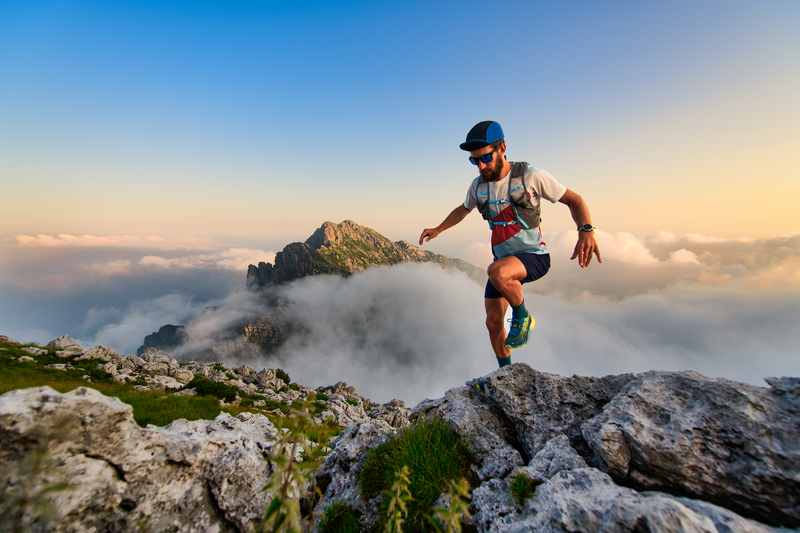
95% of researchers rate our articles as excellent or good
Learn more about the work of our research integrity team to safeguard the quality of each article we publish.
Find out more
ORIGINAL RESEARCH article
Front. Mar. Sci. , 11 June 2024
Sec. Coastal Ocean Processes
Volume 11 - 2024 | https://doi.org/10.3389/fmars.2024.1413145
This article is part of the Research Topic Processes, Mechanisms and Solutions in Coastal Wetland to Adapt to Changing Environment View all 10 articles
Introduction: The macrobenthos are crucial for the stability of estuarine ecosystems due to their burrowing behavior in the sediment and their uptake of nutrients from plants. These activities lead to significant alterations in both the morphological and biogeochemical processes within the region.
Methods: However, there is limited investigation into carbon cycling processes specifically related to crab bioturbation. Additionally, few studies have examined enzymatic activities and carbon fractions in sediments from crab burrow walls at different crab growth stages. This study aims to explore the impact of both plant invasiveness and crab bioturbation on carbon storage.
Results: Our findings suggest that plant invasion leads to higher organic accumulation due to the reduction of recalcitrant organic carbon (ROC) (decreased by 11.6% in invasive and 62.6% in native site from April to December), faster photosynthetic rates (25.8 μmol/m2 s in invasive and 10.7 μmol/m2 s in native site), and an increased presence of arbuscular mycorrhizal fungi (AMF) in the soil over time. However, the increase of easy oxidized carbon (EOC) may lead to less carbon storage in soil (increase by 67.7% in invasive and 48.8% in native site from April to December). In addition to invasiveness, the bioturbation activities of macrobenthos also affect carbon storage. Sediments from crab burrows exhibit higher EOC content (33.6% more than the bulk soil) and higher levels of carbon cycling-related enzymes, including S-ACT, S-β-GC, and S-ACP activities (24.2%, 8.99%, and 135.6% higher than the bulk soil, respectively).
Discussion: These changes contribute to reduced carbon accumulation in the soil. Therefore, crab bioturbation is a more significant factor affecting carbon sink capacity than plant invasion.
● We analyzed the biogeochemical properties of sediments in bulk soil, rhizosphere, and crab burrow walls.
● Sediments collected from mature crab burrow walls showed increased enzymatic activities related to CO2.
● Crab burrows and plant rhizosphere soil had more carbon transfer into easy oxidized carbon and labile forms than other soil.
● Plant invasion enhances carbon sequestration through increased photosynthesis and expansion of arbuscular mycorrhizal fungi.
Global climate change poses a significant threat to human survival and development (Tang et al., 2018). The burning of fossil fuels and industrial processes have resulted in substantial carbon emissions, estimated at 7.8 Pg C yr-1 (IPCC, 2013), disrupting the equilibrium between the global ecosystem and the atmosphere. Coastal salt marshes play a crucial role in carbon burial, with an estimated rate of 10.2 Tg C yr-1 (Ouyang and Lee, 2014). This burial is equivalent to approximately 1 to 2.5% of CO2 emissions from anthropogenic activities (Hinson et al., 2017; Grow et al., 2022). However, salt marshes are facing significant losses due to human activities such as filling, draining, and the “coastal squeeze” caused by sea level rise.
Crabs, such as fiddler crabs and Sesarmidae crabs, have significant impacts on sediment carbon cycling in coastal saltmarshes (Andreetta et al., 2014; Alberti et al., 2015) and other farmlands (Khoshnevisan et al., 2021). While recent studies have focused on the diversity, biomass, sediment topography, and nutrient cycling of benthic organisms in mangroves and salt marshes, little is known about the mechanisms and processes of carbon cycling within crab burrows in habitats dominated by native or invasive plants. They accelerate the rate of carbon cycling through biological and geochemical processes (Huhta, 2007; Agusto et al., 2022; Grow et al., 2022). Fiddler crab burrows increase oxygen penetration and create a more oxidized environment, leading to a 59% increase in effective surface area (Grow et al., 2022). The disturbance caused by fiddler crabs in saltmarshes results in a 12.7% increase in sediment CO2 efflux and a 51.7% increase in CH4 efflux (Agusto et al., 2022). The highest efflux rates occur immediately after burrowing activity ceases and gradually decrease over the following two hours (Grow et al., 2022). Additionally, fiddler crab-related greenhouse gas emissions decrease in the presence of oil contamination (Grow et al., 2022). This suggests that crab burrow walls harbor microbial communities with distinct functional characteristics compared to bulk soil, which may explain differences in sediment organic matter (OM) (Gillis et al., 2019). In mangrove forests, the respiration and burrowing activities of marine benthos double the CO2 emission rate from the bare sediment surface (Ouyang et al., 2021). However, the mechanism by which burrowing activity affects sediment CO2 emissions is still poorly understood.
Crabs also influence sediment physical properties through their burrowing activities. Sesarmidae crabs, in particular, create deep, multi-chambered burrows with large openings and substantial below-ground surface areas (Gillis et al., 2019). For example, Sesarmidae crabs are considered critical, which create deep (up to 2 m), multi-chambered burrows with large openings (maximum of 11 cm), and have sizeable below-ground surface areas up to 3.8 m2 (Stieglitz et al., 2000). These burrows serve as important shelters during summertime, as crabs prefer temperatures below 29°C (Thongtham et al., 2008). The burrowing activities of crabs accelerate litter decomposition and the mixing of sediment from the surface to deeper horizons (Wang et al., 2010). In Thailand, crabs remove an average of 87% of daily mangrove litter fall through ingestion or burial, with the removal rate being positively correlated with the number of crab burrows and negatively correlated with inundation time (Thongtham et al., 2008). In Japan, ocypodid crabs accelerate the removal of organic matter three times faster than weathering and mineralization processes (Koo et al., 2019). Therefore, crab bioturbation plays a crucial role in bio-geomorphological processes.
Crab bioturbation has multiple effects on the biogeochemical processes in sediments. Studies have shown significant differences in microbial diversity between wild and aquacultural macrobenthos, such as shrimps and crabs, using molecular-dependent methods (Chu et al., 2011; Liu et al., 2011; Li et al., 2012). The bacterial community plays a crucial role in various biochemical processes, including enzyme activity (Zhu et al., 2019). Sediment enzymes catalyze biochemical processes related to energy and material cycling (Bueno De Mesquita et al., 2017; Zhu et al., 2019). Extracellular enzymes target different organic carbon pools in sediments, such as cellulases and ligninase targeting polyphenolic macromolecules and simplified polysaccharides, respectively (Yang et al., 2020; Feng et al., 2023). Extracellular enzyme activity (EEA) can serve as an indicator for organic matter decomposition (Burns et al., 2013). Sediments disturbed by crabs have lower total and labile carbon contents, indicating a decrease in carbon concentration (Gutiérrez et al., 2006). However, there is limited research on the impact of crab bioturbation on EEA and organic carbon fractions, such as recalcitrant carbon and easily oxidized carbon, in sediments from active burrow walls.
