- GEOMAR, Helmholtz Centre for Ocean Research Kiel, Kiel, Germany
The Skagerrak basin represents the main sink area for fine-grained sediment in the North Sea region and constitutes a natural deposition center for sediments that are supplied from the Atlantic, the Baltic Sea and the surrounding continental margins and coasts. However, the exact sources and their proportional contributions to the North Sea sediments and to the Skagerrak deposits are not well understood. To trace the predominant sources of the sediment and to gain a better understanding of the sedimentary processes in the North Sea and the Skagerrak basin, radiogenic Sr, Nd, and Hf isotope signatures and clay mineral compositions of the detrital clay fraction of surface sediment samples from the North Sea, the Scandinavian margins and the Baltic Sea were measured. The results indicate that the major source for Skagerrak clay-size sediments is the northern North Sea but Scandinavia as well as the southern North Sea including the southern England coast also contribute material. Seabed and coastal erosion in the northern North Sea are enhanced by the inflowing Atlantic Currents, which provide the Skagerrak with high amounts of clay size sediments. In contrast, the southern North Sea, the Baltic Sea and mid-European rivers such as Weser, Elbe and Ems are only minor contributors. As Skagerrak deposits are dominated by clay sized material (up to 60%), the reconstructed sediment processes in this study deviate from findings in previous sediment budget studies, which were based on both clay and silt fraction and indicated predominant influences from the southern North Sea. These results highlight that coastal and seabed erosion in the North Sea is a previously underestimated source of fine-grained sediments for depocenters in the entire North Sea. With regard to climate change, the global sea-level rise will likely enhance erosional processes and can therefore significantly influence the sediment budget of the entire North Sea.
1 Introduction
1.1 The sedimentary system of the North Sea
The Skagerrak basin represents a major natural deposition center for fine-grained sediments supplied from various regions of the North Sea. More than half of the sediments initially deposited in the entire North Sea finally end up in the Skagerrak region following current-driven transport (van Weering, 1981; Eisma and Irion, 1988; de Haas and van Weering, 1997).
At the same time, the sediment dynamics of the North Sea are heavily affected by anthropogenic processes, i.e., bottom trawling, land reclamation and sand mining, which may lead to enhanced bottom current velocities and sediment erosion (O’Neill and Ivanović, 2016). Riverine sediment input into the North Sea has been drastically reduced due to constructions of dams and dredging activities in particular affecting the large European rivers Rhine and Elbe (Puls et al., 1997; Rovira et al., 2014). About one billion tons of dredged material is dumped in different areas of the North Sea annually (OSPAR, 2017) which significantly influences the sediment balance and dynamics of the North Sea.
Moreover, climate forcing factors such as increasing storm intensity and wave activity have particularly changed sediment dynamics in the shallow areas of the North Sea. These anthropogenic and climate-driven effects have changed the sediment budget in the North Sea (Fettweis et al., 2010), which can be observed in large depocenters where the particles settle down.
The distribution of fine-grained sediments, their main sediment transport pathways, and potential sources in the North Sea have been the subject of various studies (van Weering, 1981; Eisma and Kalf, 1987; Irion and Zöllmer, 1999). The terrigenous mud is transported and redistributed across the shelf by the water masses of the North Sea and finally accumulates in depocenters where low bottom current velocities are prevailing (de Haas et al., 2002). The suspended particulate matter (SPM) in the North Sea originates from the Atlantic Ocean, European rivers, coastal erosion, the Baltic Sea as well as seafloor erosion. Sediment deposition predominantly occurs in the Skagerrak, the Norwegian Channel, the Helgoland Mud Area (HMA), tidal flats and river estuaries (Eisma, 1981; Eisma and Kalf, 1987; van Weering et al., 1987, 1993; Pohlmann and Puls, 1994; Oost et al., 2021).
Several provenance studies have been published for the North Sea, which have mainly been based on clay mineral assemblages and heavy metal distributions in surface sediments (Zöllmer and Irion, 1993; Bengtsson and Stevens, 1998; Irion and Zöllmer, 1999; Lepland et al., 2000). These studies showed that the clay mineral distribution reflects the regional current patterns and indicates a predominant supply of sediments originating from the southern North Sea and the Atlantic, and to a minor extent from the Baltic Sea (Kuijpers et al., 1993; Pederstad et al., 1993; Bengtsson and Stevens, 1996). However, the main sediment contributors and their precise proportions in the Skagerrak are still not fully understood and has not been quantified yet. Furthermore, it is still unclear how anthropogenic impacts affect the sediment transport processes and sediment budget in the North Sea at present. As the main depocenter, the Skagerrak area receives sedimentary inputs from the entire North Sea. Given that multiple impact factors have influenced the North Sea sedimentation in the last century, it is crucial to understand the main present-day transport processes as well as the predominant sources of Skagerrak sediments. This knowledge, will allow the interpretation of down-core data of Skagerrak sediments in studies to investigate temporal changes of the sediment provenance and their driving factors over the last century or further back in time. This will help to evaluate whether and to what extent the sedimentary system in the North Sea has changed over time due to enhanced human activities and climate changes and may help to distinguish between these two impact factors. Consequently, it is crucial to determine and quantify the end-members contributing today to the main depocenter of the North Sea.
A wide range of methods exist that can be applied to identify sediment sources and quantify their contributions. They include the quantitative distribution of clay minerals (Bengtsson and Stevens, 1998; Gingele et al., 2001; Bout-Roumazeilles et al., 2013), the analyses of heavy mineral content (Luepke and Clifton, 1983; Bengtsson and Stevens, 1996; Lang and Stevens, 1999), sedimentological methods including grain-size analyses of the bulk composition (Garzanti et al., 2009), and geochemical analyses based on radiogenic isotope compositions (Kessarkar et al., 2003; Ehlert et al., 2011; Bretschneider et al. (2021a; b).
Hence, radiogenic isotope signatures of clay size sediments and clay mineral compositions represent two promising sediment provenance tracers and their combination provides a tool to gain detailed insight into characterizing the source rock and the sediment transport pathways. This enables the reconstruction of precise proportions of the different sediment contributors applying end-member modelling.
The radiogenic Nd and Sr isotope systems are primarily controlled by the age and type of the continental rocks (Dasch, 1969; Goldstein and Jacobsen, 1987; Grousset et al., 1988; Revel et al., 1996; Colin et al., 1999). The 87Sr/86Sr and 143Nd/144Nd compositions of detrital sediments have thus been shown to be a powerful tracer to identify source areas and their mixing relationships and have been widely used to trace sediment provenance (Ehlert et al., 2011; Liu et al., 2016; Yu et al., 2019; Bretschneider et al. (2021a; b).
In addition to the bulk sediment provenance, the radiogenic Sr isotope signatures are controlled by the degree of isotopic fractionation during chemical weathering due to different mineral phases of rocks exhibiting variations in the 87Sr/86Sr compositions. This can lead to large variations in radiogenic Sr isotope compositions between different mineral phases and grain sizes and consequently result in differences between the Sr isotope signatures of the bulk source rocks and the transported sediment (Eisenhauer et al., 1999; Blum and Erel, 2003). Many studies have also applied radiogenic 176Hf/177Hf signatures to reconstruct sediment transport pathways taking into account that the Hf isotope signature of the bulk source rock is also modified during sedimentation and is influenced by incongruent weathering and the zircon effect (Patchett et al., 1984; Bayon et al., 2009). Large amounts of Hf are contained in zircons, which are resistant to chemical weathering processes and are preferentially incorporated into coarser-grained sediments leading to less radiogenic Hf isotope signatures (Patchett et al., 1984). Investigations of the grain-size fractions revealed that radiogenic isotopes are useful source tracers, in particular when the same grain size fractions, e.g. clays, are considered in provenance studies (Walter et al., 2000).
In this study, we present radiogenic Nd, Sr and Hf isotope as well as clay mineral compositions of clay-sized surface sediments from the North Sea region to identify the sources of the detrital sediments and their mixing. We concentrate on the clay fraction (< 2 µm) since it can be transported by ocean currents over long distances and in contrast to bulk sediment, it is not biased by grain-size sorting effects. We then evaluate the dominant sediment transport pathways in the entire North Sea and quantify the sources of sediments deposited in the main depocenter, the Skagerrak.
1.2 Oceanographic and geographic setting
The North Sea is a marginal sea on the continental shelf of north-west Europe that can be divided into a deep northern and shallow southern part (Otto et al., 1990). The deeper northern part has a mean depth of 60 m and is geographically constrained by the Fladen Ground and the Norwegian Channel. The shallow southern part is dominated by the glacially formed Dogger Bank and Oyster Grounds as well as tidally generated sand banks (e.g. Norfolk Banks, Flemish Banks). The water depth in this area varies between 20 and 60 m and is on average 40 m. The sea floor basement mainly consists of Quaternary deposits with overlying reworked Holocene sediments (Stabell and Thiede, 1985; von Haugwitz and Wong, 1993). High mud contents are located in the deeper parts of the North Sea where current velocities decrease and bed shear stress is low, e.g. the Norwegian Channel, the Skagerrak, the Helgoland Mud Area (HMA), river estuaries, tidal flats, and the area southwest of the Doggerbank (Eisma and Kalf, 1987). Large areas of mud deposition are also located in the Fladen Ground and Oyster Ground where recent sedimentation of fine-grained sediments is restricted and fine-grained reworked early Holocene deposits prevail (Johnson and Elkins, 1979; Eisma, 1981; Bockelmann et al., 2018).
The Skagerrak basin represents the main depocenter for fine-grained sediments with annual mass accumulation rates of 28 - 46 Mt (van Weering et al., 1987; de Haas and van Weering, 1997; Spiegel et al., 2024). It is located in the northeastern part of the North Sea between Denmark, Norway and Sweden with a mean water depth of 210 m and a maximum depth of 700 m in its central part and forms a connection with the shallow North Sea and the Baltic Sea (Figure 1). The Skagerrak sediments are characterized by fine-grained sediments comprising the silt and clay fractions with small amounts of sandy material near the coastline (Stevens et al., 1996). In the deeper part of the Skagerrak basin the sediments contain clay contents above 50%, which correlate positively with depth (Pederstad et al., 1993; Bengtsson and Stevens, 1998).
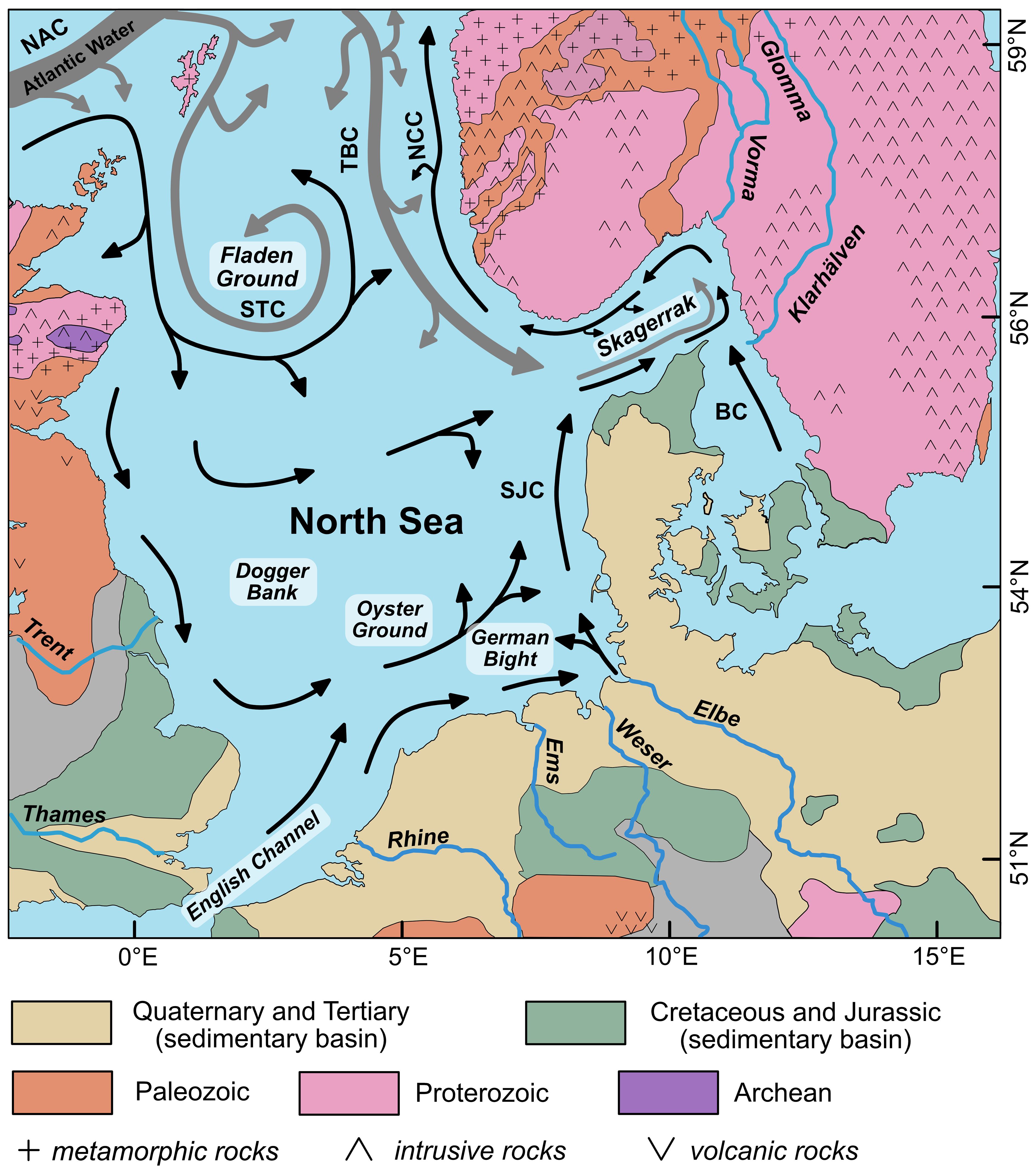
Figure 1 Schematic geological map of eastern Great Britain and western Europe modified after Bouysse (2014) together with major river systems and the circulation pattern of the North Sea modified after Turrell (1992) including main currents: NAC, North Atlantic Current; STC, Southern Trench Current; TBC, Tampen Bank Current; NCC, Norwegian Continental Current; NJC, North Jutland Current; SJC, South Jutland Current; and BC, Baltic Current. Grey arrows indicate the direct inflow of bottom water from the Atlantic, and black arrows show the coastal North Sea currents as surface waters.
