- 1Department of Fisheries, Wildlife, and Conservation Sciences, Oregon State University, Corvallis, OR, United States
- 2Coastal Oregon Marine Experiment Station, Oregon State University, Newport, OR, United States
Introduction: Plastics carried in the outflow of major rivers can be made available and subsequently ingested by marine fishes, causing lethal and sublethal effects. Highly abundant, vertically migrating myctophids play a crucial role in facilitating nutrient cycling between the epi- and mesopelagic zones. However, this diel movement may also make myctophids significant conduits for transporting ingested microparticles from surface waters to deeper food webs.
Methods: We examined the gastrointestinal tracts of 340 myctophids caught at varying distances from the Columbia River mouth in the epipelagic zone of the northeast Pacific Ocean to determine if proximity to a presumed point source influences microparticle ingestion.
Results: While we found no direct spatial connection with ingestion frequency, we discovered that (a) ~34% of myctophids had either synthetic or other anthropogenic particles retained in their GI tract, (b) microparticle ingestion was higher in an active-feeding species of myctophid (Tarletonbeania crenularis) than an inactive-feeding species (Stenobrachius leucopsarus), and (c) species and standard length were the most influential predictors of microparticle consumption in our best fit model.
Discussion: Our failure to detect a significant relationship between distance from a source and ingestion by myctophids is likely due to the particles undergoing fluctuations in dispersal patterns once they enter the ocean, particularly for microfibers which can be transported across large distances. Biological factors like body size may be more relevant to understanding microparticle ingestion patterns in mesopelagic fishes. Overall, our study highlights the potential role myctophids serve as multidirectional transporters of microparticles in Northern California Current food webs, with potential impacts on fisheries and human food systems.
1 Introduction
Though manufacturers and society prize plastic polymers and other chemically-enhanced materials for their versatility and durability, those same properties also lead to environmental persistence and accumulation (Boucher and Friot, 2017; Chen et al., 2021). Of the 353 million metric tons of plastic waste generated in 2019, 22 million metric tons have leaked from the waste management system into terrestrial and marine environments (OECD, 2022). The fragmentation of these plastic items into particles <5 mm, called microplastics, jeopardizes aquatic organisms through artificial satiation, reduced fertility from leached chemicals, and other mechanisms (Teuten et al., 2009; Lusher, 2015; Collard et al., 2017; Botterell et al., 2019; Gassel and Rochman, 2019). Natural polymers that have been treated with chemical additives can have similar effects on organisms when consumed, and thus we will use ‘microparticle’ to include both microplastics and anthropogenically modified materials (Miller et al., 2021; Lasdin et al., 2023; Torres et al., 2023). For the purpose of this work, we will adopt this definition as it reflects our focus on understanding the potential impacts of these particles on marine organisms.
Mismanaged plastic debris often pollute riverine systems (Jambeck et al., 2015; Meijer et al., 2021; Talbot et al., 2022), where physical abrasion and ultraviolet light exposure can exacerbate fragmentation (Derraik, 2002; Takada and Karapanagioti, 2019). As water flows through urban and agricultural areas, it can transport microparticles hundreds of kilometers from inland sources until ultimately reaching the ocean, dispersing offshore with the river plume and mixed by ocean currents (Hickey and Banas, 2003; Lebreton et al., 2017; Lebreton and Andrady, 2019). Atmospheric circulation can also carry and deposit plastic debris far offshore, which is especially evident for microfibers (Brahney et al., 2021).
Traversing 2,040 kilometers through parts of Canada and seven U.S. states (Lasmanis, 1991), the Columbia River empties more water (2,500 to 30,000 m3 s-1 depending on the season; Hickey and Banas, 2003) into the Pacific Ocean than any other river in North or South America. This river has the potential to carry plastic waste generated by the more than 8 million people that live in its drainage basin to the marine environment. Valine et al. (2020) found the microfiber density in the main stem of the Columbia River to be 0.225 microfibers m-3, although Kapp and Yeatman (2018) found it to be much higher (3 microplastics m-3) where the river mixes with the ocean. In the Clackamas River, a tributary of the Columbia River, Talbot et al. (2022) found mostly dark-colored fragments and fibers across their study region. Strong, seasonal upwelling events characteristic of Oregon’s coastal waters can likely influence the spread and distribution of microplastic particles as they enter the ocean from the Columbia River (Liu et al., 2009). This, combined with the river plume transport of less-dense fresh water along the surface of the ocean, makes them broadly available to fishes farther offshore. Black Rockfish caught in Oregon’s nearshore environment contained on average 7.31 microparticles per fish, indicating that microplastics are entering marine food webs (Lasdin et al., 2023).
Lanternfishes, or myctophids, constitute 60% of deep-sea biomass (Watanabe et al., 1999; Mensinger, 2011) and play a vital role in the export of carbon produced in surface waters to the deeper ocean (Davison et al., 2013). Each evening, massive numbers of myctophids undergo a diel vertical migration from the mesopelagic zone to the ocean’s surface in search of prey (Catul et al., 2011; Choy et al., 2019). Myctophids feed on zooplankton and in turn, serve as essential forage fish for marine mammals, seabirds, and commercially important fish species (Payne et al., 1987; Carbery et al., 2018), including many predators in the California Current ecosystem (Iglesias et al., 2023). By foraging in the epipelagic zone, myctophids may be consuming more buoyant microparticles either directly during surface feeding events or indirectly through contaminated zooplankton (Figure 1; Lusher et al., 2016; Hasegawa and Nakaoka, 2021). Therefore, the microplastic load in myctophids should be evaluated as they have the potential to transport surface microparticles throughout mesopelagic food webs (Carbery et al., 2018).
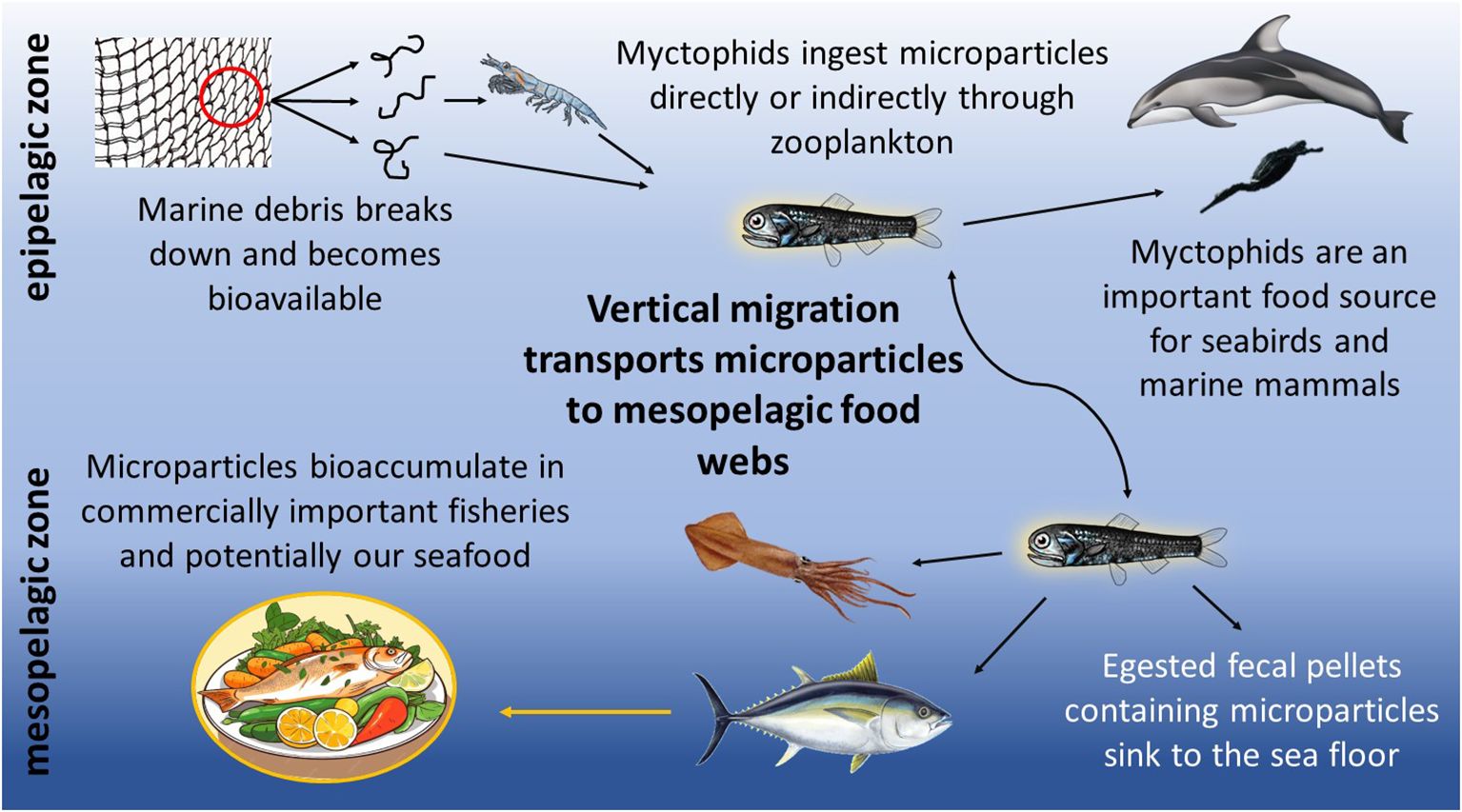
Figure 1. Proposed pathways for the biotransport of microparticles by myctophids to the mesopelagic food web.
Myctophids caught in gyres where plastic debris is known to accumulate are confirmed to consume microplastics (Boerger et al., 2010; Eriksen et al., 2013; Lusher et al., 2016; Wieczorek et al., 2018; McGoran et al., 2021; Justino et al., 2022). Building on this knowledge, our goal was to assess the presence of microparticles in coastal myctophids in the Northern California Current, which we use to provide a spatial context for the influence that distance to a major river mouth might have on the accumulation of microparticles in the digestive tracts of mesopelagic fishes. We hypothesized that myctophids caught closest to the Columbia River mouth would contain the highest amounts of microparticles.
2 Materials and methods
2.1 Sample collection
The NOAA Northwest Fisheries Science Center collected fishes during their Stock Assessment Improvement Plan (SAIP; Phillips et al., 2009) from May to September of 2005 through 2009. Four transects (Figure 2; Willapa Bay [46.67°N], Columbia River [46.16°N], Newport Hydrographic line [45.65°N], and Heceta Head [44.00°N]) with stations extending laterally offshore along the Oregon and Washington coasts, were sampled at the 30 to 42 m depth stratum at night with a Nordic 264 rope (Table 1). Three species of myctophids (Tarletonbeania crenularis, Diaphus theta, and Stenobrachius leucopsarus) were caught at eleven of the twenty SAIP stations. They were initially frozen and later stored in 70% ethanol. Many of the specimens were used for a diet analysis (Suntsov and Brodeur, 2008), and the remainder were archived at the Hatfield Marine Science Center in Newport, Oregon until being used for this project. More detailed sampling methods can be found in Suntsov and Brodeur (2008) and Phillips et al. (2009).
