- 1Centre for Geography and Environmental Science, Department of Earth and Environmental Sciences, Faculty of Environment, Science and Economy, University of Exeter, Cornwall, United Kingdom
- 2National Institute of Oceanography and Applied Geophysics (OGS), Sgonico, TS, Italy
- 3Department of Oceanography, Universidad de Concepción, Concepción, Chile
- 4Millennium Institute of Oceanography, Universidad de Concepción, Concepción, Chile
Recent decadal trends of deoxygenation in the global ocean interior have resulted in the expansion and shoaling of oxygen minimum zones (OMZs). When the OMZs upper bound nears the euphotic zone a unique community of phytoplankton, residing in extremely low light (<0.1% surface irradiance) and dissolved oxygen concentrations (<1-2 μmol kg-1), can appear. In this mini-review paper we synthesize our current understanding of the phytoplankton community that resides in an OMZ chlorophyll maximum (OMZ-CM), below the depths of the deep chlorophyll maximum found in permanently and seasonally stratified regions, and its role in OMZ biogeochemical cycles. Uncultivated basal lineages of the cyanobacterium Prochlorococcus dominate this community, forming an OMZ-CM that can contribute to integrated stocks of chlorophyll a, in some cases with a similar magnitude to the deep chlorophyll maximum. Photosynthesis by Prochlorococcus in the OMZ-CM provides a significant source of oxygen, that fuels the aerobic oxidation of nitrite and organic matter, impacting elemental biogeochemical cycling, including that of carbon and nitrogen. Yet, on a global scale, there is a lack of understanding and quantification of the spatial distribution of these OMZ-CM, their stocks of phytoplankton, their influence on fluxes of carbon and nitrogen, and how these may respond to climate change. Monitoring the dynamics of the OMZ-CM and biogeochemical cycles in OMZs is challenging, and requires a multidisciplinary approach, combining ship-based observations with autonomous platforms, satellite data, and conceptual models. Only then can the implications of enhanced deoxygenation on the future marine ecosystem be understood.
1 Introduction
Phytoplankton growth is dependent on light and nutrient availability (Bristow et al., 2017). In stratified open ocean regions, a typical vertical profile of phytoplankton is characterised by a deep chlorophyll maximum (DCM) that forms where there is above minimum light and nutrients to support photosynthesis and growth (Mignot et al., 2014; see Figure 1B). In contrast to well-oxygenated mixed (Figure 1A) and stratified waters (Figure 1B), regions characterised by sluggish circulation, long ventilation times (Pacific and Atlantic Ocean Oxygen Minimum Zones (OMZs)), and high rates of oxygen utilisation (Indian Ocean OMZ) (Karstensen et al., 2008; Keeling et al., 2010), can form OMZs (see Figure 1C). In some cases, the OMZ upper bound can approach the photosynthetically active euphotic zone, supporting the growth of an uncultivated unique community of low-oxygen-adapted phytoplankton (Figure 1C: Johnson et al., 1999; Goericke et al., 2000; Whitmire et al., 2009; Lavin et al., 2010). This community relies on other mechanisms, including the use of organic compounds (see Biller et al., 2018; Coe et al., 2016 and Ulloa et al., 2021), “helper-bacterium” (see Morris et al., 2008 and Roth-Rosenberg et al., 2020), and fermentation, to supplement growth in extremely low irradiance levels and oxygen depletion (Wong et al., 2023). Due to the depth of these phytoplankton communities, monitoring their dynamics and contributions to net stocks at a high spatial and temporal resolution, is challenging (Cox et al., 2023).
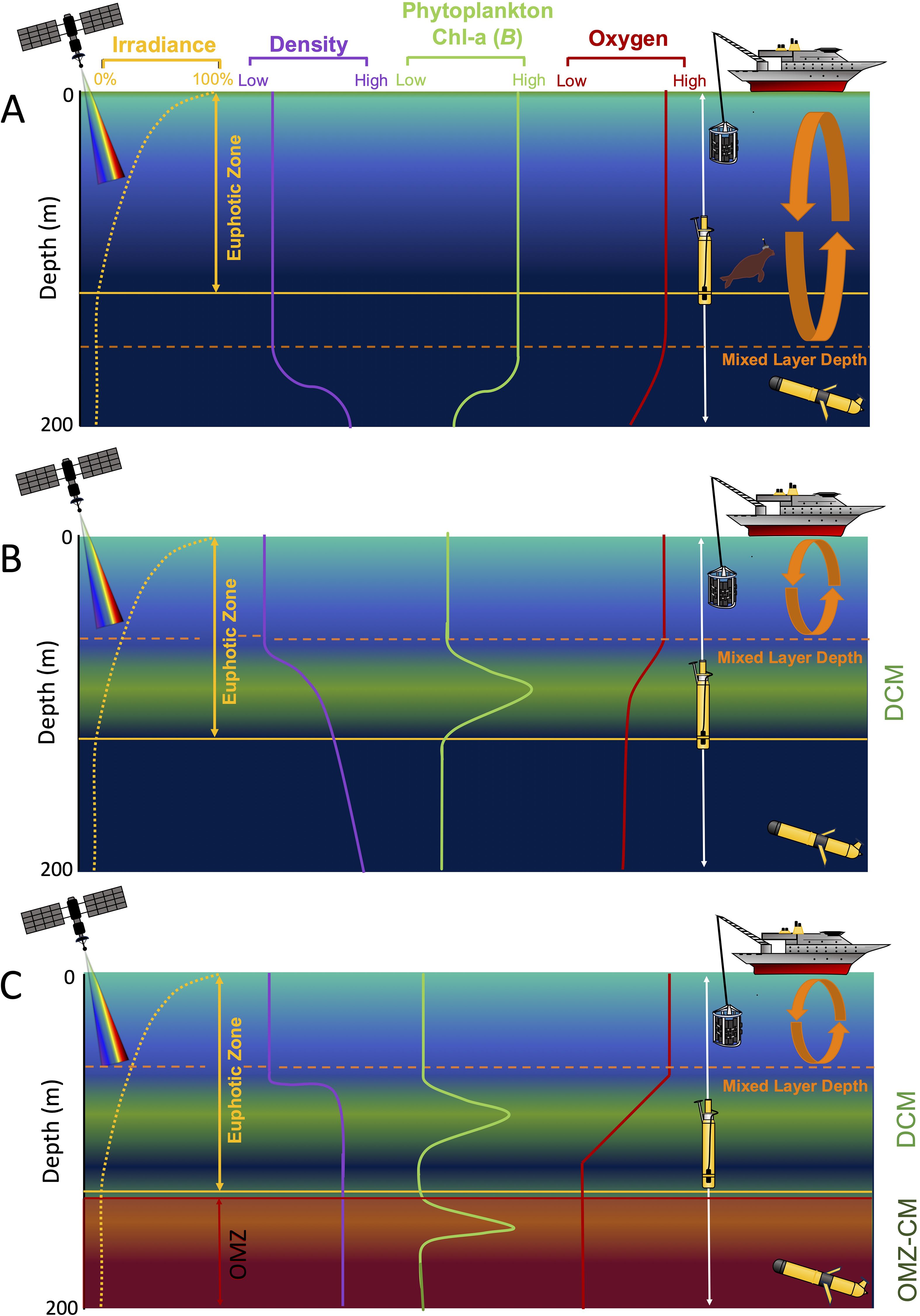
Figure 1. The typical vertical distribution of irradiance (yellow dotted line), density (purple line), phytoplankton (Chl a) (green line) and oxygen (red line), and the positions of the mixed layer depth, the deep chlorophyll maximum (DCM), the oxygen minimum zone chlorophyll maximum (OMZ-CM), and oxygen minimum zone (OMZ) within the top 200 m of the water column in the occurrence of: (A) high latitude well-mixed ocean, (B) typical subtropical ocean, and (C) tropical ocean with an OMZ approaching the euphotic zone.