1.3 Circulation pattern
The water circulation of the North Sea is primarily controlled by the North Atlantic Ocean and tidal forcing but also wind-driven currents play a role (Böhnecke, 1922; Furnes, 1980). The Atlantic water masses enter the North Sea from the north between the British and Norwegian coast and from the south-west through the English Channel (Figure 1). The Atlantic inflow causes an anti-clockwise circulation in the North Sea which dominates the transport processes of suspended matter along the North Sea coast. The North Atlantic inflow, which generally represents bottom waters, can be separated into three distinct flow paths: the Orkneys-Shetland region, the Shetland shelf area and the western part of the Norwegian Trench (Figure 1). Parts of the Atlantic water masses flow south along the east coast of England via the Southern Trench Current (STC) and continue eastward through the central North Sea into the Skagerrak (Rodhe, 1998). In contrast, the Atlantic surface water masses flowing westward in the Norwegian Trench as the Tampen Bank Current (TBC) is advected directly into the Skagerrak (Rodhe, 1998).
The southern North Sea is mainly influenced by the inflow of Atlantic water masses through the English Channel that follows the southern coastline where the mouths of large rivers draining central Europe (e.g. Rhine and Elbe) are located. The SPM supplied by those rivers and the Atlantic Ocean in the southern North Sea is transported northward along the Danish coast by the Jutland Current (JC) to the Skagerrak (Eisma and Kalf, 1987). When the waters from the Atlantic and the Jutland Current reach the inner part of the Skagerrak, they encounter the water masses of the Baltic Current (BC) which flows northward within the Kattegat between Denmark and Sweden. This results in a counter-clockwise circulation in the Skagerrak flowing northwards along the Swedish and Norwegian coastline as the Norwegian Coastal Current (NCC) and entering the Atlantic via the Norwegian Channel (Longva and Thorsnes, 1997).
1.4 Geological setting
The geological structure of the hinterland of the North Sea is highly diverse (Figure 1). The Fennoscandian shield forms the northern part of Europe (Scandinavia) and mainly consists of Precambrian bedrock representing the oldest rocks of the European continent (Kärki et al., 1993; Huhma et al., 2004). It includes metamorphic rocks with several generations of granitoids as well as metasedimentary and metavolcanic rocks. The oldest rocks are Archean gneisses and greenstone belts that crop out in the Kola peninsula and north-eastern Finland, where also some Paleoproterozoic rocks prevail (Gaál and Gorbatschev, 1987). The bedrock of Great Britain largely consists of metamorphic Precambrian and older Palaeozoic rocks whereby Scotland and Scandinavia shows similar geological settings that were separated by the Mesozoic rifting of Great Britain to the west (Hallam, 1971). The southern part of England consists of younger Jurassic, Cretaceous and Tertiary rocks representing parts of a former sedimentary basin (Figure 1). Compared to northern Europe, the geology of central Europe, in particular the sedimentary sequences of the coastal hinterland of the southern North Sea are much younger and of Quaternary origin (Pawlewicz et al., 2002). Furthermore, in parts of central Europe, Cenozoic volcanic rocks and Mesozoic sedimentary rocks crop out, which are drained by the Mid-European rivers that finally enter the coastal areas of the North Sea (Bernet et al., 2004).
2 Material and methods
2.1 Sample preparation
In total, 71 surface sediment samples (Supplementary Figure S1) in most cases the uppermost 0–5 cm subsampled from multi- and box corers) were analyzed for radiogenic Sr, Nd, and Hf isotope compositions of the clay-size fraction, as well as 32 surface samples for semiquantitative clay mineral compositions. The sample locations cover the entire North Sea and the western part of the Baltic Sea and thus all potential sediment sources.
2.2 Radiogenic isotopes
The sediment samples were freeze-dried and sieved through a 63 µm sieve to collect the fine-fraction in plastic bags. This was leached with a 0.05 M hydroxylamine hydrochloride (HH) – 15% acetic acid – 0.03 M Na-EDTA leaching solution to remove authigenic phases. Authigenic Fe-Mn oxyhydroxide phases were removed in a separate step following the modified leaching procedure described by Gutjahr et al. (2007). The sediments were first washed twice to remove the easily soluble fractions. Every rinsing step included the suspension of the sediment in deionized water and centrifugation to decant the supernatant. For decarbonization, the samples were treated with 40% acetic acid. This step was repeated until no reaction was visible anymore. Afterwards, the samples were treated with H2O2 to destroy the organic matter. The samples were rinsed three times and then the clay fraction (< 2 µm) was separated using a centrifuge-based Atterberg-method. The clay fraction was split into two aliquots for radiogenic isotope and clay mineral analyses. For radiogenic isotope analyses, the samples were leached with the HH leaching solution overnight to remove the remaining Fe-Mn oxyhydroxides and washed three times with deionized water.
After the leaching procedure, the samples were dried at 40°C in an oven. The clay fraction was completely digested using the alkaline fusion method described by Bayon et al. (2009). For separation and purification of Sr, Nd, and Hf, standard ion chromatographic procedures were applied (Cohen et al., 1988; Galer and O’Nions, 1989; Pin and Zalduegui, 1997). Sr was separated from matrix elements using 50 µl Eichrom Sr-Spec resin (50–100 µm mesh; Horwitz et al., 1992). Nd and Hf were separated from the other rare earth elements (REE) and other matrix elements using a cation exchange resin (Bio-Rad AG 50W-X8, 200–400 µm mesh). Then Nd was separated from the other REEs using 2 ml Eichrom Ln-Spec resin (50–100 µm mesh; Pin and Zalduegui, 1997). Hf was separated using 1.6 ml of Bio-Rad AG1-X8 resin (200–400 µm mesh; Sahoo et al., 2006).
The radiogenic isotope measurements of Sr, Nd, and Hf were performed on a Nu Plasma high-resolution multi-collector inductively coupled plasma mass spectrometer (MC-ICP-MS) located at GEOMAR Helmholtz Centre for Ocean Research Kiel in Germany. Before measuring the isotope compositions, the concentrations of every sample solution were determined on an aliquot of the sample solution collected after the purification procedure. Prior to the measurements, the samples were diluted to the same concentrations (50 ppb for high concentration samples and 10 ppb for lower concentration samples) as the standards, which were measured repeatedly between samples.
The instrumental mass bias was corrected by using 88Sr/86Sr of 0.1194 (Steiger and Jäger, 1977) and normalized to the NIST SRM 987 87Sr/86Sr value 0.710245. Procedural blanks contributed between 0.6 and 18.9% of the total Sr signal, whereby most of the samples show blank contributions below 8%. This high blank contribution originates from the sodium hydroxide (NaOH) and sodium peroxide (NaO2) used for the alkaline fusion. For the blank correction, the Sr concentrations and isotopic compositions of the blanks were measured. The calculated blank contribution was then removed from the total Sr isotope signal of the sample by mass balance calculation following Bretschneider et al. (2021a;b). Repeated measurements of the processed USGS rock reference material STM-1 (n = 5) gave a mean 87Sr/86Sr value of 0.703838 (+/- 0.000104), which is in good agreement with the average value of 0.703701 (+/- 0.000006) of the GeoReM database (Weis et al., 2006).
The measured Nd isotope ratios were mass bias corrected using a 146Nd/144Nd of 0.7219 (Vance and Thirlwall, 2002) and normalized to the JNdi-1 standard value 0.512115 (Tanaka et al., 2000). The Nd isotope ratios are reported as εNd values due to the small differences in 143Nd/144Nd ratios between samples, which are calculated using the following equation (Jacobsen and Wasserburg, 1980):
where CHUR is the present day chondritic 143Nd/144Nd value of 0.512638. Procedural blanks for Nd were not higher than 0.8% of the total amount of Nd in the samples. Repeated measurements of the USGS rock reference material STM-1 (n = 5) show a mean εNd value of 5.42 (+/- 0.48), which agrees well with the mean value of 5.36 (+/- 0.18) provided by Weis et al. (2006).
The Hf isotope ratios were corrected for instrumental mass bias using a 179Hf/177Hf of 0.7325 and then normalized to a JMC 475 value of 0.282163 (Blichert-Toft and Albarède, 1997; Blichert-Toft, 2008). The Hf isotope compositions are reported as εHf values analogous to the εNd values using a present day chondritic 176Hf/177Hf value of 0.282785 (Bouvier et al., 2008). Procedural blanks for Hf were not higher than 2.9% of the total amount of Hf in the samples. Repeated measurements of the USGS rock reference material STM-1 (n = 5) give a mean εHf value of 8.44 (+/- 0.68), which agrees well with the mean value of 8.36 (+/-0.79) given in the GeoReM database (Jochum et al., 2005).
2.3 Clay minerals
For clay mineral analyses, subsamples were taken from the clay-size fraction obtained from the Atterberg separation. Pedogenic iron oxides of the clays were removed using 0.3 M sodium citrate, 0.2 M sodium hydrocarbonate (NaHCO3) and sodium dithionite. The dissolved iron compounds were washed out four times with 2M sodium chloride. 1/3 of the clay suspension was saturated and flocculated with an excess of K+-ions and 2/3 of the clay suspension/solution with an excess of Mg++-ions. The ion excess of both saturated clay suspensions was removed via dialysis tubes with deionized water to electrical conductivity values below 20 µS. The K- and Mg- saturated samples were freeze-dried and 50 mg of the respective clay fraction was dispersed in 4 ml deionized water in an ultrasonic bath for 10 min. A subsample of 2 ml of the suspension was placed on a ceramic slide/plate and was dried for 48 hours in preparation of the measurement. Clay mineral measurements were performed at the Institute of Geosciences of the University of Kiel (CAU) by x-ray diffractometry (XRD) using a D8 Discover (Bruker AXS) with a Cu-cube as well as θ-θ-geometry. Samples were measured with 2θ at 0.037° steps with a scanning speed of 1.5 s at each step. The measurement was repeated after the oriented Mg-saturated sample slide was treated with ethylene glycol saturation and the K-saturated sample was heated to 550°C for two hours.
The analyses of the diffractograms were performed via the open-source software Profex using the BMBG and COD database for identifying the mineral phases. Four main groups of clay mineral phases, illite, kaolinite, chlorite and smectite were differentiated. The semi-quantitative analyses of the clay mineral content were carried out by determining the peak areas of the respective mineral phases and were calculated following Whitton and Churchman (1987) and by using the weighting factors recommended by Laves and Jähn (1972).
3 Results
3.1 Radiogenic isotope characterization of the clay-size surface sediments
The 87Sr/86Sr, εNd and εHf signatures of the detrital clay fraction (< 2 µm) of the surface samples across the entire North Sea are reported in Supplementary Table S1 and Figure 2, respectively. The Sr isotope signatures range from 0.726905 to 0.775977 and show the most radiogenic values in the Scandinavian fjords and the Baltic Sea. Less radiogenic Sr isotope ratios are found in the southern North Sea, especially in the samples originating from European estuaries (Weser, Ems, Elbe).
