- 1State Key Laboratory of Mariculture Biobreeding and Sustainable Goods, Yellow Sea Fisheries Research Institute, Chinese Academy of Fishery Sciences, Qingdao, Shandong, China
- 2Laboratory for Marine Fisheries Science and Food Production Processes, Qingdao Marine Science and Technology Center, Qingdao, Shandong, China
- 3Rongcheng Chudao Aquaculture Co., Ltd, Rongcheng, China
Farmed aquaculture species play an important role in regulating nutrient cycles in farming systems. Compared with nitrogen and phosphorus, the role of farmed species in the silicon (Si) cycle remains poorly understood. To help reduce this uncertainty, we clarified the sources and sinks of silicate and quantified the Si pools in an aquaculture system in Sanggou Bay (SGB). The results showed that dissolved inorganic nutrient levels were significantly lower during the dry season than during the wet. Dissolved silicate (DSi) is a potential limiting factor for phytoplankton growth during spring, and phosphorus limitation occurs during summer. The budget results indicated that large amounts of nitrogen, phosphate (DIP), and DSi were buried in the sediment or transformed into other forms during both the wet and dry seasons. The nitrogen and DIP cycles were strongly influenced by bivalve excretion and farmed species harvesting; however, these processes had little impact on the Si cycle. Si availability depends on both external inputs and internal recycling. DSi was primarily supplied from the Yellow Sea, with a minor contribution from the river due to river discharge during spring. However, during summer, riverine inflow (accounting for 83% of the total influx) was the major DSi source followed by benthic flux (12%). Biogenic silica (BSi) burial efficiency in the sediment was estimated to be 78% during spring and 23% during summer. The BSi preservation efficiency in bivalves during spring was high (53%), leading to a higher Si retention than in river discharge. Bivalves biodeposition plays an important role in the Si burial process. We suggest that this high retention is essentially controlled by the biodeposition mechanism, which is directly controlled by the exotic suspension feeders. Bivalves have the potential to alter Si retention in the bay by producing large amounts of biodeposits and accelerating the silica cycle, which may lead to more carbon dioxide being absorbed by diatoms.
1 Introduction
Silicate or silicic acid (dissolved silicate (DSi), Si(OH)4) is an essential nutrient for the growth of diatoms, siliceous sponges, silicoflagellates, radiolarians, siliceous rhizarians, and picocyanobacteria in the ocean (DeMaster, 2003; Tréguer and de la Rocha, 2013; Tréguer et al., 2021) and cell cycle (Brzezinski, 1992). As major primary producers, diatoms form the basis of the classical food chain which leads to secondary consumers (suspension feeders, copepods, and ultimately fish) (Cushing, 1989; Ragueneau et al., 2005, 2006). They account for approximately 40% of total marine primary production, with up to 75% of the primary production in coastal regions, and play a critical role in the marine carbon cycle (Kristiansen and Hoell, 2002; Jin et al., 2006; Tréguer et al., 2018). However, diatoms are replaced by other phytoplankton groups such as dinoflagellates when DSi is depleted (Beucher et al., 2004). A silicon (Si) availability shortage in coastal areas frequently reflects ecosystem disequilibrium and the proliferation of harmful algal blooms (Davidson et al., 2014; Glibert and Burford, 2017; Thorel et al., 2017). This phenomenon has been reported in some coastal bays and marginal seas, including in Jiaozhou Bay (Liu et al., 2008), Bohai Sea (Wang et al., 2018), the Bay of Brest (Ragueneau et al., 2005), and Biscay Bay (Loyer et al., 2006).
Filter-feeders can strongly affect nutrient cycling (Ray et al., 2021). Suspension-feeding bivalves alter biogeochemical cycles by consuming particulate particles from the water column, excreting dissolved and particulate nutrients, and capturing nutrients in their tissues and shells (Sma and Baggaley, 1976; Prins et al., 1997; Welsh and Castadelli, 2004; Svenningsen et al., 2012; Ray et al., 2021). Through biodeposition, bivalves also increase organic matter (OM) availability in sediments, which can stimulate sediment microbial processes, leading to higher nutrient recycling rates (Newell et al., 2005; Kellogg et al., 2014; Ray and Fulweiler, 2020). The ecological feedback of bivalve-mediated nutrient recycling includes higher productivity (Prins et al., 1997, Peterson and Heck, 1999, Wall et al., 2008), alteration of phytoplankton biomass and community structure (Porter et al., 2018, 2020), and increased nutrient export (Dame et al., 1984, 1992; Li et al., 2021). Many studies have indicated that filter-feeding bivalves can enhance the nitrogen and phosphorus recycling, however, their impact on Si cycling in aquatic ecosystems has largely remained unexplored (Ray and Fulweiler, 2020; Ray et al., 2021).
The potential impact of bivalves on estuarine Si cycling is important because Si availability can regulate the composition of the phytoplankton community (Turner et al., 1998), primary production (Dugdale and Wilkerson, 1998), and food web structure (Doering et al., 1989; Turner et al., 1998). As filter feeders, bivalves strain large volumes of seawater through their bodies to concentrate food such as phytoplankton, particularly diatoms (Newell and Koch, 2004; Wall et al., 2008). Filter feeders have minor or unknown requirements for Si during their growth period (Ragueneau et al., 2005; Ray et al., 2021). However, evidence on DSi excretion by bivalves is scarce (Ragueneau et al., 2005; Ray and Fulweiler, 2020). As a result, suspension-feeding bivalves could reduce Si availability by egestion onto the sediment surface as feces or pseudo-feces. Hence, bivalves may enhance biogenic silica (BSi) exportation from water to sediment, with Si captured and held in feces and pseudo-feces removed from the system through sediment burial (Ray et al., 2021). Additionally, bivalves may influence sediment Si regeneration as Si-containing biodeposits accumulate and decompose. Previous studies have reported that DSi fluxes from sediments to the water column are higher in aquaculture areas than in adjacent bare sediments (Green et al., 2013; Ray et al., 2021), and that Si recycling is enhanced. A similar phenomenon has been observed for other suspension feeders, including clams (Mercenaria mercenaria: Doering et al., 1987; Tapes philippinarum: Bartoli et al., 2001) and slipper shells (Crepidula fornicata: Chauvaud et al., 2000; Ragueneau et al., 2002a). On larger spatial and temporal scales, Si exportation from coastal ecosystems contributes to Si availability in the ocean and helps control the magnitude of the biological pump and global climate (Tréguer and Pondaven, 2000; Ragueneau et al., 2006). Thus, suspension-feeder bivalves play an important role in coastal Si cycling and may have both local and far-reaching effects.
Aquatic ecosystem productivity depends on the limiting nutrient supply (Li et al., 2021). It is known that a natural or experimental DSi scarcity has detrimental consequences for diatom growth (Riesgo et al., 2021). Once DSi is exhausted, the phytoplankton community changes from being diatom- to dinoflagellate-dominated. Filter feeder growth will also be affected. However, if other processes can provide a DSi supply, diatoms can continue to thrive, increasing the potential for new carbon exportation to the deep ocean and higher trophic levels (Allen et al., 2005). Thus, there is great interest in understanding the impact of aquaculture activity on the Si cycle.
Sanggou Bay (SGB) is a semi-enclosed bay that has been used for aquaculture for more than 60 years (yrs). Currently, the bay is a typical integrated multi-tropic aquaculture (IMTA) system, and > 80% of the area is used for marine culture. In recent decades, the long-line culture of kelp has expanded out of the bay to pursue productivity (Troell et al., 2009; Fu et al., 2013), reaching a depth of over 30 m. Large amounts of inorganic nitrogen and phosphate (DIP) have been excreted into the bay owing to bivalve culture (Li et al., 2016). The dissolved inorganic nitrogen (DIN) concentration increased by one order of magnitude between the 1980s and the 2010s, whereas the DSi concentrations remained almost unchanged (Li et al., 2016; Zhang et al., 2023). Accordingly, the DIN: DIP atomic ratio increased from 2–3 in the 1980s to 40 in the 2010s, and the DSi: DIN molar ratio decreased. Thus, both Si and phosphorus limitations and a nitrogen surplus, have been proposed (Li et al., 2016; Zhang et al., 2023). The preliminary results of the incubation experiments with enriched nutrients showed that phytoplankton growth was limited by Si in SGB during spring (Qu et al., 2008). In this ecosystem, diatoms are still the dominant species in the phytoplankton community but have shown an obvious decline over the past decades (Li et al., 2010). Therefore, a thorough understanding of Si cycling in SGB is critical for understanding the primary factors influencing the phytoplankton community structure. Eventually, it will provide information for effective ecosystem management and sustainable marine farming development.
DSi concentration dynamics in the bay are regulated by the balance between the Si sources and sinks. These include inputs from the watershed and atmosphere, outflow removal, and net burial in sediments. The increase in bivalve biomass and consequent bivalve excretion enhancement has shifted SGB into a new dynamic regime. A key open question is how aquaculture activity affects Si cycling, which may limit productivity in the bay. The aims of this study were to: 1) determine the seasonal variations in major Si sources and sinks by calculating the SGB Si budget, 2) examine the sizes of various Si pools in the IMTA system, 3) evaluate the aquaculture activity effects on phytoplankton communities, and 4) define the role of bivalves in the Si biogeochemical cycles in one of the largest aquaculture farms in China.
2 Materials and methods
2.1 Study area
SGB, located in the eastern part of Shandong Peninsula (northern China) and connected to the Yellow Sea (YS), is a shallow bay (average depth of 7.5 m) and has an approximately area of 144 km2 (Figure 1) (Mao et al., 2006; Jiang et al., 2015; Li et al., 2016). The bay has a semi-diurnal tide regime, with an average tidal range of 2 m (Mao et al., 2006; Jiang et al., 2007). The regional climate is dominated by land-ocean climate (Kuang et al., 1996), with approximately 73% of annual precipitation in wet season (June–September) and 27% in dry season (from October to May). Rivers empty into SGB with annual total discharge 1.7–2.3 × 108 m3 yr–1 (China Gulf Chronicles Compilation Committee, 1991). The freshwater discharge is highly dependent on the monsoonal rainfall and varies from 0–0.95 m3 s–1 in dry season to 0–74.1 m3 s–1 in wet season (http://www.whsw.info.). The annual primary production of the bay was estimated to be 5.48 mol C m–2 yr–1 (Jiang et al., 2015). The productive period typically extends from early May to late September.
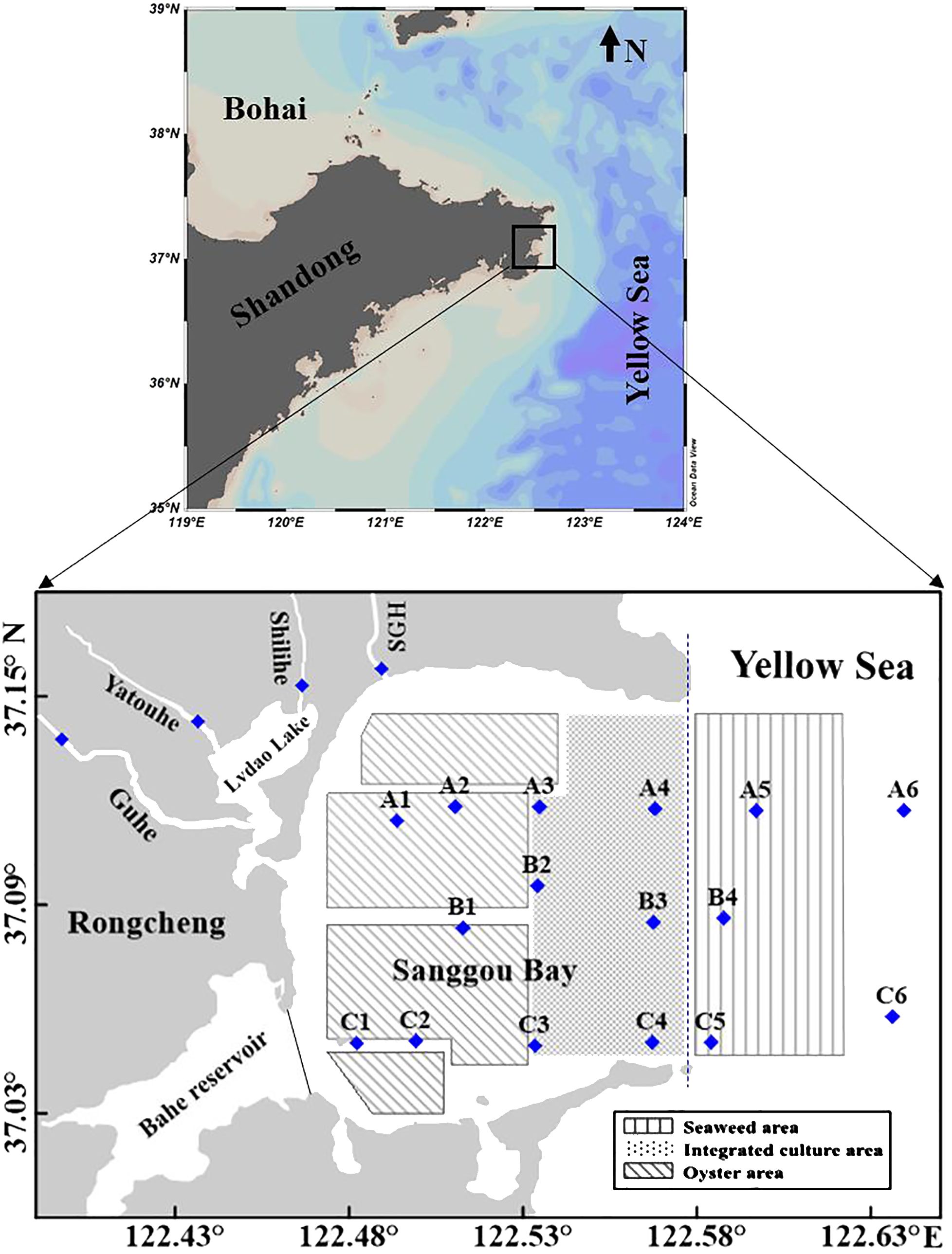
Figure 1. Map of sampling stations in Sanggou Bay, Shandong Province, China, of the cruises August 2022 (wet season) and April 2023 (dry season). (♦) investigation stations (SGH, Sanggouhe). The blue dashed line shows the boundary of the budget system. The black line represents the dam between the Bahe reservoir and the bay.
As a typical IMTA system in China, more than 30 species are farmed using different farming methods. The suspension-feeding bivalves (scallop and Pacific oyster), Saccharina japonica (S. japonica), and Gracilaria lemaneiformis (G. lemaneiformis) are the primary species cultured (Zhang et al., 2009). The decrease in reared scallops caused great damage during the 1990s, leading to the replacement of large areas of scallop farming with oyster farming since 1996 (Zhang et al., 2009) (Figure 1). Currently, S. japonica monoculture is primarily farmed at the mouth of the bay, kelp and bivalve polyculture occurs in the central region of the bay, oysters are primarily cultured in the end western region of the bay, and a small area within polyculture area in the northern region is used for scallop farming (Figure 1). There are many large-scale aquaculture facilities in the bay (e.g. rafts and suspension facility). Consequently, water flow rates were affected (Shi et al., 2011a). Compared to the 40 years prior to extensive farming, tidal currents and water exchange rates were significantly reduced (Zhao et al., 1996; Grant and Bacher, 2001; Fan and Wei, 2010). The resuspension of particulate matter and nutrient recycling have also been reduced (Grant and Bacher, 2001; Duarte et al., 2003).
2.2 Sample collection and analytical methods
Field observations were carried out during 16–20 August 2022 (wet season) and 7–10 April 2023 (dry season) (Figure 1). Sampling was performed at 1.0 m depth during high tide, in mean tidal conditions. At each station, water samples were collected using a 5-L polymethyl methacrylate water sampler from a boat and transferred to 2.5 L acid-cleaned polyethylene bottles, and kept cool and shade during transporting to the laboratory. River water samples were collected from the river edge in 2.5 L acid-cleaned polyethylene bottles.
Water temperature and salinity were measured in situ using a WTW MultiLine F/Set3 multi-parameter probe. Each water sample was immediately filtered through a preweighted 0.45 µm pore size cellulose acetate filters (pre-cleaned with hydrochloric acid, pH = 2) into a clean polyethylene bottle and the filtrates were stored at –20°C until they were analyzed. The filtrate was used to measure NO3–, NO2–, NH4+, DIP, and DSi. Dissolved nutrient concentrations were measured in the laboratory using an Auto Analyzer 3 (Seal Analytical), following the methods of Grasshoff et al. (1999). The DIN concentration is the sum of NO3–, NO2–, and NH4+ concentrations. The detection limit was 0.10 μM for NO3–, NO2–, NH4+, DSi, and 0.01 μM for DIP. The analytical precision for NO3–, NO2–, NH4+, DIP, and DSi was < 5%.