Crabs have noticeable effects on plant density, height, and nutrient uptake in natural coastal salt marshes and farmlands (Bertness and Coverdale, 2013; Khoshnevisan et al., 2021). Crabs can be classified as herbivorous, omnivorous, or carnivorous based on their dietary preferences. Factors such as moisture content, resilience, and vegetation decomposition also influence crab feeding preferences (Harada and Lee, 2016). Herbivorous crabs tend to prefer leaves over fine roots and stems when consuming different plant tissues (Luo et al., 2019). This dietary variation leads to differences in CO2 respiration rates (Ouyang et al., 2021). Crab grazing can have both positive and negative effects on plants. For example, excessive grazing by Sesarma reticulatum crabs in New England (USA) has caused significant die-off of S. alterniflora in salt marshes (Altieri et al., 2012; Coverdale et al., 2012). However, the invasion of the green crab species Carcinus maenas has promoted the recovery of cordgrass by consuming and displacing Sesarma crabs from their burrows, thus reducing herbivory pressure (Bertness and Coverdale, 2013). Similar top-down control relationship between birds, crabs and terrestrial/aquatic plants are also observed and synthesized (Ge et al., 2023). Crabs are more likely to graze on seedlings because halophytic plants have higher nitrogen and lower fiber content (Bortolus and Iribarne, 1999). However, halophytes develop defenses, such as burrs or chemical compounds, to protect themselves, which can hinder crab ingestion (Bortolus and Iribarne, 1999; Chen et al., 2021). Crabs show a preference for invasive plant species (S. alterniflora) over native species (P. australis) due to differences in crude protein content (Ji et al., 2011). Macrobenthic animals play a crucial role in accelerating the decomposition of underwater debris, regulating material exchange at interfaces, and promoting the self-purification of water bodies. They also serve as key components in the food chain, and these processes can significantly influence carbon cycling. However, these factors are often overlooked, and the impact of crab grazing on plant invasion is rarely considered.
To address this gap, we conducted a series of experiments to investigate factors influencing carbon sequestration and emission. These factors included: (1) the presence of native and invasive plant species (i.e., SA vs. PA in the Yangtze estuary); (2) sediment zones (i.e., mud flats, juvenile crab burrows, mature crab burrows, rhizosphere, and non-rhizosphere); (3) different fractions of organic carbon (i.e., total organic carbon (TOC), EOC, and ROC); (4) carbon-related extracellular enzymes activities; and (5) seasonal variations within a year.
Upon all these related factors, we hypothesize that: (1) plant invasiveness would impact carbon cycling; (2) sediments within crab burrows would have higher levels of organic carbon and enzymatic activity; and (3) there would be significant changes in the carbon pool within crab burrows throughout the year. To test these hypotheses, we conducted field and laboratory experiments over 12 months. We aim to put forward the novel notion: crab burrowing activity reduces the carbon sequestration capacity more dramatically as they grow larger in native plant dominated saltmarshes.
This study was conducted in two study sites on Chongming Island, Shanghai, China. Chongming Island has a subtropical monsoon climate with ample precipitation, sunshine, and four distinct seasons. The field experiment took place from March 2021 to February 2022. Chongming Island is located at the Yangtze River, and salinity increases from northwest to southeast (see Figure 1). One of the sampling sites is a salt marsh in the Xisha Pearl Lake scenic area (see Figure 1). The dominant plant species in this area is Phragmites australis, a native freshwater species, and the major macrobenthic species is Chiromantes haematocheir. The second site is a brackish salt marsh on the north fringe of Chongming, which has been invaded by S. alterniflora (see Figure 1). The major benthic species in this area is Chiromantes dehaani, a grayish-greencrab species. Both crab species are omnivores, feeding on sediments, detritus, and plant tissues.
Figure 1 Distribution of sampling sites in the Yangtze Estuary. (A) map of People’s Republic of China, (B) map of Shanghai, (C) map of sampling sites in Chongming Island, Shanghai, China.
To account for spatial heterogeneity and internal variability, we selected five distinct sampling locations, including mud flats, juvenile crab burrows, mature crab burrows, plant rhizosphere, and non-rhizosphere (bulk sediments). We observed the crab physical appearance with their corresponding burrows, and categorized juvenile and mature crab burrows with opening diameter of less than 2 cm and 2 to 5 cm, respectively. Each type of sampling location was replicated three times at each site. For the sediments from crab burrows, we specifically chose active burrows where crabs were observed to ensure their presence.
The concentration of CO2 was measured using a portable analyzer (UGGA, model 915–0011, Los Gatos Research Inc., Mountain View, USA). We collected 40 crabs randomly of each species for census analysis and respiration measurements in the field. The crabs were kept in a bucket with salt marsh plants to prevent injury or death from fighting. Previous research (Ouyang et al., 2021) showed that crab respiration declined rapidly after being caught, so measurements were taken on the same day using a closed-loop system to minimize bias.
We also measured the in-situ CO2 fluxes from sediment, roots, and plants every month for one year. Root respiration was measured by removing above-ground vegetation and connecting the sediment and roots to a portable greenhouse gas analyzer using a closed-loop system. Plant respiration rate was measured by subtracting the CO2 flux under dark and light environments. The system included a PVC collar with dimensions of 20 cm in diameter and 20 to 50 cm in height to create an air-concealed headspace.
From April to November, we collected root samples from S. alterniflora and P. australis sites to analyze the infection rate of arbuscular fungi (AMF). We used a combination of methods from McGonigle et al. (1990), Sheng et al. (2011), and Soti et al. (2021) to optimize the results. Fine roots were rinsed with water and only roots with a diameter of less than 2mm were selected and stored in a 70% alcohol solution. The roots were cut into 1.5 cm fragments and softened in 15% KOH at 60°C for 110 minutes for P. australis and 4 hours for S. alterniflora. The softening period differ mainly caused by the structural difference and permeability of plant root cell wall. After rinsing off the KOH, the roots were acidified in 5% CH3COOH for 5 minutes. To stain the roots, a 5% CH3COOH-ink solution (5% Quink ink and 95% CH3COOH) was used at 60°C for 30 minutes, followed by clearing the ink in DI water for 14 hours at room temperature. The roots were then stained again with Sudan III solution (3g of Sudan III in 1000 ml of 70% alcohol) at 60°C for 60 minutes and clarified using 70% alcohol for 5 minutes. The degree of mycorrhizal colonization in roots is estimated by:
Soil extracellular enzyme activity can be useful for monitoring microbial activity linked to substrate dynamics because enzymes catalyze the rate and initiation steps in soil organic matter decomposition (Sinsabaugh et al., 2008; Burns et al., 2013). We analyzed enzymatic activities in sediments collected from S. alterniflora and P. australis sites. The sampling was done in April, August, and November, corresponding to the pre-growing, growing, and post-growing seasons respectively. Sediment samples were collected from mudflats, juvenile crab burrow walls, mature crab burrow walls, rhizosphere, and non-rhizosphere in both S. alterniflora and P. australis sites. Sampling depth are different to ensure the sample’s representative for its corresponding category.
The activities of β-glucosidase, acid phosphatase, and catalase enzymes in sediments were determined using Solarbio (S-β-GC) Activity Assay Kit (Solarbio BC0160), S-ACP Activity Assay Kit (Solarbio BC0140), and (S-CAT) Activity Assay Kit (Solarbio BC0100) respectively. The detailed methods are expressed in the manual. The enzyme plays a crucial role in the carbon cycling process.
The sediment samples were dried at 60°C, ground, and sieved. For TC and TN analysis, 40 mg of dry sediments were weighed and placed in aluminum foil, then analyzed using a Vario EL III element analyzer (Elementar, Germany). For TOC analysis, 1N HCl was added to remove carbonates from the sediments. For ROC analysis, HCl was used to remove inorganic carbon, followed by a hydrolysis reaction with 20 mL of H2SO4 (5 mol/L) at 105°C for 30 minutes. The samples were rinsed with DI water, dried, and then hydrolyzed again with 2 mL of 26 mol/L H2SO4 at room temperature for 3 hours (Rovira and Vallejo, 2002). The sediment samples were rinsed, dried, and analyzed using the Vario EL III element analyzer. EOC analysis was performed using a modified method of Hassan et al. (2016) by oxidizing the sample with KMnO4 solution (333 mmol/L).
