- 1Cawthron Institute, Nelson, New Zealand
- 2Food BioSciences Department, Teagasc Food Research Centre, Dublin, Ireland
- 3Algavive Limited, Nelson, New Zealand
The giant kelp (Macrocystis pyrifera (Linnaeus) C. Agardh 1820) is a habitat-forming brown seaweed in temperate systems with an unexplored potential as a source of seaweed bioproducts. This study used M. pyrifera sporophytes sourced in Tasmania, Australia, to investigate the effect of photoperiod and temperature on growth rates and the nutritional characteristics of the resulting juvenile biomass. Four cultivation treatments combined growth temperatures of 12 °C, 15 °C, 18 °C with light:dark (L:D) of 12:12 and 16:8 (L:D) photoperiods, (12 °C – (12:12); 12 °C – (16:8); 15 °C – (12:12); 18 °C – (12:12) to investigate their effect on the number and size of sporophytes, biomass accumulation and nutritional composition. After 60 days of cultivation the 12 °C – (12:12) treatment had the greatest number of juvenile sporophytes, and the greatest biomass of 14 ± 1.3 g dry weight (DW). The lowest biomass of 1 g DW, was obtained from the 18 °C – (12:12) treatment. The protein content across all treatments ranged from 16-22.48% DW, with the 12 °C (12:12) treatment having largest range, then the 12°C (16:18) treatment was next with 18.48-22.48% DW, and the 15°C (12:12) treatment had the lowest protein range with 16.48-18% DW. These results are in the range of protein content previously reported for brown seaweeds of 5-20%. Total polysaccharide content ranged from 9.6-16.2% DW with the highest content of 16.2% DW obtained for the 15 °C – (12:12) treatment, and the lowest total polysaccharide content of 9.6% DW obtained for the 12 °C (16:18) treatment. After 66 days of cultivation, the highest yield of sulphated polysaccharides of 0.4% DW was obtained for the 12 °C (12:12) treatment. Total fatty acids were analysed, with the highest polyunsaturated fatty acid content of 60.4% detected in the 12 °C (12:12) treatment. This study demonstrates that temperature and photoperiod are factors impacting juvenile sporophyte growth, biomass accumulation and biochemical composition. The study showed the least stressed sporophytes produced the most potentially beneficial nutritional or bioactive profile.
1 Introduction
Brown seaweeds (phylum Ochrophyta) are an important aquaculture species for human food and animal feed industries, predominately as whole foods or as feed additives (Bixler and Porse, 2011). Their cultivation has increased annually by 10.9% from 1950 to 2019, resulting in a global harvest of 16.4 million tonnes in 2019 compared to 13,000 tonnes in 1950. This economic production rate has exceeded all other aquaculture species (e.g. finfish, crustaceans, molluscs and other aquatic animals), which only grew by 7.9% during the same period (Cai et al., 2021). In 2019, brown seaweed accounted for 47.3% of the world’s seaweed cultivation needs in terms of tonnage and 52% in terms of value.
Despite nearly 300 seaweed species investigated for potential commercial species (Ferdouse et al., 2018), the large majority of the production of brown seaweed comprises only two genera, Saccharina and Undaria, with Saccharina japonica being the most widely cultivated and accounting for 12.3 million tonnes or 35.4% of all seaweeds produced in 2019 (Cai et al., 2021). Thus, the brown seaweed species play a vital role in the seaweed industry, but the commercial potential of many species has not been very well investigated.
The majority of seaweed industry products (~85%) are for human consumption (Ferdouse et al., 2018). The main commercial seaweed extracts are hydrocolloids which include agar, alginates and carrageenans, and they account for ~40% of the global hydrocolloids market in terms of food in 2018 (Ferdouse et al., 2018). In the food industry, the most useful phycocolloid sourced from brown seaweed is alginate (McHugh, 2003). Alginates are mainly used in food as thickening or gelling agents and emulsion stabilizers (McHugh, 2003; Bixler and Porse, 2011). Other brown seaweed products include fucoidans which are also sulphated polysaccharides and exhibit bioactivity including, e.g. anti-inflammatory, antioxidant, antibacterial and immunological activity; lipid inhibition; and obesity prevention or treatment (Cumashi et al., 2007; Park et al., 2011; Fitton et al., 2015; Synytsya et al., 2015).
Fatty acids from seaweeds and plants are used as a source of carbon-18 (C18) polyunsaturated fatty acids (PUFAs) for human and fish diets. They are excellent sources of n−3 fatty acids with 18 to 20+ carbons, such as C20:5n3 eicosapentaenoic acid (EPA) and C22:6n3 docosahexaenoic acid (DHA), which have been mainly sourced from fish oils yet had originally been found in seaweed (Schmid et al., 2018; Tocher et al., 2019; Rocha et al., 2021). Seaweed species generally contain higher proportions of PUFAs in their fatty acid profile and a low ratio of n−6/n−3 PUFAs (Schmid et al., 2018). Saccharina japonica showed seasonal variations in PUFAs, with higher content during the colder months when seaweed thalli were young (Hafting et al., 2015; Purcell-Meyerink et al., 2021). Total fatty acid (TFA) content varies considerably among seaweed types, with a range of 0.6%–7.8% dry weight (DW), with the browns having the highest content, followed by the greens, and then by the red seaweeds having the lowest average TFA % DW (Schmid et al., 2018). The fat content in seaweeds includes fatty acids and is <5% DW. A previous study on M. pyrifera found fat content reached 3.3%–3.7% DW and <1.5% DW in another study sampled in proximity to salmon farms. Two other commercially grown brown seaweeds had similar values of 0.5%–1% and 0.5% fat respectively for Laminaria digitata and Saccharina latissima (Morrissey et al., 2001; Manns et al., 2014; Purcell et al., 2022; Méndez et al., 2024).
The protein content in brown seaweeds has been reported to be between 5% and 20%, and are being considered as an alternative protein source to animal protein (Tønnesen and Karlsen, 2002; Qin, 2008; Synytsya et al., 2015; Fertah et al., 2017). For kelps, M. pyrifera has a protein content of 13.2% ± 0.30% DW, L. digitata with 15.9% ± 0.83% DW and Undaria pinnatifida with 16.8% DW (MacArtain et al., 2007; Marsham et al., 2007; Taboada et al., 2013; Purcell et al., 2022). The protein content fluctuated seasonally with the highest protein in the first quarter and the lowest in the third quarter of the year for four kelp species, with ranges of 6.9% ± 1.1% in L. digitata, 6.8% ± 1.3% in Laminaria hyperborea, 7.1% ± 1.7% in S. latissima and 11.0% ± 1.4% in Alaria esculenta (Schiener et al., 2015).
Carbohydrates account for 13%–60% DW of brown seaweed (Holdt and Kraan, 2011; Rajauria, 2015). For kelps, carbohydrate content is within this range with 34.6% ± 3.1%, 33.2% ± 3.8%, 28.5% ± 3.9% and 37.4% ± 4.0% DW in L. digitata, L. hyperborea, S. latissima and A. esculenta, respectively (Schiener et al., 2015). The nutritional content of the fats (fatty acids and lipids), proteins and carbohydrates in brown seaweeds depends on the season and location of harvest (Morrissey et al., 2001; Manns et al., 2014; Purcell et al., 2022).
Macrocystis pyrifera (order Laminariales; giant kelp) is a large habitat-forming seaweed found in Australia, New Zealand, South Africa, California and Chile (Graham et al., 2007; Bolton et al., 2012; Schiel and Foster, 2015; Duong et al., 2022). It is an important habitat provider throughout its range and is a significant contributor to nitrogen cycling and primary production (Arnold and Manley, 1985; Hay, 1990; Krumhansl et al., 2016; Tait et al., 2021). There is a wide range of knowledge on the fundamental ecology and physiology of M. pyrifera and its potential aquaculture from California, Chile and Peru, with biomass yield projections based on seaweed farm size (Neushul, 1986; Perissinotto and McQuaid, 1992; McHugh, 2003; MacArtain et al., 2007; Vásquez, 2009; Westermeier et al., 2011; Vásquez et al., 2012; Correa et al., 2016; FAO, 2016; Camus and Buschmann, 2017; Avila-Peltroche and Padilla-Vallejos, 2020; Visch et al., 2023a; Kopczak et al., 1991; Mabin et al., 2019; Umanzor et al., 2020). However, there are fewer studies that have focused on the impact of seasonal cultivation conditions and environmental parameters on the biochemical and nutritional composition of laboratory/hatchery scale cultivated juvenile sporophyte grown from gametophytes for bioproduct potential. To facilitate the development of a wider range of seaweed species for aquaculture potential, optimal growth conditions and optimal biomass and compositional data specific to each species of interest are essential for the seaweed industry.
We investigated the effect of different photoperiods and temperatures on the growth performance and nutritional characteristics of the harvested seaweed biomass from juvenile sporophytes of M. pyrifera. Temperature and photoperiod regimes were chosen to mimic summer, autumn and winter conditions, with temperatures ranging from 12°C to 18°C and two photoperiods (light:dark) (12:12) and (16:8). These seasonal conditions were used to provide vital guidance on the effect of key environmental growth parameters on high-value nutrient composition including proteins, PUFAs and carbohydrates, specifically total polysaccharides and sulphated polysaccharides.
2 Materials and methods
2.1 Macrocystis pyrifera cultivation
An experimental flowchart detailing the cultivation methodology for M. pyrifera (Linnaeus) C. Agardh, 1820 juvenile sporophytes and the results gathered during the various growth phases is included in the (Supplementary Information). Essentially, M. pyrifera sporophytes were cultured in tanks for 90 days in four different conditions and sampled at three time points to monitor culture health and growth. Quadruplicate cultures were set up for each condition. Data were collected from T1 (30 days), T2 (60 days) and T3 (90 days), for sporophyte number and sporophyte size. Starting at T2 (60 days), multiple harvests were performed between T2 and T3 (every 6–7 days), and data were collected from both wet and dry weight biomass, as well as compositional analysis from the harvested biomass. Treatment #4* 18°C (12:12) was excluded from nutritional compositional analysis due to insufficient biomass.
The cultivation method employed was based on (Visch et al. 2023b). Fertile sorus tissue from 15 individuals was collected by diving in Blackmans Bay, Tasmania, Australia (43.0122°S, 147.3312°E), and zoospores were released using the methods described in (Forbord et al. 2018). Mature sorus tissue was dissected out of the main thallus with individuals treated separately. Sori were thoroughly cleaned with paper towel and washed three times for 10 s in an iodine solution (Betadine 112®; 5 mL L−1) with successive rinsing using sterile seawater, prior to being kept in a damp paper towel in the dark at 12°C overnight. Subsequently, zoospores were released in autoclaved seawater with F/2 media (Guillard and Ryther, 1962) and kept in aerated 0.2-μm PTFE syringe filters, in 3 L glass flasks at 12°C under red light conditions of ~15 μmol photons m−2 s−1, with a photoperiod of 16:8 h light–dark using LED lights (Fluval Aquasky®). The spores developed into gametophytes, which were then left to grow vegetatively for ~3 months under these culture conditions, with twice a week media changes (Bartsch, 2018).