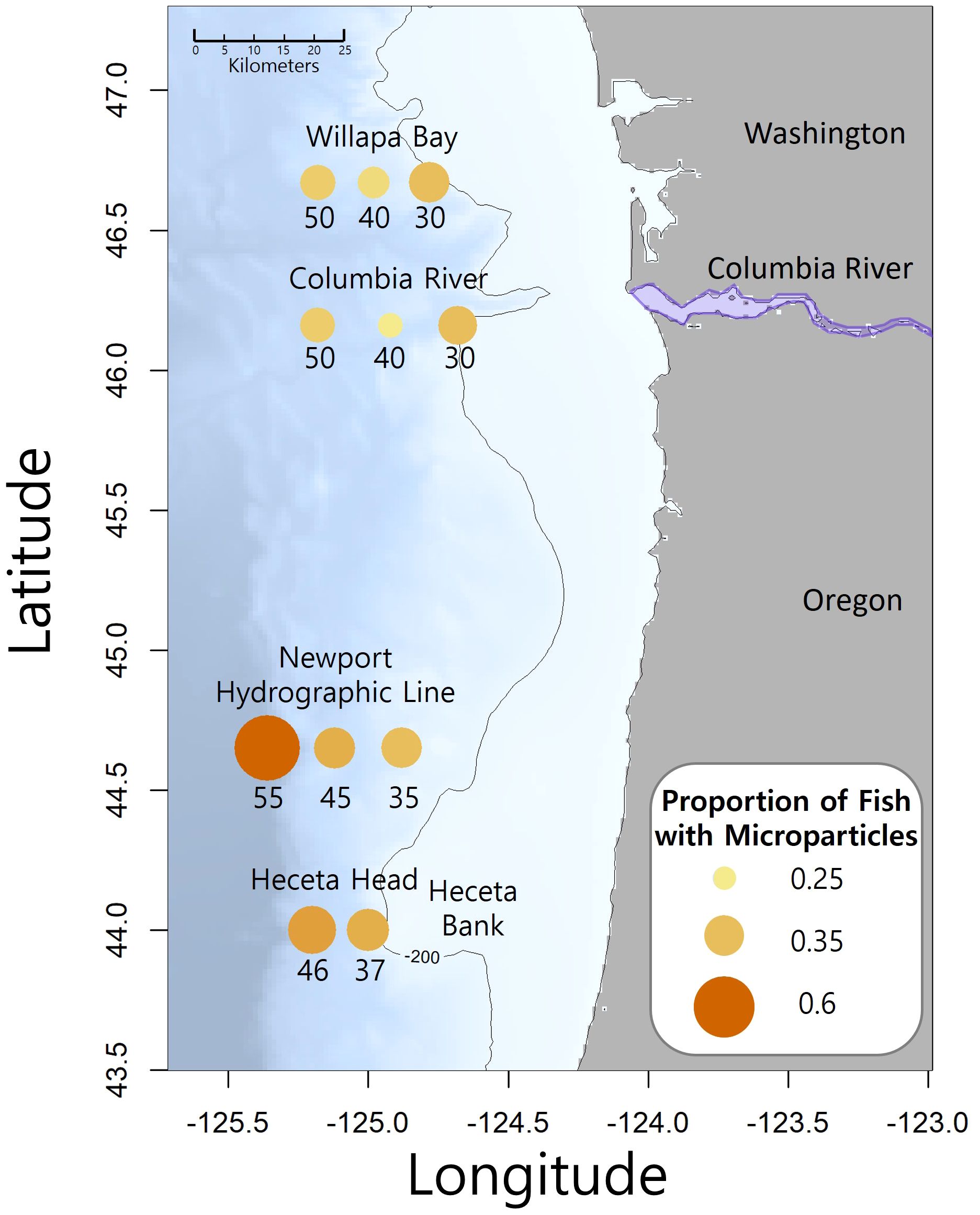
Figure 2. Map of the study area with transect names and sampling station number is the approximate nautical miles offshore. The Columbia River divides Washington and Oregon. The size and color intensity of the circles at each station represents the proportion of fish that contained microparticles. The contour line is 200 meters depth and the location of Heceta Bank is identified.
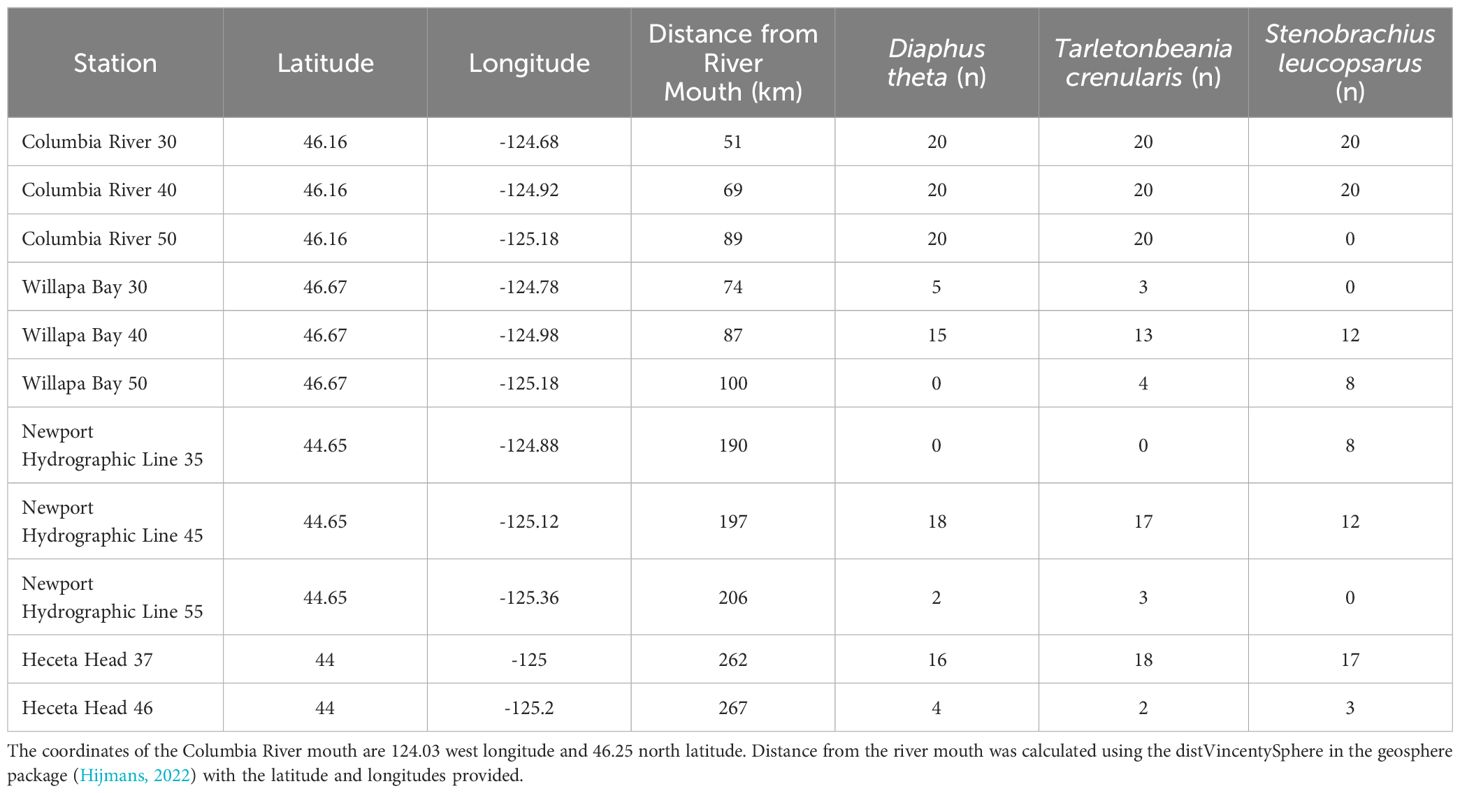
Table 1. Stations and their associated latitude, longitude, distance from the Columbia River mouth, and samples sizes for each species.
The three species used in this study are commonly found from the Bering Sea and the Gulf of Alaska to Southern California, and generally comprise the majority of myctophids collected in the Transition Zone of the Northern California Current (Brodeur et al., 2003). In our study, Diaphus theta are smaller on average (52.1 ± 8.7 mm) than Stenobrachius leucopsarus (59.2 ± 8.8 mm) and Tarletonbeania crenularis (59.7 ± 9.6 mm). Diaphus theta and T. crenularis are considered fully-migratory, residing at depths of 300 to 500 m during the day, and 0 to 100 m at night. S. leucopsarus have been found from 30 m (or less) to much deeper at 3,824 m (Love and Passarelli, 2020). This species is considered a semi-migrant as a portion of the population migrates to the surface at night (20 to 200 m), while others do not migrate and remain at the daytime depth (400 to 700 m) (Watanabe et al., 1999). Additionally, Suntsov and Brodeur (2008) categorized D. theta and T. crenularis, as ‘active’ feeders in their diet study on other SAIP myctophids, noting their preference for protein-rich euphausiids and hyperiid amphipods. In contrast, they described S. leucopsarus as an ‘inactive’ feeder that consumes slower, lipid-rich copepods.
Each myctophid was identified to species using photophore patterns described in Love and Passarelli (2020). Twenty adults from each of these three species were selected from the SAIP collection for Columbia River stations 30, 40, and 50 (with the exception that no Stenobrachius leucopsarus were preserved from CR50). Because of low sample sizes, species were combined across stations for Heceta Head, Willapa Bay, and Newport Hydrographic line transects.
2.2 Dissection and digestion
Myctophids were processed under a laminar flow hood (Erlab Captairflow, Rowley, Massachusetts) with a high-efficiency particulate absorbing filter (0.3 μm pore size) to prevent airborne particle contamination (Brander et al., 2020). Each myctophid was thoroughly rinsed with filtered (1 μm pore size) reverse osmosis water, weighed to the nearest 0.1 g, and measured prior to dissection. Total length, standard length, and body depth (at the widest point) were measured to the nearest mm. To remove the gastrointestinal (GI) tract, an incision was made from the vent to the base of the jaw, and then the GI was cut at the anus and esophagus. The GI tract was weighed to the nearest 0.0001 g and was placed in a glass scintillation vial with 5 mL of 20% filtered potassium hydroxide (KOH; made using Sigma-Aldrich >86% pellets and reverse osmosis water, 1 μm pore size) to chemically digest the organic matter. If 5 mL did not cover the GI tract, additional 20% KOH solution was added until all material was suspended. The samples were incubated at room temperature (approximately 20°C) and lightly agitated once a day until the solid material was fully liquified (Brander et al., 2020; Lasdin et al., 2023). Liquification required two to seven days.
