- Coastal Marine Field Station, School of Science, University of Waikato, Tauranga, New Zealand
This study set out to provide a preliminary assessment of nutritional profiles of five selected New Zealand macroalgae to investigate the potential of land-cultivated Ulva spp. The New Zealand seaweeds species, selected from a limited range, were either collected from the wild (Pyropia plicata from Tauranga and Kaikōura), or were sourced from commercial wild harvest suppliers (Macrocystis pyrifera and Undaria pinnatifida), and were compared to land-based cultivated Ulva species (Ulva ralfsii and Ulva stenophylloides). Species were assessed for their content of crude protein, total lipids, carbohydrates, fibers, amino acids, mineral profiles, heavy metals and antioxidant capacity (Oxygen Radical Absorbance Capacity, ORAC). The two cultivated Ulva spp. had the highest crude protein content of the six seaweeds analyzed (approximately 21% dw, N×6.25), as expected with nutrient supplemented cultivation. They also had the highest total, essential and branched chain amino acid quantities (151, 70, and 29 mg/g for U. stenophylloides and 138, 62, and 27 mg/g for U. ralfsii). Though the two Ulva spp. were grown to the same cultivation specifications, they varied in carbohydrate and total fiber content (U. stenophylloides: 63% and 62%; U. ralfsii: 39% and 40%, respectively). Pyropia plicata collected in Kaikōura had the highest carbohydrate levels (65%) though not the highest total fiber. Both P. plicata had the highest trace minerals (417 mg/kg and 720 mg/kg for Kaikōura and Tauranga P. plicata respectively). All seaweeds analyzed showed no lipophilic antioxidants, though the two Phaeophyceae had the highest hydrophilic antioxidant content at 115 TE and 168 TE for Macrocystis pyrifera and Undaria pinnatifida, respectively. This paper provides a preliminary indication of the relative nutritional attributes of a range of potential New Zealand seaweed aquaculture targets benchmarked against sea-lettuce (Ulva spp.).
Introduction
Quantitative analytical investigation into seaweeds for human nutrition began in the 1960s (Iwasaki, 1967) and has since demonstrated that the nutritional value, including crude protein, varies amongst species, with correlations across lifecycle stage, location, season and whether cultivated or wild harvested (Fleurence, 1999a; Galland-Irmouli et al., 1999; Hou et al., 2015; Angell et al., 2016). A meta-analysis across 236 published articles found the differences among crude protein content of different phyla (% d/w) was minimal: Rhodophyta 9.83% (± 8.33); Chlorophyta 9.97% (± 8.56); Ochrophyta 9.20% (± 4.87) (Angell et al., 2015). Hence, perceptions of consistency in protein content among the phyla can be misleading (Angell et al., 2016). Seaweeds typically contain a high number of essential amino acids (EAA) required by the human body (Maehre et al., 2016; Fleurence et al., 2018; O’ Connor et al., 2020); hence, they have the potential to be nutritionally valuable (Bleakley and Hayes, 2017). There is inherent variety in seaweeds which also affects differences in amino acid (AA) profiles and thus some may be more favorable such as those with higher ratio of branched chain amino acids for human health benefits (O’ Connor et al., 2020). Differences in compositional components relevant to human nutrition other than AAs such as mineral content have also been identified to vary across algal species (Fleurence et al., 2012; Maehre et al., 2014; Charoensiddhi et al., 2016; Fleurence et al., 2018). As such, seaweeds have been identified as a promising novel source for food and pharmaceutical products (Pliego-Cortés et al., 2020).
New Zealand has over 1000 species of seaweeds across the three phyla (Nelson, 2013a). Some of the species are already used for nutrition and nutraceuticals. Macrocystis pyrifera is distributed predominantly along the east coast of New Zealand (Brown et al., 1997) and is harvested (White and White, 2020) under the Quota Management System administered by the Ministry for Primary Industries (MPI) (MPI). It is currently sold for agricultural and horticultural use as “Zelp”, as well as human nutrition as “Valére Kelp” in New Zealand (NZ Kelp, 2022). Undaria pinnatifida is an introduced species that is widespread in New Zealand, predominantly found along the east coast from as far north as the Hauraki Gulf/Tīkapa Moana to Stuart Island/Rakiura (White and White, 2020). This versatile seaweed is one of the main species for seaweed aquaculture globally (Smith et al., 2010; White and White, 2020) and is used for the production of nutraceuticals (Nadeeshani et al., 2022) and biostimulants (Salcedo et al., 2020). Porphyra/Pyropia spp. are somewhat cryptic in their distribution due to similar morphology and varied seasonal appearance around the New Zealand coastline but are known to inhabit rocky shores (Schweikert et al., 2012). These two related genera make the backbone of the prominent nori industry in Japan and China and constitute a significant proportion of the diet in Japan (Bocanegra et al., 2009). Sea lettuce (Ulva spp.) has 24 genetically distinct species found in New Zealand (Heesch et al., 2007). This genus of seaweed holds particular interest as a nutritional target algal group along with other bioproducts (Lamare and Wing, 2001; Ohno, 2006; Bolton et al., 2008; Bolton et al., 2016; Glasson et al., 2017; Glasson et al., 2019), particularly due to its ease of growth in land-based cultivation (Lawton et al., 2021).