The gametophytes were selected for use in the experiment after being screened for viability and contamination using a Nikon Eclipse TS2 microscope. Three weeks before the start of the experiment, the culture conditions were changed from red light to white light to stimulate gametogenesis and fertilization (Visch et al., 2023b). The gametophytes were kept in 0.5-L conical flasks at 12°C in a 12:12 h light–dark photoperiod, before being screened again. No more than 50,000 gametophytes mL−1 were used for seeding, and the gametophyte culture was evenly sprayed using a sterilized 1.25-L handheld pressure sprayer (Hozelock Ltd. Viton®) onto ~1 mm diameter rough texturized polyester seeding twine specifically developed for kelp aquaculture (AtSeaNova, AlgaeTex®) tightly wrapped around four PVC cylinder spools with a height 250 cm and a diameter of 60 mm × 4 per replicate. To allow the gametophytes and microscopic juvenile sporophytes to adhere, aeration in the tanks was absent for the first 3 days after spraying, after which it was gradually increased until the tanks were gently aerated, using a moderate airflow throughout at a rate of 400 L h−1, providing consistent water motion throughout each tank. Each rectangular cultivation tub contained 40 L of sterilized F/2 media made up of 0.22 μm pore, Millipore, ultraviolet-treated sterilized seawater. Aeration was provided by an air supply, which was filtered through sterile 0.2-μm syringe filters in a 25-mm diameter (PTFE) and connected to both ends of each tank using air stones, to ensure uniform aeration throughout the tank. All spools were suspended in the tanks from rods that ran across the top of each tank. Contamination was controlled using antibiotics: 3 mL of penicillin (100 mg L−1), 3 ml of streptomycin (100 mg L−1) and 1.5 mL of ampicillin (500 mg L−1) for bacteria and germanium dioxide (5 μM L−1) for diatoms. Antibiotics were only added when bacteria were detected during routine counts each week throughout the experiment, whereas germanium dioxide was used for diatom control throughout the experiment.
2.2 Experimental design
Four experimental treatments were run in parallel in a controlled temperature (CT) room, each experimental treatment combination consisting of temperature and photoperiod (Supplementary Information). Irradiance, nutrient concentrations (F/2 media) and air flow rate were constant across all treatments. Temperature was maintained using 55 W Aqua One submersible glass aquarium heaters with external temperature controls for all tanks. Photoperiod was regulated using pre-set timers, and each cultivation zone was enclosed to prevent light impact from other treatments. Irradiance was maintained using LED lights (Phillips, Cool Daylight & Warm, Model: WT060C:20W-LED25S/840), with neutral density filters to maintain a light level of 30 μmol photons m−2 s−1. Light levels were measured using a LI-COR Light Meter (LI-250A) with a spherical quantum sensor at the surface of the culture vessel. Temperature and light conditions were recorded using loggers (HOBO pendant, MX2202) in all treatments. Each treatment received air filtered with a 0.22-μm syringe (Millipore, 25 mm diameter, PTFE). Media changes (F/2 medium) and filtered seawater changes for each 40 L of replicate container were done twice a week.
The density of sporophytes in the cultures was evaluated by counting their number of on four sections of string (2 mm in length each) under a stereomicroscope (Nikon Eclipse TS2) at ×20 magnification on days 30, 60 and 90. Sporophyte size was measured using callipers (± 0.1 mm) at 30, 60 and 90 days on each 2 mm section of string at ×4 and ×10 magnifications following the method of (Camus and Buschmann 2017). At 60 days of cultivation, the increased size of the cultures required additional space and so the polyester seeding twine was removed from the PVC spools and hung in the tanks on the metal rods that held the spools in place, to keep them floating in the tanks while cultivation continued and to increase the surface-to-volume ratio of the growing sporophytes and to facilitate the nutrients and light availability. In addition, the irradiance was increased to 60 μmol photons m−2 s−1, and the media changes were increased from twice to four times a week to facilitate growth. The juvenile sporophytes were harvested at media changes, by draining them through a 100-µm polyester filter fabric sieve, then placed in sterile pre-weighed Ziplock bags and weighed to establish wet weight (WW) of biomass prior to freezing at −20°C. Frozen samples were then freeze-dried for 24–72 h (Model: GUSI Freeze Dryer) and re-weighed to identify dry weight (DW) of biomass. Wet weight (WW) and dry weight (DW) were subtracted to calculate the water content of the seaweed.
To provide enough biomass for compositional analysis, replicates within treatments had to be pooled. One of the four treatments, with the highest temperature of 18°C (12:12) #4, failed to produce enough biomass for compositional analysis, and biomass was harvested after 60 days (Figure 1). The other three treatments produced sufficient biomass for compositional analysis.
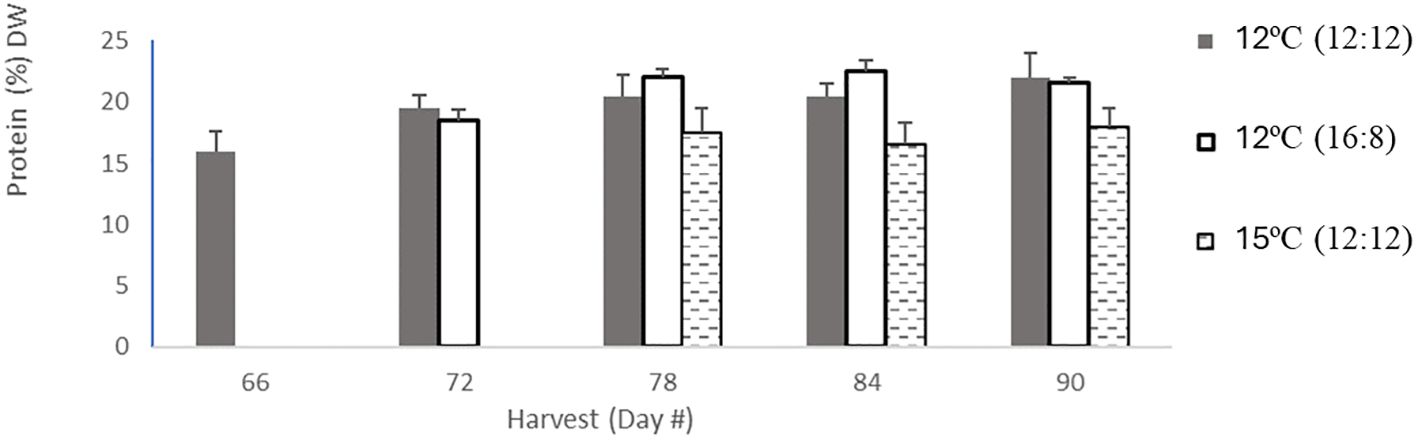
Figure 1 Protien content in percentage dry weight per harvest. Protein content for 12°C (12:12) had 5 harvests in light grey bars, then 12°C (16:8) had 4 harvests coloured in dark grey in bars, and 15°C (12:12) had 3 harvests in hatched black bars. Error bars are based on SD with n=3 analytical replicates.
2.3 Chemical analyses
Protein was analyzed using the Dumas AOAC method (AOAC, 1995) using a correction factor of 5 to quantify the seaweed protein (Bikker et al., 2020).
The total polysaccharide extraction method was a modification of a previously published method (Manns et al., 2014). In brief, sulphuric acid (12 M) was added to 0.1 g of freeze-dried sample and heated to 37°C for 1 h. The digest was diluted with Milli-Q to dilute the acid to ~4% and placed in a boiling water bath for a further 1 h. The hydrolyzed sample was then reacted with para-hydroxybenzoic acid hydrazide, and the absorbance was measured at 410 nm. The response was quantified against a galactose standard.
Sulphated polysaccharides were measured using a modified method based on (Zayed et al. 2020). Sulphate [SO4]2 was released from the total biomass using 1 mL of 2 M trifluoroacetic acid (TFA) and adding it to 0.01 g of dried sample and heating to 95°C for 20 min, thus liberating the sulphate groups. Sulphate was then precipitated from the solution using a barium chloride and gelatine solution. The absorption was measured using a spectrophotometer at 405 nm wavelength for sulphation. The response was quantified against a solution of sodium sulphate of known concentration.
Fatty acid detection and identification was performed using a standard AOAC 963.22 OMA online method for methyl esters of fatty acids (FAMEs) (AOAC, 1995). Due to the limited biomass available, a modification to the sample preparation was made, with the lipids within the available biomass being subjected to direct methylation without prior extraction of the sample. This was performed using a C19 internal standard. The same procedure for methylation and subsequent analysis was followed as outlined in the AOAC 963.22 OMA online method (AOAC, 1995).
2.4 Statistical analysis
Each treatment was analyzed against sporophyte size and density and compositional data. Both one-way and two-way ANOVA analyses were applied to the compositional data with treatment and culture time as factors. The software used for this analysis was the Excel add-in software Analysis ToolPak. All error bars on sporophyte size and density in the results are based on three independent measurements and three standard deviations (SD).
3 Results
3.1 Macrocystis pyrifera sporophyte biomass, density and size
Total biomass on day 90 from the four replicates was pooled for each of the four treatments, weighed and dried, and moisture loss was calculated (Appendix I). The total biomass after 90 days was as follows: 198 g for the 12°C (12:12) treatment, 100 g for the 12°C (16:8) treatment and 56 g WW for the 15°C (12:12) treatment, whereas the 18°C (12:12) treatment produced 1 g WW after 60 days. The dry weights (DWs) were as follows: 14 ± 1.3 g for 12°C (12:12), 7 ± 0.8 g for 12°C (16:8) and 5 ± 1.2 g for 15°C (12:12). Moisture loss was between 91.5% and 93% for all treatments. Changes in wet and dry biomass on different sampling days are detailed in (Appendix II).
The greatest sporophyte density (in count cm−1) was found in the 12°C (12:12) treatment, ranging from 744 ± 110 to 876 ± 213, and was similar for each of the three sampling times, based on a two-way ANOVA, and the factor regulating the growth of kelp sporophytes is temperature F = 32.59; p ≤ 0.001. Sporophyte densities were lower in all the other treatments. For both 12°C (16:8) and 15°C (12:12), density increased between 30 days and 60 days and remained similar thereafter, with the 12°C (16:8) treatment having 40% higher density at 90 days compared to 15°C (12:12). In the 18°C (12:12) treatment, there was little sporophyte development as well as the lowest densities of all treatments (Appendix III), likely due to the stress of being at this higher temperature.
The 12°C (12:12) treatment had the largest sporophyte size increase of all treatments with 0.11 ± 0.005 mm, 0.51 ± 0.020 mm and 2.00 ± 0.005 mm, at 30 days, 60 days and 90 days, respectively (Appendix III). In the 12°C (16:8) treatment, sporophyte size increases from 0.03 ± 0.015 mm, 0.32 ± 0.010 mm and 1.25 ± 0.006 mm, at 30 days, 60 days and 90 days, respectively, ~40% smaller than 12°C (12:12). In the 15°C (12:12) treatment, sporophyte size was almost half the size of the 12°C (16:8), with sizes of 0.014 ± 0.003 mm, 0.120 ± 0.003 mm and 0.21 ± 0.005 mm, at 30 days, 60 days and 90 days, respectively. Overall, the data indicated that sporophytes increased in size by 5-fold after 60 days and 20-fold after 90 days (Appendix III).