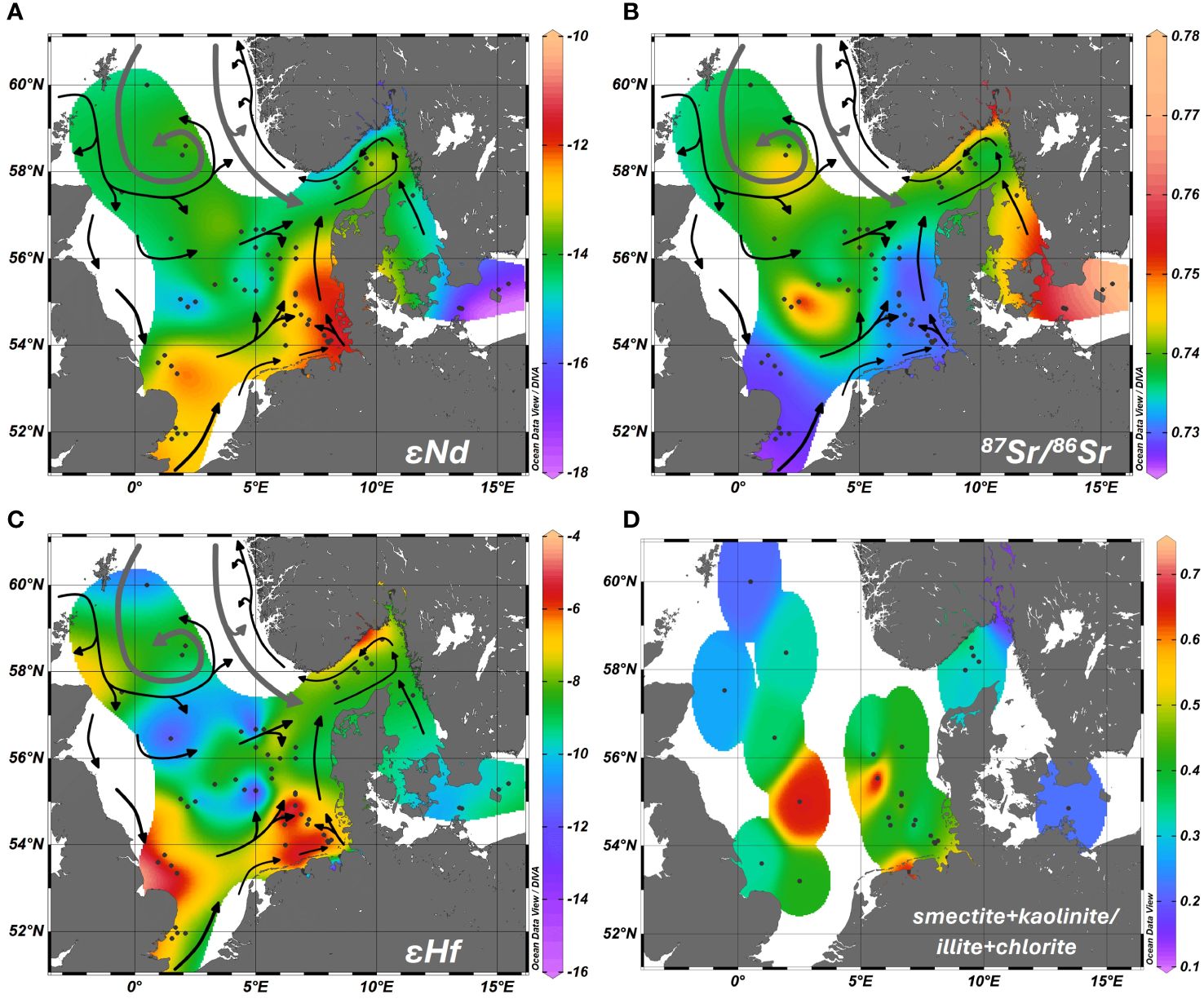
Figure 2 Distribution of radiogenic isotopes of the clay-sized surface samples in the North Sea with circulation patterns: (A) εNd (B) 87Sr/86Sr (C) εHf (D) Clay mineral distribution of the secondary and primary clay minerals (smectite+kaolinite/illite+chlorite). Black dots indicate the sample locations. Black arrows indicate the subsurface currents and grey arrows mark the Atlantic deep water inflow. The maps were generated by using ODV software, version 5.6.3 (Schlitzer, Reiner, Ocean Data View, https://odv.awi.de, 2021).
The εNd values range between -17.7 and -10.6 and show the least radiogenic values in the Baltic Sea and Scandinavian rivers and most radiogenic values in the southern North Sea and German river estuaries. The εHf signatures vary between -15.2 and -4.8 with the least radiogenic values in the central North Sea and the highest values in the southern North Sea. However, some sediment samples from the Weser and Ems estuaries also show low εHf signatures between -6.7 and -5.7.
The isotopic signatures of the surface samples collected from the sediment basin of the Skagerrak range between intermediate εNd values of -14.6 to -13.4, between 0.73875 and 0.74260 for the 87Sr/86Sr isotopic compositions and are comparable to the average isotopic values of the surface sediment samples. Similarly, the εHf signatures of the Skagerrak surface sediments are close to the average North Sea values ranging between -8.4 and -7.0. The surface samples from the northern North Sea reflecting the Atlantic inflow into the northern North Sea are also characterized by intermediate εNd values of around -14 and 87Sr/86Sr ratios around 0.74.
3.2 Clay mineralogical composition of the surface samples
The smectite distribution in the North Sea shows a clear pattern with highest contents in the sediments of the southern North Sea and the Central-European rivers with a maximum value of 32.3% in the Ems estuary and a decreasing trend along the west coast of Denmark with lowest values in the Skagerrak between 4.48 and 8.37% (average of 13.7; Supplementary Table S2; Figure 3). The surface samples in the northern North Sea which likely reflect the Atlantic input reveal contents similar to the Skagerrak. Compared to the smectite distribution the kaolinite content in the North Sea reveals smaller variations between 7.2% and 22.1% (average of 14.3%). The dominant clay mineral contents of illite and chlorite exhibit values ranging from 38.8 to 67.9% (average of 53.1%) and 3.7 and 38.18% (average of 18.9%), respectively.
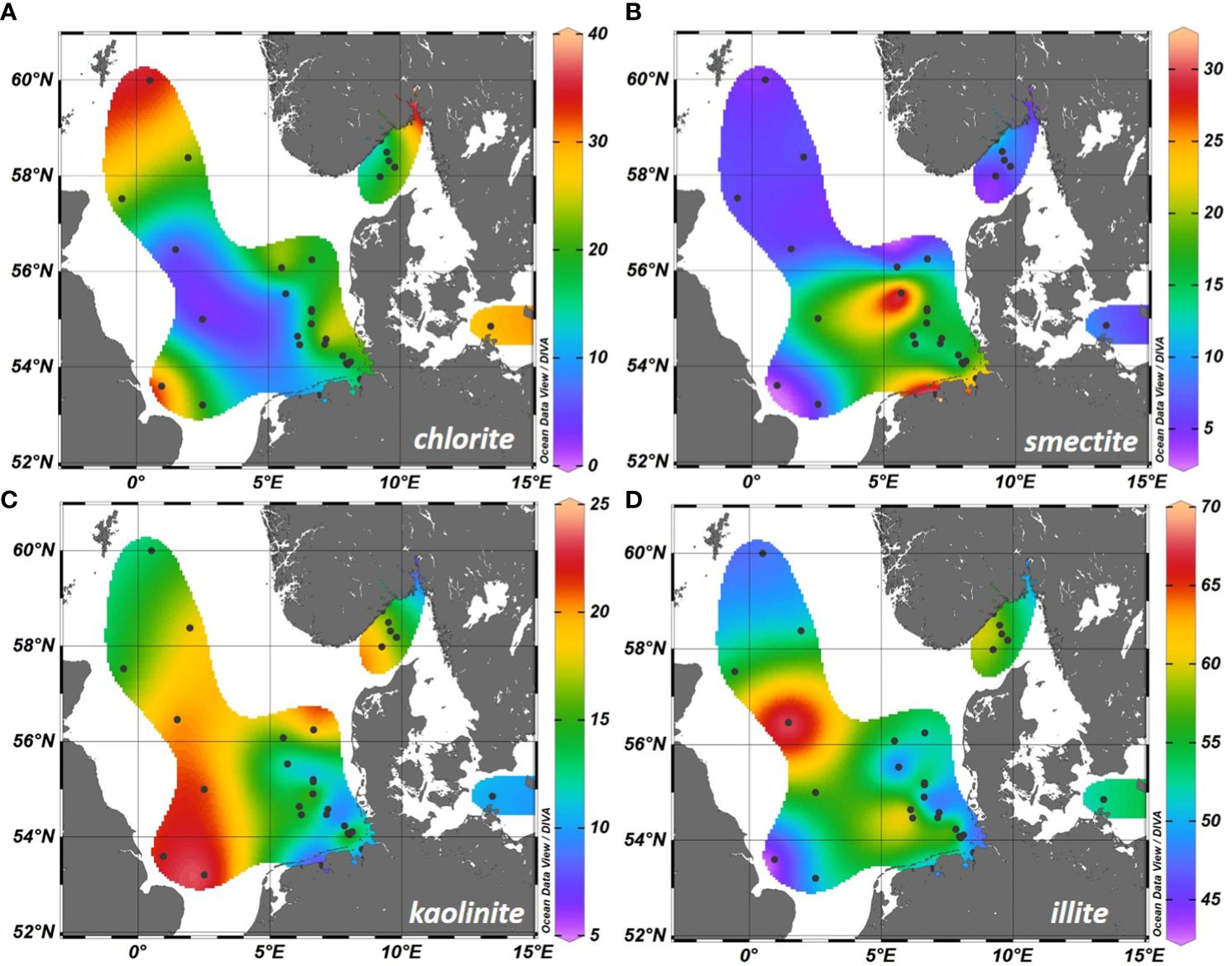
Figure 3 Clay mineral distribution of the clay sized surface sediments in the North Sea in percentage: (A) chlorite, (B) smectite, (C) kaolinite, (D) illite. Black dots indicate the sample locations. The maps were generated by using ODV software, version 5.6.3 (Schlitzer, Reiner, Ocean Data View, https://odv.awi.de, 2021).
4 Discussion
4.1 The main sediment contributors to the Skagerrak region
To disentangle the sediment contributions from different sources that accumulate in the depocenter of the Skagerrak, the potential source areas need to be defined and characterized. The isotopic signatures reveal a sharp boundary between the northern and southern North Sea reflecting the contribution from different source areas (Figure 2). The clays originating from the southern North Sea and in particular of the estuaries of the middle European rivers Elbe, Weser and Ems are characterized by more radiogenic εNd and less radiogenic 87Sr/86Sr isotope values than clays originating from the Baltic Sea and the Scandinavian coast (Figures 2A, B), which reflects the distinct source rocks of the hinterland. During partial melting the mother isotope of the radiogenic 143Nd behaves more compatible in the mantle than the primordial 144Nd resulting in lower 143Nd/144Nd ratios in continental crust compared to the mantle (DePaolo and Wasserburg, 1976). In contrast, the mother isotope of the radiogenic 87Sr is incorporated into the magmatic melt rather than staying in the mantle leading to higher 87Sr/86Sr ratios in old continental rocks compared to young mantle-derived rocks (Wilson, 1989). The sediments from Scandinavia typically originate from old Paleozoic-Proterozoic rocks (Figure 1) and consequently detrital sediments from Scandinavian fjords have unradiogenic Nd and radiogenic Sr isotope ratios. Surface sediments from the Baltic Sea show even less radiogenic εNd and more radiogenic Sr isotope signatures than the surface sediments of the Norwegian fjords. This is consistent with the estuarine systems of the Baltic Sea predominantly supplying older crustal Proterozoic-Archean sediments into the Baltic Sea (Andersson et al., 1992). Furthermore, most fine-grained sediments in the Baltic Sea are provided by coastal and seabed erosion, particularly of glacial till (Wallmann et al., 2022) originating from Scandinavia, particularly from Finland characterized by unradiogenic εNd and radiogenic Sr isotope values. Moreover, the measured isotope signatures of the Baltic Sea are consistent with εNd signatures of Scandinavian-river sediments (Kymiijoki, Ume, Lule) ranging between -17.6 and -20.4 previously determined by Bayon et al. (2015).