2.3 Filtration and filter picking
The contents of each vial were vacuum filtered through 5 μm Sterlitech polycarbonate track etch membrane filters using a fritted Büchner funnel setup. These filters were inspected under a stereoscope and any particles present were removed prior to use. The vials were rinsed three times with filtered reverse osmosis water, which we then poured into the glass funnel to flush any remaining sample material onto the filter. The sides of the funnel were then rinsed down with filtered reverse osmosis water before the filter was removed from the apparatus and placed in a covered dish.
The filters were examined under a stereomicroscope (Leica EZ4 fitted with a Moticam 3+; 16x magnification) in a flow hood for any particles that appeared to be anthropogenic in origin. These putative particles were classified by their color and morphology (fiber, film, or fragment). Using Motic Images Plus 3.0(x64) software, particles were photographed and measured to the nearest 0.01 mm. Each particle was removed and placed onto a microscope slide with a coverslip for later spectroscopic analysis.
2.4 QA/QC measures
QA/QC protocols followed recommendations and protocols implemented in Brander et al. (2020) and Lasdin et al. (2023), respectively. Briefly, the interior surfaces of the laminar flow hood and any items entering the hood were wiped down with Kimwipes and filtered ethanol (1 μm pore size) to remove any settled particles. The glassware was thoroughly scrubbed with Alconox and rinsed five times with deionized water before being covered with aluminum foil and baked for four hours at 450°C in a muffle furnace to incinerate any potential microparticles or fibers. Orange cotton lab coats were worn throughout tissue processing and filter picking, and particles similar in appearance to those of the lab coats were not included in analyses.
Air (n = 13) and KOH (n = 9) controls were taken to account for background contamination during the dissection, filtration, and filter picking processes (Brander et al., 2020). A wetted Whatman filter was left uncovered for the duration that samples were exposed to the air. KOH procedural blanks were taken in a similar manner as the myctophid samples, where 5 mL of KOH was poured through a filter as if it contained GI tract material. The Whatman filter and the KOH filters were examined for particles with the stereomicroscope after each batch and the particles were classified, measured, and spectroscopy was performed. The plastics found in the background controls (air blanks, procedural blanks) were totaled across the study (n = 34) and divided by the number of samples processed (n = 340), resulting in a background contamination level of 0.10 particles per myctophid.
Additionally, a spiked-recovery test was performed similar to Budimir et al. (2018) and Dawson et al. (2020) to determine the microplastic recovery levels using these methods. Ten myctophid GI tracts were spiked with a random quantity (1–10 particles) of green polypropylene fibers ranging from 0.7–1.3 mm in length. These were then digested and filtered before being picked. The recovery rate for the particles was 78.6%.
2.5 Micro-Fourier Transform Infrared (μFTIR) spectroscopy
The chemical composition of suspected microplastic particles from the samples and controls were verified via Micro-Fourier Transform Infrared (μFTIR) spectroscopy. If more than three particles of the same classification were found (ex. black fiber, clear fragment, etc.), a 30% subset from the group was selected for spectroscopy. Approximately 40% of particles were analyzed.
The Thermo Fisher Scientific Nicolet iN5 μFTIR measures attenuated total reflectance, which is a sampling technique that uses a crystal with a high refractive index and IR transmission to measure the absorbance of the material being tested (Larkin, 2011). We used a germanium crystal (wavenumber range 5000–850 and refractive index 4.0) with a fine tip and an aluminum foil-covered slide to run our samples. The infrared spectra generated were smoothed for atmospheric suppression and compared against the OMNIC library of known materials. The OMNIC software produces a correlation-based match value and a list of the top ten closest matches. Only spectra with greater than a 70% match in OMNIC were considered as a positive identification per protocols described in Kapp and Yeatman (2018); Harris et al. (2022), and Talbot et al. (2022).
Open Specy is an open source, online tool with an extensive library of known spectra from microparticles, including weathered and treated microparticles (Cowger et al., 2021). Smoothing (Savitzky−Golay filter) and baseline correction features (ImodPolyFit) were applied to all spectra saved from OMNIC. A list of the top five materials with their percent match values were generated in Open Specy with matches >80% being accepted. The highest matches from OMNIC and Open Specy were compared to determine the final reported material type (Supplementary Figure 1; Supplementary Table 1) (Harris et al., 2022; Talbot et al., 2022; Lasdin et al., 2023).
2.6 Analysis and statistics
All statistical analyses were performed using R v.4.3.1. Chi-squared tests were used to identify differences in the characteristics (color, morphology, and composition) of the particles recovered from the myctophids from the particles on the air controls. Lengths of the particles were compared with a one-way Analysis of Variance (ANOVA). The goal of these tests were to determine if the microparticles detected in the myctophids originated from what was ingested rather than being a result of air contamination. A one-way ANOVA with a Tukey’s post hoc test was performed to compare the standard lengths between species.
The response variable in our study represents the binary outcome, or frequency of occurrence (Ferreira et al., 2023), of presence (‘1’) or absence (‘0’) of microparticles in the GI tract. We decided against using quantities because few GI tracts contained more than one particle and in cases where multiple were found, there was evidence suggesting that some of these may have been the result of a single microparticle item being fragmented during extraction. A generalized linear model (GLM) with a binomial distribution and a logit link function was used to determine if distance from the Columbia River mouth (km) and species are associated with the probability of microparticle presence in the GI tract. A deviance goodness-of-fit test was used to evaluate the fit of the GLM and determine how well the predictor variables explain the observed variability.
To explore additional environmental and biological variables that could be better predictors of microparticle ingestion, we conducted model selection using Akaike information criterion (AIC). We used the dredge function in the MuMIn package (Bartoń, 2023) to compare all possible combinations of explanatory variables derived from the global model. Models with lower AIC values were prioritized, balancing the fit of the model with complexity. Oceanographic explanatory variables included temperature, salinity, and turbidity measured by a CTD profiler deployed concurrently with the trawl. We averaged these measurements across the 5 m, 20 m, and 30 m depth layers. The flow rate (cubic feet per second) of the Columbia River at the time of the myctophid collection event was also included in the model (USGS, 2024). Additionally, myctophid species and standard length (mm) in the model allows for the examination of species-specific and size-dependent factors that may influence microparticle consumption.
The global model was tested for collinearity among the predictor variables using the Variance Inflation Factor calculations performed by the vif() function in the car package (Fox and Weisberg, 2019). Overdispersion in the data was tested using the ratio of the Pearson chi-square statistic to its degrees of freedom. There was no evidence of collinearity or overdispersion.
3 Results
3.1 Particle summary
A total of 142 suspected microplastic particles ranging in size from 0.01 to 9.04 mm were found in the 340 myctophids (120 Tarletonbeania crenularis, 120 Diaphus theta, and 100 Stenobrachius leucopsarus). Of these myctophids, 34.1% had particles that appeared anthropogenic in origin (n = 116 with one or more microparticles, and n = 224 without microparticles), with only 21 fish containing more than one particle. There was a mean abundance of ~0.42 ± 0.66 particles per fish, consisting mostly of fibers (76%) and filaments (11%; Figure 3). Blue (44%), black (27%), and clear (20%) were the most common particle colors found. Of the subset of particles from the myctophids analyzed with the μFTIR, approximately 73% were confirmed to be anthropogenically modified cellulose (i.e., dyed or treated cotton). The rest include polyethylene terephthalate, polypropylene, polyamide, acrylic paint, and synthetic unknown polymers that had different materials listed as top matches in OMNIC and in Open Specy (Supplementary Figure 1; Supplementary Table 1).
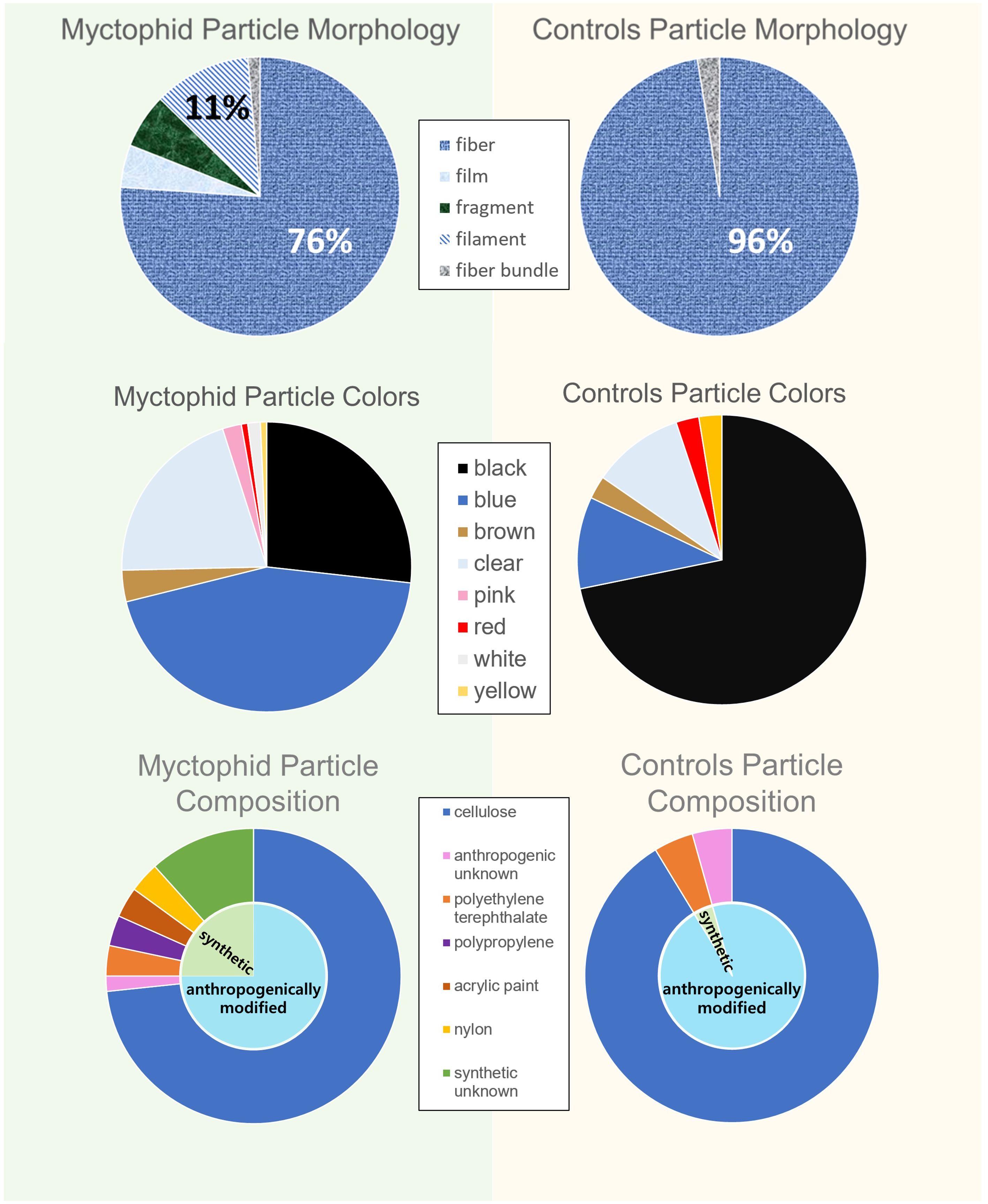
Figure 3. Particle breakdown between the myctophids and controls. The morphologies, colors, and particle compositions were more varied in the myctophids than the controls.