3.2 Macrocystis pyrifera nutritional composition
3.2.1 Protein
The protein content across all treatments ranged from 16% to 22% DW, with the 12°C (12:12) treatment also having this largest range, the 12°C (16:18) treatment having a narrower range of 18.48%–22.48% DW and the 15°C (12:12) treatment having the lowest protein range of 16.48%–18% DW (Table 1). All three treatments were analyzed, and the p-values of 0.01 and 0.0106 indicate statistically significant differences between all three treatments with p <0.05. Specifically, the 12°C (12:12) treatment was compared to the 12°C (16:18) treatment, with a p-value of 0.01, indicating that these treatments were significantly different, and the 12°C (12:12) treatment was compared to the 15°C (12:12) treatment, with a p-value of 0.0106 also indicating significant differences between these treatments (Appendix IV).
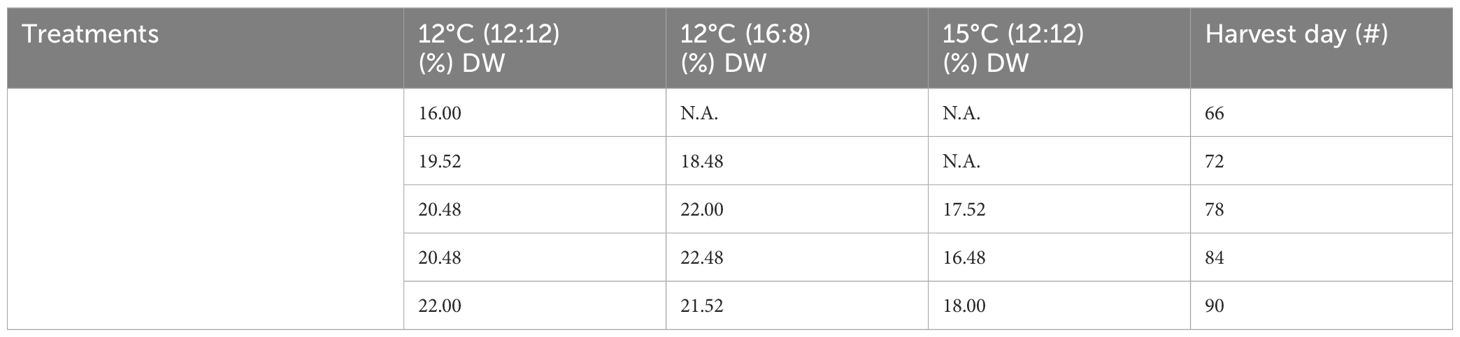
Table 1 Protein content from all treatments of Macrocystis pyrifera in (%) dry weight (DW) and all harvests.
Trends over time in the protein content were examined. The protein content of the 12°C (16:8) treatment and the 15°C (12:12) treatment both remained relatively constant over time, as shown in (Figure 1). In contrast, the 12°C (12:12) treatment protein content increased with each successive harvest. It is interesting to note in this study that the protein content numerically increased but was not indicative of biomass content (Appendix IV).
3.2.2 Total polysaccharides
Total polysaccharide results showed differences across all three treatments, with an increase over time for all the three treatments (Table 2; Figure 2). The lowest increase was for 12°C (12:12), with 9.9% DW after 66 days of cultivation, increasing to 10.6%, 12.3%, 12.4% and 13.8% DW for harvests on days 72, 78, 84 and 90, respectively. The 12°C (16:8) treatment increased to 9.6%, 11.4%, 12.5% and 14.5% DW on days 72, 78, 84 and 90, respectively. The 15°C (12:12) treatment had the largest polysaccharide content at 15%, 15% and 16.2% DW for harvest days 78, 84 and 90, respectively. Three replicates were analyzed and produced p-values of 0.0015 and 0.0090 between treatments indicating statistical significance (p < 0.05). Specifically, when the 12°C (12:12) was compared to the 12°C (16:8) treatment, the p-value was 0.0015 and therefore significant. The 12°C (12:12) treatment was also compared to the 15°C (12:12) treatment, and the p-value was 0.0090, indicating significance as the value is p <0.05 (Appendix V).
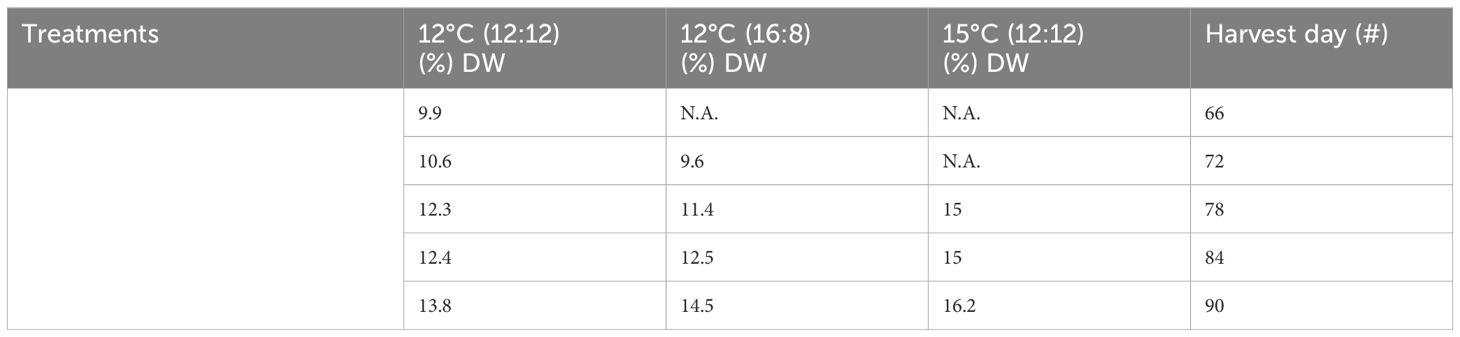
Table 2 Total polysaccharide content from all treatments and harvests of Macrocystis pyrifera in (%) DW.
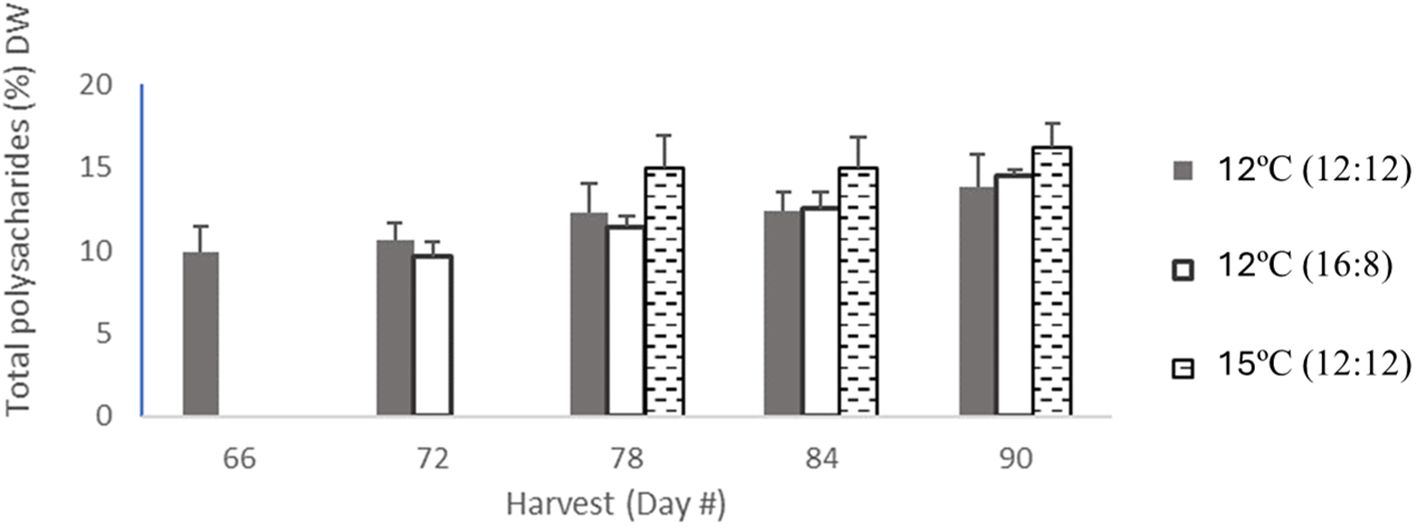
Figure 2 Total polysaccharide content from all treatments of Macrocytis pyriferia in % DW for each harvest which occurred between day 66 and day 90, with between 3 and 5 harvests. The total polysaccharide content for 12°C (12:12) with 5 harvests was coloured in light grey bars, then the 12°C (16:8) had 4 harvests coloured in dark grey in bars, and 15°C (12:12) had 3 harvests in hatched black bars. Error bars are based on SD with n=3 analytical replicates.
Analysis of the treatments at a per harvest scale in (Figure 2) noted that overall total polysaccharides for the 12°C (12:12) treatment had the lowest content per harvest but had the highest number of harvests (5), with the other two treatments having (4) and (3) harvests, respectively.
3.2.3 Sulphated polysaccharides
The highest level of sulphated polysaccharides (SPs) obtained in the study was 0.4% DW in the 12°C (12:12) treatment after 66 days of cultivation (Table 3; Figure 3). The per harvest SP content decreased over time in the 12°C (12:12) treatment, with levels of 0.4%, 0.3%, 0.27%, 0.24% and 0.21% DW being recorded for days 66, 72, 78, 84 and 90, respectively. The 12°C (16:8) and 15°C (12:12) treatments also showed a decreasing trend over time. For SP data per treatment analysis, had p-values of 0.016. and 0.038, indicating statistical significance between treatments. Specifically, 12°C (12:12) was compared to the 12°C (16:8) treatment, with a p-value of 0.016, and the 12°C (12:12) treatment was also compared to 15°C (12:12) with a p-value of 0.038 indicating significant differences between these treatments as the (p <0.05) (Appendix VI).
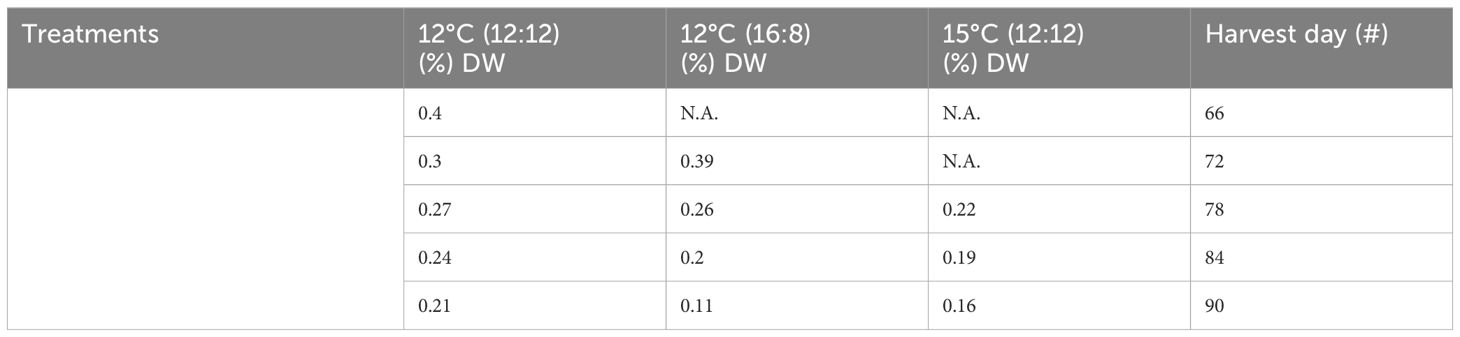
Table 3 Sulphated polysaccharide content from all treatments of Macrocystis pyrifera in (%) DW and all harvests.