Clay-size sediments in the southern North Sea, including the English Channel, German Bight and large parts of the Danish North Sea sector, reveal more radiogenic εNd and less radiogenic Sr isotope signatures with peak values observed for the clay fractions of the central European-river estuaries (Figures 2A, B). These sediment signatures reflect the hinterland geology of central Europe which is dominated by Quaternary and Tertiary sediments (Figure 1; Pawlewicz et al., 2002). Highly radiogenic Nd and isotope signatures of -9.10 and -11.5 were also found for sediments of Rhine and Seine by Bayon et al. (2015). The Skagerrak clay sediments are a mixture of these two source regions given that the radiogenic isotope signatures are intermediate between Mid-European-like and Scandinavian-like isotope ratios. That implies that the surrounding Scandinavian hinterland is not the main contributor to Skagerrak sediments. This is supported by previous studies who reported that large amounts of particles (50 - 90%) are transported laterally into the Skagerrak, whereas only a small proportion of Skagerrak deposits originate from local sources including local riverine and coastal erosion inputs (van Weering et al., 1993; de Haas and van Weering, 1997). Strong differences between the isotope signatures of the Skagerrak and the Baltic Sea surface sediments (Figure 2) suggest that the Baltic Sea is an insignificant source for Skagerrak clays. This is in good agreement with further studies which have shown that the SPM supply entering the North Sea through the Skagerrak-Kattegat transition is comparatively low (Eisma and Irion, 1988; Pohlmann and Puls, 1994). Conversely, the clays in the northern part of the North Sea reveal isotopic ratios similar to the Skagerrak sediments (Figure 2). These clays partly reflect the Atlantic input following the circulation pattern that clearly shows that Atlantic water masses entering the North Sea from the north between the British and Norwegian coasts flow directly into the Skagerrak region (Figure 1). In fact, Bengtsson and Stevens, (1998) as well as Pederstad et al. (1993) already mentioned that the North Atlantic deep water transports muds into the Skagerrak, but only in small proportions compared to the Jutland Current coming from the southern North Sea. However, when following the Jutland Current from the southern North Sea to the Skagerrak, the isotopic compositions of the clays clearly show a decreasing εNd and increasing Sr isotope signature (Figures 2A, B), which makes the southern North Sea and the Mid-European rivers unlikely main contributors to the Skagerrak. This is also supported by the clay mineral distribution which reveals a profound decrease in smectite and increase in kaolinite content from the German Bight along the west coast of Denmark to the Skagerrak (Figures 3B, C).
Combining mineralogical and isotopic data, the Skagerrak and the northern North Sea surface sediments display similar characteristics in similar ranges of the data (Figure 4) which implies that the northern North Sea is the predominant supply area of fine-grained sediments in the Skagerrak.
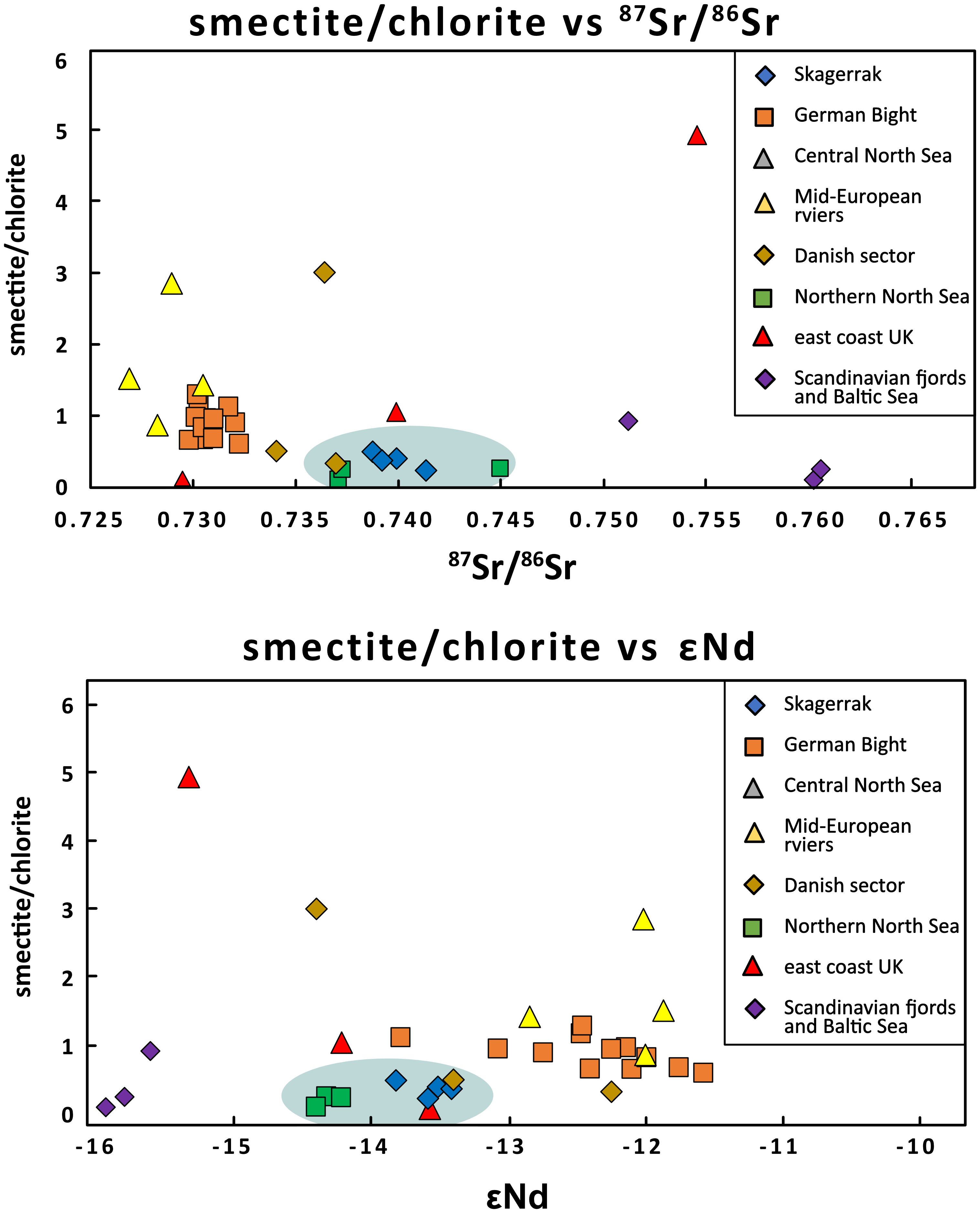
Figure 4 Clay minerals (smectite/chlorite) and radiogenic isotopes (87Sr/86Sr, εNd) in surface samples of the North Sea. The light blue colored area marks the northern North Sea and Skagerrak surface sediments with similar isotopic and clay mineral characteristics.
These observations based on Nd and Sr isotopic data as well as clay mineral data allow the definition of three main source end members including the northern North Sea, southern North Sea, and Scandinavia, whereby the northern North Sea appears to be the dominant source of the Skagerrak clays, which cannot be explained by a two-endmember mixture of the southern North Sea and Scandinavia only (Figure 5). This contrasts with the conclusions of previous provenance studies of Skagerrak sediments, which rather considered the southern North Sea to be the dominant contributor (Zöllmer and Irion, 1993; Bengtsson and Stevens, 1998; Irion and Zöllmer, 1999; Lepland et al., 2000).
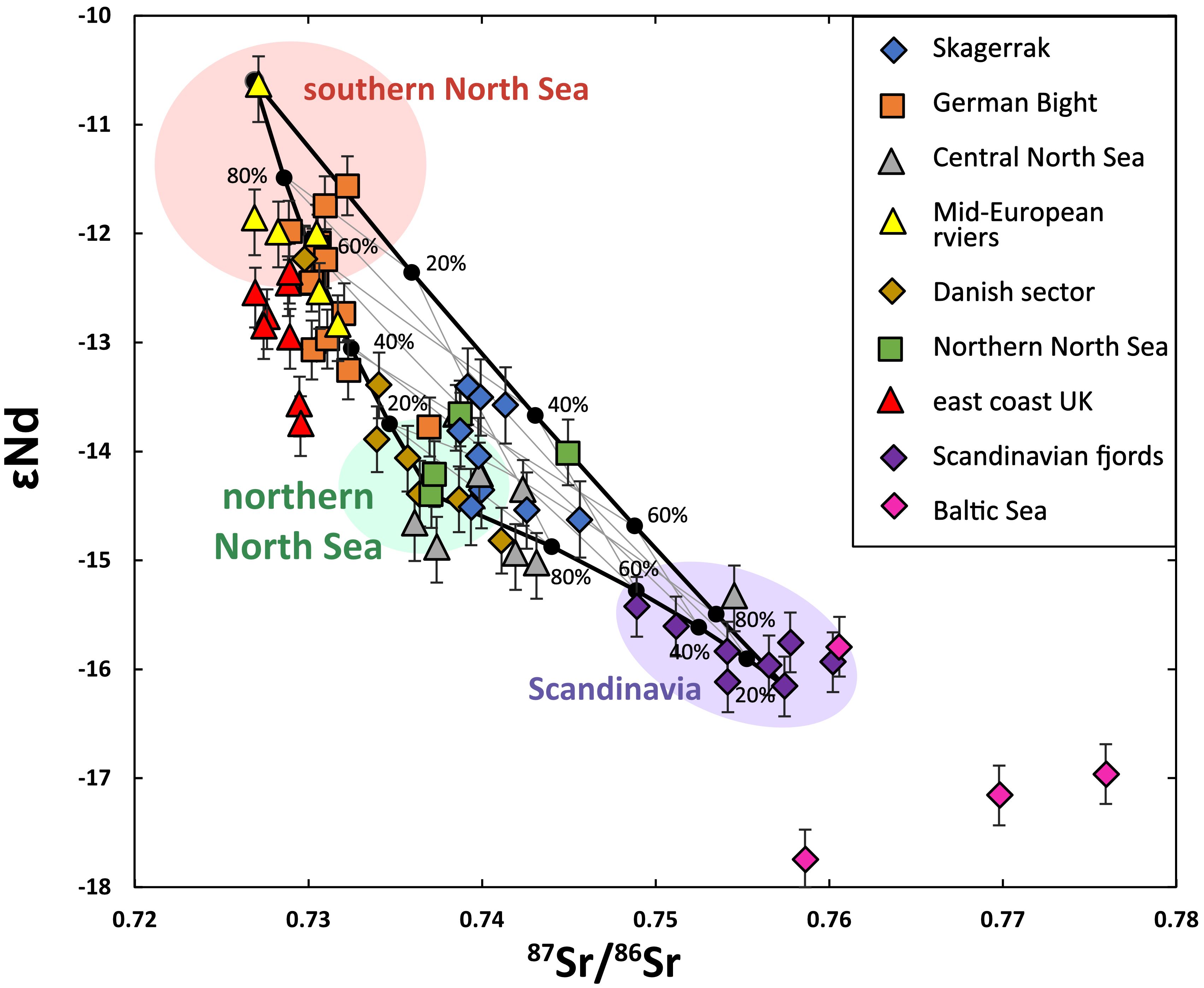
Figure 5 Nd-Sr isotope composition of the clay size fraction of the surface sediments in the North Sea with the defined end members of the southern North Sea, northern North Sea and Scandinavia and the resulting mixing field. The 2SD error bars for Sr isotopes are smaller than the symbol size. The grid was calculated according to a 10% increment of the mixture composition. The isotopic compositions are based on measured data of the respective source area. The concentration data for the calculated endmembers is based on literature data of the respective source area (Blanchet, 2019).
Figure 5 shows that the Skagerrak clays are plotting close within the field defining samples from the northern North Sea, suggesting high supplies from this region, although some Skagerrak samples seem to be rather a mixture between the southern North Sea and Scandinavian region. However, the spatial distributions of the radiogenic isotopes and the clay minerals (Figures 2, 3) clearly indicate that the northern North Sea and the Skagerrak sediments have similar geochemical characteristics, which differ from the southern North Sea and Scandinavian sediments. This shows that the northern North Sea is the predominant sediment contributor to the Skagerrak basin.
Our results demonstrated that the radiogenic isotope signatures not only provide information about geochemical characteristics of the parent bedrock of the respective source, but also about the transport pathways of clays and the circulation pattern which significantly influences the distribution of fine-grained-sediments in the North Sea. This can be seen in the northern North Sea where major transport pathways from the North Atlantic such as the Tampen Bank and Central North Sea Current transport particles directly to the Skagerrak resulting in similar isotopic characteristics in both regions. Most previous studies mentioned that the SPM dynamics of the North Sea are dominated by the input from the Atlantic, although the sediment supply across Dover Strait is higher than that of the northern pathway (Eisma and Irion, 1988; Sündermann, 1993; Puls et al., 1997). This results in higher SPM concentrations in the southern North Sea compared to the northern North Sea (Eisma, 1981). This evidence is now compared to the clay mineralogical and radiogenic hafnium isotope distribution in the study area.