A 1°C rise in global temperatures since the pre-industrial period has driven rapid oceanic changes, including shifts in oceanic circulation, thermal states, oxygen solubility, export production, remineralisation, and air-sea gas exchange (Matear and Hirst, 2003; Keeling et al., 2010; Winder and Sommer, 2012; Hoegh-Guldberg et al., 2018). Enhanced thermally-driven stratification limits deep vertical mixing, isolating oxygen-rich surface waters from oxygen-depleted subsurface waters (where O2 <60 μmol kg-1), intensifying and forming OMZs (Reid, 1965; Diaz, 2001; Franz et al., 2012; Márquez-Artavia et al., 2019). OMZs are host to a diverse assembledge of anaerobic microorganisms and organosulfer-based heterotrophs (Ulloa et al., 2012; Wright et al., 2012; Callbeck et al., 2021). The anaerobic microbial community aids the transformation of nitrate, nitrite, and ammonium through two dominant, oxygen-sensitive processes that form N2, anaerobic ammonium oxidation and denitrification (Lam and Kuypers, 2011; Ulloa et al., 2012). The formation of N2 in OMZs is responsible for around 30-50% of fixed nitrogen loss in the global ocean (Codispoti et al., 2001). Nanomolar dissolved oxygen (DO) rates in OMZs support denitrification – the sequential reduction of nitrate to gaseous N2O and N2 via nitrite, and, nitric oxide (Lam and Kuypers, 2011) – yet, may be limited by the availability of organic matter (Ulloa et al., 2012). Denitrification has been found to be the dominant pathway of N2 production, however, anammox contributes significantly to N2 formation in the upper parts of the OMZ (Lam and Kuypers, 2011). The deficient levels of oxygen and nitrate in OMZs, can also promote enhanced rates of sulfate reduction (Canfield et al., 2010; Callbeck et al., 2021). Sulfate reduction in OMZs, alongside organic matter oxidation and sulfidogenisis, produces sulfide which is then oxidized by sulfide-oxidizing nitrate-reducing bacteria (Canfield et al., 2010; Callbeck et al., 2021). Projected expansions (Keeling et al., 2010) and shoaling (Gilly et al., 2013) of OMZs to shallower depths may alter OMZ biogeochemical cycling.
Our understanding of low-oxygen-adapted phytoplankton physiology (Wong et al., 2023), and their role in biogeochemical cycling and evolution is improving (Garcia-Robledo et al., 2017; Aldunate et al., 2020, 2022; Ulloa et al., 2021), however, many questions remain that can only be addressed by improved monitoring. This mini review aims to provide a summary on the current state of knowledge on the distribution of low-oxygen-adapted phytoplankton and their contribution to integrated phytoplankton stocks and anoxic biogeochemical cycles, whilst highlighting future work needed to advance understanding as projected shoaling of OMZs (Long et al., 2019) may enhance the presence of low-oxygen-adapted phytoplankton in a future ocean.
2 Distribution of phytoplankton
Optimal environmental conditions for phytoplankton community growth are expected to vary seasonally with cycles of light, stratification and ocean mixing, influencing phytoplankton and OMZ distribution (Figure 2: Cox et al., 2023; Winder and Sommer, 2012). At low latitudes, DCMs are a permanent and frequent feature as a result of light/nutrient availability and water-mass stability, yet at higher latitudes DCMs are seasonally dependent (see Figure 2; Cornec et al., 2021). The formation of a chlorophyll-a maximum below the traditional DCM, and near to (i.e. within suboxic regions) or within the upper bound of an OMZ (hereafter defined as an OMZ-CM, see Figure 1C) has been observed in low latitudes, such as the Eastern Tropical North Pacific (ETNP), the Eastern Tropical South Pacific (ETSP) and the Arabian Sea (see Figure 2; Goericke et al., 2000; Cepeda-Morales et al., 2009; Garcia-Robledo et al., 2017), and can vary seasonally (see Figure 2 and Cox et al., 2023; Wong et al., 2023).