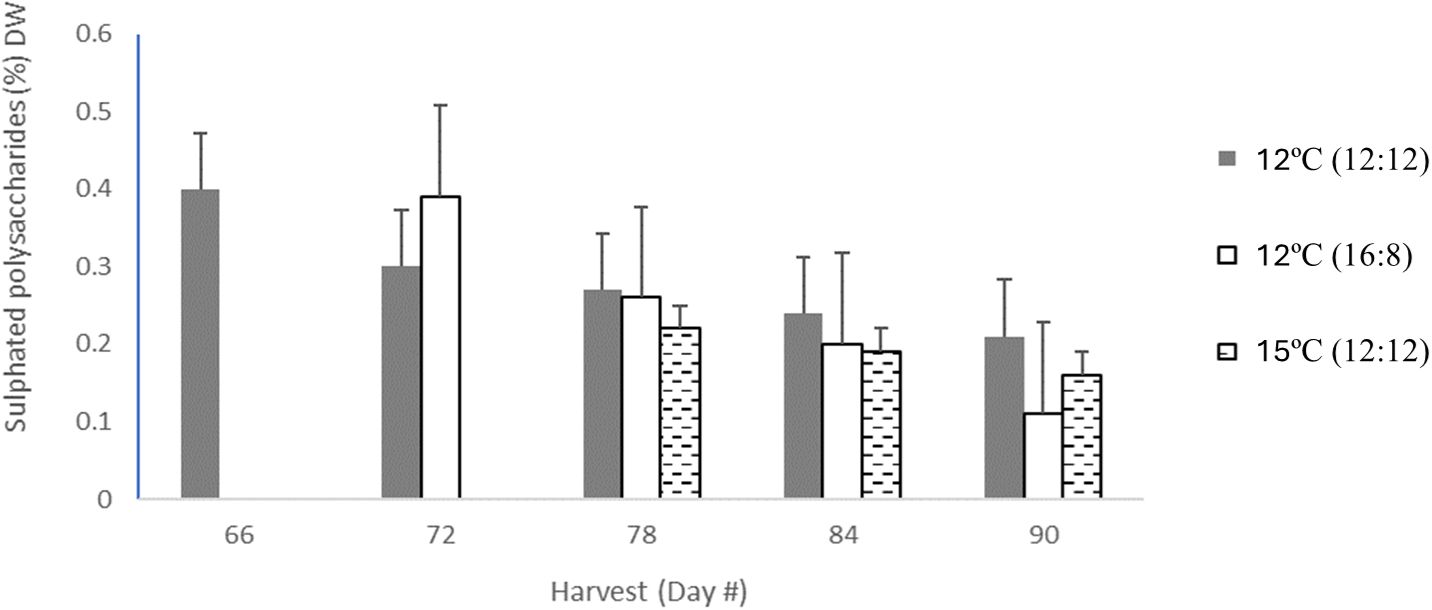
Figure 3 Sulphated polysaccharide content from all treatments of Macrocysis pyrifera in listed in units of (% DW) for each harvest which occurred between day 66 and day 90, with 3-5 harvests dependent on treatment.The sulphated polysaccharide content for 12°C (12:12) with 5 harvests was coloured in light grey bars, the 12°C (16:8) had 4 harvests coloured in dark grey in bars, 15°C (12:12) had 3 harvests in hatched black bars. Error bars are based on SD with n=3 based on 3 analytical replicates.
3.2.4 Fatty acid profiles
The total fatty acid (TFA) profiles for each of the three treatments—12°C (12:12), 12°C (16:8) and 15°C (12:12)—are listed in (Table 4) (all values listed are >0.1% TFA). The 12°C (12:12) treatment had the highest PUFAs with 60.44% of TFAs (Table 4). The top 3 most abundant fatty acids in M. pyrifera were palmitic acid, stearidonic acid (SDA) and oleic acid.
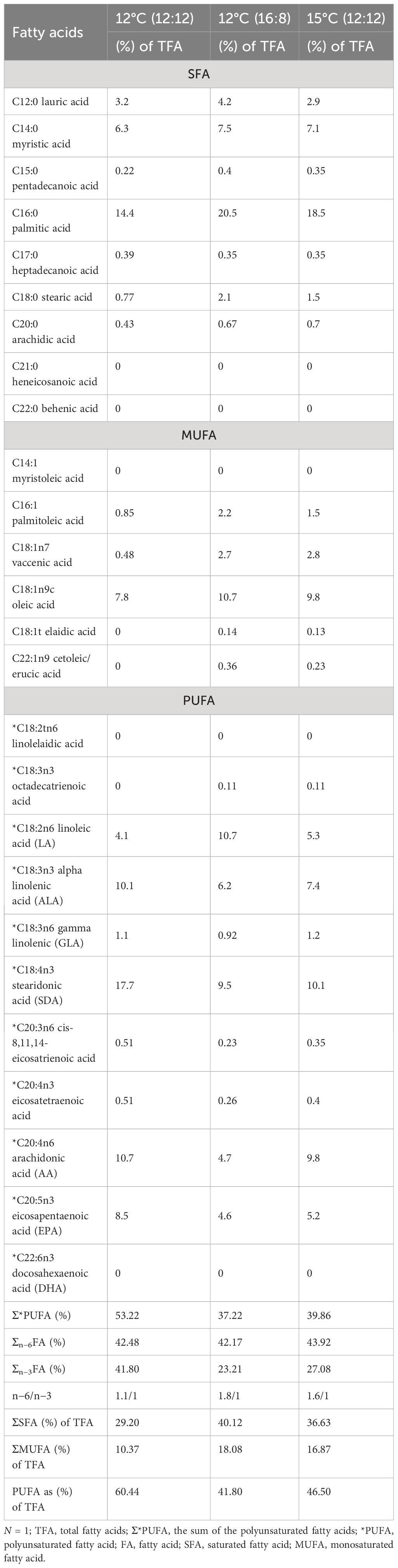
Table 4 Fatty acid profiles from all treatments of Macrocystis pyrifera during laboratory cultivation trials.
Palmitic acid (C16:0), was the highest fatty acid in this study, with values of 20.5% TFA and 18.5% TFA for the 12°C (16:18) and 15°C (12:12) treatments, respectively; however, for the 12°C (12:12) treatment, palmitic acid was the second highest fatty acid with 14.4% TFA (Table 4).
Eicosapentaenoic acid (EPA), is a (PUFA) and its highest value was 8.5% TFA for the 12°C (12:12) treatment. Interestingly, another PUFA, alpha linoleic acid (ALA), was highest for the 12°C (12:12) treatment with 10.1% TFA (Table 4). The 12°C (12:12) treatment registered the highest value of SDA was 17.7% of TFA and an important PUFA. Another PUFA with notably high values was arachidonic acid (AA), at 10.7% DW for the 12°C (12:12) treatment, 10.1% for the 15°C (12:12) treatment and 9.5% for the 12°C (16:8) treatment. The highest PUFA content for the 15°C (12:12) treatment was 10.1% for SDA, followed by AA with 9.8%.
PUFAs account for almost half of the TFAs for the 12°C (16:8) and 15°C (12:12) treatments, with values of 41.80% and 46.50%, respectively, and over half for the 12°C (12:12) treatment at 60.44%. Out of 26 fatty acids, 11 PUFAs account for an average of 49.66% of TFAs across all treatments.
The PUFA profiles include all omega-3 and omega-6 fatty acids with values >0.1% for all the harvests throughout these laboratory trials listed in (Table 5). Linoleic acid (LA) had an increasing trend over time in both the 12°C (12:12) and 12°C (16:8) treatments, with content of 3%–4% for 12°C (12:12) and a higher content of 2.4%–10.7% for 12°C (16:8), with the highest value of 10.7% TFA for LA across all treatments and harvests. The LA content for the 15°C (12:12) treatment was 4.8%, 5.6% and 5.3% from the harvests on days 78, 84 and 90, respectively. For the 12°C (12:12) treatment, ALA, following LA with an increasing content from each harvest, had the highest range of values for this PUFAs at 4.2%–10.1%, with 10.1% the highest ALA value across all treatments. The 12°C (16:8) treatment had mixed values for ALA with 4.7%, 4.3%, 9.1% and 6.2%, values increasing and decreasing through the four harvests from days 72, 78, 84 and 90 respectively. The ALA content for the 15°C (12:12) treatment was 6.3%, 6.2% and 7.4% for the three harvests on days 78, 84 and 90. The C18:3n6 gamma linolenic (GLA) content was lower than the other PUFAs, with values ranging from 1% -1.2% for all harvests of the 12°C (12:12) treatment, 0.88% to 1.1% for the 12°C (16:8) treatment from four harvests and 1.2% to 1.3% for the 15°C (12:12) treatment from three harvests (Table 5). The arachidonic acid C20:4n6 (AA) content of the 12°C (12:12) treatment increased through all five harvests from 3.6% to 10.7%, with 10.7% the highest content AA across all treatments.
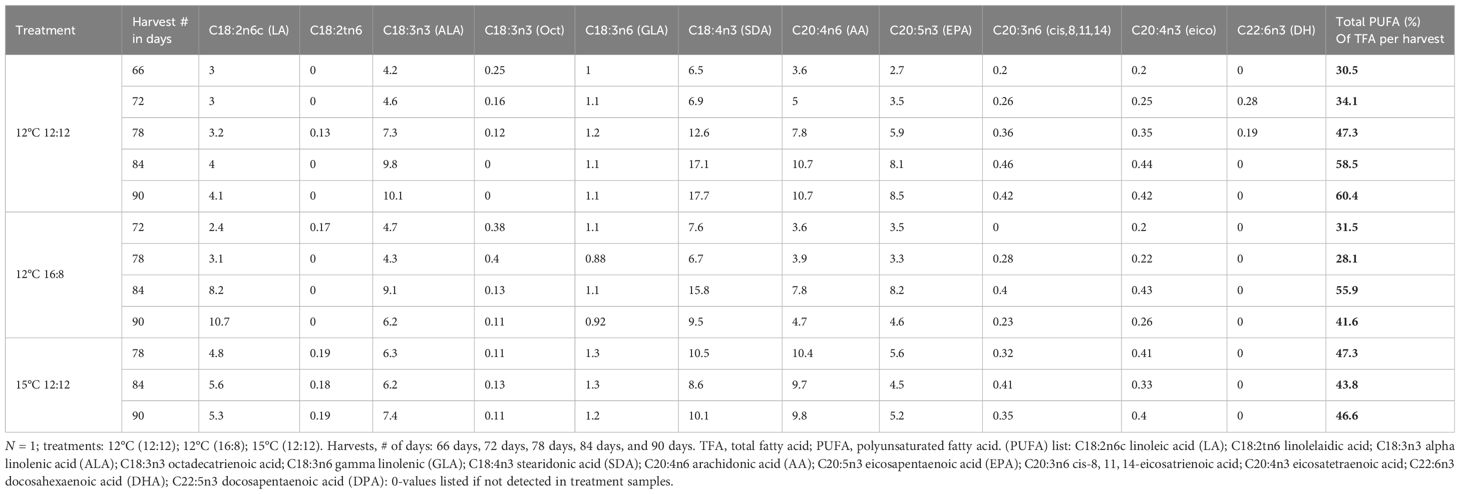
Table 5 Polyunsaturated fatty acid (PUFA) profiles for each harvest and treatment for Macrocystis pyrifera in (%) of TFA.