Filters were used to determine the suspended particulate matter (SPM). The filters were stored at –20°C until they were analyzed. The filters were dried at 45 °C and weighed to determine the mass of SPM. Samples were immediately filtered with 0.4 µm polycarbonate membrane filters. The filters were then used for BSi analysis. The BSi in the SPM (particulate BSi, PBSi) was analyzed according to the method described by Ragueneau et al. (2005). The filter was submitted to a first digestion in 0.2 M NaOH at 100°C for 40 min, then neutralized with hydrochloric acid (1.0 M). At the end of the first leaching, the DSi ([Si]1) in the leaching solution was analyzed via the molybdate-blue method using a spectrophotometry. Dissolved aluminum (Al) was also analyzed for mineral correction (Kamatani and Takano, 1984). The Al ([Al]1) in the leaching solution was analyzed using a high-resolution inductively coupled plasma mass spectrometer. After rinsing and drying, the filter was subjected to a second digestion step identical to the first step to determine the (Si: Al)2 ratio characteristics of the lithogenic particles present on the filter. This ratio was then used to correct for the Si concentration in BSi form as follows: [BSi] = [Si]1 - [Al]1 × (Si: Al)2. The analytical precision of PBSi was < 10%.
Water sample (500 ml) was filtered through 0.45 μm pore size cellulose acetate membrane for total chlorophyll a (Chl a). Another 500 ml seawater for size-fractionated Chl a was filtered through 20 μm bolting-silk, 2 μm, and 0.45 μm cellulose acetate membrane, in that order. The filters were stored at –20°C until they were analyzed. The Chl a extracted in 10 ml of 90% (v/v) acetone in the dark at 4°C for 14–24 h, and was then measured before and after acidification with 1 μM hydrochloric acid using a Turner designs Trilogy laboratory fluorometer (Parsons et al., 1984).
The samples of 500 ml water for phytoplankton species identification and counting were fixed on board with Lugol’s solution (5%). The fixed water sample volume was finally concentrated to 20 ml by sedimentation in the lab. Then, the concentrated samples (0.3 ml) were identified and counted by microscopic examination using an inverted microscope (Motic AE2000) at magnifications of 100 × or 400 × according to the method of Utermöhl (1958). Phytoplankton abundance was expressed as cell numbers per liter (cells L–1).
2.3 Nutrient budgets
The Land-Ocean Interactions in the Coastal Zone (LOICZ) box model was used to estimate nutrient budgets for the study system (Gordon et al., 1996). This model has been widely used to construct nutrient budgets in estuarine and coastal ecosystems to identify the internal biogeochemical processes and external nutrient inputs of a system study (Savchuk, 2005; Liu et al., 2008; Li et al., 2016). Detailed descriptions of the budgets are provided in the supplementary data (Supplementary Data Sheet 1).
2.4 Statistical analysis
Statistical analyses were performed using the SPSS 20.0 software for IBM. One-way ANOVAs were used to analyze the individual effects of seasons on nutrient variations, and two-way ANOVAs were used to analyze the combined effects of seasons and cultivation areas on nutrient variations. Based on a posteriori homogeneity test, Tukey’s HSD or Tamhane’s T2 comparisons were applied to assess the statistical significance of the differences (p < 0.05), following ANOVA.
3 Result
3.1 Hydrographic properties
The average monthly precipitation collected from 33 long-term observation sites in Rongcheng City ranged from 1.0 to 259 mm during January 2022−May 2023. The monthly precipitation varied markedly (Figure 2). The total precipitation during the wet season in 2022 was approximately 2.2 times higher than that during the dry season (October 2022–May 2023) (Figure 2). The total precipitation in August 2022 was approximately 2.9 times higher than that in April 2023 (Table 1, Figure 2). Rainfall during the wet season of 2022 was approximately 30% higher than that during a normal year (~640 mm). In April 2023, rainfall (~89 mm) was much higher than that in a normal year (~45 mm) owing to heavy rainfall (~70 mm), that occurred on April 5, 2023. Changes in rainfall influence freshwater discharge and nutrient inputs to the bay.
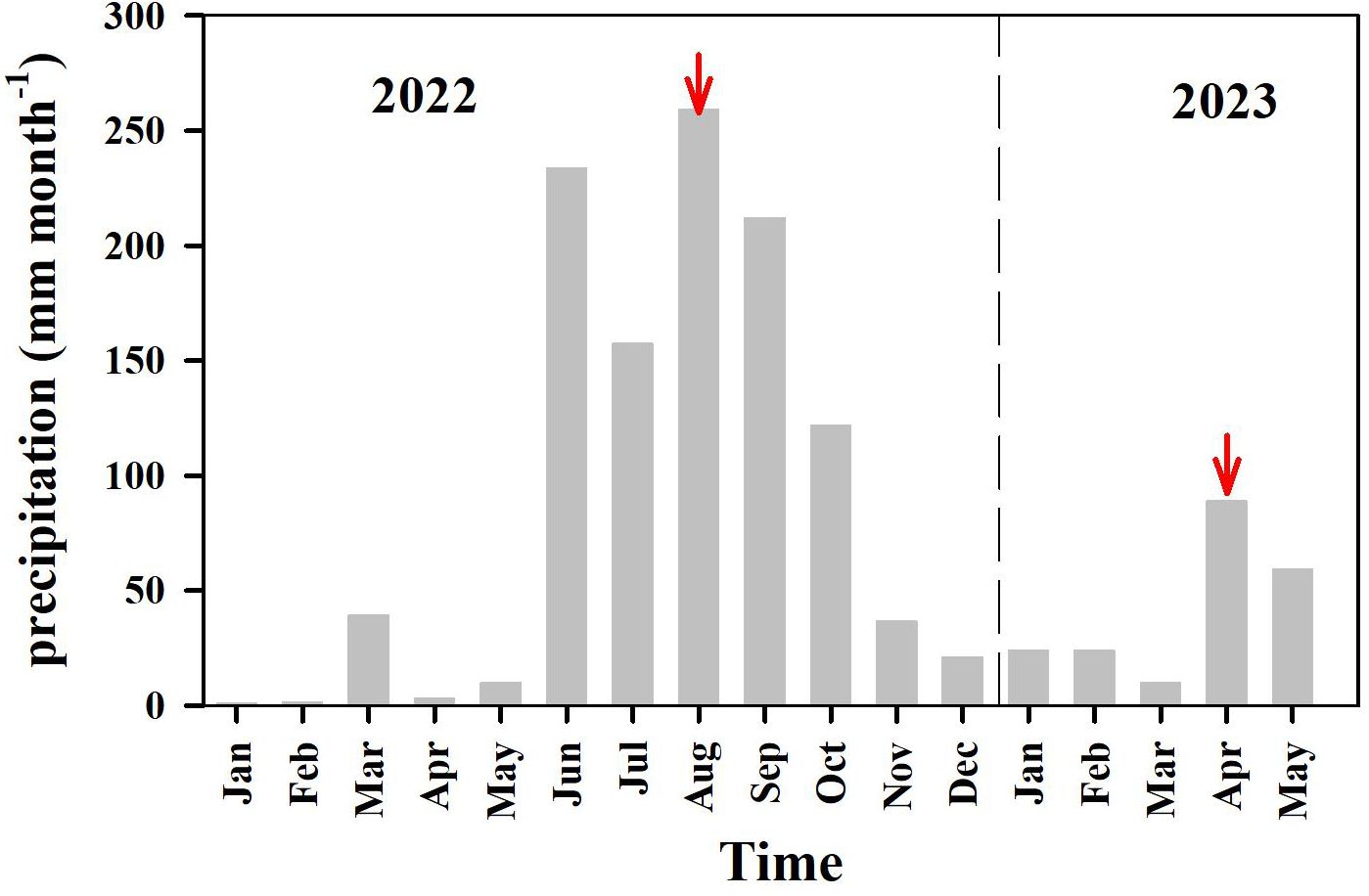
Figure 2. Monthly precipitation during 2022−2023 in Rongcheng city. Precipitation data were obtained from http://www.whsw.info. The red arrows pointed to the sampling month.

Table 1. Temperature, salinity, SPM, and precipitation in Sanggou Bay during the investigation periods.
The water properties, specifically the salinity in SGB, are largely determined by the seasonal freshwater discharge from surrounding rivers during the wet season and seawater during the dry season, owing to seasonal exchange between the southwesterly and northeasterly monsoons. Both the temperature and salinity in the surface water (Table 1) exhibited seasonal variability in this temperate system. The water temperature decreased from the mouth to the west of the bay during both summer and spring (Figure 3). During the study period the water temperature in SGB ranged from 9.8°C during spring to 24°C during summer. Salinity in August 2022 and April 2023 gradually increased from west of the bay to the mouth (Figure 3). Salinity varied greatly in the bay, which was 30.1−30.9 in April 2023 and 22.4−29.4 in August 2022 (Table 1, Figure 3). Salinity changed with freshwater discharge and tidal levels.
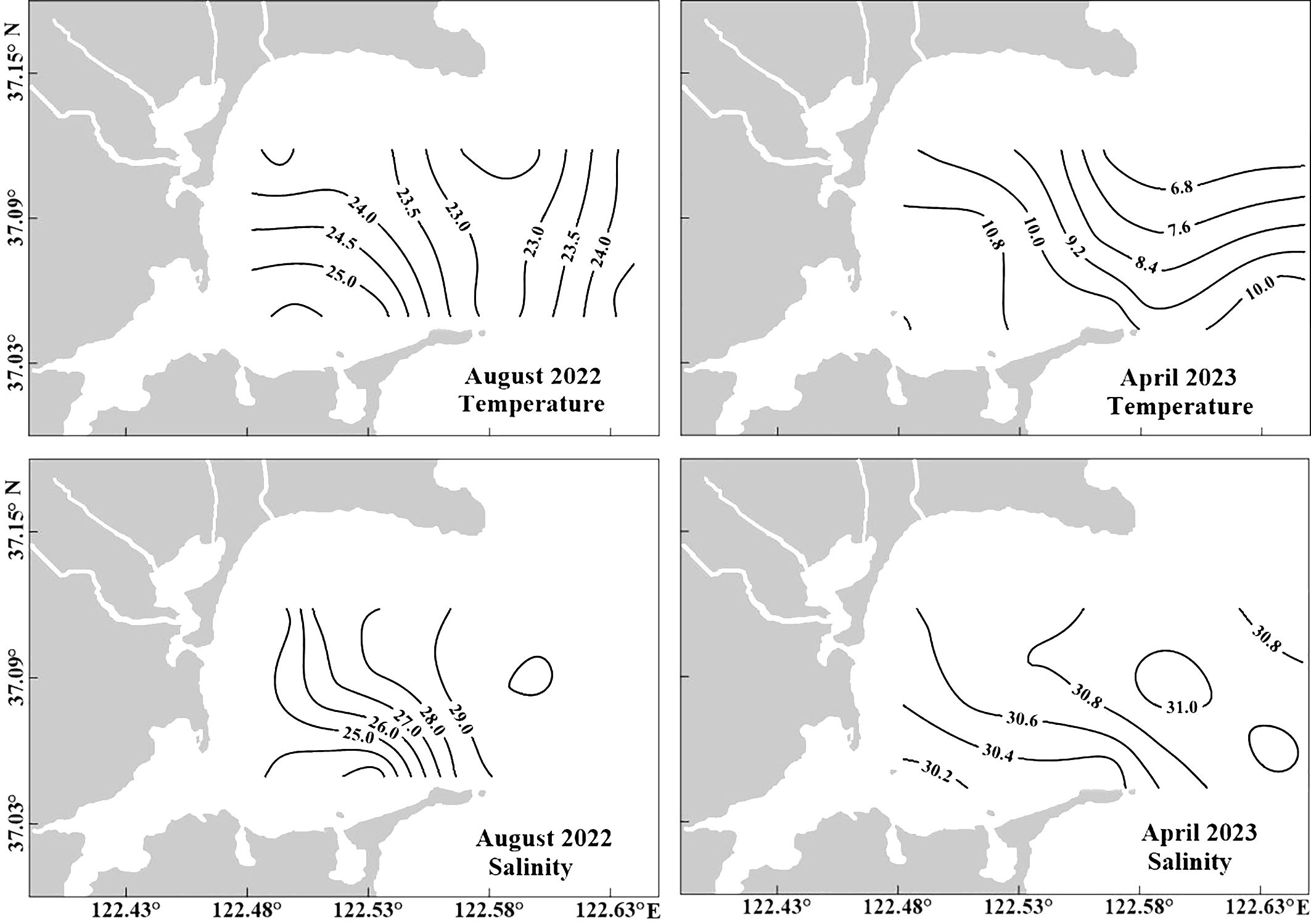
Figure 3. Horizontal distribution of temperature (°C) and salinity at the surface in SGB in August 2022 and April 2023.
The SPM concentrations in the bay also showed clear seasonal variations, reaching 94.1 mg L–1 in April 2023 because of the large amount of precipitation that occurred during this period (Table 1). The SPM levels in offshore water in April 2023 (97.8 ± 10.5 mg L–1) were significantly higher than those in August 2022 (3.83 ± 0.32 mg L–1). This substantial difference may be related to water mixing such as water stratification during summer. One-way ANOVA indicated significant (p < 0.05) differences in SPM concentrations between the offshore area and the bay, particularly during spring.
3.2 DIN and DIP spatiotemporal variations
Spatiotemporal variability in the DIN and DIP concentrations was evident in the bay (Figure 4). The DIN and DIP concentrations gradually increased from offshore to the inner region of the bay (Figure 4). Regarding nitrogen compounds, NO3− comprised 67.6−90.9% of DIN in the surface water. The surface water was depleted in DIP (0.02−0.49 μM), which led to the DIN: DIP ratios (average 207 ± 244) being significantly higher than the Redfield ratio (16:1). Previous studies on nutrient uptake kinetics have shown that the threshold values for phytoplankton growth are 1.0 µM DIN and 0.1 µM DIP (Justić et al., 1995). The DIP concentrations in the surface layer at 38% of the stations were lower than the threshold values, suggesting that phytoplankton growth may be limited by phosphorus during summer.
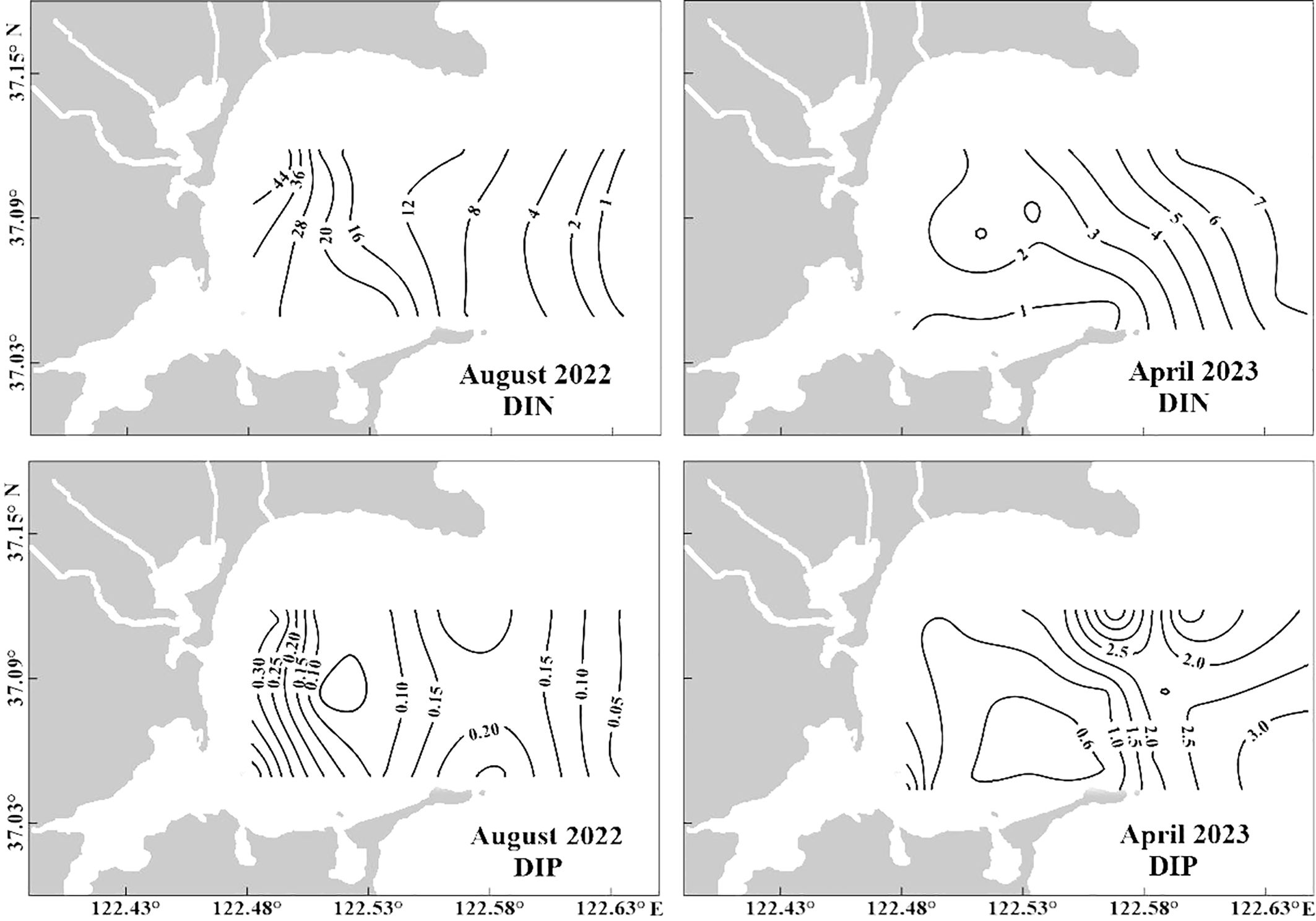
Figure 4. Horizontal distributions of DIN (μM) and DIP (μM) in August 2022 and April 2023 in Sanggou Bay. DIN, dissolved inorganic nitrogen; DIP, dissolved inorganic phosphorus.