We measured the photosynthesis of S. alterniflora and P. australis during the pre-growing season, growing season, and post-growing season in May, August, and November respectively. This was done using a portable photosynthesis system (LI-6800) with a Multiphase Flash™ Fluorometer (LI-COR, Nebraska). The parameters measured included net photosynthetic rate, transpiration rate, intercellular CO2 concentration, and stomatal conductance. All measurements were conducted under sunny weather conditions, with a pause in measurements from 11 am to 2 pm to avoid photoinhibition of P. australis.
The data for gas flux were organized in Excel and calculated using MATLAB R2020b. Statistical analyses were performed using ANOVA and t-test at a significance level of 0.05 (P=0.05) in IBM statistics SPSS 23. The goal of ANOVA is to determine the amount of variability in groups of data, and test if this variation is greater within groups to between groups. Linear regression was conducted in Excel to predict the value of a variable during the sampling period. PCA analysis and graphing were conducted using GraphPad Prism 9.5.3. aiming to extract the important information from a set of summary indices.
We tested the total organic carbon content (TOC) for sediments collected from mudflat, juvenile crab burrow walls, mature crab burrow walls, rhizosphere, and non-rhizosphere at both S. alterniflora and P. australis sites. The highest TOC content value was found in the rhizosphere sediment at the S. alterniflora site, but not significant. In the P. australis site, TOC was significantly higher in mature crab burrows (0.157 ± 0.040%) compared to all other sediment types (0.048 ± 0.027%, 0.058 ± 0.019%, 0.099 ± 0.033%, and 0.059 ± 0.024% in mudflat, juvenile crab burrows, rhizosphere, and non-rhizosphere soil, respectively) (Figure 2A). Rhizosphere sediment in P. australis also exhibited significantly higher TOC content compared to other sediment types (P<0.05). The presence of plant litter in the rhizosphere contributes to a substantial portion of organic carbon in the sediment carbon pool. The changes in TOC content correspond well with total carbon (TC) and total nitrogen (TN) content in the five sampling locations at both sites.
Figure 2 Comparion of sediment total organic carbon (TOC) (A), easy oxidized carbon (EOC) (B) and recalcitrant orgnic carbon (ROC) (C) at five sampling sites, including sediments from mudflats, juvenile crab burrows, mature crab burrows, rhizosphere and non rhizosphere from S. alterniflora and P. australis dominated salt marshes. Stars connecting two boxes represent valuses of significant difference. Changes of EOC and ROC contents in sediments collected from mudflats (D), juvenile crab burrows (E), mature crab burrows (F), rhizosphere (G) and non-rhizosphere (H) of S. alterniflora and P. australis from April to December.
The content of EOC in sediments did not show significant differences among sampling locations in the S. alterniflora site. However, the EOC in mature crab burrow walls (0.211 ± 0.087 mg/g) and rhizosphere (0.142 ± 0.079 mg/g) was slightly higher than that in other sediment types (0.061 ± 0.043 mg/g, 0.085 ± 0.055 mg/g, and 0.129 ± 0.054 mg/g in mudflat, juvenile crab burrows, and non-rhizosphere soil, respectively) (Figure 2B). In the P. australis site, EOC content was significantly higher in mature crab burrows (0.211 ± 0.087 mg/g) compared to mudflat (0.061 ± 0.042 mg/g), juvenile crab burrows (0.085 ± 0.055 mg/g), and non-rhizosphere soil (0.129 ± 0.055 mg/g) (P<0.005) (Figure 2B). This indicates a significant amount of carbon in mature crab burrows that are readily available for oxidation due to crab burrowing and metabolic activities. Meanwhile, the background EOC content was lower in the P. australis site (0.126 ± 0.083 mg/g) compared to the S. alterniflora site (0.213 ± 0.099 mg/g) (P<0.005) (Table 1).
Table 1 Two-way ANOVA analysis results of the carbon and nitrogen storage comparison at S. alterniflora and P. australias sites.
The sediment ROC content is higher in mature crab burrows at the P. australis site (0.651 ± 0.169%) and the S. alterniflora site (0.725 ± 0.111%) compared to the other four sediment sources (average of 0.686 ± 0.112% and 0.309 ± 0.133% at S. alterniflora and P. australis site, respectively). However, there is a decrease in ROC content and an increase in EOC in mature crab burrows over the sampling period (Table 2), indicating that sediments organic carbon in crab burrows are becoming a carbon source to the atmosphere.
Table 2 Linear regression results of the changes of EOC and ROC at S. alterniflora and P. australis sites in the five types of sediments during sampling period.
Overall, the TC, TN, TOC, EOC, and ROC contents are significantly higher at the S. alterniflora site compared to the P. australis site (Table 1). As shown in Figure 2, the sediment from mature crab burrow walls is the only type with higher TOC content in P. australis than in S. alterniflora. All other sediment types have higher carbon and nitrogen contents in S. alterniflora compared to P. australis throughout the sampling period (Supplementary Figure S1A, B).
We conducted monthly sampling and analysis of sediments from five sources at S. alterniflora and P. australis sites to examine the annual variation in EOC and ROC content. Our results showed that EOC content increased at sampling locations disturbed by plants and macrobenthos, such as sediments collected from crab burrows and rhizosphere. In contrast, ROC content decreased at all sampling locations throughout the year, particularly at crab-disturbed locations (Figure 2 and Table 2) (P<0.05 for all mature crab burrows, P<0.05 for juvenile crab burrows at P. australis site). These fluctuations of EOC and ROC among the sampling periods suggest that soil organic matter tends to switch to labile and atmospheric carbon in the presence of crab bioturbation activities.
Figure 2 and Table 2 illustrate the seasonal changes in EOC and ROC at the invasive S. alterniflora and native P. australis sites. The increase in EOC content was more pronounced in sediments collected from crab burrows and rhizosphere regions in S. alterniflora compared to P. australis. The regression slopes between month and EOC were steeper at S. alterniflora (0.0165, 0.0161, and 0.034 in juvenile crab burrows, natural crab burrows, and rhizosphere, respectively) than that at P. australis site (0.005, 0.002, and 0.018 in juvenile crab burrows, natural crab burrows, and rhizosphere, respectively), indicating a more significant transformation of soil organic carbon into a labile form at the invasive site. In contrast, ROC content did not show the same trend at the invasive site, while it decreased in all sampling locations at the native site (with significance). This decrease in ROC content suggests a lower capability for organic matter accumulation in P. australis.
Overall, the comparison of ROC and EOC at invasive and native sites give rise to an assertion that invasive habitats can increase OM accumulation through less transformation of ROC into labile carbon; and decrease OM accumulation through higher transformation rate of carbon into EOC. Thus, plant invasiveness does not significantly affect total organic carbon in soil.
Our findings show that the highest photosynthetic rate for P. australis occurs during the growing season (Figure 3). This photosynthetic rate is negatively correlated with intercellular CO2 concentration (Supplementary Figure S2A) and positively correlated with evapotranspiration and stomatal conductance (Supplementary Figure S2B). S. alterniflora exhibits a higher photosynthetic rate from spring to summer compared to other seasons, while P. australis has the highest photosynthetic rate during summer. Additionally, the photosynthetic rate of S. alterniflora is significantly higher than that of P. australis. Consequently, the longer growing period and higher photosynthetic rate of S. alterniflora result in greater carbon accumulation in S. alterniflora fields compared to P. australis fields.
Figure 3 Comparison of photosynthesis (A, B), roots respiration of mature stands (C) and net exchange of CO2 of sprouts at marsh front (D) in S. alterniflora and P. australis during pre-growing season, growing season and post-growing season. Parameters include photosynthetic rate (A), evapertranspiration (B). Root respiration of mature S. alterniflora and P. australis (C) and the net CO2 exchange of whole sprouts at edge of S. alterniflora and P. australis salt marshes from January to December.
Figure 3C demonstrates that the roots of P. australis release more CO2 into the atmosphere than S. alterniflora. The highest CO2 efflux occurs in June for S. alterniflora and October for P. australis. During the warm summer period, the favorable environmental conditions promote rhizosphere microbial activity and root respiration.