4.2 Clay mineral distribution in the North Sea
In general, the clay mineral distribution shows a domination of the primary clay minerals illite and chlorite together representing 72% of the fine-grained North Sea sediments with the highest abundances in the northern North Sea, as well as the Skagerrak (Figure 2D). These abundances reflect limited influence of clay minerals newly formed during weathering processes and confirm dominant physical erosion under cold climatic conditions, which is consistent with the environmental conditions prevailing in the North Sea hinterland where reworking of immature glacial deposits currently occurs. During cold periods of the Pleistocene the ice sheets transported illite and chlorite-rich till from the Fennoscandian Shield into the North Sea basin, resulting in high illite and chlorite contents in the northern part of the basin (Irion and Zöllmer, 1999; Figures 3A, D). Griffin et al. (1968) and Chamley (1975) also found high illite and chlorite contents in marine sediments from the North Atlantic, which would be consistent with an Atlantic input to the northern North Sea and which complicates distinguishing these source areas. However, the low smectite content in the Fladen Ground (Figure 3), which represents a hotspot of mud deposition, differ from clay mineral contents determined by Johnson and Elkins (1979) in sediments north of the North Sea shelf, which makes the Atlantic an unlikely dominant source for northern North Sea sediments. Furthermore, the SPM concentrations in the North Atlantic at the northern part of the North Sea shelf are much lower than observed in the Fladen Ground area (Pohlmann and Puls, 1994; Gayer et al., 2006). This supports the conclusions of Eisma (1973) and Eisma and Kalf (1987) that Fladen Ground sediments are reworked early Holocene deposits and that the North Atlantic is only a minor contributor to North Sea sediments. From these results it can be concluded that the sediments entering the Skagerrak via the Atlantic Currents preferentially originate from seabed erosion of old relict deposits in the northern North Sea rather than from the Atlantic inflow north of the Shetland Islands. This is also consistent with the previously mentioned observation that Skagerrak sediments display similar mineralogical and isotopic characterizations as the northern North Sea sediments and supports the conclusion that the northern North Sea is a predominant source for clays deposited in the Skagerrak.
The kaolinite content in the North Sea shows strikingly high values at the (southern) east coast of England (Figure 3C). Kaolinite is primarily formed by continental chemical weathering processes and mostly originates from Weichselian tillites with Tertiary origin or old eroded rocks transported seawards by fluvial or coastal processes (Exley, 1976; Chamley, 1989). Furthermore, it is the dominant clay mineral in the Upper Triassic and Lower Jurassic rocks (Mørk et al., 2003) which dominate the south-eastern coast of England (Figure 1). Consequently, the kaolinite-rich sediments in the south-western North Sea imply an England origin by riverine input or coastal erosion. McCave (1987), as well as McManus and Prandle (1997), found that the supply from intensive cliff erosion at the Holderness and Norfolk cliffs, are the predominant source of SPM in this region, whereas fluvial supply is negligible. This demonstrates the importance of coastal erosion in the sediment budget of the sediments in the south-eastern North Sea. Furthermore, most clays from the east coast of UK plot outside of the Nd-Sr mixing triangle (Figure 5) suggesting a fourth end member in addition to the previously defined endmembers for the Skagerrak (southern North Sea, northern North Sea, Scandinavia). With the strong influences of cliff erosion in these regions in mind, it is likely that coastal erosion is a dominant sediment source for suspended matter at the east coast of England. It is conspicuous that certain parts of the Skagerrak show relatively high kaolinite contents (Figure 3C) which are also observed in the clay mineral data of Irion and Zöllmer (1999) from East Anglia to the Skagerrak region. This implies a significant input from the south-eastern coast of England to the Skagerrak sediments.
The southern North Sea is characterized by higher secondary clay mineral contents compared to the northern part of the North Sea (Figure 2D). This is the result of relatively high amounts of smectite in the south-eastern North Sea with the highest contents in the German river estuaries (Figure 3) which may be supplied by altered volcanic rocks from central Europe. Therefore, the profoundly lower smectite contents in the German Bight sediments indicate only a relatively small fluvial sediment supply from Mid-European rivers. Puls et al. (1997) established a SPM budget for the German Bight that clearly indicated that German rivers only supply negligible amounts of SPM to the southern North Sea. Further investigations of sediment dynamics in the southern North Sea have shown that the highest concentrations of suspended matter prevail in the English Channel where they are entrained by currents from the south into the southern North Sea and the German Bight (Eisma, 1981; Pohlmann and Puls, 1994). In fact, the southwestern North Sea, including the English Channel and the southwest coast of the UK, show isotope signatures similar to the German Bight. Hence, given that the German rivers show much higher smectite contents than the open southern North Sea also including the English Channel, it is likely that the radiogenic isotope distribution of the clay fraction in this area reflects the circulation pattern of the southern North Sea.
Irion and Zöllmer (1999) presented an extensive study of the clay mineral distribution of the entire North Sea that is similar to our study. They clay mineral calculation follows Biscaye (1965) and therefore resembles the calculation method used in this study. However, our smectite contents in the Skagerrak and parts of the German Bight reveal much lower values and resemble the smectite contents measured by Bengtsson and Stevens, (1998) in the Skagerrak area. Irion and Zöllmer (1999) mainly analyzed surface samples from the upper 20 cm which represent modern sedimentary conditions of the North Sea, but rather within the Holocene period. In contrast, surface sediments from our study and those from Bengtsson and Stevens, (1998) only include the upper 0–5 cm of the sediments which is more representative for the current century in the Skagerrak. Therefore, these differences in smectite contents may suggest a decline of smectite input to the southern North Sea and the Skagerrak. Since the Mid-European rivers constitute a predominant source of smectite, this likely indicates a decreasing riverine input to the North Sea over time. A possible explanation could be river damming, which has progressively increased for major European rivers such as the Elbe, Weser, and Ems (Hübner and Schwandt, 2018) and has led to a decrease in the SPM concentration in the rivers (Ericson et al., 2006; Rovira et al., 2014). Consequently, river damming may have diminished the riverine sediment supply to the ocean and resulted in a reduced contribution to the German Bight and Skagerrak sediments.
4.3 Radiogenic Hf isotopes tracing sedimentary processes
Despite that the radiogenic Hf isotope composition can be strongly influenced by fractionation processes during weathering, the εHf distribution in the North Sea reveals patterns generally similar to the Nd and Sr radiogenic isotope systems, but also shows distinct differences in specific regions (Figure 2). These imply a decoupling of Nd and Hf isotopic composition in the respective source areas due to incongruent weathering and sorting processes. Comparison of εHf and εNd data exhibits that the clays are isotopically similar to global river clays (Bayon et al., 2016; Figure 6A) suggesting that the clay fraction experienced a loss of Hf-bearing zircons and only a moderate degree of chemical weathering, which is consistent with expectations for relatively cold climate conditions. However, some samples, in particular most of the Weser and Ems surface samples, plot near the terrestrial array (Vervoort et al., 1999) with unexpectedly unradiogenic εHf signatures. Even though zircons are thought to only occur in negligible amounts in the clay fraction, Marchandise et al. (2014) concluded that an influence of zircons remains in clay-sized sediments given that they can also occur in very fine grains. Prior to grain-size separation for the clay analyses, the river sediments revealed high sand proportions. It is likely that the clay-sized sediments we measured partly experienced a high degree of physical weathering during long transport pathways through rivers causing the disintegration and abrasion of sand-sized sediments which tends to contain high amounts of Hf-bearing zircons. It is noteworthy that the εHf signatures in the German Bight are much more radiogenic than the river sediments of Weser and Ems (Figure 2C), which supports the observation that German rivers are minor contributors to the sediment budget of the North Sea and that major amounts of SPM are supplied to the southern North Sea via the English Channel.
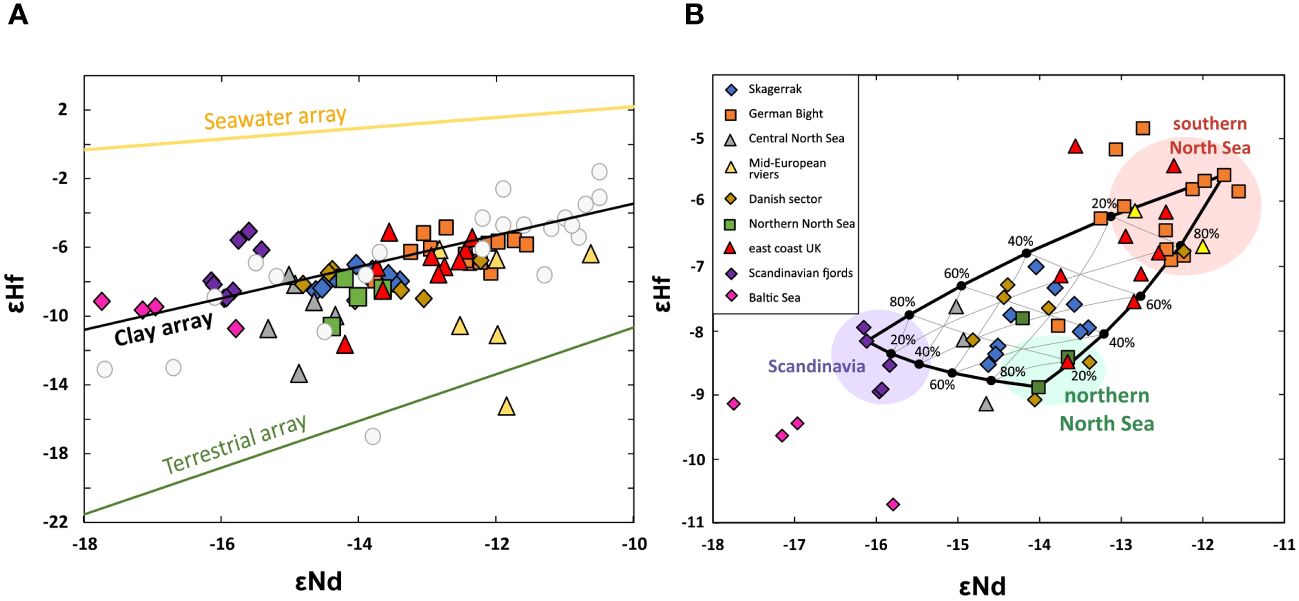
Figure 6 Combination of εHf and εNd signatures of surface samples of the North Sea: (A) clay surface samples with Seawater Array (Albarède et al., 1998), Clay Array (Bayon et al., 2015), and Terrestrial Array (Vervoort et al., 1999), (B) Hf-Nd isotope with the defined end members of the southern North Sea, northern North Sea and Scandinavia and the resulting mixing field. The grid was calculated according to a 10% increment of the mixture composition. The isotopic compositions are based on measured data of the respective source area. The concentration data for the calculated endmembers is based on literature data of the respective source area (Blanchet, 2019).
The ΔεHfclay signatures, which describe the deviation of the measured Hf isotopic compositions from the Clay Array, are linked to climatic parameters and correlate positively with the degree of weathering (Bayon et al., 2016). Since some samples show relatively high positive or negative ΔεHfclay signatures (Figure S2), probably due to strong fractionation processes, e.g. incongruent weathering, they were excluded in the end-member mixing field of εHf and εNd (Figure 6B). Compared to the Nd-Sr isotope mixing field where most clays from the east coast of UK show less radiogenic Sr values and therefore plot outside the mixing field (Figure 5), which were previously interpreted to be influenced by coastal erosion, plot near the southern North Sea endmember in the Hf-Nd isotope mixing relationship (Figure 6B). Sediments transported via large rivers are often physically and chemically weathered by intensive erosion and transport processes (Bouchez et al., 2012). Therefore, river sediments mostly have a higher degree of alteration than sediments originating from rock coast erosion, which supplies newly exposed rocks from cliffs (Costa et al., 2015; Prémaillon et al., 2018). The east coast of England, as Holderness and Norflok, is composed mainly of Quaternary sediments with glacial till (McCave, 1987; Pye and Blott, 2015), but also large parts at the south-eastern coast of England consist of upper Triassic and Lower Jurassic rocks where chalk cliffs are present (Mørk et al., 2003), the exposed sediments of which are likely less altered than river sediments. Consequently, the slight shift of some UK clays toward less radiogenic values can possibly be explained by a lower chemical weathering intensity compared to other samples originating from the southern North Sea when it is assumed that these sediments are mainly contributed by cliff erosion. This would also support the finding that UK sediments are mainly contributed by coastal erosion.
When calculating the contributions of the northern North Sea, the southern North Sea and Scandinavia to the sediments deposited in the Skagerrak based on the three radiogenic systems of Nd, Sr and Hf, it results in 43% originating from the north, 26% from the south and 31% from Scandinavia. These calculated contributions of the respective endmembers are in good agreement with the previous findings and confirms that the seabed erosion in the northern North Sea plays a major role in contributing sediments to the Skagerrak compared to the southern North Sea. This also demonstrates that the combination of all three isotope systems is a sensitive and powerful tool to trace and quantify sources of erosional material.