The DIN and DIP concentrations declined gradually from the offshore to the inner region of SGB during spring (Figure 4), showing an opposite horizontal distribution compared with that during summer. The DIN levels in April 2023 were lower than those during summer. The DIN: DIP molar ratios ranged from 0.5 to 5.2 (average 2.6 ± 1.7). The average DIN: DIP ratio in the surface water was significantly lower than that of the Redfield ratio. The DIN concentrations were lower than or comparable to the threshold values in the southern region of the bay (section C) (Figures 1, 4). This suggests that nitrogen may be a potential limiting element for phytoplankton growth in this region of the bay.
3.3 Biologically available Si and nutrient ratios
The DSi concentrations varied considerably among the different streams by a factor of 2–4 between the dry and wet seasons. DSi generally increased from the inner region of the bay to offshore during the dry season and decreased during the wet season (Figure 5). The DSi distribution characteristics in the surface water were similar to those of the DIN. The DSi levels were higher in the northwest than in the southwest in August 2022 (Figures 4, 5) because of rainstorms (approximately 150 mm prior to investigation), which led to a high freshwater discharge emptying into the bay. The DSi concentration in the streams during summer was 9−40-fold higher than that in the surface water of SGB. The mean DSi concentrations in surface water during summer (16.4 ± 10.1 μM) were higher than those during spring (4.31 ± 2.00 μM) (Figure 5). From the dry season to the wet season, a significant increase was observed (p < 0.05), coinciding with the maximum riverine impact (minimum salinity) in the bay. Additionally, the DSi distribution characteristics in the surface water were similar to those of Chl a (Figures 5, 6). The DSi: DIN molar ratios in SGB ranged from 0.45 to 1.43 with an average of 0.84 during summer, whereas the DSi and DIN concentrations were higher than the threshold values. The DSi: DIN ratios varied between 0.65 and 3.50 with an average 2.04 in April 2023. In addition, the DSi concentrations were lower than or comparable to the threshold values in Section C (Figures 1, 5). Moreover, the contribution of the 0.45−2 μm fraction phytoplankton was higher than that in the other sections. This suggests that DSi may be the most limiting element for phytoplankton growth in this bay region.
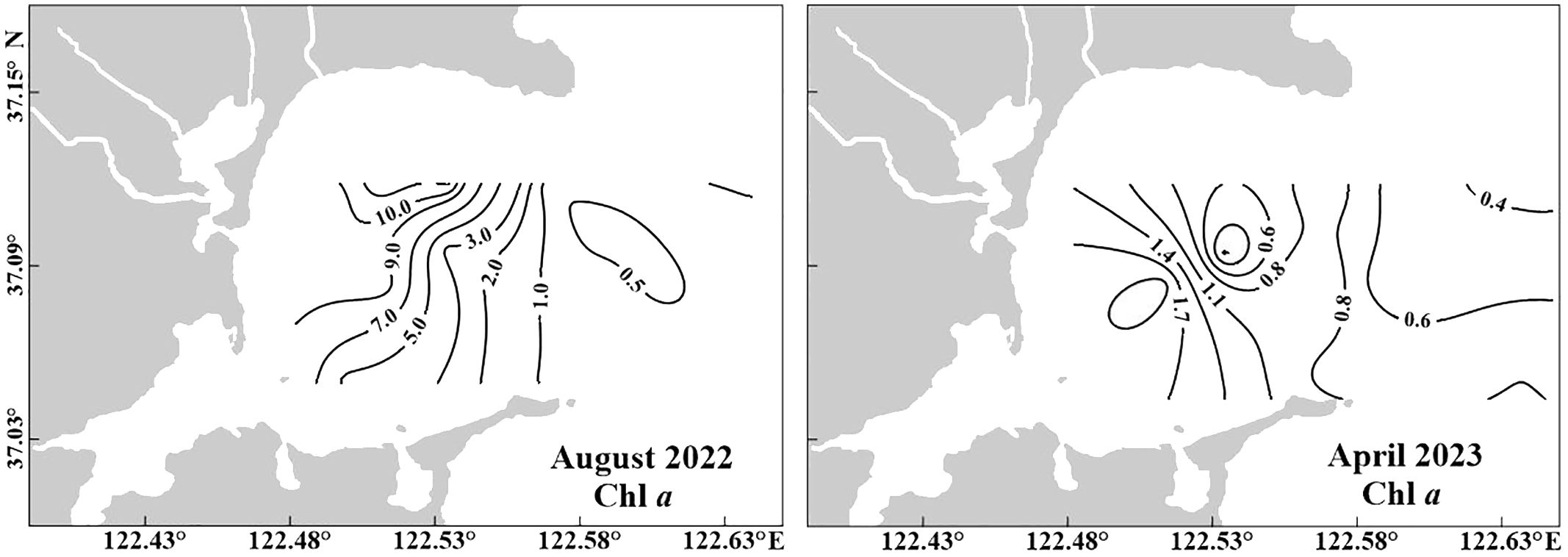
Figure 6. Horizontal distribution of chlorophyll a concentration (Chl a, μg L−1) at the surface water in August 2022 and April 2023.
The average PBSi concentrations in the major SGB rivers were 48.5 μM during spring and 23.8 μM during summer. The horizontal PBSi distributions during the wet and dry seasons showed the opposite tendency, whereas the spatial distributions were the same as those of the DSi (Figure 5). The PBSi concentrations ranged from 0.43 to 6.90 μM with a mean value of 3.42 ± 2.35 μM in April 2023. In August 2022, the average PBSi concentration was 2.33 ± 1.50 μM. The PBSi concentrations were high in the northern region and central of the mouth (Figure 5). The average PBSi concentration during the dry spring was slightly higher than that during the wet season. This was due to DSi fixation by diatoms and SPM concentrations in the water column. Our results showed a significantly positive correlation between PBSi and SPM (R2 = 0.63; p < 0.01) during spring. The PBSi concentrations during summer were significantly correlation with Chl a (R2 = 0.55; p < 0.01). The average cell abundance of phytoplankton during spring (31.6 × 103 cell L–1) was comparable to that during summer (36.3 × 103 cell L–1). However, the proportion of diatoms in the total phytoplankton abundance was 99% during spring and 70% during summer. The results for the wet season indicated that PBSi was primarily affected by primary production, which was dominated by diatoms. The PBSi concentration was poorly correlated with the total Chl a concentration during the dry season, suggesting that diatoms, although dominant, were not the only contributors to PBSi. Sediment resuspension may be the primary reason the PBSi concentration during the dry season exceeded that during the wet season.
Seasonality in nutrient concentrations was evident in SGB (Figures 4, 7). The average DIN (20.6 ± 12.9 μM) and DSi concentrations during summer exceeded those during spring by factors of 7.6 and 3.8, respectively. The subsequent post hoc Tukey’s HSD test showed that the NO3−, NO2−, NH4+, and DSi concentrations were higher during the flood season than during the dry season (p < 0.05) (Figure 7). The average NH4+ concentrations during summer exceeded that during spring by a factor of 4.1. This may be related to shellfish metabolism. Two-way ANOVA indicated highly significant differences in DIN concentrations between seasons and areas (Figure 8, p < 0.01), particularly during the wet season, suggesting that aquaculture activity and freshwater discharge affect the nutrient composition in SGB.
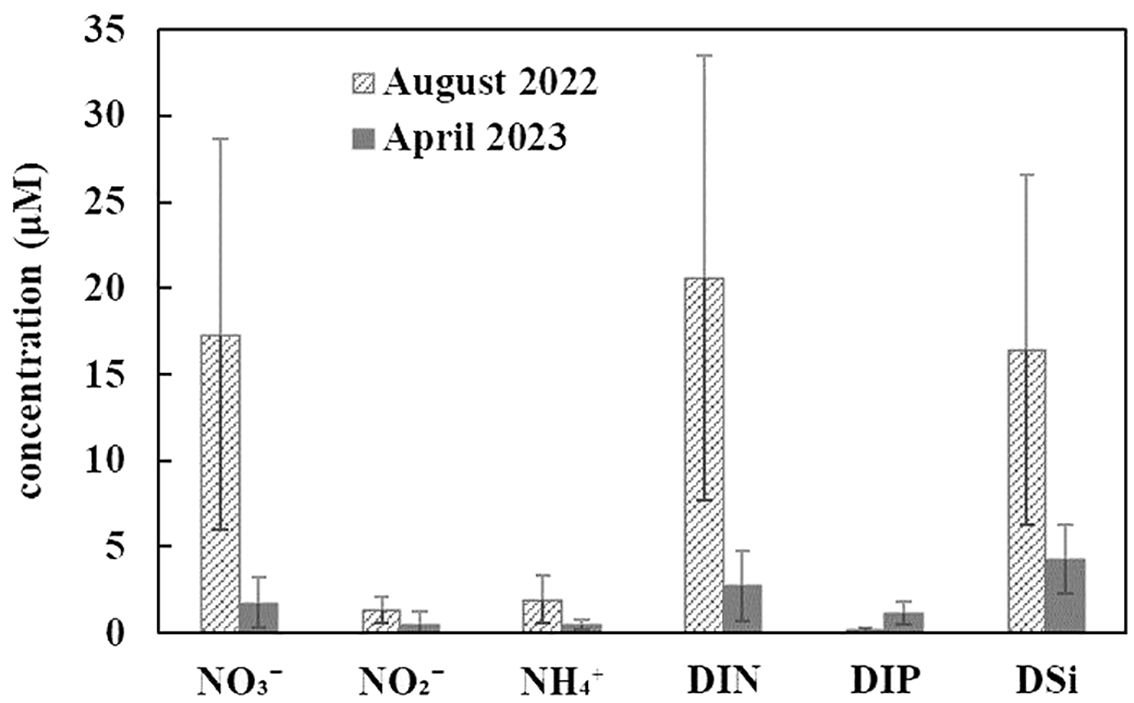
Figure 7. Seasonal variations in nutrient concentrations (μM) in Sanggou Bay during the study period. DIN, dissolved inorganic nitrogen; DIP, dissolved inorganic phosphorus; DSi, dissolved silicate.
3.4 Chl a and phytoplankton community structural dynamics
The Chl a concentration in SGB declined gradually from the inner region to offshore, during both spring and summer (Figure 6). The Chl a concentration varied considerably among seasons. In April 2023, the average Chl a concentration was 1.2 ± 0.6 μg L–1, and a peak occurred at Station B1 (~2.1 μg L–1). The observed Chl a concentrations (5.7 ± 4.4 μg L–1) were significantly higher in August 2022 than in April 2023, and a maximum (~11.6 μg L–1) occurred at Station A2. The Chl a concentration was significantly (p < 0.05) lower in coastal regions (0.8 ± 0.3 μg L–1) in non-farming areas than in the bay during summer. However, no significant differences were observed between the two regions during spring.
The phytoplankton community structure showed seasonal variation based on size-fractionated Chl a concentration (Figure 9). In April, the 2−20 μm fraction contributed 45.9 ± 18.9% to the total Chl a concentration, micro- (43.7 ± 17.5%) and nano-phytoplankton reached similar proportions. The proportion of the 20−200 μm fraction increased to a maximum of 66 ± 21% in the surface layer in August. The contributions of the 2−20 μm fraction in the offshore region were 77.0 ± 14.6% and 86.0 ± 1.40% in April and August, respectively. This indicates that bivalve farming profoundly influenced the phytoplankton size structure, which reduced the nano-Chl a contribution. Surface water in the bay had an average phytoplankton abundance of 3.68 × 104 cells L–1 in August and 3.16 × 104 cells L–1 in April. In April, only two diatom species had dominance index higher than 0.02 in SGB. In August, two diatoms and one dinoflagellate species had a dominance index higher than 0.02 in the bay.
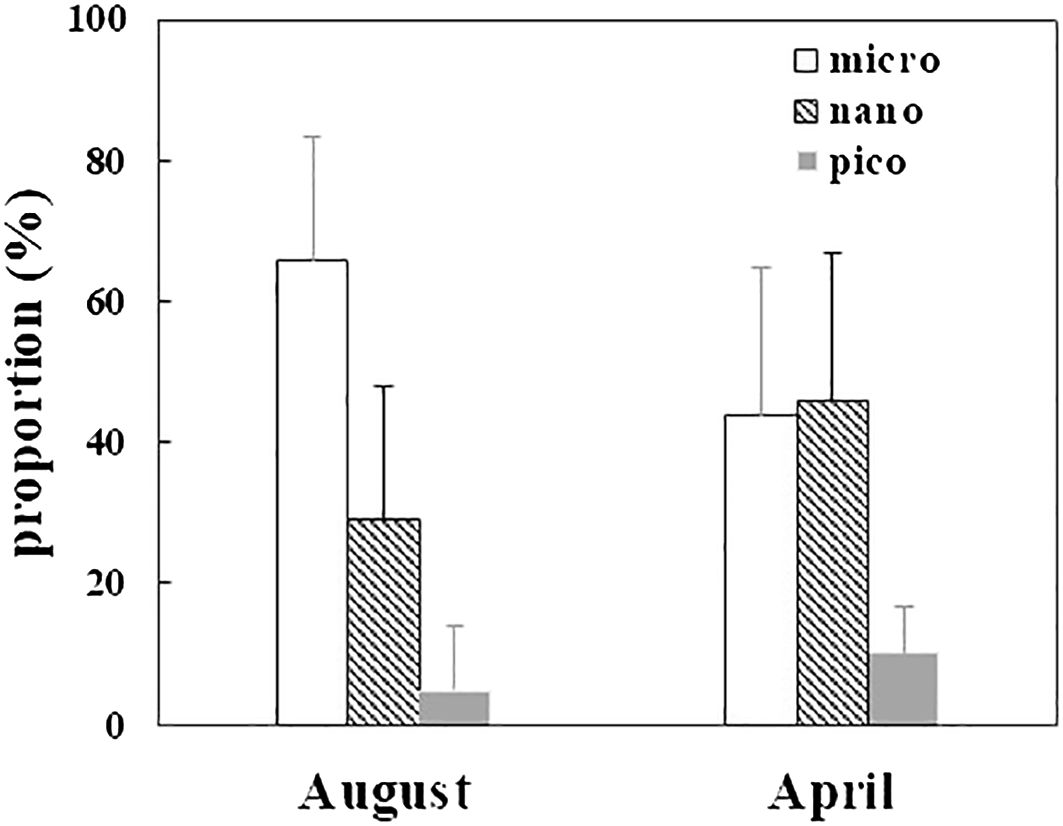
Figure 9. Temporal variations of the proportion of size-fractionated Chl a concentration (%) at surface water of Sanggou Bay in August 2022 and in April 2023.
3.5 Water and nutrient budgets in the bay
Li et al. (2016) described the annual balance of dissolved nutrient budgets. In this study, we describe the nutrient budgets during the wet and dry seasons to show the controlling factors in different seasons under the influence of aquaculture activity and Si budgets for SGB. Sewage discharge was included in the river discharge, as previously reported by Li et al. (2016). Freshwater discharge data emptying into SGB are limited. The Guhe (GH) is the largest of the three rivers that empty into SGB through Lake Lvdao (Figure 1). Hence, the average GH discharge (VQ) during summer in 2022 and spring in 2023 was used to estimate the water budget (Figure 6). The total river discharge into the study system was nine times higher during summer than during spring (Figure 10). The submarine groundwater discharge (SGD) was estimated from a previous study by Wang et al. (2014) which calculated the SGD as (2.59−3.07) × 107 m3 d–1 based on the naturally occurring 228Ra isotope in June 2012. A total of 81.8% of the annual rainfall occurred during the wet season. Thus, based on the assumption that recirculated seawater could account for 90% (Beck et al., 2008) of total SGD in SGB, the SGD was estimated as (2.59−3.07) × 106 m3 d–1 in August and 0.63 × 106 m3 d–1 in April.