Both S. alterniflora and P. australis are perennial plants that produce sprouts from below-ground rhizomes during the growing season. We measured the net CO2 exchange of these sprouts using similar method for plant respiration under light condition. Sprouts typically have a height ranging from 30 to 70 cm. As depicted in Figure 3D, S. alterniflora sprouts exhibit negative net CO2 exchange from March to October, whereas P. australis sprouts only show negative net CO2 exchange for 1 to 2 months during the growing season. This indicates that the carbon accumulation capacity of S. alterniflora sprouts is higher than that of P. australis.
C. haematocheir crabs have higher respiration rates (0.960 ± 0.506 μmol/s individual) compared to C. dehaani crabs (0.289 ± 0.188 μmol/s individual) (Figure 4A). There is also a significant difference in respiration rates between male and female C. haematocheir crabs (1.12 ± 0.627 μmol/s individual for females and 0.780 ± 0.254 μmol/s individual for males, P<0.005) (Figure 4A). Generally, crabs in P. australis marshes have higher respiration rates.
Figure 4 Crab related parameters, including (A) the respiration rate of female and male crabs from C. dehaani and C. haematocheir species; (B) the correlation between crab respiration rate and their carapace width; (C) the juvenile and mature crab burrow density in both S. alterniflora and P. australis salt marshes; (D) the opening area of burrows occupied by juvenile and mature crabs in S. alterniflora and P. australis salt marshes. Different lower-cased letters represent values of significant differences. All boxes and dots colored in green represent results of C. dehaani living in S. alterniflora site, colored in yellow represent results of C. haematocheir living in P. australis.
We observed a positive correlation between the carapace width of crabs and their respiration rate (Figure 4B). Despite being smaller in size (4.675 ± 2.442 cm), C. haematocheir crabs have higher respiration rates (1.789 ± 0.858 μmol/s individual) compared to C. dehaani crabs (8.55 ± 0.721 cm) (1.188 ± 0.574 μmol/s individual, P<0.005) (Figure 4B).
There is no significant difference in mature crab burrow densities between S. alterniflora and P. australis sites (9.9 ± 3.1 per m2 on average, P>0.005) (Figure 4C). However, the density of juvenile crab burrows is significantly higher in the invaded S. alterniflora salt marsh compared to the P. australis marsh (106.4 ± 8.8 per m2 and 80.6 ± 35.2 per m2 in S. alterniflora and P. australis, respectively, P<0.005) (Figure 4C). Despite the smaller carapace size of C. haematocheir crabs in the P. australis site (Figure 4B), their burrow openings are significantly wider (2.96 ± 0.32 cm and 3.50 ± 0.37 cm for C. haematocheir and C. dehaani, respectively) (Figure 4D). This finding challenges the prevailing belief that bigger crabs create bigger burrows. However, it may be linked to the need to disperse higher levels of CO2 generated by C. haematocheir crabs within the burrow. Additionally, the larger burrows indicate increased energy expenditure during burrowing, leading to higher metabolic and respiration rates. This corresponds with the observed higher respiration rate in C. haematocheir crabs (Figure 4A).
Figure 5A displays the infection rates of roots collected from high and low tidal zones. There is no significant difference in infection rates between tidal zones. However, P. australis exhibits a significantly higher infection rate (67.8 ± 17.0%) compared to S. alterniflora (37.9 ± 22.5%). We also collected root samples from sprouts and mature stands of S. alterniflora and P. australis. There is no difference in infection rates within species (Figure 5B), but P. australis shows a higher infection rate. Additionally, we collected mature root samples from high tidal zones during the pre-growing season (April), growing season (August), and post-growing season (November). For S. alterniflora, there is no significant change in AMF infection throughout the year. However, the infection rate is higher in P. australis roots during the three growing seasons, with a slight increase during the growing period (Figure 5C).
Figure 5 Comparison of Arbuscular-Mycorrhizal-Fungi infection rate in S. alterniflora and P. australis roots. (A) infection rate at samples collected from high and mid tidal heights. (B) infection rate at sprout and mature stage. (C) infection rate of mature plant roots collected at pre-growing, growing and post growing seasons. (D) morphological differences of AMF colonized in the two species. Different lower-cased letters represent values of significant differences.
The morphology of mycorrhiza in P. australis and S. alterniflora roots is distinct (Figure 5D). Most mycorrhiza found in P. australis are endo-mycorrhiza or vesicular-arbuscular. In contrast, the mycorrhizae in S. alterniflora are generally ectomycorrhiza, which spread hyphae further into the sediment matrix and significantly increase the surface area of roots and the volume of sediments that the roots can access. This facilitates the invasion of S. alterniflora into salt marshes initially dominated by P. australis by enhancing nutrient accessibility.
According to Figure 6A, S-CAT shows significantly higher activity in rhizosphere sediments compared to other locations at both S. alterniflora and P. australis sites during the pre-growing season. The activity levels were 60.8 ± 0.99 U/g dry soil and 47.6 ± 1.05 U/g dry soil at S. alterniflora and P. australis sites, respectively, while the average activity levels at other locations were 38.6 ± 2.76 U/g dry soil and 37.7 ± 4.4 U/g dry soil at S. alterniflora and P. australis sites, respectively. In the P. australis site, S-CAT is less active in catalyzing organic matter decomposition in the soil. In April, the S-CAT activities in crab burrow walls are as low as those in bulk sediments, which corresponds to the lower metabolism level of crabs at lower temperatures. As plants grow and crabs mature, there is an overall decrease in S-CAT activities during the growing season (Figures 6A, B). However, S-CAT activity becomes significantly higher in mature crab burrows (44.72 ± 2.82 U/g dry soil and 53.19 ± 2.36 U/g dry soil for C. dehaani and C. haematocheir burrow walls, respectively) and rhizosphere (42.76 ± 2.11 U/g dry soil and 51.96 ± 4.22 U/g dry soil for S. alterniflora and P. australis sites, respectively) compared to bulk sediments and juvenile crab burrow walls (27.99 ± 2.064 U/g dry soil and 41.86 ± 2.30 U/g dry soil for S. alterniflora and P. australis sites, respectively) (Figure 6C). The enzymatic activity is higher in P. australis, which enhances the carbon cycling process and increases CO2 efflux in this native salt marsh. In November, the rhizosphere sediments showed the highest enzymatic activity, followed by sediments collected from mature crab burrow walls in both S. alterniflora and P. australis sites.
Figure 6 Comparison of sediments enzymatic activities at five sampling sites, including sediments from mudflats, juvenile crab burrows, mature crab burrows, rhizosphere and non-rhizosphere from S. alterniflora and P. australis dominated salt marshes. (A, D, G) are results from April (pre-growing season). (B, E, H) are results from August (growing season). (C, F, I) are results from November (post growing season). Different lower-cased letters represent values of significant differences.
There was no significant difference in S-β-GC activity among the five sampling locations and different sampling periods at the S. alterniflora site (Figures 6D–F). However, at the P. australis site, we observed higher enzymatic activity in mature crab burrow walls (22.00 ± 4.96 U/g dry soil in April, 16.89 ± 1.36 U/g dry soil in August, and 22.31 ± 1.71 U/g dry soil in November) compared to other sampling locations (18.70 ± 3.45 U/g dry soil in April, 16.09 ± 1.26 U/g dry soil in August, and 17.25 ± 1.98 U/g dry soil in November). Although there was no significant difference in S-β-GC activity in August, the activity was slightly higher in mature crab burrows and rhizosphere sediments (Figure 6E). In November, the S-β-GC activity was significantly higher in mature crab burrows (22.31 ± 1.71 U/g dry soil) compared to juvenile crab burrows (16.08 ± 1.83 U/g dry soil), which corresponds to the larger size of crab burrows and higher respiration rate in mature crab burrows compared to juvenile crab burrows (Figures 4A, C).