4.4 Reconstruction of the sedimentary systems in the North Sea and the role of coastal and seabed erosion
Previous studies on the sediment budget of the North Sea have shown that the main source of SPM in the North Sea is the North Atlantic which supplies material between Scotland and Norway and across the Strait of Dover (McCave, 1973; Eisma and Kalf, 1987; Puls et al., 1997). As strong currents prevail in the English Channel, the SPM does not settle in this region but is transported further into the southern North Sea. These strong currents lead to intensive erosion processes due to high bed shear stress velocities in the eastern Channel where sediment is also supplied by coastal and seabed erosion, which results in much higher suspended matter concentrations compared to the Atlantic entry (Eisma, 1981; Sündermann and Puls, 1990). This is also supported by much higher suspended matter concentrations in the Dover Strait than observed in the adjacent Atlantic (UKMMAS, 2010). In addition, coastal cliff erosion at the west coast of the North Sea, especially at the East Anglia Coast with the highest SPM concentrations in the North Sea (Stanev et al., 2009), could lead to high suspended matter concentration in this region. These observations are also supported by similar isotopic signatures in the English Channel, the southern east coast of England and the German Bight indicating that the coastal erosion at the English coast and within the English Channel supplies the southern North Sea with SPM. Furthermore, the rather radiogenic εNd values suggest a source rock of Mid-European origin which can be found at the coast surrounding the English Channel and the southern North Sea (Figures 1, 2). Since we already showed that Mid-European rivers only provide negligible amounts of suspended matter to the ocean, it is likely that large proportions of fine-grained sediments which are transported and partly deposited in the inner German Bight originate from erosion processes within the English Channel.
The northern North Sea, which we proposed to be the predominant source for Skagerrak sediments, is dominated by the Fladen Ground where fine-grained sediments can be found (Eleftheriou and Basford, 1989; Bailey et al., 1993). Eisma (1981) suggested that seafloor erosion in the northern North Sea represents a source for fine-grained sediment, which is primarily caused by the inflow of Atlantic Waters supplying the Skagerrak with sediment (Eisma and Kalf, 1987; van Weering and Kalf, 1987). This suggests, that Skagerrak sediments mainly originate from erosion processes occurring in the northern North Sea by the Atlantic Currents, as our study already indicated by the geochemical characterization of the surface samples. However, most studies about the provenance of the Skagerrak and sediment budget of the North Sea indicated a predominant influence from the southern North Sea in the Skagerrak sediments (Eisma and Irion, 1988; Zöllmer and Irion, 1993; 0Bengtsson and Stevens, 1998; Lepland et al., 2000). It is noted that most of these studies focused on the mud fraction, which comprises both clay and silt sized sediments. However, the sediment depocenter of the Skagerrak mainly consists of clay deposits up to 60% (Lepland and Stevens, 1996). Bockelmann et al. (2018) predicted the spatial distribution of the median grain size in the North Sea including the clay size and clearly showed that especially the Fladen Ground and the Skagerrak are clay dominated in comparison to other depocenters in the North Sea. This strengthens the assumption that the clays deposited in the Skagerrak predominantly originate from erosion processes in the Fladen Ground with some Atlantic input. Since our study only considers the clay fraction, this would explain the apparently discrepant findings between the older studies and ours. In addition, Oost et al. (2021) suggested that the Wadden Sea region acts as a major sink of sediments transported along the southern pathway of the North Sea, which would explain why large proportions of suspended matter in the southern North Sea do not reach the Skagerrak, which is in a good agreement with our radiogenic isotopic distributions.
The above-mentioned findings emphasize the importance of coastal and seabed erosion for the sediment budget of the entire North Sea region. Puls et al. (1997) and Gerritsen et al. (2000) already mentioned that coastal erosion provides large amounts of suspended matter, which is transported by currents and deposited in deeper parts of the North Sea. However, these studies suggested smaller contributions of coastal erosion than Atlantic supplies. This contrasts with recent studies about the Baltic Sea (Wallmann et al., 2022) that showed that the coastal and seabed erosion is the main sediment contributor in this region surrounded by abundant coastal cliffs. This may indicate that the estimated seabed erosion of 6 -12 Mt per year calculated by van Alphen (1990) as well as Eisma and Irion (1988) for the entire North Sea is an underestimated source for fine-grained sediments and thus also highlighted the crucial role of erosion processes in the sediment budget. Furthermore, in the context of climate change, a rising sea-level enhances erosional processes especially at coastal areas (Bird, 1996; Leatherman et al., 2000) and may lead to an increasing SPM budget and consequently to a higher sediment supply to the depocenters of the North Sea.
In order to gain a better understanding of whether and to what extent these climate forcing factors change the sedimentary processes in the North Sea, a future work is necessary to resolve the temporal variability of the contribution of different endmembers. Additionally, it is still unclear how anthropogenic impacts such as bottom trawling as well as the dumping from dredging activities influence the sediment supply to the depocenters in the North Sea. A temporal resolution of the endmembers may also help to identify and assess these anthropogenic sediment sources.
5 Conclusions
The application of the radiogenic isotopic systems of Nd, Sr and Hf to clay-sized sediments in combination with clay mineralogy provides new insights into the sedimentary processes in the North Sea and allows to distinguish between three main sources of clay-sized sediments, which are the northern North Sea, Scandinavian fjords and Mid-European rivers. The isotopic and mineralogical data clearly show that the Skagerrak clay sediments predominantly originate from the northern North Sea. Coastal and seabed erosion, especially in the Fladen Ground, are shown to contribute high amounts of clays to the Skagerrak. In contrast, Scandinavian fjords and central European rivers are only minor contributors of clays. Our study also highlights that it is important to differentiate between different grain sizes of clay and silt when investigating the source of Skagerrak sediments, given that this region represents the main depocenter for clays. In addition, we demonstrate that suspended matter transported along the southern North Sea mainly originates from coastal erosion in the English Channel and along the south-eastern coast of England. Conversely, the central European rivers only provide negligible amounts to the SPM budget in the southern North Sea. Furthermore, the material originating from coastal erosion in the south-western North Sea is shown to be a minor contributor to Skagerrak sediments, consistent with the hypothesis that large proportions of SPM are deposited in the inner German Bight or trapped in the Wadden Sea region before reaching the Skagerrak.
Data availability statement
The original contributions presented in the study are included in the article/Supplementary Material. Further inquiries can be directed to the corresponding author/s.
Author contributions
NL: Writing – original draft, Writing – review & editing, Data curation. TS: Writing – review & editing. EH: Writing – review & editing. KW: Writing – review & editing. AE: Writing – review & editing. MF: Writing – review & editing, Supervision.
Funding
The author(s) declare financial support was received for the research, authorship, and/or publication of this article. This work was carried out in the frame of the project APOC “Anthropogenic impacts on particulate organic carbon cycling in the North Sea” (03F0874B) and was funded by the German Federal Ministry of Education and Research (BMBF).
Acknowledgments
We thank Stefan Dreibrodt for the introduction to quantitative clay mineral preparation and Brendan Ledwig for conducting XRD measurements at the University of Kiel (CAU). Niko Lahajnar (University of Hamburg) is thanked for providing surface sediment samples collected during HEINCKE cruises from the Danish and Norwegian sectors of the North Sea as well as the German Bight. Andreas Neumann (Hereon) is thanked for surface sediment samples from German river estuaries and Bryce Van Dam for sediment samples collected during cruise AL557. We thank Lasse Sander (AWI) for providing surface sediment samples from several HEINCKE cruises in the German Bight. We also thank Jerome Kaiser (IOW) for providing surface sediment samples from the Baltic Sea and Małgorzata Szymczak-Żyła (IOPAN) for surface samples from Norwegian fjords. The British Ocean Sediment Cores Research Facility (BOSCORF) is thanked for supplying sediment samples from the British sector of the North Sea. Finally, we thank Edelgard Baumann and Cosima Schulze for support in the lab.
Conflict of interest
The authors declare that the research was conducted in the absence of any commercial or financial relationships that could be construed as a potential conflict of interest.
Publisher’s note
All claims expressed in this article are solely those of the authors and do not necessarily represent those of their affiliated organizations, or those of the publisher, the editors and the reviewers. Any product that may be evaluated in this article, or claim that may be made by its manufacturer, is not guaranteed or endorsed by the publisher.
Supplementary material
The Supplementary Material for this article can be found online at: https://www.frontiersin.org/articles/10.3389/fmars.2024.1416519/full#supplementary-material
References
Albarède F., Simonetti A., Vervoort J. D., Blichert-Toft J., Abouchami W. (1998). A Hf-Nd isotopic correlation in ferromanganese nodules. Geophys. Res. Lett. 25, 3895–3898. doi: 10.1029/1998GL900008
Andersson P. S., Wasserburg G. J., Ingri J. (1992). The sources and transport of Sr and Nd isotopes in the Baltic Sea. Earth Planet. Sci. Lett. 113, 459–472. doi: 10.1016/0012-821X(92)90124-E
Bailey N., Chapman C. J., Kinnear J., Bova D., Weetman A. (1993). Estimation of Nephrops stock biomass on the Fladen ground by TV survey. ICES CM/K: 34..
Bayon G., Barrat J. A., Etoubleau J., Benoit M., Bollinger C., Revillon S., et al. (2009). De-termination of rare Earth elements, Zr, Ba, Hf and Th in geological samples by ICP-MS after Tm addition and alkaline fusion. Geostand. Geoanal. Res. 33, 51–62. doi: 10.1111/j.1751–908X.2008.00880.x
Bayon G., Skonieczny C., Delvigne C., Toucanne S., Bermell S., Ponzevera E., et al. (2016). Environmental Hf–Nd isotopic decoupling in World river clays. Earth Planet. Sci. Lett. 438, 25–36. doi: 10.1016/j.epsl.2016.01.010
Bayon G., Toucanne S., Skonieczny C., André L., Bermell S., Cheron S., et al. (2015). Rare earth elements and neodymium isotopes in world river sediments revisited. Geochim. Cosmochim. Acta 170, 17–38. doi: 10.1016/j.gca.2015.08.001
Bengtsson H., Stevens R. L. (1996). Heavy mineral provinces in southern Skagerrak and northern Kattegat. Nor. Geol. Unders. Bull. 430, 47–55. doi: 10.1180/000985598545381
Bengtsson H., Stevens R. L. (1998). Source and grain-size influences upon the clay mineral distribution in the Skagerrak and northern Kattegat. Clay Miner. 33, 3–13. doi: 10.1180/000985598545381
Bernet M., Brandon M. T., Garver J. I., Molitor B. (2004). Fundamentals of detrital zircon fission-track analysis for provenance and exhumation studies with examples from the European Alps. Geol. Soc Spec. Pub. 378, 25–36. doi: 10.1130/SPE378
Bird E. C. F. (1996). “Coastal erosion and rising sea level,” in Sea level rise and coastal subsidence. Eds. Millimann J. D., Haq B. U. (Kluwer Acad., Dordrecht, the Netherlands), 87–103.