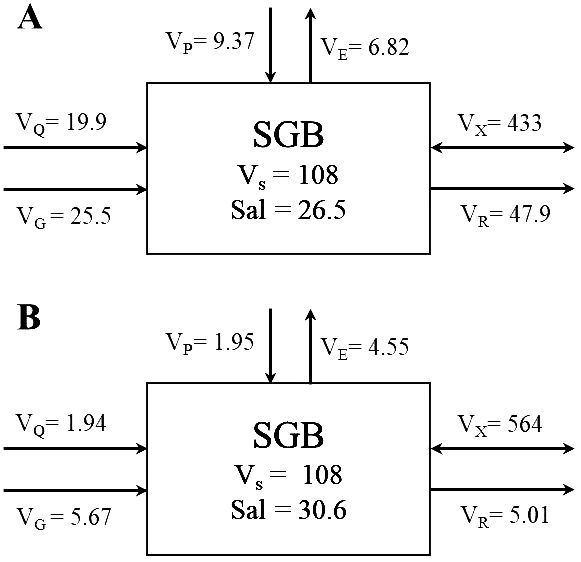
Figure 10. Water and salt budgets for Sanggou Bay (SGB). The numbers (A, B) refer to spring and spring, respectively. Units: water valume, 107 m3; water and salt fluxes, 107 m3 and 107 psu m3 d−1, respectively. VQ, VP, VE, VG, VS, VR, and VX are the mean flow rate of river water, precipitation, evaporation, groundwater, the volume of the system of interest, the residual flow, and the mixing flow between the system of interest and the adjacent system, respectively. Adjacent system: Socn = 29.6 (August) and 30.8 (April).
Nutrient fluxes from groundwater and benthic sediments in SGB were determined from surveys undertaken during the period 2012−2014 (Wen, 2013; Ning et al., 2016). Data on atmospheric nutrient deposition are limited. Thus, the summer wet deposition fluxes were based on measurements on Qianliyan Island, which is located in the western YS (Han et al., 2013) and precipitation at Chudao during summer (Table 1). Rainwater samples were collected during the dry season at Chudao, a site close to SGB, from March 1 to May 10, 2023. Calculations of dry deposition fluxes were based on atmospheric aerosol measurements around Yangma Island in the northern YS (Xie et al., 2021). Thus, the total SGB atmospheric deposition was estimated as the sum of the wet and dry deposition.
Oysters (C. gigas) and scallops (C. farreri) were the primary suspension bivalves cultured in SGB. Oyster and scallop cultivation involved approximately 1.08 × 109 and 4.47 × 108 individuals, respectively, in the bay (Li, 2019). Based on excretion rates determined in a previous study (Zhou et al., 2002a) for bivalves in Sishili Bay (China), the total DIN and DIP excreted by C. gigas and C. farreri in SGB amounted to 0.13 × 106 mol d–1 and 0.87 × 104 mol d–1, respectively. However, evidence regarding DSi excretion by bivalves is limited (Ray and Fulweiler, 2020). Therefore, the DSi excreted by scallops and oysters was not included in the budget. The nutrients were removed from the bay when the bivalves were harvested. The dry weights of soft tissues and shells for individual C. gigas at harvest were 1.43 g and 70.75 g, and 1.63 g and 12.65 g for C. farreri, respectively. Annually, 80000 t of C. gigas (total dry weight) and 10000 t of C. farreri (total dry weight) are harvested from the bay. The dry weight nitrogen and phosphorus contents of the C. gigas soft tissue were 8.19 and 0.379%, respectively, and in the shell were 0.12 and 62.1 × 10−4% (Zhou et al., 2002b), respectively. The nitrogen content (dry weight) of the C. farreri soft tissue and shells was 12.36% (Zhou et al., 2002b) and 0.14% (Ren, 2014), respectively, and the phosphorus content was 0.839% (Zhou et al., 2002b) and 0.026% (Ren, 2014), respectively. Bivalves are always harvested during summer. Thus, 402 t of nitrogen and 24.9 t of phosphorus were removed from the bay as a consequence of C. gigas and C. farreri harvesting during the wet season (Table 2). A previous study showed that the Si contents of the dried soft tissue and shells of C. gigas and C. farreri were both < 0.01% at harvest (Niu, 2014). Si held in market-size C. gigas and C. farreri was minimal compared to bivalve-mediated enhancements in sediment Si cycling. Therefore, the C. gigas and C. farreri Si content was not included in the budget.
S. japonica and G. lemaneiformis are the primary seaweeds cultivated in SGB. Currently, S. japonica is primarily cultivated near the mouth of the bay (Figure 1). Approximately, one-fourth of the S. japonica cultivation area is located in the bay. Dried kelp (80000 t) is produced annually in the bay (Mao et al., 2018). Thus, one-fourth of the nutrients removed through kelp harvesting were included in the budget. Based on dry weight nitrogen and phosphorus contents determined in a previous study (Zhou et al., 2002b) for S. japonica, the total nitrogen and phosphorus removed by S. japonica amounted to 326 t and 76 t as a consequence of harvesting during spring (Table 2). Similarly, 25410 t (wet weight) of G. lemaneiformis is produced annually in the bay (data from Rongcheng Fishery Technology Extension Station, 2012). The dry to wet weight ratio of G. lemaneiformis is approximately 1:7 (Jiang et al., 2010). The G. lemaneiformis individual dry weight nitrogen and phosphorus contents were 5.55 and 0.10% (Zhou et al., 2002b), respectively. Hence, G. lemaneiformis harvesting removed 201 t of nitrogen and 3.68 t of phosphorus from the bay during summer (Table 2).
Nutrient budget results showed that SGB behaved as a DIN, DIP, and DSi sink during both spring and summer (Table 2), as large quantities of nutrients were buried in the bay. The results indicate that the DIP load was primarily derived from the YS, which accounted for 82% of the total influx during spring, followed by bivalve excretion (11%). The DIP flux to SGB via rivers was limited during spring (accounting for only 1% of the total influx). During summer, bivalve excretion was the major source of DIP entering SGB, accounting for 58% of the total influx (Table 2). Riverine flux accounted for 26% of the total influx. Bivalve excretion plays an important role in phosphorus cycling in the bay, particularly under phosphorus limitation. In summer, groundwater (42%) was the major source of DIN entering SGB, whereas benthic flux (28%) and groundwater (28%) were the major sources during spring. Bivalve excretion accounted for 24% of the total DIN influx during spring and 7.70% during summer. Riverine discharge accounted for 23% and 11% of the total DIN influx into SGB during summer and spring, respectively. The riverine DIN input was estimated from the products of freshwater discharge with DIN concentrations. Given that rivers are characterized by seasonal freshwater discharge, there are substantial differences in seasonal DIN input estimates (Table 2). DIN and DIP were primarily removed by kelp harvesting during spring, which accounted for up to 99% and 97% of the total outflux, respectively. DIP was primarily removed through bivalve harvesting during summer, which accounted for 65% of the total outflux. Bivalve and G. lemaneiformis harvesting resulted in 33% and 17% of the DIN being removed from the bay, respectively. The results showed that aquaculture activity plays an important role in DIP and DIN cycling in SGB.
The model results indicated that DSi was primarily derived from riverine input during summer, which contributed 83% of the total input, followed by benthic flux (12%) (Table 2). Riverine DSi input during spring contributed 8.2% of the total influx (Table 2). The DSi flux in the rivers varied between the dry and flood seasons by a factor of up to 31. The DSi load in SGB originated primarily from the YS and benthic flux during spring, accounting for 72 and 17% of the total influx, respectively. Atmospheric deposition as a source of DSi was very limited during both dry and wet seasons.
4 Discussion
4.1 Nutrient budgets and their controlling factors
A box model was used to provide an overview of the nutrient cycles under the influence of human activity and physical processes during different seasons. The budget results indicated that large quantities of DIN, DIP, and DSi would probably be buried in the sediments or transformed into other forms (phytoplankton cellular structure and shellfish tissue) during both wet and dry seasons (Table 2). Large amounts of dissolved nutrients can be utilized by seaweeds in the water column and removed from the system when they are harvested (Schneider et al., 2005). Moreover, large amounts of DIN and DIP could be used by bivalves through phytoplankton and were removed from the bay through harvesting (Table 2), demonstrating that cultured species are a significant sink for nitrogen and phosphorus in SGB.
Nutrient fluxes from SGB to the YS were estimated as the sum of the net residual (VRCR) and the mixing fluxes (VXCX) based on the budget (Tabel 2). The export flux of DIN during summer was 1.2 times that of the riverine input (Table 2), suggesting that additional DIN (regeneration, aquaculture effluents, local sewage) may magnify the riverine flux. The riverine DIP and DSi fluxes exceeded the export fluxes to the YS during summer. This result suggests that processes (burial in the sediment or biological utilization) in SGB may reduce the riverine flux. During the dry season, riverine nutrient fluxes were significantly lower than those during the wet season (Table 2) because of low freshwater discharge. River flow measured during summer was exceptionally high (0−74.1 m3 s–1) and declined dramatically (0−0.95 m3 s–1) during spring. River nutrient fluxes significantly decreased, particularly DSi (Table 2). This may have resulted in lower DIN and DSi levels during spring than during summer.
Primary production in the YS was estimated as 794 mg C m–2 d–1 (Yang et al., 1999). The bay DIN input accounted for approximately 0.1% of the total nitrogen influx to the YS during summer. The bay contribution to primary production in the YS is limited. However, the total DIN: DIP and DSi: DIN influx ratios during summer were approximately 93 and 0.5, respectively, and the corresponding flux ratios in the output water to the YS were approximately 136 and 0.3, respectively. These ratios deviate significantly from the Redfield ratio, demonstrating that the YS coastal zone ecosystem may have been affected by nutrient transportation during the wet season.
As typically observed in this ecosystem, the seasonal pattern of nutrient loading fluxes closely follows that of precipitation (Figure 2). SGD is also affected by precipitation (Moore, 1996). Rainfall events can lead to nutrient input from the hinterland areas. The monthly rainfall in Rongcheng in August 2022 was 259 mm (Table 1). The precipitation in August 2022 was approximately double that in April 2023. River discharge can be enhanced by rainfall, and weathering rates are affected by precipitation and temperature (Liu et al., 2011), which can lead to higher nutrient values during the wet season. High nutrient concentrations (DIN and DSi) and low salinity were observed in the bay (Figures 3−5), suggesting that rainfall is an important factor affecting the nutrient supply to SGB during summer. Additionally, the precipitation in April 2023 reached approximately 70 mm immediately before the investigation and was much higher (approximately 28 times) than that in a normal year (Figure 2). This may have led to a strong mixing and high nutrient levels compared with normal years (Li et al., 2016). In addition, wet deposition resulted in less DIP and DSi relative to DIN. The atomic nutrient flux DIN: DIP ratios were 38 and 25 during spring and summer, respectively. The DSi: DIN ratio was < 0.1, and that for DSi: DIP was approximately 1. This implies that more DIP and DSi in the bay were depleted due to wet deposition. This may be a reason for the lower DIP and DSi export fluxes compared to the riverine fluxes.
4.2 Role of aquaculture activity in nutrient biogeochemistry
Cultural species can influence the nutrient dynamics in aquatic ecosystems by sequestering, remineralizing, or physically moving nutrients between habitats (Li et al., 2021). Immersed structures can significantly reduce the current velocity inside an aquaculture area by generating frictional forces when currents traverse them (Fan et al., 2019; Hulot et al., 2020). The history of raft cultivation in SGB dates back to the early 1980s. Bivalves are raised in cages, nets, or other containers hung from floats or rafts. Seaweed was farmed near the entrance to the bay. The τ (17.1 d during spring and 25.2 d during summer) may be related to the presence of aquaculture facilities. The total water exchange time between SGB and the YS was reduced. Our results agree with those of previous studies that found a > 50% reduction in the average current speed owing to aquaculture facilities and cultured species (Zhao et al., 1996; Grant and Bacher, 2001; Shi et al., 2011a; Zeng et al., 2015; Li et al., 2016). Nutrients are likely to be retained in the bay because of weaker currents and depletion. Kelp assimilates large amounts of nutrients during spring (Shi et al., 2011b; Fan et al., 2019). A large-scale kelp population died during spring 2022 because of nutrient deficiency (Li et al., 2023). The net primary production of cultured kelp could reach 1.5 g C m–2 d–1 (Gao et al., 2021), which is almost one order of magnitude higher than that of phytoplankton in the bay. Hence, large quantities of DIN and DIP would be used by kelp when seawater flows through the seaweed farm and integrated culture areas during the growth period from late November to May (during the dry season), and would be removed through kelp harvesting in May. Nitrogen and phosphorus (> 97%) were removed through kelp harvesting. As a result, kelp was a net sink for DIN and DIP during the dry season and competed with phytoplankton during the kelp growth period. Nutrient concentrations were maintained at low levels during spring. In addition, the water transparency during spring was lower than that during summer (Wang, 2010). Consequently, phytoplankton growth was restrained.
Bivalve aquaculture generally commences during April–May, along with kelp (S. japonica) harvesting. Bivalves have become the primary farmed species. Many studies have reported that bivalves alter nutrient cycling by excreting nitrogen and phosphorus (Ragueneau et al., 2005; Li et al., 2021; Ray et al., 2020, 2021; Filippini et al., 2023). Our study over nine months showed that the metabolic rates for oysters and scallops were the highest in August (Li et al., 2024). Mao et al. (2006) reported that the maximum metabolic rates for oysters were recorded in July and August. Large amounts of metabolic byproducts can be generated when bivalves are in active growth stages (Li et al., 2016). Hence, large quantities of DIN and DIP can be released into the bay through excretion and may become important sources of nutrients. Based on the budgets, DIN and DIP released from bivalve excretion accounted for 24 and 58%, respectively, of the total influx to SGB during summer (Table 2). Dissolved nutrients released through bivalve excretion have the potential to stimulate phytoplankton production on a local scale and increase the risk of harmful algal blooms (van Broekhoven et al., 2014; Buschmann et al., 2008; Pietros and Rice, 2003). S. japonica was replaced by G. lemaneiformis in May, which was harvested during summer. However, the G. lemaneiformis cultivation area was smaller than that for S. japonica. Therefore, phytoplankton can absorb large amounts of nutrients during summer. Phytoplankton > 3 µm will be consumed by bivalves and their biomass will be reduced (Newell and Koch, 2004). However, the high nutrient levels in the system led to a high phytoplankton growth rate. High dissolved nutrient levels, coupled with high solar radiation and temperatures, result in higher phytoplankton production during summer compared with spring. This was consistent with previous study in SGB (Li et al., 2016) and other systems (Shpigel, 2005). The Chl a concentration was significantly higher during summer than during spring (Figure 6). Additionally, large amounts of DIN and DSi were discharged into the bay and the total DIN and DIP influx ratio was much higher than Redfield ratio (Table 1), which probably led to DIP levels decreasing rapidly to a low level in summer (Figure 8) and led to phosphorus limitation. Bivalves generally promote efficient nutrient cycling in the bay by transferring nutrients to biomass through assimilation in their tissues or the release of feces or pseudo-feces. DIP and DIN can be removed through bivalve harvesting (Shpigel, 2005; Troell et al., 2009). We found that 50 and 75% of the total nitrogen and phosphorus outfluxes, respectively, were attributed to bivalve and G. lemaneiformis harvesting (Table 2). Hence, bivalves play an important role in nutrient cycling in SGB during summer.
Furthermore, bivalves can affect nutrient cycles by modifying the benthic–pelagic exchange rates through their biological activity, and affect the long-term properties of sediments by modifying their chemical composition. Filter feeders lead to the excretion of enormous quantities of biodeposits on the sediment surface as feces and pseudo-feces (Norkko et al., 2001). Previous studies have indicated that biodeposits are enriched in organic materials such as nitrogen and phosphorus (Filippini et al., 2023); for example, 40–80% of the nitrogen filtered from the study system can be excreted through biodeposits (Cranford et al., 2007; Jansen et al., 2012; van Broekhoven et al., 2014). Dissolved nutrients are released during biodeposit decomposition (Jansen et al., 2012). The decomposition of feces and pseudo-feces is another important pathway for nutrient regeneration (van Broekhoven et al., 2014). This study shows that benthic flux is another important source of nutrients in SGB, particularly during spring, when nutrient supplementation is limited (Table 2). In addition, the benthic flux (N):benthic flux (Si) >1 (DINFlux: DSiFlux = 3.7 during both spring and summer) indicated that bivalves drive greater nitrogen than Si sediment regeneration and likely drive Si to become limiting to production. Additionally, bivalves significantly changed the ratio of sediment nitrogen to phosphorus regeneration, particularly during summer (DINflux: DIPflux > 600), and probably enhanced phosphorus limitation during the wet season. Enhanced nutrient regeneration maintains a productive ecosystem, however, changes in nitrogen availability relative to Si and DIP, can potentially lead to shifts in phytoplankton community structure (Turner et al., 1998; Ray et al., 2020).