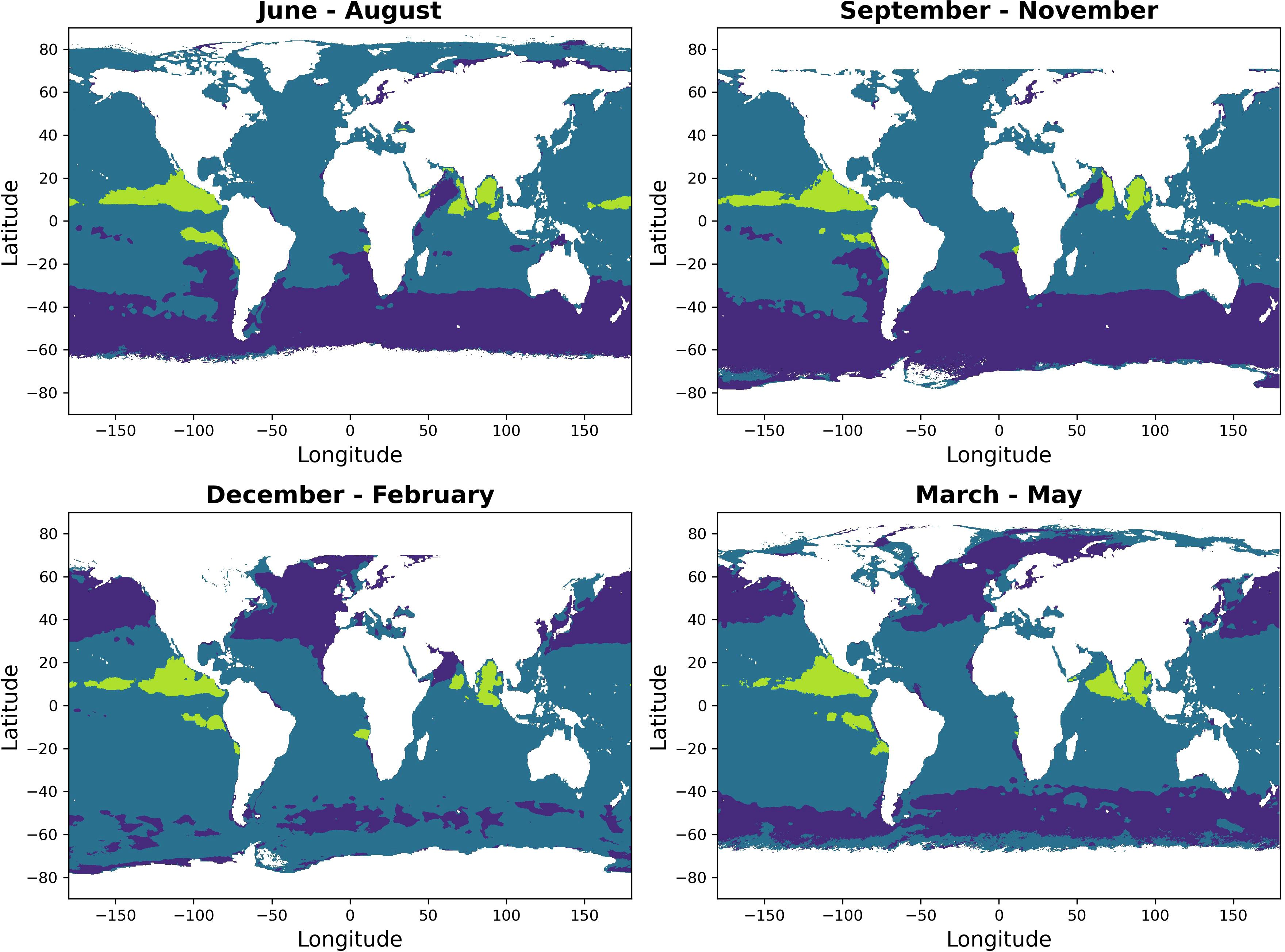
Figure 2. Seasonally estimated, global distribution of three environments for phytoplankton growth. Situations where the mixed layer is deeper than the euphotic zone are seen in purple (see Figure 1A, mixed waters); situations where the euphotic zone is deeper than the mixed-layer are seen in blue (see Figure 1B. stratified waters), and situations where the euphotic zone is deeper than the mixed-layer (stratified waters), but the depth of the upper OMZ nears the euphotic zone (depth of the 60 μmol L−1 isoline reaches the 0.1% light level at the surface), offering conditions for an OMZ-CM to form are seen in green (see Cox et al. (2023) and Figure 1C). This figure was inspired by Keeling et al. (2010). The figure was produced using climatologies of mixed layer depth (Monthly Isopycnal & Mixed-layer Ocean Climatology; Schmidtko et al., 2013), euphotic depth (derived by using NASA MODIS diffuse attenuation products at 490 nm (https://oceancolor.gsfc.nasa.gov), and the models of Morel et al. (2007), and the depth of the 60 μmol L−1 isoline from the World Ocean Database, 2018 (García et al., 2019).
In stratified regions where an OMZ exists, the oxic surface layer (typically 0 - 50 m) and the anoxic deep waters (where oxygen is absent; typically 100 - 1000 m) is separated by a suboxic zone (where there is oxygen deficiency; typically 50 – 100 m) (Wong et al., 2023). The depth and abundance of OMZ-CMs is dependent on OMZ thickness and region (Sarma et al., 2020; Wong et al., 2023). In the ETNP, the OMZ-CM depth has been found to vary between 80 - 160 m, remaining below the upper bound of the OMZ found between 80 - 116 m (see studies by Goericke et al. (2000); Cepeda-Morales et al. (2009); Garcia-Robledo et al. (2017); Aldunate et al. (2020); Cox et al. (2023)). Similarly, in the ETSP, OMZ-CMs have been identified under the upper bound of the OMZ at depths between 68 - 130 m (Garcia-Robledo et al., 2017; Aldunate et al., 2020), and in the equatorial Pacific, between 86 - 128 m (Márquez-Artavia et al., 2019). However, due to the seasonal variation in physical mechanisms governing the Arabian Sea – including convection mixing, coastal upwelling and open ocean upwelling – the presence of OMZ-CMs is seasonally and temporally variable (Goericke et al., 2000; Sarma et al., 2020).
3 OMZ-CM community composition
Cyanobacteria, specifically Prochlorococcus, are reported to dominate over other phytoplankton communities in the OMZ-CM (Goericke et al., 2000; Lavin et al., 2010). Prochlorococcus’ ability to thrive in low-light conditions (see Figure 1C) was initially attributed to the physiological acclimation observed in natural assembledges through flow cytometry and high-performance liquid chromatography (Johnson et al., 1999; Goericke et al., 2000). Distinct adaptations to light enable Prochlorococcus to occupy the entire euphotic zone, which can be explained by ecotype adaptation to differing light optima for growth (Moore et al., 1998). Cells at the base of the euphotic zone in low irradiances are termed low-light (LL) adapted ecotypes, and those closer to the surfaces are termed high-light (HL) adapted ecotypes (Biller et al., 2014). Prochlorococcus’ pigment traits of divinyl chlorophyll-a and b, and α-carotene, alongside potential use of organic compounds to supplement carbon and energy requirements, aid survival in extremely low irradiances (Ulloa et al., 2021). More recent molecular techniques have also revealed the existence of novel OMZ-specific Prochlorococcus lineages (Lavin et al., 2010; Ulloa et al., 2021). In OMZ-CM, the majority of the phytoplanktonic community is thought to be Prochlorococcus ecotypes AMZ I (formerly LLV), AMZ II (formerly LLVI), and AMZ III (Lavin et al., 2010; Ulloa et al., 2021), found only in oxygen-depleted regions (Ulloa et al., 2021). Ulloa et al. (2021) suggest that certain adaptations to oxygen depletion are ancestral, implying that Prochlorococcus may have originated in a low-oxygen subsurface ocean. Other genetic properties of the AMZ ecotypes, including a hybrid-cluster protein, a cognate regulator of the anaerobic family, and a gene encoding an iron-sulfur cluster repair protein, may support adaptations to low-oxygen environments (Ulloa et al., 2021). However, these distinct OMZ-specific Prochlorococcus lineages are uncultivated, with little known about their physiology and distribution.