The S-ACP activity in both S. alterniflora and P. australis salt marshes showed similar patterns during the three sampling periods (Figures 6G–I), indicating higher enzymatic activity in sediments collected from mature crab burrow walls (5115.56 ± 3984.73 U/g dry soil in C. dehaani and 4822.51 ± 2995.27 U/g dry soil in C. haematocheir) and plant rhizosphere (4953.52 ± 3720.62 U/g dry soil in S. alterniflora and 30669.14 ± 821.97 U/g dry soil in P. australis) compared to other sampling locations (2354.98 ± 462.70 U/g dry soil in S. alterniflora and 2253.32 ± 883.00 U/g dry soil in P. australis). In the P. australis site, the highest enzymatic activity in mature crab burrow walls was observed during the post-growing season (11782.5 ± 6934.52 U/g dry soil in S. alterniflora and 9026.84 ± 428.40 U/g dry soil in P. australis) compared to the growing season (3327.65 ± 818.36 U/g dry soil in S. alterniflora and 2472.7 ± 95.79 U/g dry soil in P. australis). During the growing season, both mature crab burrow walls and rhizosphere sediments showed significantly higher S-ACP activity compared to other sampling locations in both the invasive S. alterniflora and native P. australis sites. Additionally, the S-ACP activity in November was more than three times higher in November than that in April and August.
Table 3 indicates that sediment from mudflats, juvenile crab burrow walls, and non-rhizosphere exhibit lower enzymatic activity compared to other types. The activity of S-β-GC does not show significant variation across sampling locations (P>0.05). However, mature crab burrow walls display significantly higher enzymatic activity of S-CAT and S-ACP compared to the aforementioned sediment types. The only enzyme that exhibits significantly higher activity in the rhizosphere is S-CAT.
Table 3 Comparison of sediment enzymatic activities (U/g dry soil) between different types of sediments collected from mudflats, juvenile crab burrow walls, mature crab burrow walls, rhizosphere and non-rhizosphere.
We conducted a principal component analysis (PCA) to examine the invasiveness of salt marsh plants and sediment sources (Figure 7). Figure 7A shows that the invasive S. alterniflora and native P. australis have distinct traits. The invasive group is positively associated with PC2 and negatively associated with PC1, while the native group is positively associated with PC1 and negatively associated with PC2 (Figure 7A). The invasiveness of the plants is primarily influenced by photosynthesis, with negative effects from ROC, EOC, and TOC. In contrast, the native group is positively influenced by root respiration and negatively influenced by crabs, burrows, AMF, and sprouts.
Figure 7 Principal component analysis results based on: (A) the invasiveness, and (B) sediment source.
However, the sediment traits from different sources are not separated (Figure 7B). There is considerable overlap between sediments from mudflat, juvenile crab burrows, and non-rhizosphere, indicating similarity among these sampling types. The rhizosphere is influenced by PC1-related variables, while PC2-related variables influence mature crab burrows. PC1-related variables include photosynthesis and enzymes, while PC2-related variables include the density and size of crabs and burrows, as well as sediment carbon contents (Supplementary Figure S3).
To investigate the dietary preferences of crabs, researchers often consider the δ13C of crabs, plants, and sediments as key parameters. For instance, fiddler crabs in Georgia salt marshes exhibit a biased isotopic composition towards C4 plants (Haines, 1976). Chiromantes dehaani crabs show a preference for P. australis over sediments. Among various plant tissues, crabs favor sprout leaves (35.7%) the most and falling litter (6.8%) the least (Zhang and Tong, 2018). This difference may be attributed to variations in food sources, as C. haematocheir crabs inhabit P. australis sites, which have higher crude protein content (Ji et al., 2011). These proteins are more edible but require more energy to be converted into CO2 through cellular respiration compared to those in P. australis. A more efficient and animal-friendly method to determine their food source is by analyzing the isotopic content in their exhaled breath (Ouyang et al., 2021). The CO2 production of Sesarmid crabs is 0.12 mmol g-1 wet wt day-1 (Ouyang et al., 2021), while ocypodid crabs range from 0.05 to 0.15 mmol g-1 wet wt day-1 (Kristensen et al., 2008; Penha-Lopes et al., 2010). These CO2 production rates are lower than the CO2 production observed in Chiromantes crabs during this experiment (0.960 ± 0.506 μmol/s individual and 0.389 ± 0.188 μmol/s individual for C. haematocheir and C. dehaani, respectively), which can be attributed to the larger size of Chiromantes crabs. By considering the carapace width, the CO2 production in our study falls within a similar range as other research findings.
The invasion of S. alterniflora in mangrove forests has led to significant changes in the diets of crabs and other gastropod species (Feng et al., 2018). Herbivorous and detritus feeders exhibit species-specific changes in their food sources during invasion (Nordström et al., 2015). For instance, herbivorous feeders like Sesarma plicata rapidly change their food within three years, while detritus feeders like Uca arcuata show no immediate change (Feng et al., 2018).
The invasion of Spartina also affects the biodiversity and abundance of benthic macrofauna in seafloor and marsh habitats (Cutajar et al., 2012). However, it has minimal impact on invertebrate and fish richness and diversity in Australia (Neira et al., 2007; Curado et al., 2014; Shin et al., 2022). In salt marshes invaded by Spartina alterniflora and subsequently restored, the biodiversity remains similar or even higher compared to preserved and invaded salt marshes (Curado et al., 2014). Nevertheless, hybrid Spartina facilitates the colonization of non-indigenous invertebrate species in edge areas and oligochaetes at higher elevations (Neira et al., 2007).
In China, invasive S. alterniflora supports more epiphytic communities compared to the native P. australis region. The preference of microbenthic organisms is also influenced by different stand states. For example, dead S. alterniflora provides a favorable habitat for bacterivores and fungivores epiphytic species (Chen et al., 2015). However, the additional invasion of hybrid Spartina does not necessarily increase biomass and detrital input into the food web (Brusati and Grosholz, 2009), and can even become part of the aquatic food webs in estuarine ecosystems (Li et al., 2009). The interaction between plant invasion and the allocation of microbenthic organisms is species-specific, and further research is needed to understand the general mechanisms and ecosystem functioning processes (Li et al., 2009).
Crab burrows and plant rhizosphere soil had more carbon transfer into easy oxidized carbon (EOC) and labile forms than other soil. Plant invasion can directly and indirectly alter the physical properties of sediment. The focus of this study is the invaded salt marsh site dominated by S. alterniflora, a fast-growing plant species with high productivity and well-developed below-ground rhizomes (Li et al., 2009; Feng et al., 2018). This invasion can reduce the energy of tidal flow, leading to decreased detritus transport to the ocean and increased detritus production from litter (Neira et al., 2007). In some of the invaded sites of S. alterniflora, the rapid spread can result in the disappearance of mudflats and native vegetation (Lee and Khim, 2017). The change in food sources could significantly affect the functions of the macrobenthos food web (Tue et al., 2012). From Feng et al. (2014) all benthic species switched their heterogenous diet to a homogeneous diet within a short period. This can adversely affect carbon contents in sediments and litter. Crab can also accelerate sediment turnover rate. For example, the net sediment transport to the marsh surface in Spartina alterniflora marsh by crabs is 109.54 g m-2 d-1, with a corresponding sediment turnover time of 4.07 years.
In addition to consuming salt marsh vegetation, the burrowing activity of crabs also affects the vertical movement of carbon in sediments. Research on fiddler crab burrows has shown that the rate of CO2 flux is 536.7 nmol g-1 h-1 for crabs, while the background sediment CO2 flux is 117.4 nmol g-1 h-1 (Grow et al., 2022). This rate is similar to the respiration rate of crabs in this experiment. The combination of small fiddler crabs and the sediment matrix leads to a CO2 flux that is comparable to that of larger Chiromantes crabs. In native S. alterniflora salt marshes in the United States of America, fiddler crabs can produce approximately 60 mmol m-2 d-1 more CO2 than Chiromantes crabs, which are smaller in size than fiddler crabs. Both crab respiration and burrowing activities contribute to an increase in CO2 release (Figure 4).