Nutrient concentrations in SGB have significantly changed over the last four decades on an annual scale, particularly between the 1980s and the 1990s (Li et al., 2016; Zhang et al., 2023), similar to many other aquatic systems (Boesch, 2002; Liu et al., 2008). The DIN concentration increased 11-fold between the 1980s and the 1990s, and 12-fold between the 1980s and the 2010s. Relative to DIN, DSi remained almost unchanged on an annual scale over four decades (Li et al., 2016). The aquaculture area rapidly increased in pursuit of economic benefits during the 1990s (Fang et al., 1996). Nutrient-rich aquaculture effluents were released into natural water bodies without prior treatment in SGB. High concentrations of nitrogen in aquaculture effluents primarily originate from the excretion of farmed animals or excess feed (Burford and Williams, 2001). This demonstrates the influence of aquaculture activity on the nutrient levels in the bay.
Nutrient ratios also changed as the nutrients increased and decreased. As a result of the increased nitrogen levels, the atomic DIN: DIP ratios gradually increased from severe nitrogen limitation during the 1980s (2−3) to phosphorus limitation during the 1990s (35) (Zhang et al., 2023), following a rapid increase in aquaculture activity. Phytoplankton growth is currently limited by DIP during summer. An increase in the DIN: DIP molar ratio in SGB is a common phenomenon observed in long-term studies of semi-closed bays used for aquaculture, such as Jiaozhou Bay (Liu et al., 2005; Sun et al., 2011) and Daya Bay (Wang et al., 2009). Over the last 40 yrs, DSi: DIN molar ratios in SGB shifted from 1.4−18 during the 1980s to < 1 during the 2010s, reflecting the impact of increased nitrate. Decreasing DSi: DIP ratios also occurred because additional Si was retained in the bay sediments. It is thought that the DSi level was abundant and was not a limiting factor for phytoplankton growth (Liu et al., 2005). In recent years, DSi concentrations in SGB have always been lower than the threshold values for phytoplankton growth during spring because of less riverine input during the dry season and an increase in the DIN level. Thus, the possibility of using DSi has gradually increased. DSi limitations in marine systems have also been examined in a variety of marine environments, including Laizhou Bay (You et al., 2021), Zhangzi Island (Liang et al., 2019), and Sishili Bay (Wang et al., 2012), owing to the increase in riverine nitrogen fluxes.
Nutrients released from shellfish can be taken up by seaweed during their growth in the IMTA system. Large-scale seaweed cultivation plays an important role in the maintenance of low nutrient levels. Although water quality in SGB is maintained under a relatively good conditions, and nutrient levels in the bay are not significantly elevated compared with other bays used for monoculture, for example, Laizhou Bay (Jiang et al., 2018), Daya Bay (Li, 2020), Qinzhou Bay (Chen et al., 2022), and Gaolong Bay (Liu et al., 2011) in China, red tides have occurred in the bay (Zhang et al., 2012; Zhang et al., 2023). Farming capacity must be considered to ensure the health and sustainability of the system.
4.3 Silicon cycle in SGB
4.3.1 SGB BSi Production
The particulate organic carbon (POC) concentrations in the bay in August 2022 were 0.27−2.06 mg L−1 with an average of 0.83 ± 0.62 mg L–1. The POC content was higher than that reported by Xia et al. (2013) (0.04−1.09 mg L–1, August 2012). As a result, the average PBSi: POC molar ratio was only 0.04 mol mol–1 in August 2022. There was a correlation between PBSi and POC (R2 = 0.58, p < 0.01), indicating that PBSi and POC had the same sources during the wet season. This value was close to the average ratio in the Bay of Brest (0.08, Ragueneau et al., 2005), although lower than the BSi: POC molar ratio on the East China Sea Shelf (0.12 to 1.4) (Iseki et al., 2003; Liu et al., 2005) and Si/C stoichiometry of 0.13 mol mol–1 obtained for coastal diatoms grown under nutrient-replete conditions (Brzezinski, 1985), because diatoms are a component of suspension feeders diets, which may lead to less silicified frustules in the surface water. The POC: Chl a ratio in April was approximately 117 g g–1 which was estimated based on the average POC concentration (140 μg L–1) in April 2000 in SGB (Zhang et al., 2005). Therefore, the average PBSi: POC value in April 2023 in the bay was estimated at 0.29 mol mol–1. The value was comparable to that in Jiaozhou Bay (0.28 mol mol–1) (Liu et al., 2008). A decrease in silicate uptake from the environment into diatom cells and an increase in the BSi dissolution rate in water may result in a low PBSi: POC ratio.
Diatoms require Si to build their siliceous shells, some of which are regenerated through dissolution in the euphotic layer. There are no historical data on the production rate in SGB. However, BSi production can be indirectly estimated via primary production, the percentage of diatoms contributing to primary production, and the BSi: POC ratio of diatom cells (Nelson et al., 1995; Liu et al., 2005; Tréguer and de la Rocha, 2013). We assumed that the ratio of the BSi production to the carbon primary production rate ratio was similar to the ratio of PBSi: POC ratio in the water column. The primary production was approximately 85 mg C m−2 d−1 during spring and 570 mg C m−2 d−1 during summer (Jiang et al., 2015). Hence, BSi production was estimated at 26.6 × 106 mol Si (90d)−1 during spring and 24.6 × 106 mol Si (90d)−1 during summer. However, use of the BSi: POC ratios of natural particle assemblages to convert primary productivity data into BSi production are subject to uncertainties (Tréguer et al., 1995). It is well known that OM is recycled more rapidly than BSi (Ragueneau et al., 2000). The high PBSi: POC ratio in SGB in April 2023 may have been related to the resuspension of sediment. Therefore, there is a risk of overestimating BSi production.
4.3.2 First tentative SGB Si reservoirs
In SGB, a low DSi concentration, which was lower than the threshold value, always appeared in late May, and evidence is increasing for Si to be considered a potential limiting nutrient for diatom growth. Diatoms remain the absolute dominant species in the phytoplankton community; however, the number of diatom species has shown an obvious decline over the past decades (Li et al., 2010). Other Si sources and features of the bay (shallow water columns, good mixing, and high regeneration rates) may have supported high diatom production. BSi production may be sustained by these external inputs and internal recycling in the water column and at the sediment–water interface. Thus, the contribution of internal recycling in SGB was explored.
As discussed above, the sum of DSi and PBSi discharge from the primary streams emptying into SGB was 2.82 × 106 mol (90d)–1 during spring and 62.7 × 106 mol (90d)–1 during summer (Figure 11). Domestic wastewater is discharged directly into rivers; thus, the DSi and PBSi discharge from wastewater is included in the river discharge. The atmospheric deposition fluxes of DSi were 23.6 × 104 mol (90d)–1 during spring and 1.07 × 104 mol (90d)–1 during summer based on rainwater around SGB and aerosol sample collection and measurements at Yangmadao during December 2019−November 2020 (Xie et al., 2021), although data for atmospheric nutrient deposition of are more limited than data for surface waters, particularly dry deposition data.
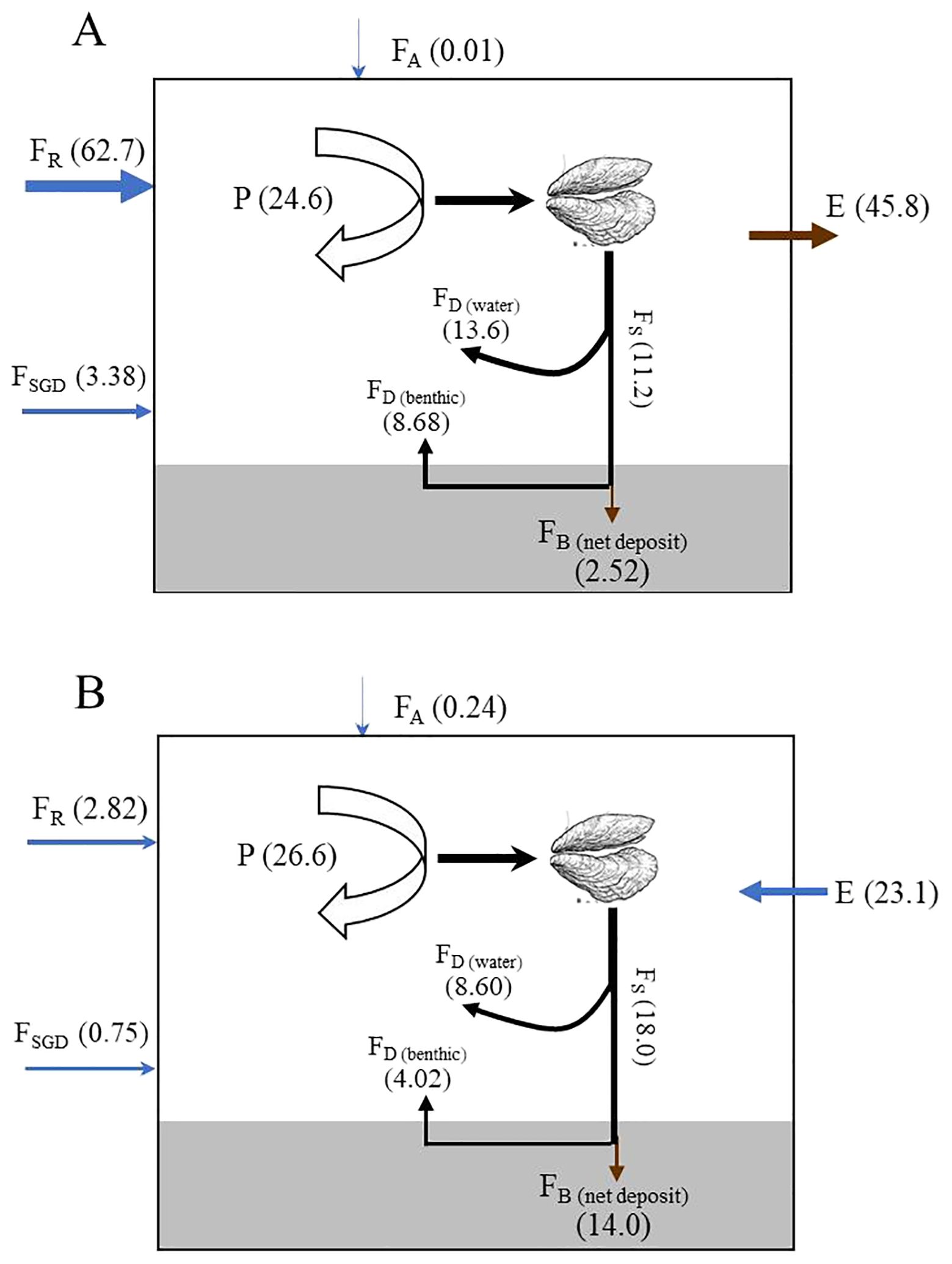
Figure 11. Scheme summarizing the silicon stocks and fluxes (106 mol/(90d)) through the planktonic-bivalves structure of Sanggou Bay. Silicon pools are shown in parentheses. (A), August 2022; (B), April 2023; FR, River input; FA, atmospheric deposition; P, BSi gross production; FB(net deposit), net deposition of BSi in sediment; FD(water), DSi flux recycled in the water column; FD(benthic), silicate flux at the sediment–water interface; FS(rain), BSi flux that reaches the sediment–water interface; E, total silicon (i.e., silicic acid plus BSi) exchange flux from the Yellow Sea.
Bivalve farming may lead to depletion of plankton abundance when the bivalve stock is too large (Tan et al., 2024). Jiang et al. (2016) found that the presence of oyster farms in Daya Bay reduced the Chl a by 60%. The Gouqi Island Chl a concentration was more than 80% lower than that in the non-farming regions (Lin et al., 2016). Although 30% of the area of Jiaozhou Bay is used for shellfish aquaculture, phytoplankton consumption by farmed shellfish accounts for 90% of the total phytoplankton consumption in the entire semi-closed ecosystem (Han et al., 2017; Tan et al., 2024). A large area is used for bivalve farming in SGB (Figure 1), which is a shallow bay. Large amounts of phytoplankton may be consumed by farmed bivalve and be rapid settlement to the sediment. Therefore, the natural deposition of diatoms was ignored in the budget. The BSi produced, which is neither recycled in the water column nor exported from the bay, becomes available for suspension feeders and is referred to as sedimentation. Bivalves filter phytoplankton cells > 3 μm as their primary food (Newell and Koch, 2004). Among phytoplankton of this size, diatoms are the major food source for bivalves. As filter feeders control the sedimentation flux and have no known requirements for Si, most of the net BSi sedimentation should be found in the biodeposition flux. Sediment traps for carbon have been reported, but no associated BSi measurements have been reported. A rigorous Si:C ratio cannot be used to estimate BSi sedimentation, as the material consists of both autochthonous material and detrital materials brought into the bay by rivers. Therefore, we used an indirect approach to estimate BSi sedimentation during these two periods. Thus, ingestion rate data were used to estimate the diatom uptake rate by bivalves. The carbon ingestion rates of bivalves were 2710 mg C (90 d)–1 during spring and 2566 mg C (90 d)–1 during summer (Li et al., 2024). Assuming the C:Chl a ratio was 117 g g–1 during spring and 199 g g–1 during summer, and the Chl a:DIN concentration ratio was 0.9 μg μmol–1 (Yin et al., 1996). Based on the Redfield ratio (DSi: DIN = 1:1), 18.0 × 106 mol Si (90d)–1 during spring and 11.2 × 106 mol Si (90d)–1 during summer would be absorbed by phytoplankton to sustain bivalve in SGB. As suspension feeders have no known requirement for nutrient DSi during their growth period (Ragueneau et al., 2005), and the silica content of bivalve tissue and shells in SGB is very low and can be ignored, almost all ingested BSi should be excreted as biodeposits in the form of feces and pseudo-feces. Hence, the BSi biodeposition flux was estimated based on the amount of silica acid consumed by diatoms. The BSi biodeposition fluxes that reached the sediment–water interface (FS) were 18.0 × 106 mol (90d)–1 during spring and 11.2 × 106 mol (90d)–1 during summer. The maximum metabolic rate was recorded during summer (Mao et al., 2006), and the carbon ingestion rate was higher during summer than during spring (Li et al., 2024). Therefore, biodeposition rates were expected to be higher during summer but were found to be higher during spring (Figure 11). This may be related to the growth stage of the bivalves. The bivalves were in the early growth stage in August 2022, and in the middle growth stage in April 2023. The carbon ingestion rate was 0.18 ± 0.06 mg C h–1 ind–1 during the early growth stage in June 2022 and could reach 1.38 ± 0.46 mg C h–1 ind–1 in June 2023 at harvesting. This may have led to a higher carbon ingestion rate during spring 2023 than during summer 2022. As a result, the BSi biodeposition flux may have been underestimated during summer 2022.
The fate of diatom silica in surface sediments is largely influenced by the active filter feeding of bivalves, which defecate Si-rich feces that facilitate diatom silica retention on the sediment surface (Chauvaud et al., 2000; López-Acosta et al., 2022). Active biodeposition by suspension feeders is known to increase downward particle fluxes near the sediment–water. Si removal is governed solely by its accumulation in the sediment. BSi burial is an important pathway for Si removal from the bay. Additionally, the amount of dissolved Si contributes to interstitial water saturation and ultimately feeds the Si benthic efflux from the sediments of the bay into the water column, a recycling process that helps sustain planktonic diatom populations during the productive season in the bay. As discussed above, the benthic DSi flux was estimated at 4.02 × 106 mol (90d)–1 during spring and 8.68 × 106 mol (90d)–1 during summer. This amount may contribute to approximately 35% of the PBSi production during summer and 15% during spring (Figure 11). The initial BSi accumulation estimate in the bay was obtained by subtracting the benthic DSi flux from the biodepositional BSi flux. Hence, the net sediment Si accumulation rate in SGB was 14.0 × 106 mol (90d)–1 during spring and 2.52 × 106 mol (90d)–1 during summer. Based on the difference between BSi production and biodeposition flux (FS), the DSi recycled in the water column was estimated at 8.60 × 106 mol (90d)–1 during spring and 13.6 × 106 mol (90d)–1 during summer, which accounted for 32% of PBSi production during spring and 55% during summer, respectively (Figure 11). In the SGB interior Si cycle, apart from the benthic flux at the water–sediment interface, recycling from the water column is the major source complementing the DSi reservoir.