The 15°C (12:12) treatment had the highest content of 9.8%–10.4%, and the 12°C (16:8) treatment had contents of 3.6%, 3.9%, 7.8% and 4.7% of PUFAs from four harvests.
One of the most important PUFAs was C20:5n3 eicosapentaenoic acid (EPA) with values of 2.7%, 3.5%, 5.9%, 8.1% and 8.5% of TFAs for five harvests on days 66, 72, 78, 84 and 90 for the 12°C (12:12) treatment. The highest EPA content across all treatments was 8.5% for the last harvest on day 90. For the 12°C (16:8) treatment, the content was 3.5%, 3.3%, 8.2% and 4.6% for four harvests, while for the 15°C (12:12) treatment, the content was 5.6%, 4.5% and 5.2% for three harvests, this was a lower range when compared to the values for the 12°C (12:12) treatment.
Docosahexaenoic acid C22:6n3 (DHA) was found only in the 12°C (12:12) treatment, and the two harvest values were 0.28% and 0.19% on harvest days 72 and 78. The percentage of TFA increased from 30.5% for the harvest on day 66 to 60.4% on day 90 for 12°C (12:12), doubling the PUFA content. For the 12°C (16:8) treatment, the PUFA of TFA % decreased from 31.5% to 28.1%, then increased to 55.9%, and then dropped to 41.6%. Of the 11 PUFAs, 7 had their highest content in the 12°C (12:12) treatment, and these results may indicate that sporophyte count and size increases that occurred in this treatment may necessitate higher PUFA content. Interestingly, for the 15°C 12:12 treatment, the PUFA content for its first harvest on day 78 was 47.3%, dropped to 43.8% on the day 84 harvest, and then increased to 46.6% in the final harvest on day 90, accounting for almost half of the FA present in biomass generated from these juvenile sporophytes (Table 5). For the 12°C (16:8) treatment, the PUFA % of TFA fluctuated with values of 31.5%, 28.1%, 55.9% and 41.6% from four harvests (Table 5).
At the per harvest scale, specific differences have been noted that decoupled biomass and nutritional content, indicating that higher biomass does not necessarily ensure higher nutritional content for high-value products; for example, the highest PUFA content for the 12°C 12:12 treatment was 60.4% of TFA from the final harvest, but the biomass was 55% lower than the first harvest biomass, which had only 30.5% PUFA of TFA (Table 5).
4 Discussion
This study compared a range of cultivation conditions to mimic local seasons in Tasmania for M. pyrifera. This study aimed to identify the optimal conditions that maximize biomass accumulation and the highest nutritional composition. Of the four treatments, the highest biomass, sporophyte number, and sporophyte size for M. pyrifera were obtained from the 12°C treatment with a photoperiod of light/dark (12:12).
From the sporophyte cell densities counted in this study, the time required for maximum sporophyte density for this species cultivar was 60 days (versus 30 and 90 days), and preliminary investigations showed that the optimal light intensity for growth was 30 µmol m−2 s−1 and nutrients were supplied using F/2 nutrient media.
Similar results were found by (Camus and Buschmann 2017) using M. pyrifera sourced in Chile where temperatures were similar, but photoperiods and nutrients did differ, noting that local conditions impacted the growth of M. pyrifera more significantly than the season in Chile (Camus and Buschmann 2017). These protein values are within the 5%–20% range previously reported for brown seaweeds and are above those found in M. pyrifera from a previous study with 13.2% ± 0.30% DW protein (Tønnesen and Karlsen, 2002; MacArtain et al., 2007; Marsham et al., 2007; Qin, 2008; Taboada et al., 2013; Synytsya et al., 2015; Fertah et al., 2017; Purcell et al., 2022). Previous cultivation studies have noted that nitrogen or protein yield is indicative of biomass accumulation (Stedt et al., 2022). Brown seaweed protein is assumed to be an alternative protein source to animal protein (Horn et al., 2000; Peteiro et al., 2019) and has a protein content similar to that of egg protein (Myers et al., 2010, Myers et al., 2016). From this study, protein from M. pyrifera was 22.48% DW, for the 12°C (16:8) treatment, exceeding these previous data. This seaweed was also used as a food additive in a zebra bull diet, which contained 8.5% crude protein yet had 85% digestibility (Edwards and Watson, 2011). When fed to abalone, crude protein content was 9%–13% DW, and for a juvenile white shrimp (Litopenaeus vannamei) diet, 3.55% protein was added over 28 days and produced a protein efficiency ratio (PER) of 1.7 indicative of weight gain (Indegaard and Minsaas, 1991; Adams, 1994; Federation Food & Drink, 2013; Nelson, 2013). This compares to soy isolates with a PER of 1.0–1.7, but soy concentrates are higher between 2.0 and 2.2, but casein (from milk) is 2.50 PER (Day et al., 2022). As a potential dietary supplement, 22.48% DW protein for brown seaweed is a very positive result and comparable to another brown seaweed L. digitata with a protein content of 23.87% DW, extracted using a scalable extraction method using food-grade enzymes (Purcell et al., 2022). Laminaria digitata is one of the top 10 most cultivated brown seaweeds worldwide (Ferdouse et al., 2018), yet more importantly, both L. digitata and M. pyrifera are listed as GRAS (generally recognized as safe) by the US Food and Drug Administration (FDA) for human consumption as flavor enhancers and flavor adjuvants, with concentrations in food not exceeding current good manufacturing practice (GMP) (Østgaard et al., 1993). The inclusion of seaweed as a food supplement to increase the nutritional value of food to deliver essential amino acids appears to have potential. The protein content data from this study indicate the potential for protein bioproducts from M. pyrifera in the US market at present.
Polysaccharides are extremely important in seaweed physiology as anionic polysaccharides, i.e. alginates and fucoidans form the main components in the cell wall of brown seaweeds (Cowey, 1976; Freund-Levi et al., 2006; Smith et al., 2011; Swanson et al., 2012). Alginates are polymers of uronic acid, β-1,4-D-mannuronate and α-1,4-L-guluronate, organized into blocks within the polysaccharide chain (Araya, 2008). Seaweed polysaccharides are recognized to be partly responsible for the flexibility of seaweeds (Cardoso et al., 2014), and this characteristic infers that brown seaweeds cultivated under stormy conditions usually have superior alginate content than those in calm oceans (Afonso et al., 2019). Total polysaccharide content and the degree of sulphation of biomass were measured as indicators of the potential yield of commercial-value polysaccharide extracts. The highest total polysaccharide (TP) content from this study was 16.2% (DW), and this is well within the range of previous brown seaweed studies with values of 4%–70% (DW) (Kraan, 2012). When M. pyrifera is compared to other brown seaweeds, such as Laminaria and Saccharina with contents of 38%–61% DW for TP, Undaria sp. having similar values of 35%–45% DW for TP and Ascophylum with 42%–61% DW for TP (Kraan, 2012), the juvenile sporophytes of M. pyrifera from this study are below the reported range of TP for these brown seaweeds, as this analysis is from adult seaweeds that are in the size order of metres compared to sporophytes with sizes in millimeters. The detection of TP in the same order of magnitude indicates that sporophytes may respond positively to the increased space and nutrients that were employed for the last 30 days of cultivation, as polysaccharides are a major component of the cell walls of growing juvenile sporophytes (Kloareg and Quatrano, 1988). Sporophyte numbers did not increase significantly in the last 30 days of growth, but the sporophyte size did significantly increase from 5 to 20 times larger (Appendix III).
Fucoidans are L-fucose-containing sulphated polysaccharides found in the extracellular matrix (ECM) within the cell walls of brown seaweeds (Kloareg and Quatrano, 1988). A recent work has found that the structure of fucoidan did not have a fucose backbone, but only fucose side chains and its backbone were galactic, similar to many others in seaweeds (Hanisch, 2023). The highest degree of sulphation of polysaccharides (SPs) per harvest in this study was 0.4% DW for the 12°C 12:12 treatment for juvenile sporophytes of 0.2 ± 0.005 mm in length after 66 days of growth. If SP is expressed as a percentage of TP, the content is 4% of TP from juvenile sporophytes of M. pyrifera, which is, for the first time, to our knowledge that this type of nutritional composition has been reported for the juvenile sporophytes of M. pyrifera.
Interestingly, the SP content decreases after the first 75 days until the final harvest at 90 days; therefore, for the optimal treatment of 12°C (12:12), the best cultivation time to produce SP in this system was before 75 days at this scale. An alternative strategy is to transfer these as feedstock to seed open-ocean lines for large-scale biomass production. A previous work found a 19% degree of sulphation from its polysaccharide fraction of M. pyrifera (Schweiger, 1962), and more recently, commercially sourced powdered high-purity fucoidan sourced from M. pyrifera had 27.32% (W/W) degree of sulphation (Zhang et al., 2015). These previous degrees of sulphation data exceed the results from this study on juvenile sporophytes, as would be expected, with these comparison studies being based on adult M. pyrifera seaweed that should potentially have higher fucoidan content. Fucoidan functions as a cell wall-reinforcing molecule and appears to assist with protection against desiccation at low tide, as well as provide structural integrity enabling the seaweed to withstand adverse open-ocean conditions (Schweiger, 1962; Kraan, 2012).
The applications of SP from M. pyrifera have been noted to function as a fucoidan immune modulator enabling delays in cell apoptosis and promoting proinflammatory cytokine production (Kılınç et al., 2013). A US patent in 2012 was filed using M. pyrifera extract-derived product range, including a high-purity fucoidan 75%–90% kelp oil and/or kelp concentrate, and these products were reported to provide total antioxidant protection as pharmaceutical products (Copp and Glantz, 2011). Fucoidans from brown seaweed have exhibited bioactivity including, e.g. anti-inflammatory, antioxidant, antibacterial and immunological activity; lipid inhibition; and obesity prevention or treatment (Cumashi et al., 2007; Park et al., 2011; Fitton et al., 2015; Synytsya et al., 2015), indicating the utility and applications of polysaccharides sourced from M. pyrifera including the juvenile sporophytes from this study produced in controlled environmental conditions. The difference in the size of adults at 1–5 m (after 6 months of growth) and juvenile sporophytes at 2 ± 0.05 mm (after 3 months of lab/hatchery-phase growth) indicates a reduced surface area-to-volume ratio for the juvenile sporophytes. This disparity in size comparison may explain the difference in SP content for sporophytes at 0.4%, when compared to adult plants at 27.3%–19% for M. pyrifera (Cumashi et al., 2007; Hepburn et al., 2007). Previously, it was noted through nutritional comparisons of M. pyrifera adult and spores that the carbon/nitrogen (C/N) ratio of adult vegetative tissue fluctuated greatly with season, due mainly to nitrogen in Macrocystis, while the spore C/N ratio remained relatively constant (Reed et al., 1996). In addition, in higher plants, phenotypic stress response causes variability in all physical plant components before seeds are impacted (Harper et al., 1970; Haig and Westoby, 1988). This type of stress response to low nutrient availability in adult kelp impacts the vegetative tissue as a preference to ensure that spore quality is maintained (Reed et al., 1996).
Fatty acids from seaweeds and plants are used as a source of carbon-18 (C18) PUFA for human and fish diets as neither can produce these PUFA's themselves. They are excellent sources of n−3 fatty acids with 18 to 20+ carbons, such as C20:5n3 eicosapentaenoic acid (EPA) and C22:6n3 docosahexaenoic acid (DHA), which have been mainly sourced from fish oils yet had originally been found in seaweed (Tocher et al., 2019; Rocha et al., 2021). The highest EPA content from this study was 8.5% TFA and the DHA content was 0.5% TFA from the 12°C (12:12) treatment, and across all treatments, the total PUFA content ranged from 47% to 60% of the TFA.