Photosynthesis by OMZ-CM Prochlorococcus provides a significant source of oxygen that fuels nitrite and organic matter oxidation (Garcia-Robledo et al., 2017). Rapid consumption of produced oxygen by aerobic microbial communities maintains the anoxic environment (below detection limit at nanomolar levels) (Tiano et al., 2014; Garcia-Robledo et al., 2017). Aerobic respiration in extremely low dissolved oxygen concentrations is suggested to be a common phenomenon that is initiated when the microbial community residing in the anoxic environment is exposed to oxygen concentrations of nanomolar to a few micromolar (Tiano et al., 2014). Garcia-Robledo et al. (2017) coined the oxygen production and rapid consumption, the ‘cryptic oxygen cycle’, that demonstrates aerobic metabolisms can occur near the upper bound of the OMZ in a dimly lit environment, as hypothesized by Ulloa et al. (2012). Seasonal trends of biological productivity can modulate this cryptic oxygen cycle, with microbial blooms intensifying during spring and summer, increasing anaerobic consumption and particle export to mid-depths (Long et al., 2021).
4 Controls of dissolved oxygen concentrations
Dissolved oxygen dynamics are controlled by physical, biological and biogeochemical processes that are sensitive to natural and anthropogenic forcing (Altieri and Diaz, 2019; Grégoire et al., 2021). Primary factors controlling DO concentrations include: (1) air-sea exchange, (2) water-mass ventilation, (3) temperature-controlled solubility, (4) phytoplankton primary production, and (5) export production and associated remineralisation (Matear and Hirst, 2003; Keeling et al., 2010; Engel et al., 2022). The upper ocean DO concentrations are affected by oxygen solubility, ocean circulation, air-sea interactions and phytoplankton primary production (Oschlies, 2019). Whilst primary production increases DO concentrations within the ocean surface, oxygen solubility in water is inversely related to temperature, resulting in oxygen loss due to ocean warming under climate change (Matear and Hirst, 2003; Garcia et al., 2005; Keeling et al., 2010; Schmidtko et al., 2017; Oschlies et al., 2018; Wu et al., 2022). The thermal sensitivity of oxygen solubility is thought to explain up to 50% of oxygen loss in the upper 1000 m and 15% of the overall observed global oxygen loss since 1960 (Matear and Hirst, 2003; Keeling et al., 2010; Schmidtko et al., 2017; Oschlies et al., 2018). Warming at the surface also increases stratification, reducing ocean circulation (Oschlies, 2019). Changes to ocean circulation in response to climate change is thought to account for the other ≤ 50% oxygen loss in the upper ocean, whilst contributing up to 98% to oxygen loss in the ocean interior (>1000 m) (Oschlies, 2019). It is thought that reduced ocean ventilation and circulation are responsible for around 85% of the current overall global oxygen loss (Schmidtko et al., 2017; Breitburg et al., 2018), whilst also impacted by decadal and multidecadal oscillations (Matear and Hirst, 2003; Schmidtko et al., 2017; Breitburg et al., 2018). Alongside reduced ventilation, enhanced stratification can generate more nutrient utilisation within surface waters, amplifying the downward flux of organic matter (Keeling et al., 2010; Schmidtko et al., 2017), increasing oxygen depletion (Keeling et al., 2010). Further increases in oxygen depletion could influence the vertical distribution of phytoplankton and the contribution of the OMZ-CM to phytoplankton stocks.
5 Monitoring the OMZ-CM
Monitoring OMZ-CM dynamics is challenging due to the depths of OMZ-CM (around 100 m; Cox et al., 2023). Satellite remote sensing provides insight into surface ocean physical and biological dynamics (< 50 m) on global and synoptic scales (Groom et al., 2019). Satellite passive ocean colour has proven useful for extrapolating phytoplankton signals seen at the surface by the satellite to deeper depths (Uitz et al., 2006). Although airborne lidar can observe phytoplankton deeper in the water column (Hostetler et al., 2018; Behrenfeld et al., 2023), and may prove useful for studying subsurface communities in the future, the OMZ-CM is invisible to passive ocean colour airborne sensors (Gordon and McCluney, 1975), and thus, monitoring phytoplankton communities at depths requires in-situ measurements. Traditionally, ship-based observations have provided in-situ measurements below the ocean surface. Whilst important for detailed analysis of phytoplankton at depth, ship-based measurements are unable to provide the high spatial and temporal coverage required to fully understand phytoplankton dynamics and distributions in the subsurface (Chai et al., 2020; Cox et al., 2023).
Ocean robotic platforms, particularly autonomous profiling floats, provide opportunities to observe and monitor the internal ocean at a greater resolution on a large scale (Chai et al., 2020). Autonomous profiling floats, such as Biogeochemical-Argo floats (BGC-Argo floats), capture biogeochemical and physical dynamics at depths up to 2000 m at higher spatial and temporal resolutions than ship-based platforms, continuously collecting data for several years and, as of September 2019, providing on average 50 observed profiles per 1° square globally (Ravichandran et al., 2012; Bittig et al., 2019; Wong et al., 2020; Addey, 2022). Environmental observations taken by optical sensors mounted on BGC-Argo floats measuring Chl a pigment concentration and particulate backscattering (bbp) have been used in conjunction with conceptual models to extract information on OMZ-CM phytoplankton (Whitmire et al., 2009; Cox et al., 2023). Cox et al. (2023) used data from a BGC-Argo float to study the environmental characteristics, and the depth, the width, and the concentration of the OMZ-CM peak, whilst also calculating the C:Chl a ratio (inferred from bbp). In such study, Cox et al. (2023) found the OMZ-CM to contribute around 45 ± 4% to chlorophyll a (Chl a) and around 30% to integrated bbp (a proxy for phytoplankton carbon) in the ETNP (after removing a background signal attributed to non-algal particles). The contributions of the OMZ-CM were similar to that of the DCM despite very low light conditions (Cox et al., 2023). Depth-dependent variation in the bbp:Chl a ratio – used as a proxy for the carbon:Chl a ratio – suggested that the OMZ-CM consists of very low-light adapted phytoplankton (Cox et al., 2023). To synoptically monitor phytoplankton and biogeochemical dynamics throughout the water column, satellite passive ocean colour data and airborne lidar may be useful to extrapolate vertical information derived from autonomous profiling floats over large spatial areas (Claustre et al., 2020).
In-vivo fluorescence is the most recorded biological property in the open ocean, often used to understand the vertical profile and dynamics of phytoplankton communities (Mignot et al., 2011). The integration of fluorometers and optical backscattering sensors onboard autonomous platforms has significantly increased the spatial and temporal resolution of in-vivo Chl a fluorescence and bbp data (Sauzède et al., 2015). However, it is important to consider the limitations in these measurement techniques. Chl a fluorescence is impacted by several factors (Sauzède et al., 2015). The range of variation in the fluorescence-to-Chl a ratio can be up to 10-fold, a function of community composition, photoacclimation, nutrient limitation, light acclimation, growth phase, and non-photochemical quenching (Seliger and Loftus, 1974; Zeng and Li, 2015; Bittig et al., 2019). Optical backscattering sensors measure all particles in the water, not just phytoplankton, so data can be difficult to interpret. To harness these platforms to their full extent requires careful quality control of optical data (Bittig et al., 2019; Dall’Olmo et al., 2023), consideration of uncertainties, and understanding how optical properties are impacted by the environment and the particles and substances present in it (Organelli et al., 2018; Petit et al., 2022). To interpret better the data from autonomous platforms and to gain a more comprehensive understanding of the OMZ-CM, there is a need to obtain cultured isolates for physiological, biochemical and molecular analysis in the laboratory (Mühling, 2012).