The BSi burial efficiency, that is, the BSi accumulation rate divided by the sum of the BSi accumulation rate and benthic DSi flux, was calculated as 78% during spring and 23% during summer. The burial efficiency during spring was comparable to that in the Bohai Sea (72%, Liu et al., 2016) and the East China Sea (81%, Wu and Liu, 2020), and much higher than that in Jiaozhou Bay (49%) (Liu et al., 2008), the South China Sea (27%) (Ma et al., 2022), and the open oceans (1.9−39%) (Ragueneau et al., 2009; Hou et al., 2019) due to the high biodepositional rate in SGB. The burial efficiency during summer was much lower than these values and comparable to the global ocean average (~20%) (Tréguer et al., 1995). The high burial efficiency during spring indicated that little room was left for export from the bay or dissolution in the water column. Export from the bay is important only during the wet season with high river flow.
The BSi preservation efficiency (the BSi production rate divided by the BSi accumulation rate) in SGB is estimated at 53% during spring and 10% during summer, which is approximately 370 and 70 times higher than global carbon preservation efficiency, respectively. This makes BSi in sediments a potential proxy for export production (Tréguer et al., 2018). SGB preservation efficiency during spring was much lower than that in the Bay of Brest (82%, Ragueneau et al., 2005) and higher than that in Jiaozhou Bay (17%) (Liu et al., 2008), the Bohai Sea (6.5%, Liu et al., 2016), the YS (24.3%, Wu et al., 2017), and the East China Sea (12%, Li, 2019). The preservation efficiency during summer was lower than that in the YS and Jiaozhou Bay and comparable to that in the East China Sea. Efficiency reflects the ability of the SGB settling and sedimentation environment to preserve BSi. The vertical transportation process through the water column (feces and pseudo-feces) and the nature of the siliceous assemblage (species composition) are important factors controlling the preservation of siliceous material between production in the surface water and final accumulation in the sediment. Preservation mechanisms are important in SGB because of their suspension-feeder filtration/biodeposition activity and aggregate formation. BSi dissolves more readily after the diatoms are filtered and digested by the bivalves in SGB. Therefore, this may lead to a lower preservation efficiency during summer than during spring. BSi dissolution in the shallow bay probably occurred at the sediment–water interface. This result is consistent with the model estimation (Figure 11). The high BSi preservation rate indicates that burial is an important sink for Si and has a significant impact on the regional carbon cycle.
Considering all Si input fluxes into SGB, the riverine DSi input (FR) accounted for 12% during spring and 84% during summer. The total DSi input to the bay represented 88% of the PBSi production during spring. Taking the average DSi concentrations (4.31 μM during spring; 16.4 μM during summer) and the total water volume of 1.08 × 109 m3 in SGB, the bay can provide a total DSi load for ca. 16 days during spring and 65 days during summer of the PBSi production. This indicates the importance of water column dissolution and DSi regeneration in the SGB sediment during the dry season. In particular, biodeposition represents 6.4 times the river input during spring. This suggests that riverine inputs could not sustain diatom production during the dry season, which was not the case during the wet season. Considering, the above results are preliminary; they must be carefully interpreted. Most of the data in the bay were estimated because of insufficient data. The net Si input was not equal to the net output during spring or summer. The total Si input flux into SGB was approximately 1.5 and 2.2 times higher than the Si output flux during summer and spring, respectively. Thus, the estimated Si budget was not steady. The primary uncertainties in the results may be related to many factors, including an underestimated BSi production during summer due to different carbon ingestion rates during different growth stages and underestimation of the benthic DSi flux due to bioturbation and strong resuspension. Further studies should focus on solving the effects of bioturbation and tides on the resuspension of the bottom sediment to obtain the actual vertical DSi flux, BSi production and dissolution rates, and seasonal changes in the input and regeneration rates of both DSi and BSi.
4.4 Biodeposition effect on Si retention
Biodeposition typically leads to high deposition rates that exceed those of natural sedimentation and creates sediment enrichment in materials such as biogenic (Ragueneau et al., 2005; van Broekhoven et al., 2014) and trace elements (Liu et al., 2023). In SGB, the deposition rate (0.6−2.1 cm yr–1) was significantly higher than that in the YS (0.15 cm yr–1) and East China Sea (0.24 cm yr–1) which are not breeding areas (Yang et al., 2010; Bai et al., 2022). As discussed above, biodeposition plays an important role in Si retention in SGB. In recent decades, the sediment carbon content has increased after the development of large-scale aquaculture activity (Liu et al., 2015; Bai et al., 2022). These results emphasize the impact of bivalve biodeposition on OM sediment enrichment. BSi enrichment in the sediment may be even greater. Si retention along the land–ocean continuum may be enhanced by biodeposition.
Grazers play an important role in Si and carbon interactions in the world oceans, such as in the upper waters or at the sediment–water interface (Ragueneau et al., 2002b). Grazers typically have no Si requirement; when they consume diatoms, they retain particulate OM but egest frustules without Si excretion (Tande and Slagstad, 1985; Ray and Fulweiler, 2020). This phenomenon has also been observed in other regions (Chauvaud et al., 2000; Dagg et al., 2003; Ragueneau et al., 2002b). Forty-six percent (ranging from 30 to 74%) of the carbon consumed was found in the feces and pseudo-feces of 10 different mollusks at the sediment–water interface (Pouvreau, 1999). Similarly, bivalves retained approximately 40% of the ingested carbon (Li et al., 2024), leaving 60% in feces and pseudo-feces in SGB. With this carbon assimilation and still assuming that 100% of the BSi is egested in the feces, the PBSi: POC ratio is expected to increase by a factor of 1.4−1.7 between its value in diatoms and its value in bivalve feces in SGB. Therefore, Si retention in this ecosystem must increase with increasing biodeposition induced by the proliferation of exotic suspension feeders because the net OM sedimentation is strongly increasing (Bai et al., 2022) and the biodeposits are enriched in BSi. DSi is primarily exported to the sediment through the sinking of DSi-rich fecal pellets. In SGB, most (77.5%) of the biodeposited BSi was recycled during the wet season (Figure 11). Benthic fluxes reached higher values at the monoculture site than at the site where kelp was co-cultivated (Ning et al., 2016), suggesting that bivalves play an important role in controlling benthic fluxes. As a result, DSi may not be retained in a recycling pool during the wet season, as is nitrogen in the ammonium pool, and it is continually exported from the system at a higher rate than nitrogen. Phytoplankton use recycled nitrogen to balance their DSi uptake, consequently, leading to greater DSi depletion in the bay. Although most of the BSi in the biodeposits is recycled and fuels summer production, a significant fraction (78%) is buried during spring (Figure 11). The high Si preservation efficiency leads to high Si retention during the dry season. Benthic flux is an important DSi source during the dry season (Table 2). This suggests that bivalves have the potential to alter Si retention in the bay by producing large amounts of biodeposits and accelerating the silica cycle, which leads to more carbon dioxide being absorbed by diatoms.
The calculations presented herein suggest that the production of biodeposits and BSi preservation in the biodeposits may be high enough to sustain an important annual Si accumulation flux in the SGB ecosystem, which is important with respect to the other Si sources and sinks in this ecosystem and possibly important with respect to what this flux was before aquaculture began. In ecosystems where suspension feeders continue to spread, Si retention along the land–ocean continuum may increase with time, particularly during the dry season. This is another route of silica depletion (Ragueneau et al., 2005). This route does not require enhanced diatom production but simply enhances BSi preservation by increasing biodeposition due to the proliferation of suspension feeders. It is important to closely examine nutrient monitoring stations and search for possible long-term changes in DSi concentrations in seaward areas that are subject to the proliferation of biodepositing organisms. An important next step in understanding the effect of bivalves on coastal biogeochemistry is the development of predictive models that use local environmental characteristics such as temperature, salinity, water-column dissolved nutrient concentrations, and/or sediment physical and chemical properties.
5 Conclusions
SGB is a typical shallow-water IMTA system with primary productivity dominated by diatoms. Our study highlights that aquaculture activity plays an important role in nutrient cycling. Under the combined effects of natural processes and aquaculture activity, the nutrient levels and cycling in the bay were affected. Nutrients showed considerable seasonal and regional variation in the bay and were higher during summer than during spring owing to seaweed and phytoplankton uptake and freshwater discharge. The results indicated that SGB behaved as a sink for DIP, DIN, and DSi during both the wet and dry seasons. DSi was primarily derived from freshwater discharge and benthic flux. Bivalve excretion is an important source of DIP, and plays an important role when phytoplankton growth is limited by phosphorus. Large amounts of DIN and DIP are removed through seaweed and bivalve harvesting during both the wet and dry seasons.
PBSi distribution was similar to that of DSi in solutions, which was influenced by SPM and Chl a. The PBSi content in the streams accounted for approximately 10% of the total silica content. A Si balance for SGB was established based on the box model. Among all the input pathways for biologically available Si, the exchange flux between SGB and the YS was the largest external Si input, accounting for 75%, followed by benthic flux (13%). The major source of Si during summer was freshwater discharge, which accounted for 84% of the total influx, followed by benthic flux (12%). The regenerated DSi represented 47% of the BSi produced during spring, of which 32% was regenerated in the water column and 15% in the sediment. The regenerated DSi accounted for 91% of the BSi produced during summer, of which 55% was regenerated in the water column and 36% in the sediment. DSi regeneration may be an important process that sustains diatoms as the dominant species in SGB. The BSi burial efficiency was estimated to be approximately 78% during spring and 23% during summer. This may lead to Si limitations in SGB. However, limited data (biodeposition and modern sedimentation rates), different siliceous production contributions, and bivalve growth stages, result in relatively skewed budgets. Therefore, further research is required.
Data availability statement
The raw data supporting the conclusions of this article will be made available by the authors, without undue reservation.
Author contributions
RL: Data curation, Formal analysis, Funding acquisition, Investigation, Methodology, Software, Writing – original draft, Writing – review & editing. ZJ: Funding acquisition, Resources, Writing – review & editing. FL: Data curation, Investigation, Writing – review & editing. YZ: Writing – review & editing. WW: Formal analysis, Investigation, Writing – review & editing. KZ: Investigation, Writing – review & editing. WL: Formal analysis, Investigation, Writing – review & editing. DW: Investigation, Writing – review & editing. YS: Investigation, Writing – review & editing.
Funding
The author(s) declare financial support was received for the research, authorship, and/or publication of this article. This study was supported by National Natural Science Foundation of China (42376151), the Laoshan Laboratory (LSKJ202204004), Central Public-interest Scientific Institution Basal Research Fund, YSFRI, CAFS (NO. 20603022023013), Central Public-interest Scientific Institution Basal Research Fund, CAFS (2023TD54), The Young Taishan Scholars Program of Shandong Province (tsqn201909166), China Agriculture Research System of MOF and MARA, and National Natural Science Foundation of China (32303035).
Acknowledgments
We thank colleagues for their help during field investigations.
Conflict of interest
Author YZ was employed by company Rongcheng Chudao Aquaculture Co., Ltd.
The remaining authors declare that the research was conducted in the absence of any commercial or financial relationships that could be construed as a potential conflict of interest.
Publisher’s note
All claims expressed in this article are solely those of the authors and do not necessarily represent those of their affiliated organizations, or those of the publisher, the editors and the reviewers. Any product that may be evaluated in this article, or claim that may be made by its manufacturer, is not guaranteed or endorsed by the publisher.
Supplementary material
The Supplementary Material for this article can be found online at: https://www.frontiersin.org/articles/10.3389/fmars.2024.1431878/full#supplementary-material
References
Allen J. T., Brown L., Sanders R., Moore C. M., Mustard A., Fielding S., et al. (2005). Diatom carbon export enhanced by silicate upwelling in the northeast Atlantic. Nature 437, 728–732. doi: 10.1038/nature03948
Bai H. Y., Liu S., Yang Q., Huang L. F., Sun Y. (2022). High-resolution records of the rate of carbon accumulation in the shellfish aquaculture area in Sanggou Bay and its response to human aquaculture activities. Prog. Fish. Sci. 43, 98–105. doi: 10.19663/j.issn2095-9869.2021
Bartoli M., Nizzoli D., Viaroli P., Turolla E., Castaldelli G., Fano E. A., et al. (2001). Impact of tapes philippinarum farming on nutrient dynamics and benthic respiration in the sacca di goro. Hydrobiologia 455, 203–212. doi: 10.1023/A:1011910422400
Beck A. J., Rapaglia J. P., Kirk Cochran J., Bokuniewicz H. J., Yang S. H. (2008). Submarine groundwater discharge to Great South Bay, NY, estimated using Ra isotopes. Mar. Chem. 109, 279–291. doi: 10.1016/j.marchem.2007.07.011
Beucher C., Tréguer P. J., Corvaisier R., Hapette A. M., Elskens M. (2004). Production and dissolution of biosilica, and changing microphytoplankton dominance in the Bay of Brest (France). Mar. Ecol. Prog. Ser. 267, 57–69. doi: 10.3354/meps267057
Boesch D. F. (2002). Challenges and opportunities for science in reducing nutrient over-enrichment of coastal ecosystems. Estuaries 25 (4B), 886–900. doi: 10.1007/BF02
Brzezinski M. A. (1992). Cell-cycle effects on the kinetics of silicic acid uptake and resource competition among diatoms. J. Plankton. Res. 14, 1511–1536. doi: 10.1093/plankt/14.11.1511
Brzezinski M. A. (1985). The Si:C:N ratio of marine diatoms: interspecific variability and the effect of some environmental variables. J. Phycol. 2, 347–357. doi: 10.1111/j.0022-3646.1985.00347.x
Burford M. A., Williams K. C. (2001). The fate of nitrogenous waste from shrimp feeding. Aquaculture 198, 79–93. doi: 10.1016/S0044-8486(00)00589-5
Buschmann A. H., Varela D. A., Hernández-González M. C., Huovinen P. (2008). Opportunities and challenges for the development of an integrated seaweed-based aquacul_ture activity in chile: determining the physiological capabilities of macrocystis and gracilaria as biofilters. J. Appl. Phycol. 20, 571–577. doi: 10.1007/978-1-4020-9619-8_17
Chauvaud L., Jean F., Ragueneau O., Thouzeau G. (2000). Long-term variation of the Bay of Brest ecosystem: benthic–pelagic coupling revisited. Mar. Ecol. Prog. Ser. 200, 35–48. doi: 10.3354/meps200035
Chen C. Q., Lao Q. B., Shen Y. L., Jin G. Z., Chen F. J., Su Q. Z., et al. (2022). Comparative study of nitrogen cycling between a bay with riverine input and a bay without riverine input, inferred from stable isotopes. Front. Mar. Sci. 9. doi: 10.3389/fmars.2022.885037
China Gulf Chronicles Compilation Committee (1991). China gulf chronicles (Bays in the northern and eastern of shandong peninsula). Ed. Chen Z. S. (Beijin: The Ocean Publishing Company press).