At the per harvest scale, DHA was found only in the 12°C (12:12) treatment, with values of 0.28% and 0.19% TFA for harvest days 72 and 78, respectively, and these results are comparable to other seaweeds that had little or no DHA present (Takagi et al., 1985; Fleurence et al., 1994). The most abundant fatty acid in this study was palmitic acid (C16:0), with values that ranged from 14% to 20% TFA across all treatments, and this compares to a previous work, where palmitic acid represents 20%–30% of TFA in membrane phospholipids and adipose triacylglycerols (Carta et al., 2015). Palmitic acid content from this study compares favourably with a previous study on M. pyrifera sampled in Chile (Ortiz et al., 2009). Stearidonic acid (C18:4n3) was the second most abundant of the 26 fatty acids identified with values >0.1% and ranged from 9.5% to 18% of TFA across all treatments. SDA is an ω-3 fatty acid, a PUFA and is biosynthesized from alpha linolenic acid (ALA) by the enzyme delta-6-desaturase (Walker et al., 2013), and exogenous stearidonic acid is possibly a better precursor than ALA for EPA biosynthesis (Calder, 2021). C18:1n9c oleic acid accounted for ~10% of the TFA content across all treatments. Oleic acid (C18:1n9c) is an ω-9 monounsaturated fatty acid and is found throughout the human diet. LA and ALA are potentially related to the growth stages of the juvenile sporophytes (Marinho et al., 2015). Arachidonic acid (AA) is only produced by humans when they eat LA, and it is also found in cells throughout the body and supports the nervous, skeletal and immune systems (Tallima and El Ridi, 2017).
The World Health Organisation (WHO) food standards for omega-3 recommend a daily dose of EPA and DHA: 0.3 g to 0.5 g for adults (US Department of Human & Human Services, 2020). The health benefits of these two n−3 fatty acids (EPA and DHA) are extensive, including proper foetal and brain development and neuronal, retinal and immune function (Freund-Levi et al., 2006; Swanson et al., 2012). The other potential uses are the prevention of mild Alzheimer’s disease, obesity and sarcopenic obesity for the elderly (Freund-Levi et al., 2006; Troesch et al., 2020). The highest PUFA in this study was SDA at 17.7% of TFA, for the 12°C (12:12) treatment. SDA is an exciting FA for human nutrition as it has greater efficacy in increasing EPA content in tissues including erythrocytes and plasma lipids (James et al., 2003). A previous work noted that the highest PUFA was linoleic acid (18:2n−6) at 43.41% for M. pyrifera, and the highest monounsaturated fatty acid (MUFA) was 18:1n−9c (oleic acid) at 19.64% (Ortiz et al., 2009). The contributary factors that may account for the differences in PUFA profile composition between M. pyrifera samples may be due to the use of growing juvenile sporophytes in this study in contrast to the dried biomass used in the study from Chile (Ortiz et al., 2009).
One of the benefits of M. pyrifera is that it contains a suitable PUFA n−6/n−3 ratio that can positively impact both human and animal nutrition (Ortiz et al., 2009). The results from this study showed an n−6/n−3 ratio ranging from 1.1/1 to 1.8/1 for M. pyrifera across all treatments, and these results compared favorably with a previous brown seaweed study that also noted a 0.8/1 ratio, indicating that n–6 FA was comparably low, which infers a health benefit and has a similar ratio to that of cold-water fishes. Traditional European foods have an n−6/n−3 ratio of approximately 15–17:1, suggesting that Western diets are deficient in n−3 FA and high in n−6 FA, with the European Nutritional Societies recommending an n−6/n−3 ratio of 5:1 as health-promoting for humans (Dawczynski et al., 2007; Calder, 2021). To counteract this n−3 FA dietary deficiency, this 1.1/1 ratio for M. pyrifera indicates that seaweed products can assist in the supply of n−3 FA to the human diet. Foods high in n−3 long chain (LC) (>20 carbons), LC-PUFA, are considered to have a positive effect on the composition of blood lipids and, therefore, can potentially assist in the prevention of arteriosclerosis (Murata et al., 2002; Nestel et al., 2002; Erkkilä et al., 2003). Brown seaweeds represent a vital source of LC-PUFA (n−3; n−6), essential for the development of structural lipids and components of cell membranes, as well as precursors of eicosanoids, which may impact inflammation processes and immune responses (De Pablo and De Cienfuegos, 2000; Calder and Grimble, 2002; Calder, 2021). This study has noted that as the growth of these juvenile sporophytes continues, PUFA content increases, indicating that harvesting at later stages of growth may benefit the accumulation of PUFA, but not the overall biomass accumulation.
This controlled study of M. pyrifera juvenile sporophyte development indicates that environmental cultivation conditions affect the production of biomass and nutrient composition (protein, PUFA, TP and SP) for potential bioproduct production. The results show that the least stressed developing tissue, as indicated by the number and mass, contained the highest levels of potential valuable metabolites. The highest level of seaweed biomass, the number of juvenile sporophytes and the highest total yield for protein, PUFA, TP and SP were from the 12°C (12:12) treatment, with 30 µmol m−2 s−1 light and F/2 nutrient media after 60 days of cultivation. Harvesting time was also important for juvenile sporophytes, which were observed to increase their PUFA content with increased growth, indicating that harvesting at a later stage may benefit the accumulation of higher levels of PUFA, but not the overall biomass accumulation. Conversely, the SP content decreased as the harvests continued, so to achieve the highest SP content, harvesting earlier at 45 days has been recommended for juvenile sporophytes at the hatchery scale (Camus and Buschmann 2017). Protein levels peaked at 22.48% DW in the final harvest indicating more growth time may be beneficial for higher protein content with present levels, comparable to other commercially cultivated brown seaweeds.
The implication of this study is that temperature and photoperiod are factors that impact the sporophyte growth rate, size, biomass and nutrient accumulation. This study has enabled new knowledge on the crucial local environmental conditions to be identified that influence the cultivation and productivity of sporophytes from native Tasmanian cultivars. This work enables progress to be made towards exploring productive aquaculture from hatchery-cultivated M. pyrifera in Tasmania.
Data availability statement
The original contributions presented in the study are included in the article/Supplementary Material. Further inquiries can be directed to the corresponding author.
Author contributions
DP: Investigation, Conceptualization, Data curation, Funding acquisition, Methodology, Validation, Writing – review & editing, Writing – original draft. TW: Writing – review & editing. MH: Conceptualization, Methodology, Writing – review & editing. MP: Writing – original draft, Writing – review & editing.
Funding
The author(s) declare financial support was received for the research, authorship, and/or publication of this article. DP has received funding from the Teagasc Research Leaders 2025 programme co-funded by Teagasc and the European Union’s Horizon 2020 research and innovation programme under the Marie Skłodowska-Curie grant agreement number 754380.
Acknowledgments
We thank Josh and the analytical chemistry team at the Cawthron Institute for their assistance with the sample analysis and Wouter Visch and Catriona L. Hurd for the support and provision of laboratory facilities for the experiments at UTAS during COVID and for the assistance in manuscript editing and structure.
Conflict of interest
Author MP was employed by the company Algavive Limited.
The remaining authors declare that the research was conducted in the absence of any commercial or financial relationships that could be construed as a potential conflict of interest.
Publisher’s note
All claims expressed in this article are solely those of the authors and do not necessarily represent those of their affiliated organizations, or those of the publisher, the editors and the reviewers. Any product that may be evaluated in this article, or claim that may be made by its manufacturer, is not guaranteed or endorsed by the publisher.
Supplementary material
The Supplementary Material for this article can be found online at: https://www.frontiersin.org/articles/10.3389/fmars.2024.1410877/full#supplementary-material
References
Adams N. M. (1994). Seaweeds of New Zealand: an illustrated guide/Nancy M. Adams (Christchurch, N.Z: Canterbury University Press).
Afonso N. C., Catarino M. D., Silva A. M. S., Cardoso S. M. (2019). Brown macroalgae as valuable food ingredients. Antioxidants 8, 365. doi: 10.3390/antiox8090365
Agardh C. A. (1820 ‘1821’). Species algarum rite cognitae, cum synonymis, differentiis specificis et descriptionibus succinctis (Lundae [Lund]: ex officina Berlingiana), [i[i-iv], [1]–168. Available at: https://www.algaebase.org/search/species/detail/?species_id=4427.
AOAC. (1995). Official Methods of Analysis of Association of Official Analytical Chemists International. 16th ed (Gaithersburg, MD (USA: AOCA), 870.
Araya J. (2008). Riesgos y beneficios del onsume de grasas y aceites (Chile: En Programa de Educación a Distancia Departamento de Nutrición Facultad de Medicina Universidad de Chile).
Arnold K. E., Manley S. L. (1985). Carbon, Allocation in Macrocystis pyrifera (Phaeophyta): Intrinsic variability in photosynthesis and respiration. J. Phycology. 21, 154–167. doi: 10.1111/j.0022-3646.1985.00154.x
Avila-Peltroche J., Padilla-Vallejos J. (2020). The seaweed resources of Peru. Botanica Marina. 63, 381–394. doi: 10.1515/bot-2020-0026
Bartsch I. (2018). Derivation of clonal stock cultures and hybridization of kelps (Protocols for Macroalgae Research: CRC Press Taylor and Francis Group), 61–78.
Bikker P., Stokvis L., van Krimpen M. M., van Wikselaar P. G., Cone J. W. (2020). Evaluation of seaweeds from marine waters in Northwestern Europe for application in animal nutrition. Anim. Feed Sci. Technol. 263, 114460. doi: 10.1016/j.anifeedsci.2020.114460
Bixler H. J., Porse H. (2011). A decade of change in the seaweed hydrocolloids industry. J. Appl. Phycology. 23, 321–335. doi: 10.1007/s10811-010-9529-3
Bolton J. J., Anderson R. J., Smit A. J., Rothman M. D. (2012). South African kelp moving eastwards: the discovery of Ecklonia maxima (Osbeck) Papenfuss at De Hoop Nature Reserve on the south coast of South Africa. Afr. J. Mar. Sci. 34, 147–151. doi: 10.2989/1814232X.2012.675125
Cai J., Lovatelli A., Aguilar-Manjarrez J., Cornish L., Dabbadie L., Desrochers A., et al. (2021). Seaweeds and microalgae: an overview for unlocking their potential in global aquaculture development (Rome, Italy: FAO).
Calder P. C. (2021). “Chapter 2 – Health benefits of omega-3 fatty acids,” in Omega-3 Delivery Systems. Eds. García-Moreno P. J., Jacobsen C., Moltke Sørensen A.-D., Yesiltas B. (London; UK: Academic Press), 25–53.