6 A changing ocean and unanswered questions
Observations suggest deoxygenation is occurring globally at a rate of -243 ± 124 Tmol O2 per decade in the upper ocean and -703 ± 244 Tmol O2 per decade in the interior ocean (Ito et al., 2017; Schmidtko et al., 2017), alongside the shoaling of OMZs (Gilly et al., 2013). The global oxygen inventory is negatively correlated with the ocean heat content, due to oxygen solubility and ocean stratification (Ito et al., 2017). Simulations by Earth System Models emphasise the thermal sensitivity of the global ocean oxygen inventory, with further declines predicted under all warming scenarios by 2100 (Ito et al., 2017). Model simulations predict a 1-7% decline in the global ocean oxygen inventory by 2100 (based on the average ocean oxygen concentration of around 178 μmol kg-1; Sarmiento and Gruber, 2006), with decreases in oxygen solubility reported to contribute 18 – 50% (Keeling et al., 2010). Climate-induced ocean warming and associated oxygen outgassing, alongside changes in circulation, are suggested to cause OMZ expansion and intensification (Li et al., 2020; Canfield and Kraft, 2022). However, recent studies have found a weakening in upwelling and decrease in organic matter transportation has led to an increase in DO concentration at the Arabian Sea OMZ core (Vallivattathillam et al., 2023; Liu et al., 2024). This recovery may be unique to the Arabian Sea due to the physical and biological mechanisms in the region (Liu et al., 2024).
Oxygen distribution and availability structures the biogeographic ranges of marine organisms (Long et al., 2021). Whilst limiting for higher trophic levels, deoxygenation is expected to benefit anaerobic microbes and low-oxygen-adapted phytoplankton, such as some Prochlorococcus ecotypes (Long et al., 2021). Further oceanic deoxygenation may enhance the presence of OMZ-CMs in the global ocean, that may affect biogeochemical cycles, elemental stoichiometry, and the regulation of atmospheric climate conditions.
The following list identifies some of the major questions that remain unanswered:
1. What is the distribution of low-oxygen-adapted phytoplankton in the ocean and how does it vary seasonally and spatially?
2. What is the contribution of low-oxygen-adapted phytoplankton to global and regional stocks of phytoplankton and biogeochemical fluxes of carbon (e.g. primary production) and other elements and compounds (e.g. nitrogen)?
3. How are low-oxygen-adapted phytoplankton likely to respond to climate change and what are the implications for biogeochemical cycles at regional and global scales?
A multidisciplinary approach is key to answering these questions. For example, combining ocean robotic platforms with satellite remote sensing (passive and active ocean colour), may help address question 1. Integrating this new spatial understanding (question 1) with conventional (ship-based) in-situ and laboratory culture measurements on rates (e.g. primary production) may help address question 2. Incorporating OMZ phytoplankton communities explicitly into ecosystem models may help address question 3.
Author contributions
IC: Writing – original draft, Writing – review & editing. RB: Supervision, Writing – review & editing. KS: Supervision, Writing – review & editing. GD’O: Supervision, Writing – review & editing. OU: Supervision, Writing – review & editing.
Funding
The author(s) declare financial support was received for the research, authorship, and/or publication of this article. This work was supported by a UKRI Future Leader Fellowship (MR/V022792/1), and a PhD studentship funded by the University of Exeter. OU was supported by a Royal Society Wolfson Visiting Fellowship. For the purpose of open access, the author has applied a ‘Creative Commons Attribution (CC BY) licence to any Author Accepted Manuscript version arising from this submission.
Acknowledgments
We thank Shubha Sathyendranath for her support. We thank both reviewers for comments that helped improve the manuscript.
Conflict of interest
The authors declare that the research was conducted in the absence of any commercial or financial relationships that could be construed as a potential conflict of interest.
Publisher’s note
All claims expressed in this article are solely those of the authors and do not necessarily represent those of their affiliated organizations, or those of the publisher, the editors and the reviewers. Any product that may be evaluated in this article, or claim that may be made by its manufacturer, is not guaranteed or endorsed by the publisher.
References
Addey C. I. (2022). Using Biogeochemical Argo floats to understand ocean carbon and oxygen dynamics. Nat. Rev. Earth Environ. 311, 739–739. doi: 10.1038/s43017-022-00341-5
Aldunate M., Henríquez-Castillo C., Ji Q., Lueders-Dumont J., Mulholland M. R., Ward B. B., et al. (2020). Nitrogen assimilation in picocyanobacteria inhabiting the oxygen-deficient waters of the eastern tropical North and South Pacific. Limnol. Oceanogr. 65, 437–453. doi: 10.1002/LNO.11315
Aldunate M., von Dassow P., Vargas C. A., Ulloa O. (2022). Carbon assimilation by the picoplanktonic community inhabiting the secondary chlorophyll maximum of the anoxic marine zones of the Eastern tropical North and South Pacific. Front. Mar. Sci. 9. doi: 10.3389/FMARS.2022.858308
Altieri A. H., Diaz R. J. (2019). “Dead Zones: Oxygen Depletion in Coastal Ecosystems,” in World Seas An Environ. Eval. Vol. III Ecol. Issues Environ. Impacts (London: Academic Press), 453–473. doi: 10.1016/B978-0-12-805052-1.00021-8
Behrenfeld M. J., Lorenzoni L., Hu Y., Bisson K. M., Hostetler C. A., Di Girolamo P., et al. (2023). Satellite lidar measurements as a critical new global ocean climate record. Remote Sens. 15, 5567. doi: 10.3390/RS15235567
Biller S. J., Berube P. M., Lindell D., Chisholm S. W. (2014). Prochlorococcus: the structure and function of collective diversity. Nat. Rev. Microbiol. 13, 13–27. doi: 10.1038/nrmicro3378