Cranford P. J., Strain P. M., Dowd M., Hargrave B. T., Grant J., Archambault M. (2007). Influence of mussel aquaculture on nitrogen dynamics in a nutrient enriched coastal embayment. Mar. Ecol. Prog. Ser. 347, 61–78. doi: 10.3354/meps06997
Cushing D. H. (1989). A difference in structure between ecosystems in strongly stratified waters and in those that are only weakly stratified. J. Plankton Res. 11, 1–13. doi: 10.1093/plankt/11.1.1
Dagg M. J., Urban-Rich J., Peterson J. ,. O. (2003). The potential contribution of fecal pellets from large copepods to the flux of biogenic silica and particulate organic carbon in the antarctic polar front region near 170°W. Deep-Sea Res. II 50, 675–691. doi: 10.1016/S0967-0645(02)00590-8
Dame R. F., Zingmark R. G., Haskin E. (1984). Oyster reefs as processors of estuarine materials. J. Exp. Mar. Biol. Ecol. 83, 239–247. doi: 10.1016/S0022-0981(84)80003-9
Dame R. F., Spurrier J. D., Zingmark R. G. (1992). In situ metabolism of an oyster reef. J. Exp. Mar. Biol. Ecol. 164, 147–159. doi: 10.1016/0022-0981(92)90171-6
Davidson K., Gowen R. J., Harrison P. J., Fleming L. E., Hoagland P., Moschonas G. (2014). Anthropogenic nutrients and harmful algae in coastal waters. J. Environ. Manage. 146, 206–216. doi: 10.1016/j.jenvman.2014.07.002
DeMaster D. J. (2003). The diagenesis of biogenic silica: Chemical transformations occurring in the water column, seabed, and crust. Treatise Geochem. 7, 407. doi: 10.1016/B0-08-043751-6/07095-X
Doering P. H., Kelly J. R., Oviatt C. A., Sowers T. (1987). Effect of the hard clam Mercenaria mercenaria on benthic fluxes of inorganic nutrients and gases. Mar. Biol. 94, 377–383. doi: 10.1007/BF00428243
Doering P. H., Oviatt C. A., Beatty L. L., Banzon V. F., Rice R., Kelly S. P., et al. (1989). Structure and function in a model coastal ecosystem: silicon, the benthos and eutrophication. Mar. Ecol. Prog. Ser. 52, 287–299. doi: 10.3354/meps052287
Duarte P., Meneses R., Hawkins A. J. S., Zhu M., Fang J. G., Grant J. (2003). Mathematical modelling to assess the carrying capacity for multi-species culture within coastal waters. Ecol. Model. 168, 109–143. doi: 10.1016/S0304-3800(03)00205-9
Dugdale R. C., Wilkerson F. P. (1998). Silicate regulation of new production in the equatorial Pacific upwelling. Nature 391, 270–273. doi: 10.1038/34630
Fan W., Zhao R. L., Yao Z. Z., Xiao C. B., Pan Y. W., Chen Y., et al. (2019). Nutrient removal from Chinese coastal waters by large-scale seaweed aquaculture using artificial upwelling. Water 11, 1754. doi: 10.3390/w11091754
Fan X., Wei H. (2010). Modeling studies on vertical structure of tidal current in a typically coastal raft-culture area. Prog. Fish. Sci. 31, 78–84. doi: 10.3969/j.issn.1672-5174.2009.02.002
Fang J. G., Sun H. L., Yan J. P., Kuang S. H., Li F., Newkirk G. F., et al. (1996). Polyculture of scallop Chlamys farreri and kelp Laminaria japonica in Sungo Bay. Chin. J. Oceanol. Limnol. 14, 322–329. doi: 10.1007/BF02850552
Filippini G., Dafforn K. A., Bugnot A. B. (2023). Shellfish as a bioremediation tool: A review and meta-analysis. Environ. pollut. 316, 120614. doi: 10.1016/j.envpol.2
Fu M. Z., Pu X. M., Wang Z. L., Liu X. J. (2013). Integrated assessment of mariculture ecosystem health in Sanggou Bay. Acta Ecol. Sin. 33, 238–316. doi: 10.5846/stxb
Gao Y. P., Zhang Y. T., Du M. R., Lin F., Jiang W. W., Li W. H., et al. (2021). Dissolved organic carbon from cultured kelp saccharina japonica: production, bioavailability, and bacterial degradation rates. Aquac. Environ. Interact. 13, 101–110. doi: 10.3354/AEI00393
Glibert P. M., Burford M. A. (2017). Globally changing nutrient loads and harmful algal blooms. Recent advances, new paradigms, and continuing challenges. Oceanography 30, 58–69. doi: 10.5670/oceanog.2017.110
Gordon D. C. Jr., Boudreau P. R., Mann K. H., Ong J.-E., Silvert W. L., Smith S. V., et al. (1996). LOICZ biogeochemical modelling guidelines. : LOICZ reports and studies, 5 (Texel, The Netherlands: LOICZ), 96 pp.
Grant J., Bacher C. (2001). A numerical model of flow modification induced by suspended aquaculture in a Chinese Bay. Can. J. Fish. Aquat. Sci. 58, 1–9. doi: 10.1139/f01-027
Grasshoff K., Kremling K., Ehrhardt M. (1999). “Methods of seawater analysis,” in Determination of nutrients. Eds. Hansen H. P., Koroleff F. (Wiley-VCH, Weinheim), 159–228.
Green D. S., Rocha C., Crowe T. P. (2013). Effects of nonindigenous oysters on ecosystem processes vary with abundance and context. Ecosystems 16, 881–893. doi: 10.1007/s10021-013-9659-y
Han D., Chen Y., Zhang C., Ren Y., Xue Y., Wan R. (2017). Evaluating impacts of intensive shellfish aquaculture on a semi-closed marine ecosystem. Ecol. Model. 359, 193–200. doi: 10.1016/j.ecolmodel.2017.05.024
Han L. J., Zhu Y. M., Liu S. M., Zhang J., Li R. H. (2013). Nutrients of atmospheric wet deposition from the qianliyan island of the yellow sea. China Environ. Sci. 33, 1174–1184. doi: 10.3969/j.issn.1000-6923.2013.07.004 (in Chinese with English abstract)
Hou Y., Hammond D. E., Berelson W. M., Kemnitz N., Adkins J. F., Lunstrum A. (2019). Spatial patterns of benthic silica flux in the north pacific reflect upper ocean production. Deep-Sea Res. Part I-Oceanographic Res. Papers 148, 25–33. doi: 10.1016/j.dsr.2019.04
Hulot V., Saulnier D., Lafabrie C., Gaertner-Mazouni N. (2020). Shellfish culture: a complex driver of planktonic communities. Rev. Aquacult. 12, 33–46. doi: 10.1111/raq.12303
Iseki K., Okamura K., Kiyomoto Y. (2003). Seasonality and composition of downward particulate fluxes at the continental shelf and okinawa trough in the east china sea. Deep_Sea Res. II 50, 457–473. doi: 10.1016/S0967-0645(02)004
Jansen H. M., Verdegem M. C. J., Strand O., Smaal A. C. (2012). Seasonal variation in mineralization rates (C–N–P–Si) of mussel mytilus edulis biodeposits. Mar. Biol. 159, 1567–1580. doi: 10.1007/s00227-012-1944-3
Jiang H. C., Wang Y. J., Li J. H., Tao H. M., Bai Y. Y., Su B., et al. (2018). Annual variation and spatial distribution of nutrients in the laizhou bay. Mar. Sci. Bull. 37 (4), 411–423. doi: 10.11840/j.issn.1001-6392.2018.0
Jiang T., Chen F. Y., Yu Z. H., Lu L., Wang Z. H. (2016). Size-dependent depletion and community disturbance of phytoplankton under intensive oyster mariculture based on HPLC pigment analysis in Daya Bay, South China Sea. Environ. pollut. 219, 804–814. doi: 10.1016/j.envpol.2016.07.058
Jiang Z. J., Fang J. G., Zhang J. H., Mao Y. Z., Wang W. (2007). Forms and bioavailability of phosphorus in surface sediments from Sungo Bay. Environ. Sci. 28, 2783–2788. doi: 10.13227/j.hjkx.2007.12.034
Jiang Z. J., Fang J. G., Mao Y. Z., Wang W., Shi H. X., Jiao H. F. (2010). Nutrient condition assessment and bioremediation strategy. Environ. Sci. Manag. 35, 162–167.
Jiang Z. J., Li J. Q., Qiao X. D., Wang G. H., Bian D. P., Jiang X., et al. (2015). The budget of dissolved inorganic carbon in the shellfish and seaweed integrated mariculture area of Sanggou Bay, Shandong, China. Aquaculture 446, 165–174. doi: 10.1016/j.aquaculture.2014.12.043
Jin X., Gruber N., Dunne J. P., Sarmiento J. L., Armstrong R. A. (2006). Diagnosing the contribution of phytoplankton functional groups to the production and export of particulate organic carbon, CaCO3, and opal from global nutrient and alkalinity distributions. Global Biogeochem. Cy. 20, GB2015. doi: 10.1029/2005GB002532
Justić D., Rabalais N. N., Turner R. E., Dortch Q. (1995). Changes in nutrient structure of river-dominated coastal waters: stoichiometric nutrient balance and its consequences. Estuar. Coast. Shelf Sci. 40, 339–356. doi: 10.1016/S0272-7714(05)80014-9
Kamatani A., Takano M. (1984). The behavior of silicic acid during the mixing of river and sea waters in Tokyo Bay. Estuar. Coast. Shelf. Sci. 19, 505–512. doi: 10.1016/0272-7714(84)90012-X
Kellogg M. L., Smyth A. R., Luckenbach M. W., Carmichael R. H., Brown B. L., Cornwell J. C., et al. (2014). Use of oysters to mitigate eutrophication in coastal waters. Estuar. Coast. Shelf. Sci. 151, 156–168. doi: 10.1016/j.ecss.2014.09.025
Kristiansen S., Hoell E. E. (2002). The importance of silicon for marine production. Hydrobiologia 484, 21–31. doi: 10.1023/A:1021392618824
Kuang S. H., Fang J. G., Sun H. L., Li F. (1996). Seston dynamics in Sanggou bay. Mar. Fish. Res. 17, 60–67.
Li F. X. (2019). Size fraction of phytoplankton and ecological contribution of microbial loop in large-scale bivalve mariculture area (Shangha: Shanghai Ocean University), 70 pp.
Li D. (2020). N2 fixation in typical bays and the south china sea (Xiamen: Xiamen University), 212 pp.
Li J. Y., Ianaiev V., Huff A., Zalusky J., Ozersky T., Katsev S. (2021). Benthic invaders control the phosphorus cycle in the world’s largest freshwater ecosystem. PANS 118, e2008223118. doi: 10.1016/j.aquaculture.2014.12.043
Li W. W., Jiang W. W., Jiang Z. J., Zhang K., Wan D. J., Shi Y. Z., et al. (2024). Comparative study on the feeding metabolism and carbon budget of the triploid and diploid Pacific oyster (Crassostrea gigas). Prog. Fish. Sci. 45, 125–134. doi: 10.19663/j.issn2095-9869.20230413001
Li R. H., Liu S. M., Zhang J., Jiang Z. J., Fang J. G. (2016). Sources and export of nutrients associated with integrated multi-trophic aquaculture in Sanggou Bay, China. Aquacult. Env. Interac. 8, 285–309. doi: 10.3354/aei00177
Li X. D., Su L., Li X. J., Li J., Xu Y. J., Chang L. R., et al. (2023). Comprehensive analysis of large-scale saccharina japonica damage in the principal farming area of rongcheng in Shandong province from 2021 to 2022. J. Agric. Sci. Tech. 25, 206–222. doi: 10.13304/j.nykjdb.2022.0728
Li C. L., Zhang Y. S., Sun S., Wu Y. L., Fang J. G., Zhang J. H. (2010). Species composition, density and seasonal variation of phytoplankton in sanggou bay, china. Prog. Fish. Sci. 31 (4), 1–8. doi: 10.3969/j.issn.1000-7075.2010.04.001
Liang Y., Zhang G. T., Wan A. Y., Zhao Z. X., Wang S. W., Liu Q. (2019). Nutrient-limitation induced diatom-dinoflagellate shift of spring phytoplankton community in an offshore shellfish farming area. Mar. pollut. Bull. 141, 1–8. doi: 10.1016/j.marpolbul.2019.02.00
Lin J., Li C. Y., Zhang S. Y. (2016). Hydrodynamic effect of a large offshore mussel suspended aquaculture farm. Aquaculture 451 (20), 147–155. doi: 10.1016/j.aquaculture.2015.0
Liu S., Huang J. S., Yang Q., Yang S., Yang G. S., et al. (2015). Burial fluxes and source apportionment of carbon in culture areas of sanggou bay over the past 200 years. Acta Oceanol. Sin. 34 (10), 23–30. doi: 10.1007/s13131-015-0724-6
Liu S. M., Li R. H., Zhang G. L., Wang D. R., Du J. Z., Herbeck L. S., et al. (2011). The impact of anthropogenic activities on nutrient cycling dynamics in the tropical wenchanghe and wenjiaohe estuary and lagoon system in east hainan, china. Mar. Chem. 125, 49–68. doi: 10.1016/j.marchem.2011.02.003
Liu S. M., Zhang J., Chen H. T., Zhang G. S. (2005). Factors influencing nutrient dynamics in the eutrophic jiaozhou bay, north china. Prog. Oceanogr. 66, 66–85. doi: 10.1016/j.pocean.2005.03.00
Liu J., Zang J.-Y., Zhang L. J., Sun T., Yu Z. G., Ran X. B. (2016). Distribution, fluxes and budget of silicon in the Yellow Sea. China Environ. Sci. 36, 157–166. doi: 1000–6923(2016)01–0157-1
Liu Q., Liao Y. B., Zhou J. H., Shi X. L., Shou L., Zeng J. N., et al. (2023). Influence of biodeposition by suspended cultured oyster on the distributions of trace elements in multiple media in a semi-enclosed bay of China. J. Hazard. Mater. 443, 130347. doi: 10.1016/j.jhazmat.2022.130347
Liu S. M., Ye X. W., Zhang J., Zhang G. S., Wu Y. (2008). The silicon balance in Jiaozhou Bay, North China. J. Mar. Sci. 74, 639–648. doi: 10.1016/j.jmarsys.2008.06.001
López-Acosta M., Maldonado M., Grall J., Ehrhold A., Sitjà C., Galobart C., et al. (2022). Sponge contribution to the silicon cycle of a diatom-rich shallow bay. Limnol. Oceanogr. 67, 2431–2447. doi: 10.1002/lno.12211
Loyer S., Lampert L., Menesguen A., Cann P., Labasque T. (2006). Seasonal evolution of the nutrient pattern on Biscay Bay continental shelf over the years 1999–2000. Sci. Mar. 70, 31–46. doi: 10.3989/scimar.2006.70n131
Ma Y. W., Zhang L. L., Liu S. M., Zhu D. D. (2022). Silicon balance in the south china sea. Biogeochemistry 157, 327–353. doi: 10.1007/s10533-021-00879-4
Mao Y. Z., Li J. Q., Xue S. Y., Lin F., Jiang Z. J., Fang J. G., et al. (2018). Ecological functions of the kelp Saccharina japonica in integrated multi-trophic aquaculture, Sanggou Bay, China. Acta Ecolog. Sin. 38, 3230–3237. doi: 10.5846/stxb201703160444
Mao Y. Z., Zhou Y., Yang H. S., Wang R. C. (2006). Seasonal variation in metabolism of cultured Pacific oyster, Crassostrea gigas, in Sanggou Bay, China. Aquaculture 253, 322–333. doi: 10.1016/j.aquaculture.2005.05.033
Moore W. S. (1996). Large groundwater inputs to coastal waters revealed by 226Ra enrichments. Nature 380, 612–614. doi: 10.1038/380612a0
Nelson D. M., Tréguer P., Brzezinski M. A., Leynaert A., Queguiner B. (1995). Production and dissolution of biogenic silica in the ocean: Revised global estimates, comparison with regional data and relationship to biogenic sedimentation. Global Biogeochem. Cycles 9, 359–372. doi: 10.1029/95GB01070
Newell R. I. E., Fisher T., Holyoke R., Cornwell J. (2005). Influence of eastern oysters on nitrogen and phosphorus regeneration in Chesapeake Bay, USA in The Comparative Roles of Suspension-Feeders in Ecosystems, Vol. 47. Eds. Dame R. F., Olenin S. (Netherlands: NATO Science Series: Earth and Environmental Sciences), 93–120. doi: 10.1007/1-4020-3030-4_6
Newell R. I. E., Koch E. W. (2004). Modelling seagrass density and distribution distribution in response to changes in turbidity stemming from bivalve filtration and seagrass sediment stabilization. Estuaries 27, 793–806. doi: 10.1007/BF02912041
Ning Z. M., Liu S. M., Zhang G. L., Ning X. Y., Li R. H., Jiang Z. J., et al. (2016). Impacts of an integrated multi-trophic aquaculture system on benthic nutrient fluxes: a case study in Sanggou Bay, China. Aquacult. Env. Interac. 8, 221–232. doi: 10.3354/aei00144
Niu Y. L. (2014). Study on seasonal variation of the C, N, P, Si, budget of the bivalves in Sanggou Bay. Zhoushan: Zhejiang Ocean Univeristy, 60 pp.
Norkko A., Hewitt J. E., Thrush S. F., Funnell G. A. (2001). Benthic–pelagic coupling and suspension-feeding bivalves: linking site specific sediment flux and biodeposition to benthic community structure. Limnol. Oceanogr. 46 (8), 2067–2072. doi: 10.4319/lo.2001.46.8
Parsons T. R., Maita Y., Laili C. M. (1984). A Manual of Chemical and Biological Methods for Seawater Analysis (Oxford: Pergamon Press), 173.