Calder P., Grimble R. (2002). Polyunsaturated fatty acids, inflammation and immunity. Eur. J. Clin. Nutr. 56, S14–S19. doi: 10.1038/sj.ejcn.1601478
Camus C., Buschmann A. H. (2017). Macrocystis pyrifera aquafarming: Production optimization of rope-seeded juvenile sporophytes. Aquaculture 468, 107–114. doi: 10.1016/j.aquaculture.2016.10.010
Cardoso M. S. G., Carvalho L J., Silva P. S., Rodrigues M. R., Pereira O., Pereira L. (2014). Bioproducts from seaweeds: a review with special focus on the Iberian Peninsula. Curr. Organic Chem. 18, 896–917. doi: 10.2174/138527281807140515154116
Carta G., Murru E., Lisai S., Sirigu A., Piras A., Collu M., et al. (2015). Dietary triacylglycerols with palmitic acid in the sn-2 position modulate levels of N-acylethanolamides in rat tissues. PloS One 10, e0120424. doi: 10.1371/journal.pone.0120424
Copp A., Glantz D. (2011). Macrocystis pyrifera Derived Health and Wellness Products and Methods of Using the Same. Patent No. WO2011/044267 Al. (United States: United States Patents).
Correa T., Gutiérrez A., Flores R., Buschmann A. H., Cornejo P., Bucarey C. (2016). Production and economic assessment of giant kelp Macrocystis pyrifera cultivation for abalone feed in the south of Chile. Aquaculture Res. 47, 698–707. doi: 10.1111/are.2016.47.issue-3
Cowey C. (1976). Use of synthetic diets and biochemical criteria in the assessment of nutrient requirements of fish. J. Fisheries Board Canada. 33, 1040–1045. doi: 10.1139/f76-132
Cumashi A., Ushakova N. A., Preobrazhenskaya M. E., D’Incecco A., Piccoli A., Totani L., et al. (2007). A comparative study of the anti-inflammatory, anticoagulant, antiangiogenic, and antiadhesive activities of nine different fucoidans from brown seaweeds. Glycobiology 17, 541–552. doi: 10.1093/glycob/cwm014
Dawczynski C., Schubert R., Jahreis G. (2007). Amino acids, fatty acids, and dietary fibre in edible seaweed products. Food Chem. 103, 891–899. doi: 10.1016/j.foodchem.2006.09.041
Day L., Cakebread J. A., Loveday S. M. (2022). Food proteins from animals and plants: Differences in the nutritional and functional properties. Trends Food Sci. Technol. 119, 428–442. doi: 10.1016/j.tifs.2021.12.020
De Pablo M. A., De Cienfuegos G. Á. (2000). Modulatory effects of dietary lipids on immune system functions. Immunol. Cell Biol. 78, 31–39. doi: 10.1046/j.1440-1711.2000.00875.x
Duong M. L., Desmond M. J., Buschmann A. H., Pritchard D. W., Camus C., Hurd C. L., et al. (2022). Reproduction, hatchery and culture applications for the giant kelp (Macrocystis pyrifera): a methodological appraisal. Appl. Phycology 3, 382. doi: 10.1080/26388081.2022.2086823
Edwards M., Watson L. (2011). Aquaculture explained no. 26: cultivating Laminaria digitata (Dun Laoghaire, Ireland: Irish Sea Fisheries Board).
Erkkilä A. T., Lehto S., Pyörälä K., Uusitupa M. I. (2003). n–3 fatty acids and 5-y risks of death and cardiovascular disease events in patients with coronary artery disease. Am. J. Clin. Nutr. 78, 65–71. doi: 10.1093/ajcn/78.1.65
FAO. The State of World Fisheries and Aquaculture (2016). Contributing to food security and nutrition for all (Rome: Food and Agriculture Organization of the United Nations (FAO)), 200.
Ferdouse F., Holdt S. L., Smith R., Murúa P., Yang Z. (2018). The global status of seaweed production, trade and utilization. Globefish Res. Programme 124, I.
Fertah M., Ahmed B., Moha T., François B. (2017). Extraction and characterization of sodium alginate from moroccan laminaria digitata brown seaweed. Arabian J. Chem. 10, S3707–S3714.
Fitton J. H., Stringer D. N., Karpiniec S. S. (2015). Therapies from fucoidan: An update. Mar. Drugs 13, 5920–5946. doi: 10.3390/md13095920
Fleurence J., Gutbier G., Mabeau S., Leray C. (1994). Fatty acids from 11 marine macroalgae of the French Brittany coast. J. Appl. Phycology. 6, 527–532. doi: 10.1007/BF02182406
Forbord S., Steinhovden K. B., Rød K. K., Handå A., Skjermo J. (2018). Cultivation protocol for Saccharina latissima. Protoc. macroalgae Res. 2, 4.1. doi: 10.1201/B21460-2
Freund-Levi Y., Eriksdotter-Jönhagen M., Cederholm T., Basun H., Faxen-Irving G., Garlind A., et al. (2006). ω-3 fatty acid treatment in 174 patients with mild to moderate Alzheimer disease: OmegAD study: a randomized double-blind trial. Arch. Neurol. 63, 1402–1408. doi: 10.1001/archneur.63.10.1402
Graham M. H., Vásquez J. A., Buschmann A. H. (2007). Global ecology of the giant kelp Macrocystis: From ecotypes to ecosystems. Oceanography Mar. Biology: Annu. Rev. 45, 39–88.
Guillard R. R., Ryther J. H. (1962). Studies of marine planktonic diatoms. I. Cyclotella nana Hustedt, and Detonula confervacea (cleve) Gran. Can. J. Microbiol. 8, 229–239. doi: 10.1139/m62-029
Hafting J. T., Craigie J. S., Stengel D. B., Loureiro R. R., Buschmann A. H., Yarish C., et al. (2015). Prospects and challenges for industrial production of seaweed bioactives. J. Phycology. 51, 821–837. doi: 10.1111/jpy.12326
Haig D., Westoby M. (1988). Inclusive fitness, seed resources, and maternal care. Plant Reprod. ecology. 1988, 60–79.
Hanisch F. G. (2023). Revised structure model of norovirus-binding fucoidan from Undaria pinnatifida: oligofucose chains branch off from a β6-galactane. Glycobiology 33, 556–566. doi: 10.1093/glycob/cwad039
Harper J. L., Lovell P., Moore K. (1970). The shapes and sizes of seeds. Annu. Rev. Ecol. systematics 1, 327–356. doi: 10.1146/annurev.es.01.110170.001551
Hay C. H. (1990). The distribution of Macrocystis (Phaeophyta: Laminariales) as a biological indicator of cool sea surface temperature, with special reference to New Zealand waters. J. R. Soc. New Zealand. 20, 313–336. doi: 10.1080/03036758.1990.10426716
Hepburn C. D., Holborow J. D., Wing S. R., Frew R. D., Hurd C. L. (2007). Exposure to waves enhances the growth rate and nitrogen status of the giant kelp Macrocystis pyrifera. Mar. Ecol. Prog. Ser. 339, 99–108. doi: 10.3354/meps339099
Holdt S. L., Kraan S. (2011). Bioactive compounds in seaweed: functional food applications and legislation. J. Appl. Phycol 23, 543–597. doi: 10.1007/s10811-010-9632-5
Horn S. J., Aasen I. M., Østgaard K. (2000). Ethanol production from seaweed extract. J. Ind. Microbiol. Biotechnol. 25, 249–254. doi: 10.1038/sj.jim.7000065
Indegaard M., Minsaas J. (1991). “Seaweed resources in europe. Uses and potential,” in Animal and Human Nutrition. Eds. Guiry M. D., Blunden G. (John Wiley & Sons Ltd., Chichester), 21–64.
James M. J., Ursin V. M., Cleland L. G. (2003). Metabolism of stearidonic acid in human subjects: comparison with the metabolism of other n-3 fatty acids. Am. J. Clin. Nutr. 77, 1140–1145. doi: 10.1093/ajcn/77.5.1140
Kılınç B., Cirik S., Turan G., Tekogul H., Koru E. (2013). Seaweeds for Food and Industrial Applications, Food Industry (Innocenzo, Muzzalupo: IntechOpen). doi: 10.5772/55834
Kloareg B., Quatrano R. (1988). Structure of the cell walls of marine algae and ecophysiological functions of the matrix polysaccharides. Oceanography Mar. biology: an Annu. review. 26, 259–315.
Kopczak C. D., Zimmerman R. C., Kremer J. N. (1991). Variation in Nitrogen physiology and growth among geographically isolated populations of the giant kelp, Macrocystis pyrifera (Phaeophyta). J. Phycology 27, 149–158. doi: 10.1111/j.0022-3646.1991.00149.x
Kraan S. (2012). “Algal polysaccharides, novel applications and outlook,” in Carbohydrates-comprehensive studies on glycobiology and glycotechnology (London; UK: IntechOpen).
Krumhansl K. A., Okamoto D. K., Rassweiler A., Novak M., Bolton J. J., Cavanaugh K. C. (2016). Global patterns of kelp forest change over the past half-century. Proc. Natl. Acad. Sciences.National Acad. Sci. 113, 13785. doi: 10.1073/pnas.1606102113
Mabin C. J. T., Johnson C. R., Wright J. T. (2019). Physiological response to temperature, light, and nitrates in the giant kelp Macrocystis pyrifera from Tasmania, Australia. Mar. Ecol. Prog. Series. 614, 1–19. doi: 10.3354/meps12900
MacArtain P., Gill C. I., Brooks M., Campbell R., Rowland I. R. (2007). Nutritional value of edible seaweeds. Nutr. Rev. 65, 535–543. doi: 10.1111/j.1753-4887.2007.tb00278.x
Manns D., Deutschle A. L., Saake B., Meyer A. S. (2014). Methodology for quantitative determination of the carbohydrate composition of brown seaweeds (Laminariaceae). RSC Advances. 4, 25736–25746. doi: 10.1039/C4RA03537B
Marinho G. S., Holdt S. L., Jacobsen C., Angelidaki I. (2015). Lipids and composition of fatty acids of saccharina latissimi cultivated year-round in integrated multi-trophic aquaculture. Mar. Drugs 13, 4357–4374. doi: 10.3390/md13074357
Marsham S., Scott G. W., Tobin M. L. (2007). Comparison of nutritive chemistry of a range of temperate seaweeds. Food Chem. 100, 1331–1336. doi: 10.1016/j.foodchem.2005.11.029
McHugh D. J. (2003). A guide to the seaweed industry Rome; 2003. Technical Paper 441 (Rome, Italy: FAO FISHERIES).
Méndez F., Rivero A., Bahamonde F., Gallardo P, Frangopulos M, Zolezzi J, et al. (2024). Fatty acid composition of different morphological structures in the sub-Antarctic kelps Macrocystis pyrifera (L.) C. Agardh and Lessonia flavicans Bory of the Magellan Ecoregion: Nutritional and biomedical potentials. J. Appl. Phycol. 36, 831–844. doi: 10.1007/s10811–023-03114–9
Morrissey J., Kraan S., Guiry M. D. (2001). A Guide to Commercially Important Seaweeds on the Irish Coast (Dublin, Ireland: Irish Sea Fisheries Board), 67.