Biller S. J., Coe A., Roggensack S. E., Chisholm S. W. (2018). Heterotroph interactions alter prochlorococcus transcriptome dynamics during extended periods of darkness. mSystems 3(3):mSystems.00040-18. doi: 10.1128/MSYSTEMS.00040-18
Bittig H. C., Maurer T. L., Plant J. N., Wong A. P., Schmechtig C., Claustre H., et al. (2019). A BGC-Argo guide: Planning, deployment, data handling and usage. Front. Mar. Sci. 6. doi: 10.3389/FMARS.2019.00502
Breitburg D., Levin L. A., Oschlies A., Grégoire M., Chavez F. P., Conley D. J., et al. (2018). Declining oxygen in the global ocean and coastal waters. Science 359, eaam7240. doi: 10.1126/SCIENCE.AAM7240
Bristow L. A., Mohr W., Ahmerkamp S., Kuypers M. M. M. (2017). Nutrients that limit growth in the ocean. Curr. Biol. 27, R474–R478. doi: 10.1016/j.cub.2017.03.030
Callbeck C. M., Canfield D. E., Kuypers M. M. M., Yilmaz P., Lavik G., Thamdrup B., et al. (2021). Sulfur cycling in oceanic oxygen minimum zones. Limnol. Oceanogr. 66, 2360-2392. doi: 10.1002/lno.11759
Canfield D. E., Stewart F. J., Thamdrup B., De Brabandere L., Dalsgaard T., Delong E. F., et al. (2010). A cryptic sulfur cycle in Oxygen-Minimum-Zone waters off the Chilean coast. Science 330 (6009), 1375–1378. doi: 10.1126/science.1196889
Canfield D. E., Kraft B. (2022). The ‘oxygen’ in oxygen minimum zones. Environ. Microbiol. 24, 5332–5344. doi: 10.1111/1462-2920.16192
Cepeda-Morales J., Beier E., Gaxiola-Castro G., Lavín M. F., Godínez V. M. (2009). Effect of the oxygen minimum zone on the second chlorophyll maximum. Cienc. Mar. 35, 389–403. doi: 10.7773/CM.V35I4.1622
Chai F., Johnson K. S., Claustre H., Xing X., Wang Y., Boss E., et al. (2020). Monitoring ocean biogeochemistry with autonomous platforms. Nat. Rev. Earth Environ. 16, 315–326. doi: 10.1038/s43017-020-0053-y
Claustre H., Johnson K. S., Takeshita Y. (2020). Observing the global ocean with biogeochemical-argo. Ann. Rev. Mar. Sci. 12, 23–48. doi: 10.1146/ANNUREV-MARINE-010419-010956
Codispoti L. A., Brandes J. A., Christensen J. P., Devol A. H., Naqvi S. W. A., Paerl H. W., et al. (2001). The oceanic fixed nitrogen and nitrous oxide budgets: Moving targets as we enter the anthropocene?*. Sci. Mar. 65, 85–105. doi: 10.3989/SCIMAR.2001.65S285
Coe A., Ghizzoni J., LeGault K., Biller S., Roggensack S. E., Chisholm S. W. (2016). Survival of Prochlorococcus in extended darkness. Limnol. Oceanogr. 61, 1375–1388. doi: 10.1002/LNO.10302
Cornec M., Claustre H., Mignot A., Guidi L., Lacour L., Poteau A., et al. (2021). Deep chlorophyll maxima in the global ocean: occurrences, drivers and characteristics. Global Biogeochem. Cycles 35, e2020GB006759. doi: 10.1029/2020GB006759
Cox I., Brewin R. J. W., Dall’Olmo G., Sheen K., Sathyendranath S., Rasse R., et al. (2023). Distinct habitat and biogeochemical properties of low-oxygen-adapted tropical oceanic phytoplankton. Limnol. Oceanogr. 68 (9), 2022-2039. doi: 10.1002/LNO.12404
Dall’Olmo G., Bhaskar TVS U., Bittig H., Boss E., Brewster J., Claustre H., et al. (2023). Real-time quality control of optical backscattering data from Biogeochemical-Argo floats. Open Res. Eur. 2, 118. doi: 10.12688/OPENRESEUROPE.15047.2
Diaz R. J. (2001). Overview of hypoxia around the world. J. Environ. Qual. 30, 275–281. doi: 10.2134/JEQ2001.302275X
Engel A., Kiko R., Dengler M. (2022). Organic matter supply and utilization in oxygen minimum zones. Ann. Rev. Mar. Sci. 14, 355–378. doi: 10.1146/annurev-marine-041921-090849
Franz J., Krahmann G., Lavik G., Grasse P., Dittmar T., Riebesell U. (2012). Dynamics and stoichiometry of nutrients and phytoplankton in waters influenced by the oxygen minimum zone in the eastern tropical Pacific. Deep Sea Res. Part I Oceanogr. Res. Pap. 62, 20–31. doi: 10.1016/J.DSR.2011.12.004
Garcia H. E., Boyer T. P., Levitus S., Locarnini R. A., Antonov J. (2005). On the variability of dissolved oxygen and apparent oxygen utilization content for the upper world ocean: 1955 to 1998. Geophys. Res. Lett. 32, 1–4. doi: 10.1029/2004GL022286
Garcia H. E., Weathers K., Paver C. R., Smolyar I., Boyer T. P., Locarnini R.A., et al. (2019). World Ocean Atlas 2018, Volume 3: Dissolved Oxygen, Apparent Oxygen Utilization, and Oxygen Saturation. A. Mishonov Technical Ed.; NOAA Atlas NESDIS 83, 38pp.
Garcia-Robledo E., Padilla C. C., Aldunate M., Stewart F. J., Ulloa O., Paulmier A., et al. (2017). Cryptic oxygen cycling in anoxic marine zones. Proc. Natl. Acad. Sci. U. S. A. 114, 8319–8324. doi: 10.1073/PNAS.1619844114
Gilly W. F., Michael Beman J., Litvin S. Y., Robison B. H. (2013). Oceanographic and biological effects of shoaling of the oxygen minimum zone. Annual Review of Marine Science 5, 393–420. doi: 10.1146/ANNUREV-MARINE-120710-100849
Goericke R., Olson R. J., Shalapyonok A. (2000). A novel niche for Prochlorococcus sp. in low-light suboxic environments in the Arabian Sea and the Eastern Tropical North Pacific. Deep Sea Res. Part I Oceanogr. Res. Pap. 47, 1183–1205. doi: 10.1016/S0967-0637(99)00108-9
Gordon H. R., McCluney W. R. (1975). Estimation of the depth of sunlight penetration in the sea for remote sensing. Appl. Opt. 14, 413. doi: 10.1364/AO.14.000413
Grégoire M., Garçon V., Garcia H., Breitburg D., Isensee K., Oschlies A., et al. (2021). A global ocean oxygen database and atlas for assessing and predicting deoxygenation and ocean health in the open and coastal ocean. Front. Mar. Sci. 8, 724913. doi: 10.3389/FMARS.2021.724913
Groom S. B., Sathyendranath S., Ban Y., Bernard S., Brewin B., Brotas V., et al. (2019). Satellite ocean colour: Current status and future perspective. Front. Mar. Sci. 6, 441199. doi: 10.3389/FMARS.2019.00485
Hoegh-Guldberg O., Jacob D., Bindi M., Brown S., Camilloni I., Diedhiou A., et al. (2018). Impacts of 1.5°C Global Warming on Natural and Human Systems. In Global Warming of 1.5°C above pre-industrial levels and related global greenhouse gas emission pathways, in the context of strengthening the global response to the threat of climate change, sustainable development, and efforts to eradicate poverty. Masson-Delmotte V., Zhai P., Pörtner H.-O., Roberts D., Skea J., Shukla P.R., et al. (eds.)]. In Press.