Salt marshes are highly productive wetlands that export dissolved inorganic and organic carbon into estuaries and oceans (Najjar et al., 2018; Xiao et al., 2021). However, the effects of burrows in these ecosystems are often underestimated and require further observation. In recent years, there has been increased attention on the carbon budget variation in mangrove forests, which is influenced by the large size of burrows and the significant changes caused by oxidation conditions and tidal fluctuations (Guimond et al., 2020). These changing environmental conditions accelerate sediment respiration processes, leading to the release of CO2, dissolved inorganic carbon (DIC), and dissolved organic carbon (DOC) through burrow walls (Kristensen et al., 2000; Maher et al., 2017). On the other hand, quantifying carbon budgets in salt marshes is more challenging compared to mangrove forests. This is due to the smaller size of burrows, the complexity of the root system, the salinity and strength of tidal wave fluxes, and the landscape variation in salt marshes. These factors contribute to exacerbated physical and chemical feedback from crab burrowing. Studies have shown that the exchange of porewater from crab burrows to the sediment matrix is lower compared to the opposite direction, and more than 75% of the exchanged carbon is in the form of DIC (Xiao et al., 2021).
From our results, sediments collected from mature crab burrow walls showed increased enzymatic activities related to CO2.Plant invasion can have varying effects on carbon-related enzymes, as shown by Zhou and Staver (2019). However, meta-analysis results indicate that invaded sites generally exhibit higher activities of nitrogen and phosphorous-related enzymes. The range of enzyme activity increases from 23% to 69% (Zhou and Staver, 2019). In our experiment, we observed significantly higher activity of the S-ACP enzyme (related to phosphorous cycling) in the rhizosphere of the invaded Spartina salt marsh. However, the activity of S-β-GC (related to carbon cycling) was not enhanced in the invasive site. There are a few possible explanations for this phenomenon. Firstly, since enzymes are proteins that require carbon and nitrogen for synthesis, the nutrient-rich environment may already provide sufficient resources, eliminating the need for increased production (Allison and Vitousek, 2005; Burns et al., 2013). Another explanation is that fast-growing invasive species contribute nitrogen-rich litter to the sediments, which may increase overall enzyme investment (Sardans et al., 2017; Zhou and Staver, 2019). The enhanced activity of sediment enzymes in releasing nutrients accelerates the nutrient cycle and creates a favorable environment for invasion. Although the activity of enzymes in crab burrow walls has not been extensively studied, the higher content of EOC (extractable organic carbon) aligns well with the increased enzymatic activity observed in sediment collected from burrow walls.
The goal of this research was to study the effects of plant invasiveness and crab on carbon cycling. Salinity levels vary between the sites, influencing the distribution of S. alterniflora in one of the sites due to its high salinity tolerance. The differences between the sites are believed to be primarily attributed to water salinity. Hydrolytic carbon-acquiring and oxidative carbon-acquiring enzyme activities are reduced by 33% and increased by 15%, respectively by under salination conditions. Although we conducted salinity tests at both sites, the samples analyzed for enzymatic activity did not correspond to the sampling period. Currently, it is challenging to assess the site effect, but this could be valuable for future studies.
The phylogenetic tree shows that the crabs inhabiting these sites are closely related, having previously belonged to the same genus before diverging. Sesarma crabs construct intricate and interconnected burrows. Initially, we planned to create casts of these burrows by using epoxy resin; however, the cool and moist conditions within these extensive burrows made it difficult to obtain casts. While we successfully carried out this operation for fiddler crab burrows, no analysis was conducted on soil enzymatic activity or crab respiration at that time. It is unfortunate that we could not investigate the effects of these burrow structures on carbon cycling in our current research. Nevertheless, this perspective presents a promising avenue for further exploration.
Our findings indicate that plant invasiveness leads to higher CO2 efflux in native P. australis sites compared to invasive S. alterniflora sites. Other factors such as crab bioturbation (respiration, food intake, and burrowing), arbuscular mycorrhiza fungi infection, photosynthetic rate, and changes in organic carbon also contribute to greater carbon sequestration in invasive sites. However, the content and enzymatic activity of EOC does not result in lower carbon sequestration in P. australis. We observed significantly higher levels of TC, TN, TOC, ROC, EOC, S-ACT, S-β-GC, and S-ACP activities in mature crab burrows. In contrast, juvenile crab burrows showed no significant differences in biogeochemical properties compared to mudflats and non-rhizosphere sediment. Therefore, the carbon pool and organic carbon fraction vary across different zones. This study emphasizes the importance of understanding below-ground carbon cycling processes concerning crab and plant invasion. Further research on microbial communities and functional groups can provide a better understanding of the mechanisms behind nutrient cycling in crab and plant invasions.
The original contributions presented in the study are included in the article/Supplementary Material. Further inquiries can be directed to the corresponding author.
The manuscript presents research on animals that do not require ethical approval for their study.
YH: Conceptualization, Data curation, Formal analysis, Investigation, Methodology, Visualization, Writing – original draft, Writing – review & editing. XL: Conceptualization, Data curation, Funding acquisition, Methodology, Project administration, Supervision, Validation, Visualization, Writing – review & editing. HC: Data curation, Investigation, Supervision, Writing – review & editing. LR: Conceptualization, Data curation, Supervision, Writing – review & editing. JT: Conceptualization, Funding acquisition, Resources, Writing – original draft.
The author(s) declare financial support was received for the research, authorship, and/or publication of this article. This research is funded by the National Natural Science Foundation of China (42141016), and Research Program of Shanghai Science and Technology (22dz1209600).
XL and JT managed projects and funding. We sincerely thank the reviewers for their insightful comments to improve the manuscript.
The authors declare that the research was conducted in the absence of any commercial or financial relationships that could be construed as a potential conflict of interest.
All claims expressed in this article are solely those of the authors and do not necessarily represent those of their affiliated organizations, or those of the publisher, the editors and the reviewers. Any product that may be evaluated in this article, or claim that may be made by its manufacturer, is not guaranteed or endorsed by the publisher.
The Supplementary Material for this article can be found online at: https://www.frontiersin.org/articles/10.3389/fmars.2024.1413145/full#supplementary-material
Agusto L. E., Qin G., Thibodeau B., Tang J., Zhang J., Zhou J., et al. (2022). Fiddling with the blue carbon: Fiddler crab burrows enhance CO2 and CH4 efflux in saltmarsh. Ecol. Indic. 144, 109538. doi: 10.1016/j.ecolind.2022.109538
Alberti J., Daleo P., Fanjul E., Escapa M., Botto F., Iribarne O. (2015). Can a single species challenge paradigms of salt marsh functioning? Estuaries Coast. 38, 1178–1188. doi: 10.1007/s12237–014-9836-z
Allison S. D., Vitousek P. M. (2005). Responses of extracellular enzymes to simple and complex nutrient inputs. Soil Biol. Biochem. 37, 937–944. doi: 10.1016/j.soilbio.2004.09.014
Altieri A. H., Bertness M. D., Coverdale T. C., Herrmann N. C., Angelini C. (2012). A trophic cascade triggers collapse of a salt-marsh ecosystem with intensive recreational fishing. Ecology 93, 1402–1410. doi: 10.1890/11-1314.1