The average background contamination level, calculated from the control samples, was 0.10 particles per sample (Figure 4). While the myctophids contained a significantly more diverse array of microparticle morphologies and colors (p < 0.05), the controls consisted only of fibers, with predominantly black microparticles (72%). The average size of the particles on the controls were also larger than what was found in the myctophids (p < 0.05). The composition of the particles in the myctophids and the controls did not differ significantly (p > 0.05) as most of the particles in the controls were also anthropogenically modified cellulose. The statistical differences in colors, morphologies, and sizes of particles between the myctophids and the controls suggest that the particles originate from within the myctophids rather than contamination from the processing.
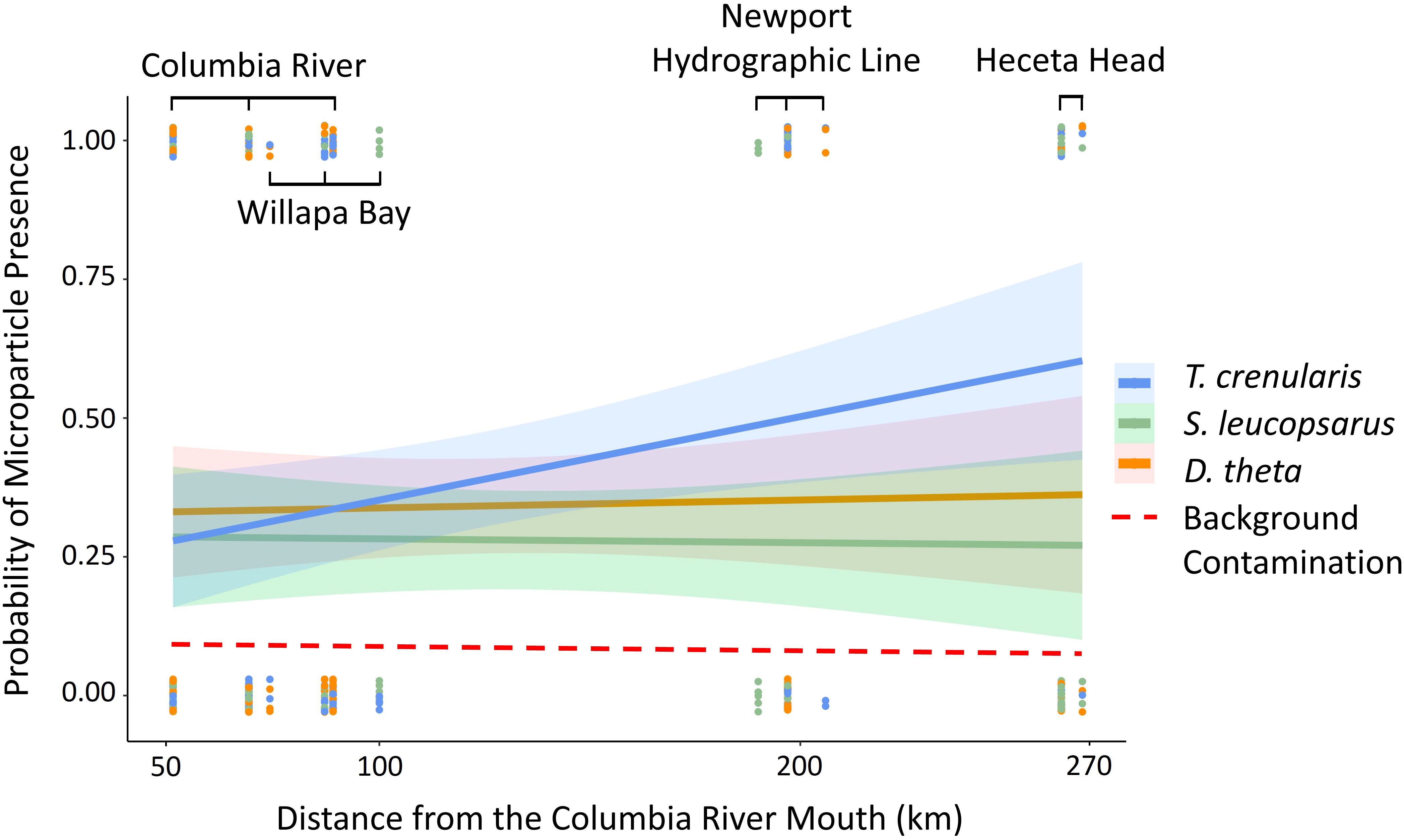
Figure 4. The regression slopes from the GLM with a binomial distribution of each species (Tarletonbeania crenularis, Stenobrachius leucopsarus, and Diaphus theta) represents the probability of microparticle presence in the GI tract across distance from the Columbia River mouth (km). The dashed line is the average background contamination calculated as 0.10 from our air and procedural controls.
3.2 Species differences
While the three species of myctophids were similar in wet weight (Diaphus theta = 2.1 ± 1.1 g, Stenobrachius leucopsarus = 2.2 ± 1.0 g, and Tarletonbeania crenularis = 2.4 ± 1.1 g), the D. theta in our study were significantly shorter than the other two species (one-way ANOVA with a Tukey’s post hoc test; p < 0.05). Approximately 34.2% of D. theta, 28.0% of S. leucopsarus, and 39.2% of T. crenularis had consumed microparticles. In a GLM with distance from the river mouth and species as the predictor variables, T. crenularis was significantly more likely to consume microparticles (p < 0.05) than S. leucopsarus when controlling for distance. There was not a significant difference in ingestion levels between either S. leucopsarus or T. crenularis and D. theta.
3.3 Predictors of microparticle ingestion
Contrary to our hypothesis, distance from the Columbia River mouth was not associated with microparticle presence in the GI tract (p > 0.05). The global model included the oceanographic and biological variables (temperature, salinity, turbidity, species, and myctophid standard length) as well as distance from the river mouth and the river flow rate. When holding the other variables constant, the species and standard length were significant predictor variables (p < 0.05). The best model based on the lowest AIC value also included just species and standard length (Table 2; global model AIC = 414.9 and the reduced model AIC = 410.68). There was no significant relationship between microparticle ingestion and the oceanographic variables (Figure 5).
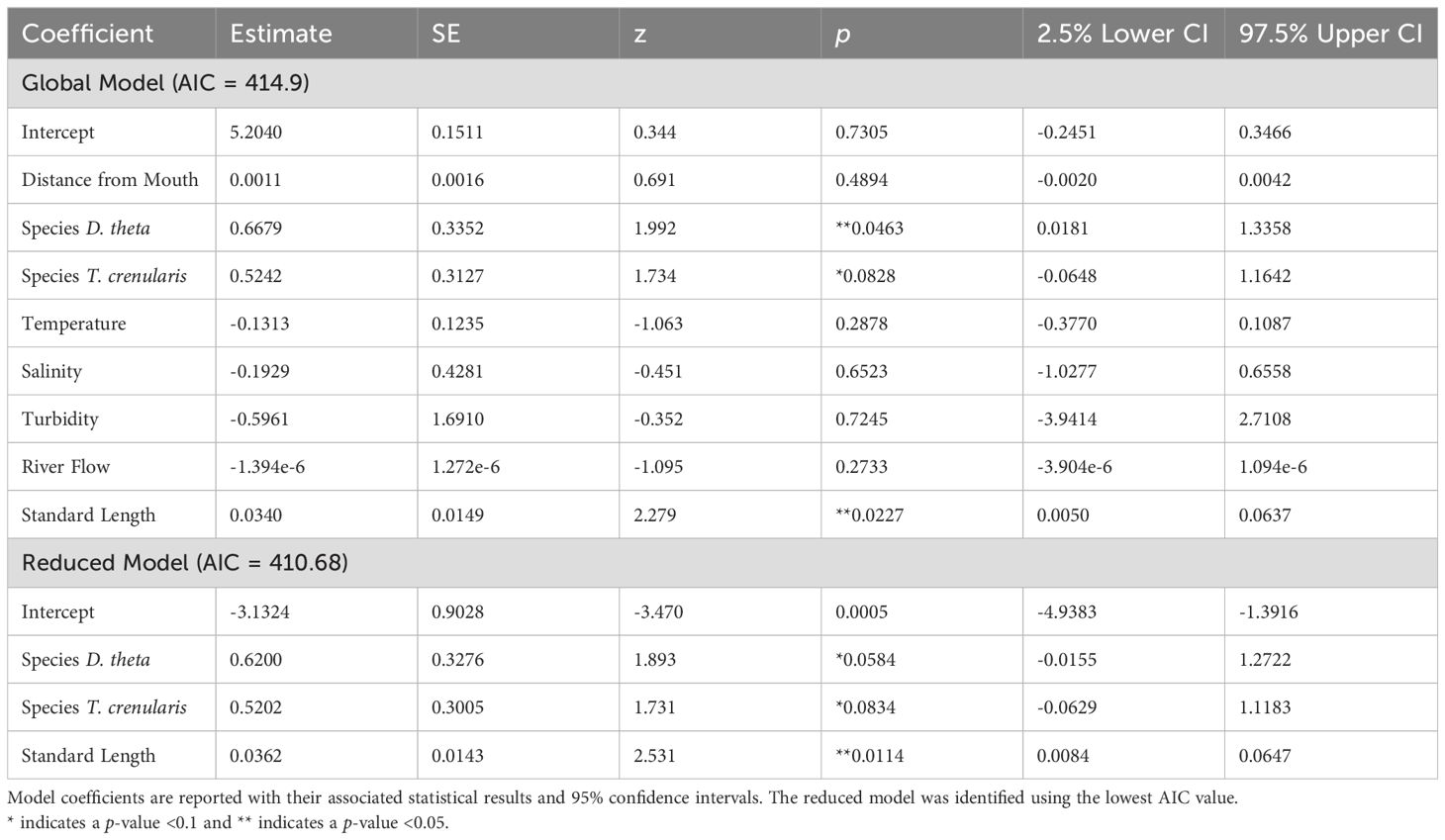
Table 2. GLM results for the presence of microparticles in the GI tract against the variables in the global and reduced models.