Peterson B. J., Heck K. L. Jr. (1999). The potential for suspension feeding bivalves to increase seagrass productivity. J. Exp. Mar. Biol. Ecol. 240, 37–52. doi: 10.1016/s0022-0981(99)00040-4
Pietros J. M., Rice M. A. (2003). The impacts of aquacultured oysters, crassotrea virginica (Gmeli) on water column nitrogen and sedimentation: results of a mesocosm study. Aquaculture 220, 407–422. doi: 10.1016/S0044-8486(02)00574-
Porter E. T., Franz H., Lacouture R. (2018). Impact of eastern oyster Crassostrea virginica biodeposits resuspension on the seston, nutrient, phytoplankton, and zooplankton dynamics: a mesocosm experiment. Mar. Ecol. Prog. Ser. 586, 21–40. doi: 10.3354/meps12417
Porter E. T., Robins E., Davis S., Lacouture R., Cornwell J. C. (2020). Effects of resuspension of eastern oyster Crassostrea virginica biodeposits on phytoplankton community structure. Mar. Ecol. Prog. Ser. 640, 79–105. doi: 10.3354/meps13277
Pouvreau S. (1999). Etude et modélisation des mécanismes impliqués dans la croissance de l'huıˆtre perlière, pinctada margaritifera, au sein de l'écosystème conchylicole du lagon de l'atoll de takapoto (Polynésie franc¸aise), thèse de doctorat (Rennes: ENSA de Rennes), 267 pp.
Prins T. C., Smaal A. C., Dame R. F. (1997). A review of the feedbacks between bivalve grazing and ecosystem processes. Aquat. Ecol. 31, 349–359. doi: 10.1023/A:1009924624259
Qu K. M., Song Y. L., Xu Y., Sun Y., Fang J. G. (2008). Experiment on nutrient limitations in cultured areas of Sanggou Bay in situ in spring and summer. Mar. Environ. Sci. 27, 124–127. doi: 10.3969/j.issn.1007-6336.2008.02.006
Ragueneau O., Chauvaud L., Moriceau B., Leynaert A., Thouzeau G., Donval A., et al. (2005). Biodeposition by an invasive suspension feeder impacts the biogeochemical cycle of Si in a coastal ecosystem (Bay of Brest, France). Biogeochemistry 75, 19–41. doi: 10.1007/s10533-004-5677-3
Ragueneau O., Conley D. J., Leynaert A., Longphuirt S. N., Slomp C. P. (2006). Role of diatoms in silicon cycling and coastal marine food webs. The Silicon Cycle: human perturbations and impacts on aquatic systems. Eds. Ittekkot V., Unger D., Humborg C., Tac An V. (Washington DC: Island Press), 275.
Ragueneau O., Chauvaud L., Leynaert A., Thouzeau G., Paulet Y. M., et al. (2002b). Direct evidence of a biologically active coastal silicate pump: ecological implications. Limnol. Oceanogr. 47, 1849–1854. doi: 10.2307/3096558
Ragueneau O., Dittert N., Pondaven P., Treguer P., Corrin L. (2002a). Si/C decoupling in the world ocean: is the southern ocean different? Deep-Sea Res. II 49 (16), 3127–3315.
Ragueneau O., Regaudie-de-Gioux A., Moriceau B., Gallinari M., Vangriesheim A., Baurand F., et al. (2009). A benthic si mass balance on the congo margin: Origin of the 4000 m DSi anomaly and implications for the transfer of si from land to ocean. Deep Sea Res. Part II 56 (23), 2197–2207. doi: 10.1016/j.dsr2.2009.04.0
Ragueneau O., Tréguer P., Leynaert A., Anderson R. F., Brzezinski M. A., DeMaster D. J., et al. (2000). A review of the si cycle in the modern ocean: recent progress and missing gaps in the application of biogenic opal as a paleoproductivity proxy. Global Planet. Change 26, 317–365. doi: 10.1016/S0921-8181(00)00052-7
Ray N. E., Al-Haj A. N., Fulweiler R. W. (2020). Sediment biogeochemistry along an oyster aquaculture chronosequence. Mar. Ecol. Prog. Ser. 646, 13–27. doi: 10.3354/meps13377
Ray N. E., Al-Haj A. N., Maguire T. J., Henning M. C., Fulweiler R. W. (2021). Coastal silicon cycling amplified by oyster aquaculture. Mar. Ecol. Prog. Ser. 673, 29–41. doi: 10.1016/j.aquaculture.2014.12.043
Ray N. E., Fulweiler R. W. (2020). Meta-analysis of oyster impacts on coastal biogeochemistry. Nat. Sustain. 4, 261–269. doi: 10.1038/s41893-020-00644-9
Ren L. H. (2014). Research on carbon sequestration of cultured oyster Crassostrea gigas and its fouling organisms in Sungo Bay. University of Chinese Academy of Sciences, 115.
Riesgo A., Taboada S., Kenny N. J., Santodomingo N., Moles J., Leiva C., et al. (2021). Recycling resources: silica of diatom frustules as a source for spicule building in antarctic siliceous demosponges. Zool. J. Linn. Soc 192, 259–276. doi: 10.1093/zoolinnean/zlaa058
Savchuk O. P. (2005). Resolving the Baltic Sea into seven sub-basins: N and P budgets for 1991–1999. J. Mar. Syst. 56, 1–15. doi: 10.1016/j.jmarsys.2004.08.005
Schneider O., Sereti V., Eding E. H., Verreth J. A. J. (2005). Analysis of nutrient flows in integrated intensive aquaculture systems. Aquacult. Eng. 32, 379–401. doi: 10.1016/j.aquaeng.2004.09.001
Shi H. H., Fang G. H., Hu L., Zheng W. (2011b). Analysis on response of pelagic ecosystem to kelp mariculture within coastal waters. J. Waterway. Harbor. 32, 213–218. doi: CNKI:SUN:SDGK.0.2011-03
Shi J., Wei H., Zhang L., Yuan Y., Fang J. G., Zhang J. H. (2011a). A physical-biological coupled aquaculture model for a suspended aquaculture area of China. Aquaculture 318, 412–424. doi: 10.1016/j.aquaculture.2011.05.048
Shpigel M. (2005) “Bivalves as biofilters and valuable byproducts in land-based aquaculture systems,” in The comparative roles of suspension feeders in ecosystems. Eds. Dame R. F., Olenin S. (Springer-Verlag, Dordrecht), NATO Science Series: Earth and Environmental Sciences, Netherlands, 183–197. doi: 10.1007/1-4020-3030-4_1
Sma R. F., Baggaley A. (1976). Rate of excretion of ammonia by the hard clam Mercenaria mercenaria and the American oyster Crassostrea virginica. Mar. Biol. 36, 251–258. doi: 10.1007/BF0038928
Sun X. X., Sun S., Zhao Z. X., Shen Z. L. (2011). Long-term changes in nutrient concentration and structure in the jiaozhou bay. Oceanol. Limno. Sin. 42 (5), 662–669. doi: CNKI:SUN:HYFZ.0.2011-05-0
Svenningsen N. B., Heisterkamp I. M., Sigby-Clausen M., Larsen L. H., Nielsen L. P., Stief P., et al. (2012). Shell biofilm nitrification and gut denitrification contribute to emission of nitrous oxide by the invasive freshwater mussel Dreissena polymorpha (Zebra mussel). Appl. Environ. Microbiol. 78, 4505–4509. doi: 10.1128/AEM.00401-12
Tande K. S., Slagstad D. (1985). Assimilation efficiency in herbivorous aquatic organisms-the potential of the ratio method using 14C and biogenic silica as markers. Limnol. Oceanogr. 30.
Tan K., Xu P., Huang L. H., Luo C., Huang J. M., Fazhan H., et al. (2024). Effects of bivalve aquaculture on plankton and benthic community. Sci. Total Environ. 914, 169892. doi: 10.1016/j.scitotenv.2024.16
Thorel M., Claquin P., Schapira M., Le Gendre R., Riou P., Goux D., et al. (2017). Nutrient ratios influence variability in Pseudo-nitzschia species diversity and particulate domoic acid production in the Bay of Seine (France). Harmful. Algae. 68, 192–205. doi: 10.1016/j.hal.2017.07.005
Tréguer P., Nelson D. M., van Bennekom A. J., DeMaster D. J., Leynaert A., Queguiner B. (1995). The silica balance in the world ocean: a reestimate. Science 268, 375–379. doi: 10.1126/science.268.5209.375
Tréguer P. J., Bowler C., Moriceau B., Dutkiewicz S., Gehlen M., Aumont O., et al. (2018). Influence of diatom diversity on the ocean biological carbon pump. Nat. Geosci. 11, 27–37. doi: 10.1038/s41561-017-0028-x
Tréguer P. J., de la Rocha C. L. (2013). The world ocean silica cycle. Ann. Rev. Mar. Sci. 5, 477–501. doi: 10.1146/annurev-marine-121211-172346
Tréguer P. J., Pondaven P. (2000). Silica control of carbon dioxide. Nature 406, 358–359. doi: 10.1038/35019236
Tréguer P. J., Sutton J. N., Brzezinski M., Charette M. A., Devries T., Dutkiewicz S., et al. (2021). Reviews and syntheses: the biogeochemical cycle of silicon in the modern ocean. Biogeosciences 18, 1269–1289. doi: 10.5194/bg-18-1269-2021
Troell M., Joyce A., Chopin T., Neori A., Buschmann A. H., Fang J. G. (2009). Ecological engineering in aquaculture-potential for integrated multi-trophic aquaculture (IMTA) in marine offshore systems. Aquaculture 297, 1–9. doi: 10.1016/j.aquaculture.2009.09.010
Turner R. E., Qureshi N., Rabalais N. N., Dortch Q., Justic D., Shaw R. F., et al. (1998). Fluctuating silicate: nitrate ratios and coastal plankton food webs. Proc. Natl. Acad. Sci. U.S.A. 95, 13048–13051. doi: 10.1073/pnas.95.22.1304
van Broekhoven W., Troost K., Jansen H., Smaal A. (2014). Nutrient regeneration by mussel Mytilus edulis spat assemblages in a macrotidal system. J. Sea. Res. 88, 36–46. doi: 10.1016/j.seares.2013.12.007
Wall C. C., Peterson B. J., Gobler C. J. (2008). Facilitation of seagrass Zostera marina productivity by suspension-feeding bivalves. Mar. Ecol. Prog. Ser. 357, 165–174. doi: 10.3354/meps07289
Wang L. (2010). Effects of the different maricultural models on sanggou bay ecosystem services (Qingdao: First Institute of Oceanography, Ministry of Natural Resources), 73pp.
Wang Y. J., Liu D. Y., Dong Z. J., Di B. P., Shen X. H. (2012). Temporal and spatial distributions of nutrients under the influence of human activities in sishili bay, northern yellow sea of china. Mar. pollut. Bull. 64 (12), 2708–2719. doi: 10.1016/j.marpolbul.2012
Wang Z., Zhao J., Zhang Y., Yu C. (2009). Phytoplankton community structure and environmental parameters in aquaculture areas of daya bay, south china sea. J. Environ. Sci. 21 (9), 1268–1275. doi: 10.1016/S1001-0742(08)62414-6
Wang J. J., Yu Z. M., Wei Q. S., Yao Q. Z. (2018). Long-term nutrient variations in the Bohai Sea over the past 40 years. J. Geophys. Res.-Oceans. 124, 703–722. doi: 10.1029/2018JC014765
Wang X. L., Du J. Z., Ji T., Wen T. Y., Liu S. M., Zhang J. (2014). An estimation of nutrient fluxes via submarine groundwater discharge into the Sanggou Bay—a typical multi-species culture ecosystem in China. Mar. Chem. 167, 113–122. doi: 10.1016/j.marchem.2014.07.002
Welsh D. T., Castadelli G. (2004). Bacterial nitrification activity directly associated with isolated benthic marine animals. Mar. Biol. 144, 1029–1037. doi: 10.1007/s00227-003-1252-z
Wen T. Y. (2013). Estimating submarine groundwater discharge via radon radioisotope: The case of sanggou bay and xiangshan, china (Shanghai: East China Normal University), 106pp.
Wu B., Liu S. M., Ren J. L. (2017). Dissolution kinetics of biogenic silica and tentative silicon balance in the Yellow Sea. Limnol. Oceanogr. 62, 1512–1525. doi: 10.1002/lno.10514
Wu B., Liu S. M. (2020). Dissolution kinetics of biogenic silica and the recalculated silicon balance of the east china sea. Sci. Total Environ. 743, 140552. doi: 10.1016/j.scitotenv.2020.140552
Xie L., Gao X. L., Liu Y. L., Yang B., Lv X. Q., Zhao J. M. (2021). Perpetual atmospheric dry deposition exacerbates the unbalance of dissolved inorganic nitrogen and phosphorus in coastal waters: A case study on a mariculture site in North China. Mar. pollut. Bull. 172, 112866. doi: 10.1016/j.marpolbul.2021.112866
Yang Q., Sun Y., Wang D. D., Xing L., Sun X. X., et al. (2010). Biogenic silica distributions in recent sediments of the east china sea and the huanghai sea and implications for productivity reconstructions. Acta Oceanol. Sin. 32 (3), 51–59. doi: 10253-4193(2010)03-0051-09
Yang S. R., Park M. G., Hong G. H., Chung C. S., Yang D. B. (1999). New and regenerated production in the Yellow Sea. In Hong G. H., Zhang J., Chung C. S. [eds.], Biogeochemical Processes in the Bohai and Yellow Sea. Seoul: The Dongjin Publication Association, 69-99.
Yin K. D., Harrison P. J., Goldblatt R. H. (1996). Spring bloom in the central strait of georgia: interactions of river discharge, winds and grazing. Mar. Ecol. Prog. Ser. 138, 255–263. doi: 10.3354/meps1
You L. P., Zhao Y. T., Sun S., Su B., Ma Y. Q., Wang L. M., et al. (2021). Characteristics of nutrient structures and limitations in Laizhou Bay in the spring and summer of 2018. Prog. Fish. Sci. 42, 15–24. doi: 10.19663/j.issn2095-9869.20200715001
Zeng D. Y., Huang D. J., Qiao X. D., He Y. Q., Zhang T. (2015). Effect of suspended kelp culture on water exchange as estimated by in situ current measurement in Sanggou Bay, China. J. Mar. Syst. 149, 14–24. doi: 10.1016/j.jmarsys.2015.04.002
Zhang J. H., Hansen P. K., Fang J. G., Wang W., Jiang Z. J. (2009). Assessment of the local environmental impact of intensive marine shellfish and seaweed farming-application of the MOM system in the Sungo Bay, China. Aquaculture 287, 304–310. doi: 10.1016/j.aquaculture.2008.10.008
Zhang J., Tishchenko P. Y., Jiang Z. J., Semkin P. Y., Tishchenko P. P., Zheng W., et al. (2023). Diverse nature of the seasonally coastal eutrophication dominated by oceanic nutrients: An ecosystem based analysis characterized by salmon migration and aquaculture. Mar. pollut. Bull. 193, 115150. doi: 10.1016/j.marpolbul.2023.115150
Zhang J. H., Fang J. G., Tang Q. S. (2005). The contribution of shellfish and seaweed mariculture in china to the carbon cycle of coastal ecosystem. Adv. Earth Sci. 20 (3), 359–365. doi: 1001-8166(2005)03-0
Zhang J. H., Wang W., Han T. T., Liu D. H., Fang J. G., Jiang Z. J., et al. (2012). The distributions of dissolved nutrients in spring of sungo bay and potential reason of outbreak of red tide. J. Fish. China 36 (1), 132–139. doi: 10.3724/SP.J.1231.2012.2
Zhao J., Zhou S. L., Sun Y., Fang J. G. (1996). Research on Sanggou bay aquaculture hydro-environment. Mar. Fish. Res. 17, 68–79.
Zhou Y., Mao Y. Z., Yang H. S., He Y. Z., Zhang F. S. (2002a). Clearance rate, ingestion rate and absorption efficiency of the scallop Chlamys farreri measured by in situ biodeposition method. Acta Ecol. Sin. 22, 1455–1462. doi: 10.3321/j.issn:1000-0933.2002.09.013
Keywords: silicon, nutrient, budget, biodeposition, aquaculture activity, Sanggou Bay
Citation: Li R, Jiang Z, Lin F, Zhang Y, Wang W, Zhang K, Li W, Wan D and Shi Y (2024) Silicon balance in an integrated multi-tropical aquaculture ecosystem, Sanggou Bay, China. Front. Mar. Sci. 11:1431878. doi: 10.3389/fmars.2024.1431878
Received: 13 May 2024; Accepted: 12 August 2024;
Published: 24 September 2024.
Edited by:
Martin F. Soto-Jimenez, National Autonomous University of Mexico, MexicoReviewed by:
Solomon Dan, Beibu Gulf University, ChinaJiaxing Liu, Chinese Academy of Sciences (CAS), China
Copyright © 2024 Li, Jiang, Lin, Zhang, Wang, Zhang, Li, Wan and Shi. This is an open-access article distributed under the terms of the Creative Commons Attribution License (CC BY). The use, distribution or reproduction in other forums is permitted, provided the original author(s) and the copyright owner(s) are credited and that the original publication in this journal is cited, in accordance with accepted academic practice. No use, distribution or reproduction is permitted which does not comply with these terms.
*Correspondence: Zengjie Jiang, amlhbmd6akB5c2ZyaS5hYy5jbg==