Andreetta A., Fusi M., Cameldi I., Cimò F., Carnicelli S., Cannicci S. (2014). Mangrove carbon sink. Do burrowing crabs contribute to sediment carbon storage? Evidence from a Kenyan mangrove system. J. Sea Res. 85, 524–533. doi: 10.1016/j.seares.2013.08.010
Bertness M. D., Coverdale T. C. (2013). An invasive species facilitates the recovery of salt marsh ecosystems on Cape Cod. Ecology 94, 1937–1943. doi: 10.1890/12-2150.1
Bortolus A., Iribarne O. (1999). Effects of the SW Atlantic burrowing crab Chasmagnathus granulata on a Spartina salt marsh. Mar. Ecol. Prog. Ser. 178, 79–88. doi: 10.3354/meps178079
Brusati E. D., Grosholz E. D. (2009). Does invasion of hybrid cordgrass change estuarine food webs? Biol. Invasions 11, 917–926. doi: 10.1007/s10530-008-9304-4
Bueno De Mesquita C. P., Knelman J. E., King A. J., Farrer E. C., Porazinska D. L., Schmidt S. K., et al. (2017). Plant colonization of moss-dominated soils in the alpine: Microbial and biogeochemical implications. Soil Biol. Biochem. 111, 135–142. doi: 10.1016/j.soilbio.2017.04.008
Burns R. G., Deforest J. L., Marxsen J., Sinsabaugh R. L., Stromberger M. E., Wallenstein M. D., et al. (2013). Soil enzymes in a changing environment: Current knowledge and future directions. Soil Biol. Biochem. 58, 216–234. doi: 10.1016/j.soilbio.2012.11.009
Chen H., Zhang P., Li B., Wu J. (2015). Invasive cordgrass facilitates epifaunal communities in a Chinese marsh. Biol. Invasions 17, 205–217. doi: 10.1007/s10530-014-0720-3
Chen X., He Q., Xin P., Gong Z., Zhou Z., Zhang C. (2021). Research progress on the biological disturbed behavior process of crabs in the tidal flats of estuaries and coasts. Mar. Sci. 45, 113–122. doi: 10.11759/hykx20200726001
Chu W., Lu F., Zhu W., Kang C. (2011). Isolation and characterization of new potential probiotic bacteria based on quorum-sensing system. J. Appl. Microbiol. 110, 202–208. doi: 10.1111/jam.2010.110.issue-1
Coverdale T. C., Altieri A. H., Bertness M. D. (2012). Belowground herbivory increases vulnerability of New England salt marshes to die-off. Ecology 93, 2085–2094. doi: 10.1890/12-0010.1
Curado G., Sánchez-Moyano J. E., Figueroa E., Castillo J. M. (2014). Do spartina maritima plantations enhance the macroinvertebrate community in European salt marshes? Estuaries Coast. 37, 589–601. doi: 10.1007/s12237–013-9713–1
Cutajar J., Shimeta J., Nugegoda D. (2012). Impacts of the invasive grass Spartina anglica on benthic macrofaunal assemblages in a temperate Australian saltmarsh. Mar. Ecol. Prog. Ser. 464, 107–120. doi: 10.3354/meps09826
Feng J., Guo J., Huang Q., Jiang J., Huang G., Yang Z., et al. (2014). Changes in the community structure and diet of benthic macrofauna in invasive spartina alterniflora wetlands following restoration with native mangroves. Wetlands (Wilmington). 34, 673–83. doi: 10.1007/s13157-014-0533-2
Feng J., Huang Q., Chen H., Guo J., Lin G. (2018). Restoration of native mangrove wetlands can reverse diet shifts of benthic macrofauna caused by invasive cordgrass. J. Appl. Ecol. 55, 905–916. doi: 10.1111/1365-2664.12987
Feng J., Yu D., Sinsabaugh R. L., Moorhead D. L., Andersen M. N., Smith P., et al. (2023). Trade-offs in carbon-degrading enzyme activities limit long-term soil carbon sequestration with biochar addition. Biol. Rev. Camb Philos. Soc. 98, 1184–1199. doi: 10.1111/brv.12949
Ge X., Criswold K. C., Newman A. J. (2023). Warmer and more seasonal climates reduce the effect of top-down population control: An example with aphids and ladybirds. Funct. Ecol. 37, 1604–1619. doi: 10.1111/1365-2435.14326
Gillis L. G., Snavely E., Lovelock C., Zimmer M. (2019). Effects of crab burrows on sediment characteristics in a Ceriops australis-dominated mangrove forest. Estuarine Coast. Shelf Sci. 218, 334–339. doi: 10.1016/j.ecss.2019.01.008
Grow A. K., Schutte C. A., Roberts B. J. (2022). Fiddler crab burrowing increases salt marsh greenhouse gas emissions. Biogeochemistry 158, 73–90. doi: 10.1007/s10533-021-00886-5
Guimond J. A., Seyfferth A. L., Moffett K. B., Michael H. A. (2020). A physical-biogeochemical mechanism for negative feedback between marsh crabs and carbon storage. Environ. Res. Lett. 15, 34024. doi: 10.1088/1748-9326/ab60e2
Gutiérrez J. L., Jones C. G., Groffman P. M., Findlay S. E. G., Iribarne O. O., Ribeiro P. D., et al. (2006). The contribution of crab burrow excavation to carbon availability in surficial salt-marsh sediments. Ecosystems 9, 647–658. doi: 10.1007/s10021-006-0135-9
Haines E. B. (1976). Relation between the stable carbon isotope composition of fiddler crabs, plants, and soils in a salt marsh1. Limnol. Oceanogr. 21, 880–883. doi: 10.4319/lo.1976.21.6.0880
Harada Y., Lee S. Y. (2016). Foraging behavior of the mangrove sesarmid crab Neosarmatium trispinosum enhances food intake and nutrient retention in a low-quality food environment. Estuarine Coast. Shelf Sci. 174, 41–48. doi: 10.1016/j.ecss.2016.03.017
Hassan W., Bashir S., Ahmed N., Tanveer M., Shah A. N., Bano R., et al. (2016). Labile organic carbon fractions, regulator of co2 emission: effect of plant residues and water regimes. CLEAN – Soil, Air, Water. 10, 1268–427. doi: 10.1002/clen.201400405
Hinson A. L., Feagin R. A., Eriksson M., Najjar R. G., Herrmann M., Bianchi T. S., et al. (2017). The spatial distribution of soil organic carbon in tidal wetland soils of the continental United States. Glob. Chang. Biol. 23, 5468–5480. doi: 10.1111/gcb.13811
Huhta V. (2007). The role of soil fauna in ecosystems: A historical review. Pedobiologia (Jena) 50, 489–495. doi: 10.1016/j.pedobi.2006.08.006
IPCC. (2013). Climate Change 2013: The Physical Science Basis. (Cambridge: Cambridge University Press).
Ji Y., Wu B., Ding Y., Qin P.. (2011). “Nutritional components of Phragmites australis and Spartina alterniflora in Dafeng free range David’s Deer habitat of Jiangsu Province, East China: A comparative analysis”. Chin. J. Ecol. 30, 2240–2244. doi: 10.3724/SP.J.1011.2011.00187
Khoshnevisan B., Bashir M. A., Sun Q., Pan J., Wang H., Xu Y., et al. (2021). Optimal rice-crab co-culture system as a new paradigm to air-water-food nexus sustainability. J. Clean Prod. 291, 125936. doi: 10.1016/j.jclepro.2021.125936
Koo B. J., Kim S., Hyun J. (2019). Feeding behavior of the ocypodid crab Macrophthalmus japonicus and its effects on oxygen-penetration depth and organic-matter removal in intertidal sediments. Estuarine Coast. Shelf Sci. 228, 106366. doi: 10.1016/j.ecss.2019.106366
Kristensen E., Flindt M. R., Ulomi S., Borges A. V., Abril G., Bouillon S. (2008). Emission of CO2 and CH4 to the atmosphere by sediments and open waters in two Tanzanian mangrove forests. Mar. Ecol. Prog. Ser. 370, 53–67. doi: 10.3354/meps07642
Kristensen E., Liebezeit G., Dittmann S., Kroencke I. (2000). Organic matter diagenesis at the oxic/anoxic interface in coastal marine sediments, with emphasis on the role of burrowing animals. Hydrobiologia 426, 1–24. doi: 10.1023/A:1003980226194
Lee S. Y., Khim J. S. (2017). Hard science is essential to restoring soft-sediment intertidal habitats in burgeoning East Asia. Chemosphere 168, 765–776. doi: 10.1016/j.chemosphere.2016.10.136
Li B., Liao C., Zhang X., Chen H., Wang Q., Chen Z., et al. (2009). Spartina alterniflora invasions in the Yangtze River estuary, China: An overview of current status and ecosystem effects. Ecol. Eng. 35, 511–520. doi: 10.1016/j.ecoleng.2008.05.013
Li S., Sun L., Wu H., Hu Z., Liu W., Li Y., et al. (2012). The intestinal microbial diversity in mud crab (Scylla paramamosain) as determined by PCR-DGGE and clone library analysis. J. Appl. Microbiol. 113, 1341–1351. doi: 10.1111/jam.2012.113.issue-6
Liu H., Wang L., Liu M., Wang B., Jiang K., Ma S., et al. (2011). The intestinal microbial diversity in Chinese shrimp (Fenneropenaeus chinensis) as determined by PCR–DGGE and clone library analyses. Aquaculture 317, 32–36. doi: 10.1016/j.aquaculture.2011.04.008
Luo B., Li X., Luo D., Xu P., Chen X., You W. (2019). Preference of Helice tientsinensis and Sesarma plicata for typical salt marsh plants in the north of Hangzhou Bay. J. East China Norm. Univ. (Natur. Sci.) 6, 123–131. doi: 10.3969/j.issn.1000.5641.2019.06.012
Maher D. T., Santos I. R., Schulz K. G., Call M., Jacobsen G. E., Sanders C. J. (2017). Blue carbon oxidation revealed by radiogenic and stable isotopes in a mangrove system. Geophys. Res. Lett. 44, 4889–4896. doi: 10.1002/2017GL073753
McGonigle T. P., Miller M. H., Evans D. G., Fairchild G. L., Swan J. A.. (1990). A new method which gives an objective measure of colonization of roots by vesicular-arbuscular mycorrhizal fungi. New Phytologist. 115, 495–501.