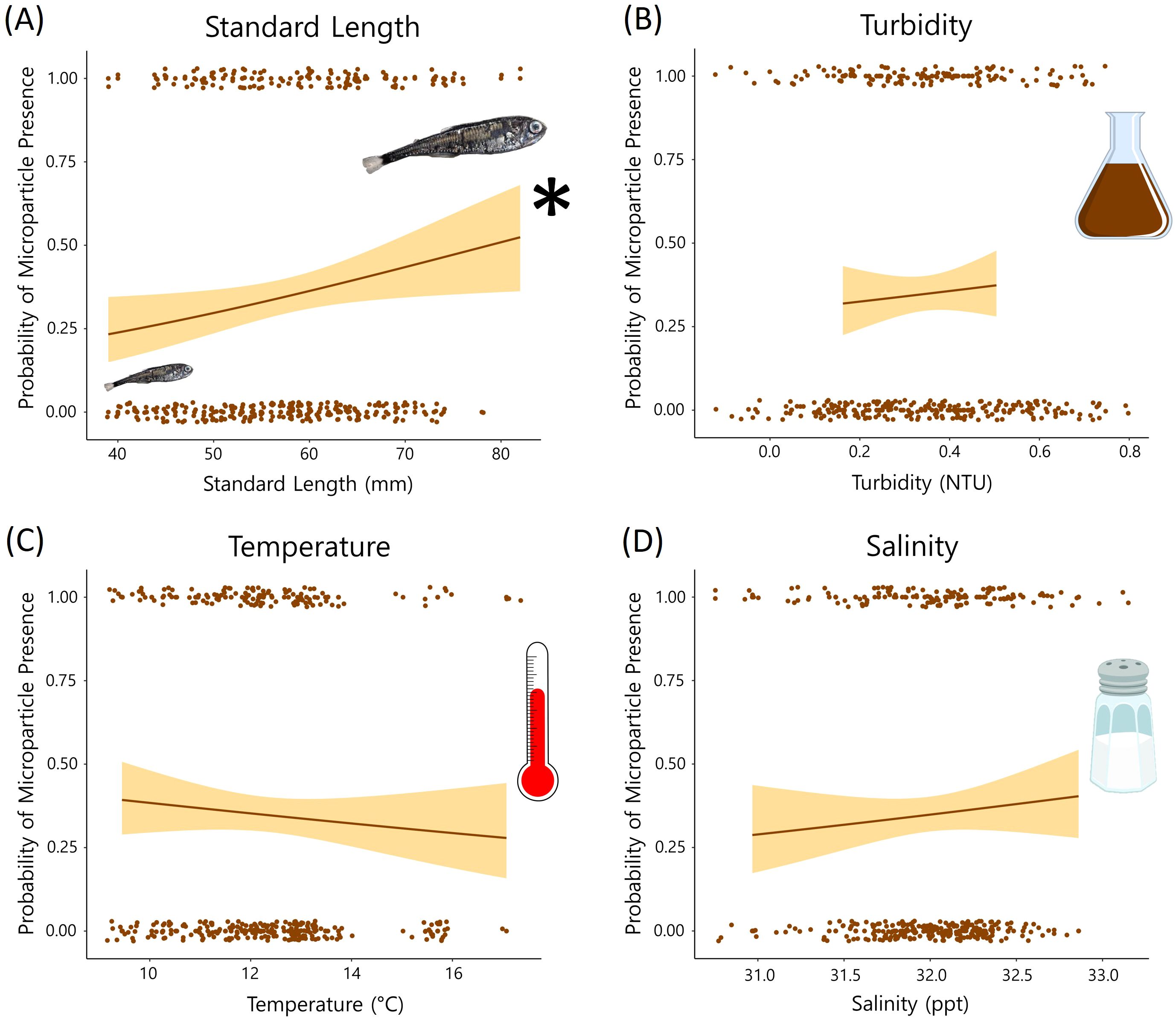
Figure 5. Results from the oceanographic variables in our global GLM. The probability of microparticle presence in the GI tract is plotted against each variable [(A) standard length, (B) turbidity, (C) temperature, and (D) salinity]. Standard length (A) was a significant (p < 0.05) predictor of microparticle ingestion. * symbol indicates that Standard length (A) was a significant (p < 0.05) predictor of microplastic ingestion.
4 Discussion
This study is the first to examine the influence that proximity to a potential point source of microplastic pollution has on microparticle presence and abundance in myctophids. We confirmed that myctophids in the Northeast Pacific are consuming microplastics and semi-synthetic microparticles, corroborating the conjecture that myctophids have the potential to transport considerable quantities of anthropogenic materials to deep sea food webs (Lusher et al., 2016; Savoca et al., 2021; Justino et al., 2022). Gaining insights into the factors that drive microplastic consumption is crucial for identifying vulnerable locations and species, as well as for directing remediation efforts.
The particle characteristics found in this study align with previous findings in myctophids and marine organisms broadly, although the percentage of myctophids containing particles is lower than what has been observed in other fish species (Pazos et al., 2017; Rodrigues et al., 2019; Lasdin et al., 2023). Our findings revealed that 34.1% of the myctophids examined contained one or more particles, a proportion closely resembling the observation made by Boerger et al. (2010) in the North Pacific Central Gyre, where they reported a 35% occurrence. It is worth noting that the myctophids we studied were collected during the same timeframe as Boerger’s 2008 investigation (Boerger et al., 2010). Of the Black Rockfish (Sebastes melanops) examined in Lasdin et al. (2023), 93% were found to have ingested microparticles. These rockfish were also collected off the Oregon coast, however most sites were much closer to shore (<5 km). Black Rockfish prefer different prey than myctophids, including primarily Cancer magister megalopae, mysids, and juvenile fishes (Doran, 2020). Collection location, diet, and size differences could explain the elevated microplastic levels in rockfish compared to myctophids. Based on our spiked-recovery test, however, we may not be recovering all of the particles with our methods as about 20% of the spiked particles were not recovered (Budimir et al., 2018; Dawson et al., 2020; Way et al., 2022). A correction factor could be applied, but here we report raw data.
The μFTIR analysis confirmed that the myctophids had consumed plastics, including polyethylene terephthalate, polypropylene, polyamide, and acrylic paint. Lasdin et al. (2023) found these materials and others, such as polyurethane and acrylonitrile butadiene styrene, in Oregon rockfish. While most other studies on myctophids did not spectrally identify the particles consumed, Ferreira et al. (2023) had similar proportions of the different polymers. Interestingly, more high-density plastics (polyethylene terephthalate, polyamide, acrylic) were found in both studies. Ferreira et al. (2023) postulates that this is due to myctophids being more susceptible to particles with faster sinking rates.
Microfibers were the most common in our samples, specifically blue and black microfibers. This phenomenon has been observed in organisms ranging from marine mammals and turtles (Pietroluongo et al., 2022) to invertebrates such as mussels (Pérez et al., 2020). Boucher and Friot (2017) estimated that two million tons of these microfibers enter the ocean each year and suggested that this morphological subset of microparticles is the most common in the environment.
McGoran et al. (2021)‘s study of South Atlantic myctophids corroborates our findings that myctophids mostly contained anthropogenically altered cellulose fibers. About half of the microfibers generated by the textile industry are modified cellulose (El-Nemr, 2012) and cotton garments also tend to shed more than some synthetic fibers (Athey et al., 2022; Granek et al., 2022). While these particles are not plastic polymers, they are treated with dyes and chemicals which make them persistent and potentially toxic in similar ways as plastics when ingested. Raman spectroscopy identified that cellulose fibers extracted from wild amphipods were dyed with Direct Red 28, a carcinogen for invertebrates (Remy et al., 2015). Additionally, toxicity testing by Siddiqui et al. (2023) found that Inland Silversides exposed to cotton fibers had altered behavior and mysid shrimp experienced reduced growth.
Additionally, the microparticles extracted from the specimens were on average 0.96 mm in length; much smaller than the euphausiids, amphipods, and copepods that they typically prey upon in the Northern California Current (Bosley et al., 2004; Suntsov and Brodeur, 2008). This suggests that myctophids are not targeting microparticles, but rather are either accidentally ingesting them or are consuming contaminated zooplankton. Desforges et al. (2015) reported that Euphausia pacifica, the primary prey of Tarletonbeania crenularis and Diaphus theta (Suntsov and Brodeur, 2008), contained particles similar in size to ours (~0.82 ± 0.11 mm). Therefore, trophic transfer from their filter-feeding prey is the likely route of ingestion of these particles by myctophids, as been seen in a number of previous studies (Desforges et al., 2015; Athey et al., 2020; Hasegawa and Nakaoka, 2021; Uy and Johnson, 2022).
4.1 Species assessment
We compared microparticle presence between three species of myctophids that occupy similar niches off the Oregon and Washington coasts. We found that the active-feeding Tarletonbeania crenularis contained significantly more microparticles than the inactive-feeding Stenobrachius leucopsarus, with T. crenularis preferentially feeding on euphausiids, while S. leucopsarus was consuming mostly Neocalanus copepods (Suntsov and Brodeur, 2008). Desforges et al. (2015) found that one in 34 copepods contained microplastics, whereas the incidence level in euphausiids was twice as high, with one in 17 containing microplastics. Given that microparticles likely enter the myctophid diet through their prey, the higher microparticle content in T. crenularis aligns with its dietary selection for euphausiids, indicating a direct link between feeding behavior and microparticle ingestion levels in these species.
4.2 Model predictors
Though our study did not find a direct relationship between proximity to the Columbia River mouth and microparticle ingestion, our results indicate that areas of particle retention may hold greater significance than mere distance from a point source. During the summer, prevailing winds cause the Columbia River plume to move southward (Burla et al., 2010), toward our more southern lines (Figure 2). Eddies then form when this water mass meets the shallow, rocky shelf at Heceta Bank (Barth et al., 2005). This area is a known larval fish and invertebrate retention area (Palumbi et al., 2003) and may also trap drifting microparticles. This is also a location where we saw higher probability of microparticle presence in our myctophids. We likely see a higher microparticles signature in those lower transects as plastics leaving the Columbia River travel south and concentrate in the Heceta Bank region in the same manner that larval fish are concentrated by oceanographic processes.