Hostetler C. A., Behrenfeld M. J., Hu Y., Hair J. W., Schulien J. A. (2018). Spaceborne lidar in the study of marine systems. Ann. Rev. Mar. Sci. 10, 121. doi: 10.1146/ANNUREV-MARINE-121916-063335
Ito T., Minobe S., Long M. C., Deutsch C. (2017). Upper ocean O2 trends: 1958–2015. Geophys. Res. Lett. 44, 4214–4223. doi: 10.1002/2017GL073613
Johnson Z., Landry M. L., Bidigare R. R., Brown S. L., Campbell L., Gunderson J., et al. (1999). Energetics and growth kinetics of a deep Prochlorococcus spp. population in the Arabian Sea. Deep. Res. Part II Top. Stud. Oceanogr. 46, 1719–1743. doi: 10.1016/S0967-0645(99)00041-7
Karstensen J., Stramma L., Visbeck M. (2008). Oxygen minimum zones in the eastern tropical Atlantic and Pacific oceans. Prog. Oceanogr. 77, 331–350. doi: 10.1016/J.POCEAN.2007.05.009
Keeling R. F., Körtzinger A., Gruber N. (2010). Ocean deoxygenation in a warming world. Ann. Rev. Mar. Sci. 2, 199–229. doi: 10.1146/ANNUREV.MARINE.010908.163855
Lam P., Kuypers M. M. M. (2011). Microbial nitrogen cycling processes in oxygen minimum zones. Ann. Rev. Mar. Sci. 3, 317–345. doi: 10.1146/ANNUREV-MARINE-120709-142814
Lavin P., González B., Santibáñez J. F., Scanlan D. J., Ulloa O. (2010). Novel lineages of Prochlorococcus thrive within the oxygen minimum zone of the eastern tropical South Pacific. Environ. Microbiol. Rep. 2, 728–738. doi: 10.1111/j.1758-2229.2010.00167.x
Li C., Huang J., Ding L., Liu X., Yu H., Huang J. (2020). Increasing escape of oxygen from oceans under climate change. Geophys. Res. Lett. 47, e2019GL086345. doi: 10.1029/2019GL086345
Liu T., Qiu Y., Lin X., Ni X., Wang L., Li H., et al. (2024). Dissolved oxygen recovery in the oxygen minimum zone of the arabian sea in recent decade as observed by BGC-argo floats. Geophys. Res. Lett. 51, e2024GL108841. doi: 10.1029/2024GL108841
Long M. C., Ito T., Deutsch C. (2019). “Oxygen projections for the future,” in Ocean deoxygenation: Everyone’s problem - Causes, impacts, consequences and solutions, (Gland, Switzerland: IUCN), 171–212.
Long A. M., Jurgensen S. K., Petchel A. R., Savoie E. R., Brum J. R. (2021). Microbial ecology of oxygen minimum zones amidst ocean deoxygenation. Front. Microbiol. 12. doi: 10.3389/FMICB.2021.748961
Márquez-Artavia A., Sánchez-Velasco L., Barton E. D., Paulmier A., Santamarı́a-Del-Ángel E., Beier E. (2019). A suboxic chlorophyll-a maximum persists within the Pacific oxygen minimum zone off Mexico. Deep. Res. Part II Top. Stud. Oceanogr. 169–170, 104686. doi: 10.1016/j.dsr2.2019.104686
Matear R. J., Hirst A. C. (2003). Long‐term changes in dissolved oxygen concentrations in the ocean caused by protracted global warming. Cycles 17, 1125. doi: 10.1029/2002GB001997
Mignot A., Claustre H., D’Ortenzio F., Xing X., Poteau A., Ras J. (2011). From the shape of the vertical profile of in vivo fluorescence to Chlorophyll-a concentration. Biogeosciences 8, 2391–2406. doi: 10.5194/bg-8-2391-2011
Mignot A., Claustre H., Uitz J., Poteau A., D’Ortenzio F., Xing X. (2014). Understanding the seasonal dynamics of phytoplankton biomass and the deep chlorophyll maximum in oligotrophic environments: A Bio-Argo float investigation. Global Biogeochem. Cycles 28, 856–876. doi: 10.1002/2013GB004781
Moore L. R., Rocap G., Chisholm S. W. (1998). Physiology and molecular phylogeny of coexisting Prochlorococcus ecotypes. Nat. 393, 464–467. doi: 10.1038/30965
Morris J. J., Kirkegaard R., Szul M. J., Johnson Z. I., Zinser E. R. (2008). Facilitation of robust growth of Prochlorococcus colonies and dilute liquid cultures by “helper” heterotrophic bacteria. Appl. Environ. Microbiol. 74, 4530–4534. doi: 10.1128/AEM.02479-07
Morel A., Huot Y., Gentili B., Werdell P. J., Hooker S. B., Franz B. A. (2007). Examining the consistency of products derived from various ocean color sensors in open ocean (Case 1) waters in the perspective of a multi-sensor approach. Remote Sens. Environ. 111, 69–88. doi: 10.1016/J.RSE.2007.03.012
Mühling M. (2012). On the culture-independent assessment of the diversity and distribution of Prochlorococcus. Environ. Microbiol. 14, 567–579. doi: 10.1111/j.1462-2920.2011.02589.x
Organelli E., Dall’Olmo G., Brewin R. J. W., Tarran G. A., Boss E., Bricaud A. (2018). The open-ocean missing backscattering is in the structural complexity of particles. Nat. Commun. 2018 91 9, 1–11. doi: 10.1038/s41467-018-07814-6
Oschlies A. "Ocean deoxygenation from climate change,". in Ocean deoxygenation:Everyone’s problem - Causes, impacts, consequences and solutions (Gland, Switzerland: ICUN), 105–116.
Oschlies A., Brandt P., Stramma L., Schmidtko S. (2018). Drivers and mechanisms of ocean deoxygenation. Nat. Geosci. 11, 467–473. doi: 10.1038/s41561-018-0152-2
Petit F., Uitz J., Schmechtig C., Dimier C., Ras J., Poteau A., et al. (2022). Influence of the phytoplankton community composition on the in situ fluorescence signal: Implication for an improved estimation of the chlorophyll-a concentration from BioGeoChemical-Argo profiling floats. Front. Mar. Sci. 9. doi: 10.3389/FMARS.2022.959131
Ravichandran M., Girishkumar M. S., Riser S. (2012). Observed variability of chlorophyll-a using Argo profiling floats in the southeastern Arabian Sea. Deep. Res. Part I Oceanogr. Res. Pap. 65, 15–25. doi: 10.1016/j.dsr.2012.03.003
Reid J. L. (1965). Intermediate Waters of the Pacific Ocean (Oceanographic Study) (Maryland: The Johns Hopkins University Press).