Najjar R. G., Herrmann M., Alexander R., Boyer E. W., Burdige D. J., Butman D., et al. (2018). Carbon budget of tidal wetlands, estuaries, and shelf waters of Eastern North America. Global Biogeochem. Cycles 32, 389–416. doi: 10.1002/2017GB005790
Neira C., Levin L. A., Grosholz E. D., Mendoza G. (2007). Influence of invasive Spartina growth stages on associated macrofaunal communities. Biol. Invasions 9, 975–993. doi: 10.1007/s10530-007-9097-x
Nordström M. C., Demopoulos A. W. J., Whitcraft C. R., Rismondo A., McMillan P., Gonzalez J. P., et al. (2015). Food web heterogeneity and succession in created saltmarshes. J. Appl. Ecol. 52, 1343–1354. doi: 10.1111/1365-2664.12473
Ouyang X., Lee S. Y. (2014). Updated estimates of carbon accumulation rates in coastal marsh sediments. Biogeosciences 11, 5057–5071. doi: 10.5194/bg-11-5057-2014
Ouyang X., Lee C. Y., Lee S. Y. (2021). Effects of food and feeding regime on CO2 fluxes from mangrove consumers – Do marine benthos breathe what they eat? Mar. Environ. Res. 169, 105352. doi: 10.1016/j.marenvres.2021.105352
Penha-Lopes G., Kristensen E., Flindt M., Mangion P., Bouillon S., Paula J. (2010). The role of biogenic structures on the biogeochemical functioning of mangrove constructed wetlands sediments – A mesocosm approach. Mar. Pollut. Bull. 60, 560–572. doi: 10.1016/j.marpolbul.2009.11.008
Rovira P., Vallejo V. R. (2002). Labile and recalcitrant pools of carbon and nitrogen in organic matter decomposing at different depths in soil; an acid hydrolysis approach. Geoderma 107, 109–141. doi: 10.1016/S0016-7061(01)00143-4
Sardans J., Bartrons M., Margalef O., Gargallo-Garriga A., Janssens I. A., Ciais P., et al. (2017). Plant invasion is associated with higher plant-soil nutrient concentrations in nutrient-poor environments. Glob. Chang. Biol. 23, 1282–1291. doi: 10.1111/gcb.13384
Sheng P., Liu R., Li M. (2011). Methodological comparison of observation and colonization measurement of arbuscular mycorrhizal fungi. Mycosystema 30, 519–525. doi: 10.13346/j.mycosystema.2011.04.002
Shin W., Oh M., Hong J., Byun C., Lee E. J. (2022). Early invasion of common cordgrass (Spartina anglica) increases belowground biomass and decreases macrofaunal density and diversity in a tidal flat marsh. Biol. Invasions 24, 3615–3629. doi: 10.1007/s10530-022-02866-8
Sinsabaugh R. L., Lauber C. L., Weintraub M. N., Ahmed B., Allison S. D., Crenshaw C., et al. (2008). Stoichiometry of soil enzyme activity at global scale. Ecol. Letter 11, 1252–1264. doi: 10.1111/j.1461-0248.2008.01245.x
Soti G. P., Toprak B., Rosa D. N., Jayachandran K.. (2021) Influence of land use intensity and management on arbuscular mycorrhizal fungi-avocado symbiosis. J. Agric. Sci. 12, 3: 10–16. doi: 10.5539/jas.v13n3p10
Stieglitz T., Ridd P., Müller P. (2000). Passive irrigation and functional morphology of crustacean burrows in a tropical mangrove swamp. Hydrobiologia 421, 69–76. doi: 10.1023/A:1003925502665
Tang J. W., Ye S. F., Chen X. C., Yang H. L., Sun X. H., Wang F. M., et al. (2018). Coastal blue carbon: Concept, study method, and the application to ecological restoration. Sci. China Earth Sci. 61, 637–646. doi: 10.1007/s11430-017-9181-x
Thongtham N., Kristensen E., Puangprasan S. (2008). Leaf removal by sesarmid crabs in Bangrong mangrove forest, Phuket, Thailand; with emphasis on the feeding ecology of Neoepisesarma versicolor. Estuarine Coast. Shelf Sci. 80, 573–580. doi: 10.1016/j.ecss.2008.09.017
Tue N. T., Hamaoka H., Sogabe A., Quy T. D., Nhuan M. T., Omori K. (2012). Food sources of macro-invertebrates in an important mangrove ecosystem of Vietnam determined by dual stable isotope signatures. J. Sea Res. 72, 14–21. doi: 10.1016/j.seares.2012.05.006
Wang J. Q., Zhang X. D., Bertness M. D., Fang C. M., Chen J. K., Hara T., et al (2010). Bioturbation of burrowing crabs promotes sediment turnover and carbon and nitrogen movements in an estuarine salt marsh. Ecosystems 13, 586–599. doi: 10.1007/s10021-010-9342-5
Xiao K., Wilson A. M., Li H., Santos I. R., Tamborski J., Smith E., et al. (2021). Large CO2 release and tidal flushing in salt marsh crab burrows reduce the potential for blue carbon sequestration. Limnol. Oceanogr. 66, 14–29. doi: 10.1002/lno.11582
Yang S., Sun X., Ding J., Jiang Z., Liu X., Xu J. (2020). Effect of biochar addition on CO2 exchange in paddy fields under water-saving irrigation in Southeast China. J. Environ. Manage 271, 111029. doi: 10.1016/j.jenvman.2020.111029
Zhang Y., Tong C. F. (2018). Stomach content characteristics and feeding preference of Chiromantes dehaani in the salt marsh of Yangtze estuary. Chin. J. Ecol. 37, 2059–2066. doi: 10.13292/j.1000–4890.201807.022
Zhou Y., Staver A. C. (2019). Enhanced activity of soil nutrient-releasing enzymes after plant invasion: a meta-analysis. Ecology 100, e2830. doi: 10.1002/ecy.2830
Keywords: carbon storage, salt marshes, crab bioturbation, plant invasion, mycorrhiza fungi, sediment organic carbon, enzymatic activity
Citation: Hua Y, Chen H, Ren L, Tang J and Li X (2024) Crab bioturbation reduces carbon storage in salt marshes under more robust mechanisms than plant invasiveness. Front. Mar. Sci. 11:1413145. doi: 10.3389/fmars.2024.1413145
Received: 06 April 2024; Accepted: 20 May 2024;
Published: 11 June 2024.
Edited by:
Qin Zhu, Southern Marine Science and Engineering Guangdong Laboratory (Guangzhou), ChinaReviewed by:
Xiaoguang Ouyang, Southern Marine Science and Engineering Guangdong Laboratory (Guangzhou), ChinaCopyright © 2024 Hua, Chen, Ren, Tang and Li. This is an open-access article distributed under the terms of the Creative Commons Attribution License (CC BY). The use, distribution or reproduction in other forums is permitted, provided the original author(s) and the copyright owner(s) are credited and that the original publication in this journal is cited, in accordance with accepted academic practice. No use, distribution or reproduction is permitted which does not comply with these terms.
*Correspondence: Xiuzhen Li, eHpsaUBza2xlYy5lY251LmVkdS5jbg==
Disclaimer: All claims expressed in this article are solely those of the authors and do not necessarily represent those of their affiliated organizations, or those of the publisher, the editors and the reviewers. Any product that may be evaluated in this article or claim that may be made by its manufacturer is not guaranteed or endorsed by the publisher.
Research integrity at Frontiers
Learn more about the work of our research integrity team to safeguard the quality of each article we publish.