Of the other predictor variables in our GLM, standard length had the strongest, positive relationship with microparticle consumption (Table 2). For each millimeter increase in length the myctophids in our study were 1.25% more likely to contain microparticles. While this is not observed in all studies on fishes (De Vries et al., 2020), many researchers make similar observations that microplastic presence increases with fish size (Alomar et al., 2017; McNeish et al., 2018). Generally, larger individuals of the same species tend to be older and have increased energetic needs, which may make them more likely to have recently consumed or retained microparticles due to their longer exposure time and higher intake rates. However, variability could stem from a range of complex factors that are challenging to pinpoint, such as differences in diet, potential impacts of bioaccumulation and biomagnification, or the fish’s ability to eliminate the ingested particles (Carbery et al., 2018; Bernal et al., 2020; Okamoto et al., 2022).
When controlling for the spatial and biological variables, the oceanographic predictors did not have a strong relationship with microparticle ingestion. Temperature and salinity can affect the buoyancy of microparticles and both have been shown to have a positive influence on microplastic consumption by fishes (Buwono et al., 2021; Zhou et al., 2021; Wang et al., 2022). Water turbidity also correlates with the distribution of microparticles in the water column and in some cases, microplastic ingestion increases with turbidity (Buwono et al., 2021; Zhou et al., 2021). Although there are documented associations between the oceanographic predictors and microplastic ingestion, our inability to detect significant relationships is possibly attributed to the less pronounced halo- and thermoclines observed at our offshore sampling locations.
4.3 Implications for fisheries
The global biomass of myctophids is estimated to be 1 to 10 gigatons (Kaartvedt et al., 2012; Irigoien et al., 2014). Currently the fishery is underexploited, with the world harvest remaining below 20,000 metric tons annually due to undesirability with myctophid’s small size and high wax content, and ineffective fishing gear (Remesan et al., 2019). However, the escalating demand for fishmeal in aquaculture is driving consideration toward the exploitation of mesopelagic fishes as a potential solution to meet this demand (Valinassab et al., 2007). Fishmeal is made by grinding up whole fish, pressing out the oil and water, and cooking the remaining solids to then be pressed into pellets (Boyd, 2015), meaning the microparticles contained in the GI tracts of these fish can be directly fed to fish raised for human consumption. Microplastics have been observed within fish fillets, suggesting translocation through the GI lining and into edible tissue is probable (Collard et al., 2017; McIlwraith et al., 2021). Therefore, the microparticles consumed by myctophids could be more readily incorporated into the human food system than assumed. Soy feed is presumed to be the microplastic-free alternative, however Walkinshaw et al. (2022) compared fishmeal with soy feed and found that microplastic contamination from both sources is unavoidable.
Myctophids are prey of commercially harvested fish in Oregon such as salmon, rockfish, tuna, and sablefish (ODFW, 2016). The diets of some marine mammals and seabirds are also predominantly comprised of myctophids (Springer et al., 1996; Würsig, 2007; Newland et al., 2011). Thus, the Oregon Department of Fish and Wildlife recognizes myctophids as important forage fish whose ecological role helps to maintain sustainable fisheries. The Oregon Forage Fish Management Plan was developed in 2016 and the adopted strategy is to prohibit directed commercial harvest of myctophids and monitor their bycatch in Oregon. At the federal level, the Pacific Fishery Management Council (PFMC) identified myctophids as Ecosystem Components, and through their Fishery Ecosystem Plan Initiative 1, the PFMC also prohibits the development of a fishery for myctophids on the U.S. West Coast (PFMC, 2022).
While humans may not presently directly consume myctophids in the California Current, myctophid predators that are harvested off Oregon may be ingesting large quantities of microparticles. Glaser et al. (2015) determined that North Pacific albacore tuna consume on average 1,600 metric tons of myctophids annually. Assuming the average myctophid in this study weighed 2.23 g, North Pacific albacore could consume 7.17 × 108 myctophids per year. At ~0.42 particles per myctophid, this tuna population alone would consume over 300 million particles each year. In summary, the downstream effects of the levels of microparticles found in myctophids may be a concern for fishery and seafood industries.
Data availability statement
The datasets presented in this study can be found in online repositories. The names of the repository/repositories and accession number(s) can be found below: Oregon State University’s Scholars Archive (ScholarsArchive@OSU) https://ir.library.oregonstate.edu/concern/datasets/n583z338m.
Ethics statement
The animal study was approved by Oregon Scientific Collection Permits (OR2005-2585, OR2006-3342, OR2007-3904, OR2008-4567, OR2009-14274). The study was conducted in accordance with the local legislation and institutional requirements.
Author contributions
OB: Conceptualization, Data curation, Formal analysis, Funding acquisition, Investigation, Methodology, Validation, Visualization, Writing – original draft, Writing – review & editing. RB: Investigation, Resources, Writing – original draft, Writing – review & editing. SB: Conceptualization, Funding acquisition, Investigation, Methodology, Project administration, Resources, Supervision, Writing – original draft, Writing – review & editing. SH: Conceptualization, Formal analysis, Funding acquisition, Investigation, Methodology, Project administration, Resources, Supervision, Writing – original draft, Writing – review & editing.
Funding
The author(s) declare financial support was received for the research, authorship, and/or publication of this article. This work was made possible by the generous support of the Hatfield Marine Science Center, Bill Wick Marine Fisheries Award. Additional support was provided by the Oregon Chapter of the American Fisheries Society through their Master’s Scholarship Award and the Jerry Bouck Memorial Scholarship. Publication of this paper was supported, in part, by the Thomas G. Scott Publication Funds and the NSF Growing Convergence Research Big Idea grant # 1935028 to SB.
Acknowledgments
We express our sincerest appreciation to the crews on the SAIP cruises led by Jason Phillips, Toby Auth, and Marisa Litz that collected the myctophids used in this study and to the NOAA Northwest Fisheries Science Center for storing the samples for this eventual use. We thank Jason for cataloging the samples and Toby for collecting and managing the CTD data. We would also like to thank Emily Pedersen and Jennifer Van Brocklin for μFTIR training and for their help developing microplastic extraction methods.
Conflict of interest
The authors declare that the research was conducted in the absence of any commercial or financial relationships that could be construed as a potential conflict of interest.
Publisher’s note
All claims expressed in this article are solely those of the authors and do not necessarily represent those of their affiliated organizations, or those of the publisher, the editors and the reviewers. Any product that may be evaluated in this article, or claim that may be made by its manufacturer, is not guaranteed or endorsed by the publisher.
Supplementary material
The Supplementary Material for this article can be found online at: https://www.frontiersin.org/articles/10.3389/fmars.2024.1426136/full#supplementary-material
References
Alomar C., Sureda A., Capó X., Guijarro B., Tejada S., Deudero S. (2017). Microplastic ingestion by Mullus surmuletus Linnaeus 1758 fish and its potential for causing oxidative stress. Environ. Res. 159, 135–142. doi: 10.1016/j.envres.2017.07.043
Athey S. N., Albotra S. D., Gordon C. A., Monteleone B., Seaton P., Andrady A. L., et al. (2020). Trophic transfer of microplastics in an estuarine food chain and the effects of a sorbed legacy pollutant. Limnol Oceanogr Lett. 5, 154–162. doi: 10.1002/lol2.10130
Athey S. N., Carney Almroth B., Granek E. F., Hurst P., Tissot A. G., Weis J. S. (2022). Unraveling physical and chemical effects of textile microfibers. Water 14, 3797. doi: 10.3390/w14233797
Barth J. A., Pierce S. D., Castelao R. M. (2005). Time-dependent, wind-driven flow over a shallow midshelf submarinebank. J. Geophysical Res. 110. doi: 10.1029/2004JC002761
Bartoń K. (2023). MuMIn: Multi-Model Inference. Available online at: https://CRAN.R-project.org/package=MuMIn.