Biscaye P. E. (1965). Mineralogy and sedimentation of a recent deep-sea clay in the Atlantic Ocean, adjacent seas and oceans. Geol. Soc Am. Bull. 76, 803–832. doi: 10.1130/0016-7606(1965)76[803:MASORD]2.0.CO;2
Blanchet C. L. (2019). A database of marine and terrestrial radiogenic Nd and Sr isotopes for tracing earth-surface processes. Earth Syst. Sci. Data 11, 741–759. doi: 10.5194/essd-11-741-2019
Blichert-Toft J. (2008). The Hf isotopic composition of zircon reference material 91500. Chem. Geol. 253, 252–257. doi: 10.1016/j.chemgeo.2008.05.014
Blichert-Toft J., Albarède F. (1997). The Lu-Hf isotope geochemistry of chondrites and the evolution of the mantle-crust system. Earth Planet. Sci. Lett. 148, 243–258. doi: 10.1016/S0012-821X(97)00040-X
Blum J. D., Erel Y. (2003). Radiogenic isotopes in weathering and hydrology. Treat. Geochem. 5, 605. doi: 10.1016/B0-08-043751-6/05082-9
Bockelmann F. D., Puls W., Kleeberg U., Müller D., Emeis K. C. (2018). Mapping mud content and median grain-size of North Sea sediments–A geostatistical approach. Mar. Geol. 397, 60–67. doi: 10.1016/j.margeo.2017.11.003
Bouchez J., Gaillardet J., Lupker M., Louvat P., France-Lanord C., Maurice L., et al. (2012). Floodplains of large rivers: weathering reactors or simple silos? Chem. Geol. 332, 166–184. doi: 10.1016/j.chemgeo.2012.09.032
Bout-Roumazeilles V., Combourieu-Nebout N., Desprat S., Siani G., Turon J.-L., Essallami L. (2013). Tracking atmospheric and riverine terrigenous supplies variability during the last glacial and the Holocene in central Mediterranean, Clim. Past 9, 1065–1087. doi: 10.5194/cp-9-1065-2013
Bouvier A., Vervoort J. D., Patchett P. J. (2008). The Lu–Hf and Sm–Nd isotopic composition of CHUR: constraints from unequilibrated chondrites and implications for the bulk composition of terrestrial planets. Earth Planet. Sci. Lett. 273, 48–57. doi: 10.1016/j.epsl.2008.06.010
Bouysse P. (2014). “Geological map of the world,” in Commission for the Geological Map of the World, 2 sheets, scale 1:35,000,000, 3rd ed.(Paris, France: Commission for the Geological Map of the World). doi: 10.14682/2014CGM3R
Bretschneider L., Hathorne E. C., Bolton C. T., Gebregiorgis D., Giosan L., Gray E., et al. (2021b). Enhanced late Miocene chemical weathering and altered precipitation patterns in the watersheds of the Bay of Bengal recorded by detrital clay radiogenic isotopes. Paleoceanogr. Paleoclimatol 36, e2021PA004252. doi: 10.1029/2021PA004252
Bretschneider L., Hathorne E. C., Huang H., Lübbers J., Kochhann K. G. D., Holbourn A., et al. (2021a). Provenance and Weathering of Clays Delivered to the Bay of Bengal during the Middle Miocene: Linkages to Tectonics and Monsoonal climate. Paleoceanogr. Paleoclimatol 36, e2020PA003917. doi: 10.1029/2020PA003917
Chamley H. (1975). Remarques sur la se ídimentation argileuse quaternaire en mer de Norv` ege. Bull. Union Oce íanogr. Fr. 7, 15–20.
Cohen A. S., O’Nions R. K., Siegenthaler R., Griffin W. L. (1988). Chronology of the pressure-temperature history recorded by agranulite terrain. Contrib. Mineral. Petrol. 98, 303–311. doi: 10.1007/BF00375181
Colin C., Turpin L., Bertaux J., Desprairies A., Kissel C. (1999). Erosional history of the Himalayan and Burman ranges during the last two glacial–interglacial cycles. Earth Planet. Sci. Lett. 171, 647–660. doi: 10.1016/S0012-821X(99)00184-3
Costa S., Letortu P., Laignel B. (2015). “The hydro-sedimentary system of the Upper-Normandy coast: synthesis,” in Sediment fluxes in coastal areas, vol. 10 . Eds. Maanan M., Robin M. (Coastal Research Library, Springer Netherlands, Dor-drecht), 121–147.
Dasch E. J. (1969). Strontium isotopes in weathering profiles, deep-sea sediments, and sedimentary rocks. Geochim. Cosmochim. Acta 33, 1521–1552. doi: 10.1016/0016-7037(69)90153-7
de Haas H., van Weering T. C. E. (1997). Recent sediment accumulation, organic carbon burial and transport in the northeastern North Sea. Mar. Geol. 136, 173–187. doi: 10.1016/S0025-3227(96)00072-2
de Haas H., van Weering T. C. E., de Stigter H. (2002). Organic carbon in shelf seas: sinks or sources, processes and products. Cont. Shelf Res. 22, 691–717. doi: 10.1016/S0278-4343(01)00093-0
DePaolo D., Wasserburg G. (1976). Nd isotopic variations and petrogenetic models. Geophys. Res. Lett. 3, 249–252. doi: 10.1029/GL003i005p00249
Ehlert C., Frank M., Haley B. A., Böniger U., De Deckker P., Gingele F. X. (2011). Current transport versus continental inputs in the eastern Indian Ocean: Radiogenic isotope signatures of clay size sediments. Geochem. Geophys. Geosyst 12, Q06017. doi: 10.1029/2011GC003544
Eisenhauer A., Meyer H., Rachold V., Tuetken T., Wiegand B., Hansen B. T., et al. (1999). Grain size separation and sediment mixing in Arctic Ocean sediments: evidence from the strontium isotope systematic. Chem. Geol. 158, 173–188. doi: 10.1016/S0009-2541(99)00026-1
Eisma D. (1973). “Sediment distribution in the North Sea in relation to marine pollution,” in North sea science. Ed. Goldberg E. D. (MIT Press, Cambridge, Mass.), 131–150.
Eisma D. (1981). Supply and deposition of suspended matter in the North Sea. Spec. Pub. Int. Ass. Sedimentologists 5, 415–428. doi: 10.1002/9781444303759.ch29
Eisma D., Irion G. (1988). “Suspended matter and sediment transport,” in Pollution of the north sea. Eds. Salomons W., Bayne B. L., Duursma E. K., Förstner U. (Springer Berlin Heidelberg, Berlin, Heidelberg), 20–35. doi: 10.1007/978–3-642–73709-1_2
Eisma D., Kalf J. (1987). Distribution, organic content and particle size of suspended matter in the North Sea. Neth. J. Sea Res. 21, 265–285. doi: 10.1016/0077-7579(87)90002-0
Eleftheriou A., Basford D. J. (1989). The macrobenthic infauna of the offshore Northern North Sea. J. Mar. Biol. Assoc. UK 69, 123–143. doi: 10.1017/S0025315400049158
Ericson J., Vorosmarty C., Dingman S., Ward L., Meybeck M. (2006). Effective sea-level rise and deltas: Causes of change and human dimension implications. Glob. Planet. Change 50, 63–82. doi: 10.1016/j.gloplacha.2005.07.004
Exley C. S. (1976). Observations on the formation of kaolinite in the St. Austell Granite, Cornwall. Clay Miner. 11, 51–63. doi: 10.1180/claymin.1976.011.1.06
Fettweis M., Francken F., Van den Eynde D., Verwaest T., Janssens J., Van Lancker V. (2010). Storm influence on SPM concentrations in a coastal turbidity maximum area with high anthropogenic impact (southern North Sea), Cont. Shelf Res. 30, 1417–1427. doi: 10.1016/j.csr.2010.05.001
Furnes G. K. (1980). Wind effects in the north sea. J. Phys. Oceanogr. 10, 978–984. doi: 10.1175/1520-0485(1980)010<0978:WEITNS>2.0.CO;2
Gaál G., Gorbatschev R. (1987). An outline of the Precambrian evolution of the Baltic Shield. Precambrian Res. 35, 15–52. doi: 10.1016/0301-9268(87)90044-1
Galer S. J. G., O’Nions R. K. (1989). Chemical and isotopic studies of ultramafic inclusions from the San Carlos volcanic field, Arizona: A bearing on their petrogenesis. J. Geophys. Res. 30, 1033–1064. doi: 10.1093/petrology/30.4.1033
Garzanti E., Andò S., Vezzoli G. (2009). Grain-size dependence of sediment composition and environmental bias in provenance studies. Earth Planet. Sci. Lett. 277, 422–432. doi: 10.1016/j.epsl.2008.11.007
Gayer G., Dick S., Pleskachevsky A., Rosenthal W. (2006). Numerical modeling of suspended matter transport in the North Sea. Ocean Dyn. 56, 62–77. doi: 10.1007/s10236-006-0070-5
Gerritsen H., Vos R. J., van der Kaaij T., Lane A., Boon J. G. (2000). Suspended sediment modelling in a shelf sea (North Sea). Coast. Eng. 41, 317–352. doi: 10.1016/S0378-3839(00)00042-9
Gingele F. X., De Deckker P., Hillenbrand C.-D. (2001). Late Quaternary fluctuations of the Leeuwin Current and palaeoclimates on the adjacent land masses: clay mineral evidence. Austrian J. Earth Sci. 48, 867–874. doi: 10.1046/j.1440-0952.2001.00905.x
Goldstein S. L., Jacobsen S. B. (1987). The Nd and Sr isotopic systematics of river-water dissolved material: Implications for the sources of Nd and Sr in seawater. Chem. Geol. 66, 245–272. doi: 10.1016/0168-9622(87)90045-5
Griffin J. J., Windom H., Goldberg E. D. (1968). The distribution of clay minerals in the world ocean. Deep-sea Res. 15, 433–459. doi: 10.1016/0011-7471(68)90051-X
Grousset F. E., Biscaye P. E., Zindler A., Prospero J., Chester R. (1988). Neodymium isotopes as tracers in marine sediments and aerosols: North Atlantic. Earth Planet. Sci. Lett. 87, 367–378. doi: 10.1016/0012-821X(88)90001-5
Gutjahr M., Frank M., Stirling C. H., Klemm V., van de Flierdt T., Halliday A. N. (2007). Reliable extraction of a deepwater trace metal isotope signal from Fe–Mn oxyhydroxide coatings of marine sediments. Chem. Geol. 242, 351–370. doi: 10.1016/j.chemgeo.2007.03.021
Hallam A. (1971). Mesozoic geology and the opening of the North Atlantic. J. Geol 79, 129–157. doi: 10.1086/627605
Horwitz E. P., Chiarizia R., Dietz M. L. (1992). A novel strontium-selective extraction chromatographic resin. Solvent Extr. Ion Exch. 10, 313–336. doi: 10.1080/07366299208918107
Hübner G., Schwandt D. (2018). Extreme low flow and water quality–A long-term view on the River Elbe. Erdkunde 72, 235–252. doi: 10.3112/erdkunde.2018.03.05
Huhma H., Mutanen T., Whitehouse M. (2004). Oldest rocks of the Fennoscandian Shield: the 3.5 Ga Siurua trondhjemite gneiss in the Archaean Pudasjärvi granulite belt, Finland. GFF 126, 10.
Irion G., Zöllmer V. (1999). Clay mineral associations in fine-grained surface sediments of the North Sea. J. Sea Res. 41, 119–128. doi: 10.1016/S1385-1101(98)00041-0
Jacobsen S. B., Wasserburg G. J. (1980). Sm-nd isotopic evolution of chondrites. Earth Planet. Sci. Lett. 50, 139–155. doi: 10.1016/0012-821X(80)90125-9
Jochum K. P., Nohl U., Herwig K., Lammel E., Stoll B., Hofmann A. W. (2005). GeoReM: a new geochemical database for reference materials and isotopic standards. Geostand. Geoanal. Res. 29, 333–338. doi: 10.1111/j.1751-908X.2005.tb00904.x
Johnson T. C., Elkins S. R. (1979). Holocene deposits of the Northern North Sea: evidence for dynamic control of their mineral and chemical composition. Geol. Mijnbouw 58, 353–366.
Kärki A., Laajoki K., Luukas J. (1993). Major Palaeoproterozoic shear zones of the central Fennoscandian Shield. Precambrian Res. 64, 207–223. doi: 10.1016/0301-9268(93)90077-F
Kessarkar P. M., Rao V. P., Ahmad S. M., Babu G. A. (2003). Clay minerals and Sr–Nd isotopes of the sediments along the western margin of India and their implication for sediment provenance. Mar. Geology 202, 55–69. doi: 10.1016/S0025-3227(03)00240-8
Kuijpers A., Dennegard B., Albinsson Y., Jensen A. (1993). Sediment transport pathways in the Skagerrak and Kattegat as indicated by sediment chernobyl radioactivity and heavy-metal concentrations. Mar. Geol. 111, 231e244. doi: 10.1016/0025-3227(93)90133-G
Lang L. O., Stevens R. L. (1999). Source, transport, and weathering influences upon grain-size and heavy mineral trends in glacial deposits of southwestern Sweden. GFF 121, 145–153. doi: 10.1080/11035899901212145
Laves D., Jähn G. (1972). Zur quantitativen röntgenographischen bodenton-mineralanalyse. Arch. Acker-Pflanzenb. Bodenk. 16, 735–739.
Leatherman S. P., Zhang K., Douglas B. C. (2000). Sea level rise shown to drive coastal erosion. EOS Trans. Am. Geophys. Union 81, 55–59. doi: 10.1029/00EO00034
Lepland A., Sæther O., Thorsnes T. (2000). Accumulation of barium in recent Skagerrak sediments: sources and distribution controls. Mar. Geol. 163, 13–26. doi: 10.1016/S0025-3227(99)00104-8
Lepland A., Stevens R. L. (1996). Mineral magnetic and textural interpretations of sedimentation in the Skagerrak, eastern North Sea. Mar. Geol. 135, 51–64. doi: 10.1016/S0025-3227(96)00060-6
Liu Z., Zhao Y., Colin C, Stattegger K., Wiesner M. G., Huh C.-A., et al. (2016). Source-to-sink transport processes of fluvial sediments in the South China Sea. Earth Sci. Rev. 153, 238–273. doi: 10.1016/j.earscirev.2015.08.005
Longva O., Thorsnes T. (1997). Skagerrak in the past and atthe present – an integrated study of geology, chemistry, hydro-graphy and microfossil ecology. Nor. Geol. Unders. Spec. Pub. 8, 100.