Murata M., Sano Y., Ishihara K., Uchida M. (2002). Dietary fish oil and Undaria pinnatifida (wakame) synergistically decrease rat serum and liver triacylglycerol. J. Nutr. 132, 742–747. doi: 10.1093/jn/132.4.742
Myers S. P., Mulder A. M., Baker D. G., Robinson S. R., Rolfe M. I., Brooks L., et al. (2016). Effects of fucoidan from Fucus vesiculosus in reducing symptoms of osteoarthritis: a randomized placebo-controlled trial. Biologics 10, 81–88. doi: 10.2147/BTT.S95165
Myers S. P., O’Connor J., Fitton J. H., Brooks L., Rolfe M., Connellan P., et al. (2010). A combined phase I and II open label study on the effects of a seaweed extract nutrient complex on osteoarthritis. Biologics: Targets Ther. 4, 33–44. doi: 10.2147/btt.s8354
Nestel P., Shige H., Pomeroy S., Cehun M., Abbey M., Raederstorff D. (2002). The n–3 fatty acids eicosapentaenoic acid and docosahexaenoic acid increase systemic arterial compliance in humans. Am. J. Clin. Nutr. 76, 326–330. doi: 10.1093/ajcn/76.2.326
Neushul M. (1986). Marine Farming: macroalgal production and genetics (Chicago, Illinois, USA: GRI 87/0070. Gas Research Institute).
Ortiz J., Uquiche E., Robert P., Romero N., Quitral V., Llantén C. (2009). Functional and nutritional value of the Chilean seaweeds Codium fragile, Gracilaria Chilensis and Macrocystis pyrifera. Eur. J. Lipid Sci. Technology. 111, 320–327. doi: 10.1002/ejlt.200800140
Østgaard K., Indergaard M., Markussen S., Knutsen S. H., Jensen A. (1993). Carbohydrate degradation and methane production during fermentation of Laminaria saccharina (Laminariales, Phaeophyceae). J. Appl. Phycology 5, 333–342. doi: 10.1007/BF02186236
Park M.-K., Jung U., Roh C. (2011). Fucoidan from marine brown algae inhibits lipid accumulation. Mar. Drugs 9, 1359–1367. doi: 10.3390/md9081359
Perissinotto R., McQuaid C. D. (1992). Deep occurrence of the giant kelp Macrocystis laevis in the Southern Ocean. Mar. Ecol. Prog. Ser. Oldendorf 81, 89–95. doi: 10.3354/meps081089
Peteiro C., Bidegain G., Sánchez N. (2019). Experimental evaluation of the effect of water velocity on the development of string-attached kelp seedlings (Laminariales) with implications for hatchery and nursery production. Algal Res. 44, 101678. doi: 10.1016/j.algal.2019.101678
Purcell D., Packer M. A., Hayes M. (2022). Angiotensin-I-Converting enzyme inhibitory activity of protein hydrolysates generated from the macroalga laminaria digitata (Hudson) JV lamouroux 1813. Foods 11, 1792. doi: 10.3390/foods11121792
Purcell-Meyerink D., Packer M. A., Wheeler T. T., Hayes M. (2021). Aquaculture production of the brown seaweeds Laminaria digitata and Macrocystis pyrifera: applications in food and pharmaceuticals. Molecules 26, 1306. doi: 10.3390/molecules26051306
Qin Y. (2008). Alginate fibres: an overview of the production processes and applications in wound management. Polym. Int. 57, 171–180. doi: 10.1002/pi.2296
Rajauria G. (2015). “Chapter 15 - seaweeds: a sustainable feed source for livestock and aquaculture,” in Seaweed sustainability. Eds. Tiwari B. K., Troy D. J. (U.K: Academic Press), 89–420. doi: 10.1016/B978-0-12-418697-2.00015-5
Reed D. C., Ebeling A. W., Anderson T. W., Anghera M. (1996). Differential reproductive responses to fluctuating resources in two seaweeds with different reproductive strategies. Ecology 77, 300–316. doi: 10.2307/2265679
Rocha C. A., Pacheco D. A., Cotas J. A., Marques J. C., Pereira L. A., Gonçalves A. A. (2021). Seaweeds as valuable sources of essential fatty acids for human nutrition. Int. J. Environ. Res. Public Health 8, 4968. doi: 10.3390/ijerph18094968
Schiel D. R., Foster M. S. (2015). The Biology and Ecology of Giant Kelp Forests (Oakland California, USA: University of California Press), 395. doi: 10.1525/california/9780520278868.001.0001
Schiener P., Black K. D., Stanley M. S., Green D. H. (2015). The seasonal variation in the chemical composition of the kelp species Laminaria digitata, Laminaria hyperborea, Saccharina latissima and Alaria esculenta. J. Appl. Phycol 27, 363–373. doi: 10.1007/s10811-014-0327-1
Schmid M., Kraft L. G. K., Loos L. M.v. d., Kraft G. T., Virtue P., Nichols P. D., et al. (2018). Southern australian seaweeds: A promising resource for omega-3 fatty acids. Food Chem. 265, 70–77. doi: 10.1016/j.foodchem.2018.05.060
Schweiger R. G. (1962). Methanolysis of fucoidan. I. Preparation of methyl α-L-fucoside and L-fucose1. J. Organic Chem. 27, 4267–4269. doi: 10.1021/jo01059a033
Smith G. I., Atherton P., Reeds D. N., Mohammed B. S., Rankin D., Rennie M. J., et al. (2011). Dietary omega-3 fatty acid supplementation increases the rate of muscle protein synthesis in older adults: a randomized controlled trial. Am. J. Clin. Nutr. 93, 402–412. doi: 10.3945/ajcn.110.005611
Stedt K., Trigo J. P., Steinhagen S., Nylund G. M., Forghani B., Pavia H., et al. (2022). Cultivation of seaweeds in food production process waters: Evaluation of growth and crude protein content. Algal Res. 63, 102647. doi: 10.1016/j.algal.2022.102647
Swanson D., Block R., Mousa S. A. (2012). Omega-3 fatty acids EPA and DHA: health benefits throughout life. Adv. Nutr. 3, 1–7. doi: 10.3945/an.111.000893
Synytsya A., Čopíková J., Kim W. J., Park Y. I. (2015). “Cell wall polysaccharides of marine algae,” in Springer handbook of marine biotechnology. Ed. Kim S. K. (Springer, Berlin, Heidelberg), 543–590.
Taboada C., Millan R., Miguez I. (2013). Evaluation of marine algae undaria pinnatifida and porphyra purpurea as a food supplement: composition, nutritional value and effect of intake on intestinal, hepatic and renal enzyme activities in rats. J. Sci. Food Agric. 93, 1863–1868. doi: 10.1002/jsfa.5981
Tait L. W., Thoral F., Pinkerton M. H., Thomsen M. S., Schiel D. R. (2021). Loss of giant kelp, Macrocystis pyrifera, driven by marine heatwaves and exacerbated by poor water clarity in New Zealand. Front. Mar. Sci. 8. doi: 10.3389/fmars.2021.721087
Takagi T., Asahi M., Itabashi Y. (1985). Fatty acid composition of twelve algae from Japanese waters. J. Japan Oil Chemists’ Society. 34, 1008–1012. doi: 10.5650/jos1956.34.1008
Tallima H., El Ridi R. (2017). Arachidonic acid: Physiological roles and potential health benefits – A review. J. Adv. Res. 11, 33–41. doi: 10.1016/j.jare.2017.11.004
Tocher D. R., Betancor M. B., Sprague M. A.-O., Olsen R. E., Napier J. A. (2019). Omega-3 long-chain polyunsaturated fatty acids, EPA and DHA: bridging the gap between supply and demand. Nutrients 11, 89. doi: 10.3390/nu11010089
Tønnesen H. H., Karlsen J. (2002). Alginate in drug delivery systems. Drug Dev. Ind. Pharm. 28 (6), 621–630. doi: 10.1081/DDC-120003853
Troesch B. A., Eggersdorfer M., Laviano A. A., Rolland Y., Smith A. D., Warnke I. A., et al. (2020). Expert opinion on benefits of long-chain omega-3 fatty acids (DHA and EPA) in aging and clinical nutrition. Nutrients 12, 2555. doi: 10.3390/nu12092555
Umanzor S., Ramírez-García M. M., Sandoval-Gil J. M., Zertuche-González J. A., Yarish C. (2020). Photoacclimation and photoprotection of juvenile sporophytes of macrocystis pyrifera (Laminariales, phaeophyceae) under high-light conditions during short-term shallow-water cultivation1. J. Phycol. 56, 380–392. doi: 10.1111/jpy.12951
US Department of human & human Services. (2020). Omega-3 Fatty acids. Available online at: https://ods.od.nih.gov/factsheets/Omega3FattyAcids-HealthProfessional/ (Accessed 8/11/2022).
Vásquez J. A. (2009). “Production, use and fate of Chilean brown seaweeds: re-sources for a sustainable fishery,” in Nineteenth International Seaweed Symposium: Proceedings of the 19th International Seaweed Symposium, held in Kobe, Japan, 26–31 March 2007. Eds. Borowitzka M. A., Critchley A. T., Kraan S., Peters A., Sjøtun K., Notoya M. (Springer Netherlands, Dordrecht), 7–17.
Vásquez J. A., Piaget N., Vega J. M. A. (2012). The Lessonia nigrescens fishery in northern Chile: “how you harvest is more important than how much you harvest”. J. Appl. Phycology 24, 417–426. doi: 10.1007/s10811-012-9794-4
Visch W., Layton C., Hurd C. L., Macleod C., Wright J. T. (2023a). A strategic review and research roadmap for offshore seaweed aquaculture—A case study from southern Australia. Aquaculture 5, 1467–1479. doi: 10.1111/raq.12788
Visch W., Lush H., Schwoerbel J., Hurd C. L. (2023b). Nursery optimization for kelp aquaculture in the Southern Hemisphere: the interactive effects of temperature and light on growth and contaminants. Appl. Phycology 4, 44–53. doi: 10.1080/26388081.2023.2174903
Walker C. G., Jebb S. A., Calder P. C. (2013). Stearidonic acid as a supplemental source of ω-3 polyunsaturated fatty acids to enhance status for improved human health. Nutrition 29, 363–369. doi: 10.1016/j.nut.2012.06.003
Westermeier R., Patiño D. J., Murúa P., Müller D. G. (2011). Macrocystis mariculture in Chile: growth performance of heterosis genotype constructs under field conditions. J. Appl. Phycology 23, 819–825. doi: 10.1007/s10811-010-9581-z
Zayed A., El-Aasr M., Ibrahim A.-R. S., Ulber R. (2020). Fucoidan characterization: determination of purity and physicochemical and chemical properties. Mar. Drugs 18, 571. doi: 10.3390/md18110571
Keywords: seaweed, cultivation, juvenile, sporophytes, bioproduct, nutrient, composition, protein
Citation: Purcell D, Wheeler TT, Hayes M and Packer MA (2024) Effect of photoperiod and temperature on bioproduct production from juvenile sporophytes of Macrocystis pyrifera. Front. Mar. Sci. 11:1410877. doi: 10.3389/fmars.2024.1410877
Received: 02 April 2024; Accepted: 08 May 2024;
Published: 07 June 2024.
Edited by:
Saravana Periaswamy Sivagnanam, Cork Institute of Technology, IrelandReviewed by:
Yueying Li, University of Oklahoma, United StatesTeresa Margarida Mouga, Polytechnic Institute of Leiria, Portugal
Copyright © 2024 Purcell, Wheeler, Hayes and Packer. This is an open-access article distributed under the terms of the Creative Commons Attribution License (CC BY). The use, distribution or reproduction in other forums is permitted, provided the original author(s) and the copyright owner(s) are credited and that the original publication in this journal is cited, in accordance with accepted academic practice. No use, distribution or reproduction is permitted which does not comply with these terms.
*Correspondence: Diane Purcell, ZGlhbmVwdXJjZWxsNjUxQGdtYWlsLmNvbQ==