Bernal A., Toresen R., Riera R. (2020). Mesopelagic fish composition and diets of three myctophid species with potential incidence of microplastics, across the southern tropical gyre. Deep Sea Res. Part II: Topical Stud. Oceanography 179, 104706. doi: 10.1016/j.dsr2.2019.104706
Boerger C. M., Lattin G. L., Moore S. L., Moore C. J. (2010). Plastic ingestion by planktivorous fishes in the North Pacific Central Gyre. Mar. pollut. Bull. 60, 2275–2278. doi: 10.1016/j.marpolbul.2010.08.007
Bosley K. L., Lavelle J. W., Brodeur R. D., Wakefield W. W., Emmett R. L., Baker E. T., et al. (2004). Biological and physical processes in and around Astoria submarine Canyon, Oregon, USA. J. Mar. Syst. 50, 21–37. doi: 10.1016/j.jmarsys.2003.06.006
Botterell Z. L. R., Beaumont N., Dorrington T., Steinke M., Thompson R. C., Lindeque P. K. (2019). Bioavailability and effects of microplastics on marine zooplankton: A review. Environ. pollut. 245, 98–110. doi: 10.1016/j.envpol.2018.10.065
Boucher J., Friot D. (2017). Primary microplastics in the oceans: A global evaluation of sources (IUCN International Union for Conservation of Nature). doi: 10.2305/IUCN.CH.2017.01.en
Boyd C. E. (2015). “Overview of aquaculture feeds,” in Feed and Feeding Practices in Aquaculture (Elsevier), 3–25. doi: 10.1016/B978-0-08-100506-4.00001-5
Brahney J., Mahowald N., Prank M., Cornwell G., Klimont Z., Matsui H., et al. (2021). Constraining the atmospheric limb of the plastic cycle. Proc. Natl. Acad. Sci. U.S.A. 118, e2020719118. doi: 10.1073/pnas.2020719118
Brander S. M., Renick V. C., Foley M. M., Steele C., Woo M., Lusher A., et al. (2020). Sampling and quality assurance and quality control: A guide for scientists investigating the occurrence of microplastics across matrices. Appl. Spectrosc 74, 1099–1125. doi: 10.1177/0003702820945713
Brodeur R. D., Pearcy W. G., Ralston S. (2003). Abundance and distribution patterns of nekton and micronekton in the northern california current transition zone. J. Oceanography 59, 515–535. doi: 10.1023/A:1025548801541
Budimir S., Setälä O., Lehtiniemi M. (2018). Effective and easy to use extraction method shows low numbers of microplastics in offshore planktivorous fish from the northern Baltic Sea. Mar. pollut. Bull. 127, 586–592. doi: 10.1016/j.marpolbul.2017.12.054
Burla M., Baptista A. M., Zhang Y., Frolov S. (2010). Seasonal and interannual variability of the Columbia River plume: A perspective enabled by multiyear simulation databases. J. Geophys. Res. 115, 2008JC004964. doi: 10.1029/2008JC004964
Buwono N. R., Risjani Y., Soegianto A. (2021). Distribution of microplastic in relation to water quality parameters in the Brantas River, East Java, Indonesia. Environ. Technol. Innovation 24, 101915. doi: 10.1016/j.eti.2021.101915
Carbery M., O’Connor W., Palanisami T. (2018). Trophic transfer of microplastics and mixed contaminants in the marine food web and implications for human health. Environ. Int. 115, 400–409. doi: 10.1016/j.envint.2018.03.007
Catul V., Gauns M., Karuppasamy P. K. (2011). A review on mesopelagic fishes belonging to family Myctophidae. Rev. Fish Biol. Fisheries 21, 339–354. doi: 10.1007/s11160-010-9176-4
Chen Y., Awasthi A. K., Wei F., Tan Q., Li J. (2021). Single-use plastics: Production, usage, disposal, and adverse impacts. Sci. Total Environ. 752, 141772. doi: 10.1016/j.scitotenv.2020.141772
Choy C. A., Robison B. H., Gagne T. O., Erwin B., Firl E., Halden R. U., et al. (2019). The vertical distribution and biological transport of marine microplastics across the epipelagic and mesopelagic water column. Sci. Rep. 9, 7843. doi: 10.1038/s41598-019-44117-2
Collard F., Gilbert B., Compère P., Eppe G., Das K., Jauniaux T., et al. (2017). Microplastics in livers of European anchovies (Engraulis encrasicolus, L.). Environ. pollut. 229, 1000–1005. doi: 10.1016/j.envpol.2017.07.089
Cowger W., Steinmetz Z., Gray A., Munno K., Lynch J., Hapich H., et al. (2021). Microplastic spectral classification needs an open source community: open specy to the rescue! Anal. Chem. 93, 7543–7548. doi: 10.1021/acs.analchem.1c00123
Davison P. C., Checkley D. M., Koslow J. A., Barlow J. (2013). Carbon export mediated by mesopelagic fishes in the northeast Pacific Ocean. Prog. Oceanography 116, 14–30. doi: 10.1016/j.pocean.2013.05.013
Dawson A. L., Motti C. A., Kroon F. J. (2020). Solving a sticky situation: microplastic analysis of lipid-rich tissue. Front. Environ. Sci. 8. doi: 10.3389/fenvs.2020.563565
Derraik J. G. B. (2002). The pollution of the marine environment by plastic debris: a review. Mar. pollut. Bull. 44, 842–852. doi: 10.1016/S0025-326X(02)00220-5
Desforges J.-P. W., Galbraith M., Ross P. S. (2015). Ingestion of microplastics by zooplankton in the Northeast Pacific ocean. Arch. Environ. Contam Toxicol. 69, 320–330. doi: 10.1007/s00244-015-0172-5
De Vries A. N., Govoni D., Árnason S. H., Carlsson P. (2020). Microplastic ingestion by fish: Body size, condition factor and gut fullness are not related to the amount of plastics consumed. Mar. pollut. Bull. 151, 110827. doi: 10.1016/j.marpolbul.2019.110827
Doran R. (2020). Diet analysis of Black Rockfish (Sebastes melanops) from stomach contents off the coast of Newport, Oregon (Oregon State University).
El-Nemr A. (Ed.) (2012). Textiles: types, uses, and production methods (New York: Nova Science Publishers).
Eriksen M., Maximenko N., Thiel M., Cummins A., Lattin G., Wilson S., et al. (2013). Plastic pollution in the South Pacific subtropical gyre. Mar. pollut. Bull. 68, 71–76. doi: 10.1016/j.marpolbul.2012.12.021
Ferreira G. V. B., Justino A. K. S., Eduardo L. N., Schmidt N., Martins J. R., Ménard F., et al. (2023). Influencing factors for microplastic intake in abundant deep-sea lanternfishes (Myctophidae). Sci. Total Environ. 867, 161478. doi: 10.1016/j.scitotenv.2023.161478
Fox J., Weisberg S. (2019). An R Companion to Applied Regression. 3rd ed. (Sage). Available at: https://socialsciences.mcmaster.ca/jfox/Books/Companion/.
Gassel M., Rochman C. M. (2019). The complex issue of chemicals and microplastic pollution: A case study in North Pacific lanternfish. Environ. pollut. 248, 1000–1009. doi: 10.1016/j.envpol.2019.03.002
Glaser S. M., Waechter K. E., Bransome N. C. (2015). Through the stomach of a predator: Regional patterns of forage in the diet of albacore tuna in the California Current System and metrics needed for ecosystem-based management. J. Mar. Syst. 146, 38–49. doi: 10.1016/j.jmarsys.2014.07.019
Granek E. F., Traylor S. D., Tissot A. G., Hurst P. T., Wood R. S., Brander S. M. (2022). “Clothes encounters of the microfibre kind,” in Polluting Textiles (Routledge, London), 63–99. doi: 10.4324/9781003165385-5
Harris L. S. T., La Beur L., Olsen A. Y., Smith A., Eggers L., Pedersen E., et al. (2022). Temporal variability of microparticles under the seattle aquarium, Washington State: documenting the global Covid-19 pandemic. Enviro Toxic Chem. 41, 917–930. doi: 10.1002/etc.5190
Hasegawa T., Nakaoka M. (2021). Trophic transfer of microplastics from mysids to fish greatly exceeds direct ingestion from the water column. Environ. pollut. 273, 116468. doi: 10.1016/j.envpol.2021.116468
Hickey B. M., Banas N. S. (2003). Oceanography of the U.S. Pacific Northwest Coastal Ocean and estuaries with application to coastal ecology. Estuaries 26, 1010–1031. doi: 10.1007/BF02803360
Hijmans R. (2022). geosphere: Spherical Trigonometry. Available at: https://CRAN.R-project.org/package=geosphere.
Iglesias I. S., Santora J. A., Fiechter J., Field J. C. (2023). Mesopelagic fishes are important prey for a diversity of predators. Front. Mar. Sci. 10. doi: 10.3389/fmars.2023.1220088
Irigoien X., Klevjer T. A., Røstad A., Martinez U., Boyra G., Acuña J. L., et al. (2014). Large mesopelagic fishes biomass and trophic efficiency in the open ocean. Nat. Commun. 5, 3271. doi: 10.1038/ncomms4271
Jambeck J. R., Geyer R., Wilcox C., Siegler T. R., Perryman M., Andrady A., et al. (2015). Plastic waste inputs from land into the ocean. Science 347, 768–771. doi: 10.1126/science.1260352
Justino A. K. S., Ferreira G. V. B., Schmidt N., Eduardo L. N., Fauvelle V., Lenoble V., et al. (2022). The role of mesopelagic fishes as microplastics vectors across the deep-sea layers from the Southwestern Tropical Atlantic. Environ. pollut. 300, 118988. doi: 10.1016/j.envpol.2022.118988
Kaartvedt S., Staby A., Aksnes D. (2012). Efficient trawl avoidance by mesopelagic fishes causes large underestimation of their biomass. Mar. Ecol. Prog. Ser. 456, 1–6. doi: 10.3354/meps09785
Kapp K. J., Yeatman E. (2018). Microplastic hotspots in the Snake and Lower Columbia rivers: A journey from the Greater Yellowstone Ecosystem to the Pacific Ocean. Environ. pollut. 241, 1082–1090. doi: 10.1016/j.envpol.2018.06.033
Larkin P. (2011). Infrared and Raman Spectroscopy: Principles and Spectral Interpretation (Elsevier). Available at: https://ebookcentral.proquest.com/lib/osu/detail.action?docID=692432.
Lasdin K. S., Arnold M., Agrawal A., Fennie H. W., Grorud-Colvert K., Sponaugle S., et al. (2023). Presence of microplastics and microparticles in Oregon Black Rockfish sampled near marine reserve areas. PeerJ 11, e14564. doi: 10.7717/peerj.14564
Lasmanis R. (1991). The geology of Washington: rocks and minerals. Helen Dwight Reid Educ. Foundation 66, 262–277. doi: 10.1080/00357529.1991.11761628
Lebreton L., Andrady A. (2019). Future scenarios of global plastic waste generation and disposal. Palgrave Commun. 5, 6. doi: 10.1057/s41599-018-0212-7
Lebreton L. C. M., van der Zwet J., Damsteeg J.-W., Slat B., Andrady A., Reisser J. (2017). River plastic emissions to the world’s oceans. Nat. Commun. 8, 15611. doi: 10.1038/ncomms15611
Liu Y., MacCready P., Hickey B. M. (2009). Columbia River plume patterns in summer 2004 as revealed by a hindcast coastal ocean circulation model: COLUMBIA RIVER PLUME PATTERNS. Geophys. Res. Lett. 36, n/a–n/a. doi: 10.1029/2008GL036447
Love M. S., Passarelli J. K. (2020). Miller and Lea’s Guide to the Coastal Marine Fishes of California. 2nd Edition (University of California Agriculture and Natural Resources). Available at: https://books.google.com/books?id=Puc7ywEACAAJ.