Luepke G., Clifton H. E. (1983). Heavy-mineral distribution in modern and ancient bay deposits, Willapa Bay, Washington, U.S.A.: Sediment. Geol. 35, 233–247. doi: 10.1016/0037-0738(83)90060-X
Marchandise S., Robin E., Ayrault S., Roy-Barman M. (2014). U–Th–REE–Hf bearing phases in Mediterranean Sea sediments: Implications for isotope systematics in the ocean. Geochim. Cosmochim. Acta 131, 47–61. doi: 10.1016/j.gca.2014.01.017
McCave I. N. (1973). “Mud in the north sea,” in North sea science. Ed. Goldberg E. D. (MIT Press, London), 75–100.
McCave I. N. (1987). Fine sediment source and sinks around the East Anglian Coast (UK). J. Geol. Soc 144, 149–152. doi: 10.1144/gsjgs.144.1.0149
McManus J. P., Prandle D. (1997). Development of a model to reproduce observed suspended sediment distributions in the southern North Sea using Principal Component analysis and Multiple Linear Regression. Cont. Shelf Res. 17, 761–778. doi: 10.1016/S0278-4343(96)00057-X
Mørk M. B. E., Vigran J. O., Smelror M., Fjerdingstad V., Bøe R. (2003). Mesozoic mudstone compositions and the role of kaolinite weathering-a view from shallow cores in the Norwegian Sea (Møre to Troms). Norwegian J. Geol. 83, 61–78.
O’Neill F., Ivanović A. (2016). The physical impact of towed demersal fishing gears onsoft sediments. ICES J. Mar. Sci. 73, i5–i14. doi: 10.1093/icesjms/fsv125
Oost A., Colina Alonso A., Esselink P., Wang Z. B., Kessel T., van Maren B. (2021). Where mud matters: towards a mud balance for the trilateral Wadden Sea Area: mud supply, transport and deposition (Leeuwarden: Wadden Academy).
OSPAR (2017). Dumping and placement of dredged material: OSPAR thematic assessment (OSPAR Commission). Available online at: https://oap.ospar.org.
Otto L., Zimmerman J. T. F., Furnes G. K., Mork M., Saetre R., Becker G. (1990). Review of the physical oceanography of the North Sea. Neth. J. Sea Res. 26, 161–238. doi: 10.1016/0077-7579(90)90091-T
Patchett P. J., White W. M., Feldmann H., Kielinczuk S., Hofmann A. W. (1984). Hafnium/rare Earth element fractionation in the sedimentary system and crustal recycling into the Earth’s mantle. Earth Planet. Sci. Lett. 69, 365–378. doi: 10.1016/0012-821X(84)90195-X
Pawlewicz M. J., Steinshouer D. W., Gautier D. L. (2002). Map showing geology, oil and gas fields, and geologic provinces of Europe including Turkey. U.S. Geological Survey. Open-File Report 97–470-I, 14. doi: 10.3133/ofr97470I
Pederstad K., Roaldset E., Rønningsland T. M. (1993). Sedimentation and environmental conditions in the inner Skagerrak—outer Oslofjord. Mar. Geo 111, 245–268. doi: 10.1016/0025-3227(93)90134-H
Pin C., Zalduegui J. F. S. (1997). Sequential separation of light rare-earth elements, thorium and uranium by miniaturized extraction chromatography: Application to isotopic analyses of silicate rocksAnal. Chim. Acta 339, 79–89. doi: 10.1016/S0003-2670(96)00499-0
Pohlmann T., Puls W. (1994). “Currents and transport in water,” in Circulation and contaminant fluxes in the north sea. Ed. Sündermann J. (Springer, Berlin/Heidelberg/New York), 345–402. doi: 10.1007/978–3-642–78294-7_11
Prémaillon M., Regard V., Dewez T. J. B., Auda Y. (2018). GlobR2C2 (Global Recession Rates of Coastal Cliffs): a global relational database to investigate coastal rocky cliff erosion rate variations. Earth Surf. Dyn. 6, 651–668. doi: 10.5194/esurf-6-651-2018
Puls W., Heinrich H., Mayer B. (1997). Suspended particulate matter budget for the German Bight. Mar. pollut. Bull. 34, 398–409. doi: 10.1016/S0025-326X(96)00161-0
Pye K., Blott S. J. (2015). Spatial and temporal variations in soft-cliff erosion along the Holderness coast, East Riding of Yorkshire, UK. J. Coast. Conserv. 19, 785–808. doi: 10.1007/s11852-015-0378-8
Revel M., Cremer M., Grousset F. E., Labeyrie L. (1996). Grain-size and Sr-Nd isotopes as tracers of paleo-bottom current strength, Northeast Atlantic Ocean. Mar. Geol. 131, 233–249. doi: 10.1016/0025-3227(96)00005-9
Rodhe J. (1998). “The Baltic and Northern Seas: a process-orientated review of the physical oceanography. 750 J. Sharples et al./Continental Shelf Research 26 (2006) 733–751,” in The sea, vol. 11 . Eds. Robinson A. R., Brink K. H. (Wiley, New York), 699–732.
Rovira A., Ballinger R., Ibanez C., Parker P., Dominguez M. D., Simon X., et al. (2014). Sediment imbalances and flooding risk in European deltas and estuaries. J. Soils Sediments 14, 1493–1512. doi: 10.1007/s11368-014-0914-4
Sahoo Y. V., Nakai S. I., Ali A. (2006). Modified ion exchange separation for tungsten isotopic measurements from kimberlite samples using multi-collector inductively coupled plasma mass spectrometry. Analyst 131, 434–439. doi: 10.1039/B511557D
Spiegel T., Diesing M., Dale A. W., Lenz N., Schmidt M., Sommer S., et al. (2024). Modeling mass accumulation rates and 210 Pb rain rates in the Skagerrak: Lateral sediment transport dominates the sediment input. Front. Mar. Sci. 11. doi: 10.3389/fmars.2024.1331102
Stabell B., Thiede J. (1985). Upper Quaternary marine Skagerrak (NE North Sea) deposits: stratigraphy and depositional environment. Nor. J. Geol. 65, 1–149.
Stanev E. V., Dobrynin M., Pleskachevsky A., Grayek S., Günther H. (2009). Bed shear stress in the southern North Sea as an important driver for suspended sediment dynamics. Ocean Dyn. 59, 183–194. doi: 10.1007/s10236-008-0171-4
Steiger R. H., Jäger E. (1977). Subcommission on Geochronology: Convention on the use of decay constants in geo- and cosmochronology. Earth Planet. Sci. Lett. 36, 359–362. doi: 10.1016/0012-821X(77)90060-7
Stevens R. L., Bengtsson H., Lepland A. (1996). Textural provinces and transport interpretations with fine-grain sediments in the Skagerrak. J. Sea Res. 96, 99–110. doi: 10.1016/S1385-1101(96)90739-X
Sündermann J. (1993). Suspended particulate matter in the North Sea: field observations and model simulations. Philos. Trans. R. Soc. London. Ser. A: Phys. Eng. Sci. 343, 423–430. doi: 10.1098/rsta.1993.0056
Sündermann J., Puls W. (1990). Modelling of suspended sediment dispersion and related transport of lead in the north sea. Mitt. Geol.-Paläont. Institut Univ. Hamburg 69, 143–155.
Tanaka T., Togashi S., Kamioka H., Amakawa H., Kagami H., Hamamoto T., et al. (2000). JNdi-1: a neodymium isotopic reference in consistency with LaJolla neodymium. Chem. Geol. 168, 279–281. doi: 10.1016/S0009-2541(00)00198-4
Turrell W. R. (1992). New hypotheses concerning the circulation of the northern North Sea and its relation to North Sea fish stock recruitment. ICES J. Mar. Sci. 49, 107–123. doi: 10.1093/icesjms/49.1.107
UKMMAS (2010). Suspended Particulate Matter and Turbidity. Charting Progress 2 Feeder Report: Ocean Processes. Eds. Huthnance J. Department for Environment Dood and Rural Affairs on behalf of the UK Marine Science Co-ordination Committee.
van Weering T. C. E., Berger G. W., Kalf J. (1987). Recent sediment accumulation in the Skagerrak, Northeastern North Sea. Neth. J. Sea Res. 21, 177–189. doi: 10.1016/0077-7579(87)90011-1
van Alphen J. S. L. J. (1990). A mud balance for Belgian–Dutch coastal waters between 1969 and 1986. Neth. J. Sea Res. 25, 19–30. doi: 10.1016/0077-7579(90)90005-2
Vance D., Thirlwall M. (2002). An assessment of mass discrimination in MC-ICPMS using Nd isotopes. Chem. Geol. 185, 227–240. doi: 10.1016/S0009-2541(01)00402-8
van Weering T. C. E. (1981). Recent sediments and sediment transport in the northern North Sea; surface sediments of the Skagerrak. Spec. Publ. Int. Assoc. Sedimentol. 5, 335–359. doi: 10.1002/9781444303759.ch25
van Weering T. C. E., Berger G. W., Okkels E. (1993). Sediment transport, resuspension and accumulation rates in the northeastern Skagerrak. Mar. Geol. 111, 269–285. doi: 10.1016/0025-3227(93)90135-I
Vervoort J. D., Patchett P. J., Blichert-Toft J., Albarède F. (1999). Relationships between Lu–Hf and Sm–Nd isotopic systems in the global sedimentary system. Earth Planet. Sci. Lett. 168, 79–99. doi: 10.1016/S0012-821X(99)00047-3
von Haugwitz W. R., Wong H. K. (1993). Multiple Pleistocene ice advances into the Skagerrak: A detailed seismic stratigraphy from high resolution seismic profiles. Mar. Geol. 111, 189–207. doi: 10.1016/0025-3227(93)90130-N
Wallmann K., Diesing M., Scholz F., Rehder G., Dale A. W., Fuhr M., et al. (2022). Erosion of carbonate-bearing sedimentary rocks may close the alkalinity budget of the Baltic Sea and support atmospheric CO2 uptake in coastal seas. Front. Mar. Sci. 9. doi: 10.3389/fmars.2022.968069
Walter H. J., Hegner E., Diekmann B., Kuhn G., van der Loeff M. M. R. (2000). Provenance and transport of terrigenous sediment in the South Atlantic Ocean and their relations to glacial and interglacial cycles: Nd and Sr isotopic evidence. Geochim. Cosmochim. Acta 64, 3813–3827. doi: 10.1016/S0016-7037(00)00476-2
Weis D., Kieffer B., Maerschalk C., Barling J., Jong J. D., Williams G. A., et al. (2006). Highprecision isotopic characterization of USGS reference materials by TIMS and MCICP-MS. Geochem. Geophys. Geosyst 7, Q08006. doi: 10.1029/2006GC001283
Whitton J. S., Churchman G. J. (1987). Standard methods for mineral analysis of soil survey samples for characterisation and classification in NZ soil bureau. NZ Soil Bureau Sci. Scientific Report 79.
Wilson M. (1989). Igneous petrogenesis: A global tectonic approach (London: Unwin Hyman), 466. doi: 10.1007/978–1-4020–6788-4
Yu Z., Colin C., Wan S., Saraswat R., Song L., Xu Z., et al. (2019). Sea levelcontrolled sediment transport to the eastern Arabian Sea over the past 600 kyr: Clay minerals and Sr Nd isotopic evidence from IODP site U1457. Quat. Sci. Rev. 205, 22–34. doi: 10.1016/j.quascirev.2018.12.006
Keywords: radiogenic isotopes, clay minerals, provenance, North Sea, Skagerrak
Citation: Lenz N, Spiegel T, Hathorne E, Wallmann K, Eisenhauer A and Frank M (2024) Provenance of clay-sized detrital sediments in the North Sea and the Skagerrak region based on radiogenic Nd-Sr-Hf isotopes and clay mineral compositions: assessing the impact of coastal and seabed erosion. Front. Mar. Sci. 11:1416519. doi: 10.3389/fmars.2024.1416519
Received: 12 April 2024; Accepted: 17 June 2024;
Published: 01 July 2024.
Edited by:
Juan Jose Munoz-Perez, University of Cádiz, SpainCopyright © 2024 Lenz, Spiegel, Hathorne, Wallmann, Eisenhauer and Frank. This is an open-access article distributed under the terms of the Creative Commons Attribution License (CC BY). The use, distribution or reproduction in other forums is permitted, provided the original author(s) and the copyright owner(s) are credited and that the original publication in this journal is cited, in accordance with accepted academic practice. No use, distribution or reproduction is permitted which does not comply with these terms.
*Correspondence: Nina Lenz, bmxlbnpAZ2VvbWFyLmRl