1034. doi: 10.1111/1365-2435.13331
Keywords: coral symbiosis, Oculina arbuscula, nitrogen isotope, coral evolution, climate change
Citation: Donnelly HA, Valadez-Ingersoll M, Lin M, Rivera HE, Tramonte CA, Davies SW and Wang XT (2024) Groundtruthing nitrogen isotopes as a symbiosis proxy using the facultatively symbiotic coral Oculina arbuscula. Front. Mar. Sci. 11:1433382. doi: 10.3389/fmars.2024.1433382
Received: 15 May 2024; Accepted: 07 August 2024;
Published: 29 August 2024.
Edited by:
Wei Jiang, Guangxi University, ChinaReviewed by:
Manuel Aranda, King Abdullah University of Science and Technology, Saudi ArabiaJin-Yu T. Yang, Xiamen University, China
Copyright © 2024 Donnelly, Valadez-Ingersoll, Lin, Rivera, Tramonte, Davies and Wang. This is an open-access article distributed under the terms of the Creative Commons Attribution License (CC BY). The use, distribution or reproduction in other forums is permitted, provided the original author(s) and the copyright owner(s) are credited and that the original publication in this journal is cited, in accordance with accepted academic practice. No use, distribution or reproduction is permitted which does not comply with these terms.
*Correspondence: Xingchen Tony Wang, eGluZ2NoZW4ud2FuZ0BiYy5lZHU=
†These authors have contributed equally to this work and share first authorship