Roth-Rosenberg D., Aharonovich D., Luzzatto-Knaan T., Vogts A., Zoccarato L., Eigemann F., et al. (2020). Prochlorococcus cells rely on microbial interactions rather than on chlorotic resting stages to survive long-term nutrient starvation. MBio 11, 1–13. doi: 10.1128/MBIO.01846-20
Sarma V. V. S. S., Udaya Bhaskar T. V. S., Pavan Kumar J., Chakraborty K. (2020). Potential mechanisms responsible for occurrence of core oxygen minimum zone in the north-eastern Arabian Sea. Deep. Res. I 165, 103393. doi: 10.1016/j.dsr.2020.103393
Sarmiento J. L., Gruber N. (2006). Ocean Biogeochemical Dynamics (Princeton: Princeton University Press). doi: 10.2307/j.ctt3fgxqx
Sauzède R., Lavigne H., Claustre H., Uitz J., Schmechtig C., D’Ortenzio F., et al. (2015). Vertical distribution of chlorophyll a concentration and phytoplankton community composition from in situ fluorescence profiles: A first database for the global ocean. Earth Syst. Sci. Data 7, 261–273. doi: 10.5194/essd-7-261-2015
Schmidtko S., Johnson G., Lymann J. (2013). Monthly Isopycnal/Mixed-layer Ocean Climatology (MIMOC). EGU Gen. Assem. Conf. Abstr. 15, 11972. Available at: http://adsabs.harvard.edu/abs/2013EGUGA..1511972S%5Cnpapers3://publication/uuid/38463872-723D-4C39-B3A3-6CC16D50401A
Schmidtko S., Stramma L., Visbeck M. (2017). Decline in global oceanic oxygen content during the past five decades. Nat. 2017 5427641 542, 335–339. doi: 10.1038/nature21399
Seliger H. H., Loftus M. E. (1974). Growth and dissipation of phytoplankton in chesapeake bay. II. A statistical analysis of phytoplankton standing crops in the rhode and west rivers and an adjacent section of the chesapeake bay I. Chesapeake Science 15, 185-204. doi: 10.2307/1350969
Tiano L., Garcia-Robledo E., Dalsgaard T., Devol A. H., Ward B. B., Ulloa O., et al. (2014). Oxygen distribution and aerobic respiration in the north and south eastern tropical Pacific oxygen minimum zones. Deep. Res. Part I Oceanogr. Res. Pap. 94, 173–183. doi: 10.1016/j.dsr.2014.10.001
Uitz J., Laustre H., Orel A., Hooker S. B. (2006). Vertical distribution of phytoplankton communities in open ocean: An assessment based on surface chlorophyll. J. Geophys. Res. 111, 8005. doi: 10.1029/2005JC003207
Ulloa O., Canfield D. E., DeLong E. F., Letelier R. M., Stewart F. J. (2012). Microbial oceanography of anoxic oxygen minimum zones. Proc. Natl. Acad. Sci. U. S. A. 109, 15996–16003. doi: 10.1073/PNAS.1205009109
Ulloa O., Henríquez-Castillo C., Ramírez-Flandes S., Plominsky A. M., Murillo A. A., Morgan-Lang C., et al. (2021). The cyanobacterium Prochlorococcus has divergent light-harvesting antennae and may have evolved in a low-oxygen ocean. Proc. Natl. Acad. Sci. U. S. A. 118, e2025638118. doi: 10.1073/PNAS.2025638118
Vallivattathillam P., Lachkar Z., Lévy M. (2023). Shrinking of the Arabian Sea oxygen minimum zone with climate change projected with a downscaled model. Front. Mar. Sci. 10. doi: 10.3389/FMARS.2023.1123739
Whitmire A. L., Letelier R. M., Villagrán V., Ulloa O. (2009). Autonomous observations of in vivo fluorescence and particle backscattering in an oceanic oxygen minimum zone. Opt. Express 17, 21992–22004. doi: 10.1364/OE.17.021992
Winder M., Sommer U. (2012). Phytoplankton response to a changing climate. Hydrobiol. 698, 5–16. doi: 10.1007/S10750-012-1149-2
Wong J. C. Y., Raven J. A., Aldunate M., Silva S., Gaitán-Espitia J. D., Vargas C. A., et al. (2023). Do phytoplankton require oxygen to survive? A hypothesis and model synthesis from oxygen minimum zones. Limnol. Oceanogr. 68 (7), 1417-1437. doi: 10.1002/LNO.12367
Wong A. P. S., Wijffels S. E., Riser S. C., Pouliquen S., Hosoda S., Roemmich D., et al. (2020). Argo data 1999–2019: two million temperature-salinity profiles and subsurface velocity observations from a global array of profiling floats. Front. Mar. Sci. 7. doi: 10.3389/FMARS.2020.00700
Wright J. J., Konwar K. M., Hallam S. J. (2012). Microbial ecology of expanding oxygen minimum zones. Nat. Rev. Microbiol. 10, 381–394. doi: 10.1038/nrmicro2778
Wu Y., Bakker D. C. E., Achterberg E. P., Silva A. N., Pickup D. D., Li X., et al. (2022). Integrated analysis of carbon dioxide and oxygen concentrations as a quality control of ocean float data. Commun. Earth Environ. 3, 1–11. doi: 10.1038/s43247-022-00421-w
Keywords: phytoplankton, oxygen, monitoring, climate change, cyanobacteria
Citation: Cox I, Brewin RJW, Sheen K, Dall’Olmo G and Ulloa O (2024) Monitoring low-oxygen-adapted subsurface phytoplankton distribution in a changing ocean. Front. Mar. Sci. 11:1425250. doi: 10.3389/fmars.2024.1425250
Received: 29 April 2024; Accepted: 03 September 2024;
Published: 04 October 2024.
Edited by:
Sanjeev Kumar, Physical Research Laboratory, IndiaReviewed by:
Haimanti Biswas, Council of Scientific and Industrial Research (CSIR), IndiaMichael Behrenfeld, Oregon State University, United States
Copyright © 2024 Cox, Brewin, Sheen, Dall’Olmo and Ulloa. This is an open-access article distributed under the terms of the Creative Commons Attribution License (CC BY). The use, distribution or reproduction in other forums is permitted, provided the original author(s) and the copyright owner(s) are credited and that the original publication in this journal is cited, in accordance with accepted academic practice. No use, distribution or reproduction is permitted which does not comply with these terms.
*Correspondence: Isabelle Cox, aWMzMTRAZXhldGVyLmFjLnVr