Lusher A. (2015). “Microplastics in the marine environment: distribution, interactions and effects,” in Marine Anthropogenic Litter. Eds. Bergmann M., Gutow L., Klages M. (Springer International Publishing, Cham), 245–307. doi: 10.1007/978-3-319-16510-3_10
Lusher A., O’Donnell C., Officer R., O’Connor I. (2016). Microplastic interactions with North Atlantic mesopelagic fish. ICES J. Mar. Sci. 73, 1214–1225. doi: 10.1093/icesjms/fsv241
McGoran A. R., Maclaine J. S., Clark P. F., Morritt D. (2021). Synthetic and semi-synthetic microplastic ingestion by mesopelagic fishes from Tristan da Cunha and St Helena, South Atlantic. Front. Mar. Sci. 8. doi: 10.3389/fmars.2021.633478
McIlwraith H. K., Kim J., Helm P., Bhavsar S. P., Metzger J. S., Rochman C. M. (2021). Evidence of microplastic translocation in wild-caught fish and implications for microplastic accumulation dynamics in food webs. Environ. Sci. Technol. 55, 12372–12382. doi: 10.1021/acs.est.1c02922
McNeish R. E., Kim L. H., Barrett H. A., Mason S. A., Kelly J. J., Hoellein T. J. (2018). Microplastic in riverine fish is connected to species traits. Sci. Rep. 8, 11639. doi: 10.1038/s41598-018-29980-9
Meijer L. J. J., Van Emmerik T., van der Ent R., Schmidt C., Lebreton L. (2021). More than 1000 rivers account for 80% of global riverine plastic emissions into the ocean. Sci. Adv. 7, eaaz5803. doi: 10.1126/sciadv.aaz5803
Mensinger A. F. (2011). “THE SKIN | Bioluminescence in fishes,” in Encyclopedia of Fish Physiology (Elsevier), 497–503. doi: 10.1016/B978-0-12-374553-8.00160-X
Miller E., Sedlak M., Lin D., Box C., Holleman C., Rochman C. M., et al. (2021). Recommended best practices for collecting, analyzing, and reporting microplastics in environmental media: Lessons learned from comprehensive monitoring of San Francisco Bay. J. Hazardous Materials 409, 124770. doi: 10.1016/j.jhazmat.2020.124770
Newland C., Field I., Cherel Y., Guinet C., Bradshaw C., McMahon C., et al. (2011). Diet of juvenile southern elephant seals reappraised by stable isotopes in whiskers. Mar. Ecol. Prog. Ser. 424, 247–258. doi: 10.3354/meps08769
ODFW (2016). Oregon Forage Fish Management Plan (Oregon Department of Fish and Wildlife Marine Resources Program).
OECD (2022). Global Plastics Outlook: Economic Drivers, Environmental Impacts and Policy Options (OECD). doi: 10.1787/de747aef-en
Okamoto K., Nomura M., Horie Y., Okamura H. (2022). Color preferences and gastrointestinal-tract retention times of microplastics by freshwater and marine fishes. Environ. pollut. 304, 119253. doi: 10.1016/j.envpol.2022.119253
Palumbi S. R., Gaines S. D., Leslie H., Warner R. R. (2003). New wave: high-tech tools to help marine reserve research. Front. Ecol. Environ. 1, 73–79. doi: 10.1890/1540-9295(2003)001[0073:NWHTTT]2.0.CO;2
Payne A. I. L., Rose B., Leslie R. W. (1987). Feeding of hake and a first attempt at determining their trophic role in the South African west coast marine environment. South Afr. J. Mar. Sci. 5, 471–501. doi: 10.2989/025776187784522667
Pazos R. S., Maiztegui T., Colautti D. C., Paracampo A. H., Gómez N. (2017). Microplastics in gut contents of coastal freshwater fish from Río de la Plata estuary. Mar. pollut. Bull. 122, 85–90. doi: 10.1016/j.marpolbul.2017.06.007
Pérez A. F., Ojeda M., Rimondino G. N., Chiesa I. L., Di Mauro R., Boy C. C., et al. (2020). First report of microplastics presence in the mussel Mytilus Chilensis from Ushuaia Bay (Beagle Channel, Tierra del Fuego, Argentina). Mar. pollut. Bull. 161, 111753. doi: 10.1016/j.marpolbul.2020.111753
PFMC (2022). Ecosystem Initiatives Appendix to the Pacific Coast Fishery Ecosystem Plan (Pacific Fishery Management Council).
Phillips J. A., Brodeur R. D., Suntsov A. V. (2009). Micronekton community structure in the epipelagic zone of the northern California Current upwelling system. Prog. Oceanography 80, 74–92. doi: 10.1016/j.pocean.2008.12.001
Pietroluongo G., Quintana Martín-Montalvo B., Antichi S., Miliou A., Costa V. (2022). First assessment of micro-litter ingested by dolphins, sea turtles and monk seals found stranded along the coasts of Samos Island, Greece. Animals 12, 3499. doi: 10.3390/ani12243499
Remesan M. P., Prakash R. R., Prajith K. K., Jha P. N., Renjith R. K., Boopendranath M. R. (2019). A review on techniques and challenges in the harvest of mesopelagics. Fishery Technol. 56, 243–253.
Remy F., Collard F., Gilbert B., Compère P., Eppe G., Lepoint G. (2015). When microplastic is not plastic: the ingestion of artificial cellulose fibers by macrofauna living in seagrass macrophytodetritus. Environ. Sci. Technol. 49, 11158–11166. doi: 10.1021/acs.est.5b02005
Rodrigues S. M., Almeida C. M. R., Silva D., Cunha J., Antunes C., Freitas V., et al. (2019). Microplastic contamination in an urban estuary: Abundance and distribution of microplastics and fish larvae in the Douro estuary. Sci. Total Environ. 659, 1071–1081. doi: 10.1016/j.scitotenv.2018.12.273
Savoca M. S., McInturf A. G., Hazen E. L. (2021). Plastic ingestion by marine fish is widespread and increasing. Glob Change Biol. 27, 2188–2199. doi: 10.1111/gcb.15533
Siddiqui S., Hutton S. J., Dickens J. M., Pedersen E. I., Harper S. L., Brander S. M. (2023). Natural and synthetic microfibers alter growth and behavior in early life stages of estuarine organisms. Front. Mar. Sci. 9. doi: 10.3389/fmars.2022.991650
Springer A. M., Piatt J. F., Vliet G. V. (1996). Sea birds as proxies of marine habitats and food webs in the western Aleutian Arc. Fisheries Oceanogr 5, 45–55. doi: 10.1111/j.1365-2419.1996.tb00016.x
Suntsov A., Brodeur R. (2008). Trophic ecology of three dominant myctophid species in the northern California Current region. Mar. Ecol. Prog. Ser. 373, 81–96. doi: 10.3354/meps07678
Takada H., Karapanagioti H. K. (Eds.) (2019). Hazardous Chemicals Associated with Plastics in the Marine Environment (Cham: Springer International Publishing). doi: 10.1007/978-3-319-95568-1
Talbot R., Granek E., Chang H., Wood R., Brander S. (2022). Spatial and temporal variations of microplastic concentrations in Portland’s freshwater ecosystems. Sci. Total Environ. 833, 155143. doi: 10.1016/j.scitotenv.2022.155143
Teuten E. L., Saquing J. M., Knappe D. R. U., Barlaz M. A., Jonsson S., Björn A., et al. (2009). Transport and release of chemicals from plastics to the environment and to wildlife. Phil. Trans. R. Soc B 364, 2027–2045. doi: 10.1098/rstb.2008.0284
Torres L. G., Brander S. M., Parker J. I., Bloom E. M., Norman R., Van Brocklin J. E., et al. (2023). Zoop to poop: assessment of microparticle loads in gray whale zooplankton prey and fecal matter reveal high daily consumption rates. Front. Mar. Sci. 10. doi: 10.3389/fmars.2023.1201078
USGS (2024). Columbia River Below Bonneville Dam, OR - 14128870. Available online at: https://waterdata.usgs.gov/nwis/monthly/?site_no=14128870&referred_module=sw&format=sites_selection_links (Accessed February 12, 2024).
Uy C. A., Johnson D. W. (2022). Effects of microplastics on the feeding rates of larvae of a coastal fish: direct consumption, trophic transfer, and effects on growth and survival. Mar. Biol. 169, 27. doi: 10.1007/s00227-021-04010-x
Valinassab T., Pierce G., Johannesson K. (2007). Lantern fish (Benthosema pterotum) resources as a target for commercial exploitation in the Oman Sea. J. Appl. Ichthyology 23, 573–577. doi: 10.1111/j.1439-0426.2007.01034.x
Valine A. E., Peterson A. E., Horn D. A., Scully-Engelmeyer K. M., Granek E. F. (2020). Microplastic prevalence in 4 oregon rivers along a rural to urban gradient applying a cost-effective validation technique. Environ. Toxicol. Chem. 39, 1590–1598. doi: 10.1002/etc.4755
Walkinshaw C., Tolhurst T. J., Lindeque P. K., Thompson R., Cole M. (2022). Detection and characterisation of microplastics and microfibres in fishmeal and soybean meal. Mar. pollut. Bull. 185, 114189. doi: 10.1016/j.marpolbul.2022.114189
Wang X., Zhu L., Liu K., Li D. (2022). Prevalence of microplastic fibers in the marginal sea water column off southeast China. Sci. Total Environ. 804, 150138. doi: 10.1016/j.scitotenv.2021.150138
Watanabe H., Kawaguchi K., Ohno A. (1999). Diel vertical migration of myctophid fishes (Family Myctophidae) in the transitional waters of the western North Pacific. Fish. Oceanogr. 13, 115–127. doi: 10.1046/j.1365-2419.1999.00103.x
Way C., Hudson M. D., Williams I. D., Langley G. J. (2022). Evidence of underestimation in microplastic research: A meta-analysis of recovery rate studies. Sci. Total Environ. 805, 150227. doi: 10.1016/j.scitotenv.2021.150227
Wieczorek A. M., Morrison L., Croot P. L., Allcock A. L., MacLoughlin E., Savard O., et al. (2018). Frequency of microplastics in mesopelagic fishes from the Northwest Atlantic. Front. Mar. Sci. 5. doi: 10.3389/fmars.2018.00039
Würsig B. (2007). Dusky dolphins (Lagenorhynchus obscurus) in New Zealand waters: present knowledge and research goals. New Z. Department Conserv.
Keywords: microparticles, microfiber, lanternfish, myctophids, mesopelagic fishes, Columbia River, Northern California Current, vertical migration
Citation: Boisen OC, Brodeur RD, Brander SM and Heppell SA (2024) Spatial patterns of microparticle ingestion by myctophids near a major river mouth in the northeast Pacific Ocean. Front. Mar. Sci. 11:1426136. doi: 10.3389/fmars.2024.1426136
Received: 30 April 2024; Accepted: 26 July 2024;
Published: 16 August 2024.
Edited by:
Jenny Fong, Griffith University, AustraliaReviewed by:
Anne K. S. Justino, Federal Rural University of Pernambuco, BrazilGuilherme V. B. Ferreira, Federal University of Rio de Janeiro, Brazil
Copyright © 2024 Boisen, Brodeur, Brander and Heppell. This is an open-access article distributed under the terms of the Creative Commons Attribution License (CC BY). The use, distribution or reproduction in other forums is permitted, provided the original author(s) and the copyright owner(s) are credited and that the original publication in this journal is cited, in accordance with accepted academic practice. No use, distribution or reproduction is permitted which does not comply with these terms.
*Correspondence: Olivia C. Boisen, b2xpdmlhLmJvaXNlbkBvcmVnb25zdGF0ZS5lZHU=; Scott A. Heppell, c2NvdHQuaGVwcGVsbEBvcmVnb25zdGF0ZS5lZHU=
†These authors have contributed equally to this work