The aim of this study was to initiate a preliminary investigation of the nutritional profiles of these seaweeds which are present in New Zealand to assess the nutritional potential of cultivated Ulva in comparison to other types of seaweed. A set of seaweed samples were analyzed for content of crude protein, amino acids, total lipids, carbohydrates, fiber, and mineral profiles. Antioxidant capacity, and heavy metals are also considered as these components influence the nutritional value and safe dietary inclusion levels for human consumption.
Materials and methods
Sample collection and preparation
Six seaweeds (five different species) were analyzed in this study, with each having three biological replicates of c. 60 g dry weight per sample. Samples were either collected from natural populations (P. plicata), cultivated in land-based recirculating aquaculture systems (U. stenophylloides and U. ralfsii), or sourced from commercial supplier NZ Kelp (M. pyrifera and U. pinatiffida), depending on availability. The commercial kelps were harvested by hand under commercial permit from Akaroa coastline within NZ Kelp’s farm where both species grow naturally. Three individual U. pinnatifida specimens were harvested in December and provided fully intact; blades were separated to be used for analysis with details below. Macrocystis pyrifera were provided as three separate samples already in dried, milled form (from whole plant milled to approximately 1 mm); time of harvest is unknown. Pyropia plicata (T) were collected wild by hand from Mt Maunganui, Tauranga, Bay of Plenty, New Zealand (37° 38’ 1” S, 176° 10’ 1” E) following consultation with mana whenua (Traditional Owners Ngāi Te Rangi, Ngāti Ranginui and Ngāti Pūkenga), in November 2020 under Ministry of Primary Industries – Special Permit Number MPI-SP#742. Pyropia plicata (K) was collected at the same time from Kaikōura, Canterbury, New Zealand by Professor David Schiel and his team from the University of Canterbury in November and December 2020, under MPI-SP#728, following consultation with Ngāi Tahu – the Māori iwi (tribe) in whose traditional waters the collection was made. Both species of Ulva were cultivated from stock sourced in Tauranga, Bay of Plenty, with original broodstock collected under MPI-SP#560, following consultation with Tauranga Moana mana whenua. Ulva stenophylloides [GenBank accession number MW250819.1], previously Ulva sp. B (Nelson et al., 2021) and U. ralfsii [GenBank accession number MW250805.1] were harvested from mixed gametophyte/sporophyte stock cultures cultivated at the University of Waikato Coastal Marine Field Station, Tauranga. These were cultivated indoors under artificial lighting as per Lawton et al (Lawton et al., 2021),. Harvesting occurred weekly through October 2020 for both Ulva spp.
All biomass (other than M. pyrifera) was frozen fresh (-20°C) and freeze-dried (-50°C, 72 h, 2 mBar, BUCHI LyovaporTM L-200 Freeze Dryer) prior to milling (FRITCH cutting mill PULVERISETTE 15 with a 0.5 mm sieve) following which biomass for each species was homogenized and subsequently split into three replicates. All seaweed powder biomass was sealed in plastic bags at room temperature with silica gel sachets in situ to prevent absorption of moisture.
Species identification
Macrocystis pyrifera and U. pinnatifida were identified (by the supplier) based on morphological characteristics. Both species of Ulva were maintained in culture since their original collection and identification, as described in Lawton et al (Lawton et al., 2021),. Due to morphological ambiguity in the genus (Meynard et al., 2019; Nelson et al., 2021), Pyropia samples were analyzed via genetic barcoding outsourced to NIWA (National Institute of Water and Atmospheric Research, New Zealand). DNA was extracted from 50 mg dried tissue of each sample using the using the Chelex method (Goff and Moon, 1993). Approximately 1300 bp of the rbcL locus was amplified using the primers F57/RbcS–S (Bartolo et al., 2020). All samples were successfully amplified and were sequenced in both directions by Macrogen Inc. Resulting DNA sequences were trimmed and assembled using Geneious Prime 2021.1.1., and the consensus sequences were compared with sequences in GenBank using BLAST (https://blast.ncbi.nlm.nih.gov/). Samples collected from the same location had identical DNA sequences, and sequences only differed between sites by 2 bp. DNA sequences were submitted to Genbank under the following accession numbers: P. plicata (T) samples: OK073982, and P. plicata (K) samples: OK073983.
Proximate analyses
Analysis of N, iodine, and ash content was outsourced to OEA labs (http://www.oealabs.com, Callington, UK), where samples were combusted in pure oxygen before being separated and quantified using gas chromatography-thermal conductivity detection (GC–TCD) (an organic elemental analysis technique, based on Dumas method, with ash quantified by dry ashing). Results were expressed as a percentage of the total mass of dry biomass (n = 3). Samples were analyzed for total lipid content using a previously described protocol (Gosch et al., 2012) that employs chloroform: methanol (2:1 v/v) for lipid extraction. Total carbohydrates were calculated by difference (with N to protein conversion factor of 6.25 for crude protein) using the equation (Ortiz et al., 2009):
Fiber analysis
Total dietary fiber analysis was performed using a Megazyme Total Dietary Fiber Assay Kit (K-TDFR-100A) as per the manufacturers’ protocol for Method 1, except centrifugation was used for separation of solid and liquid phases. Solids were washed as per the manufacturers protocol and dried at 50°C to remove excess acetone prior to freeze-drying. The solids were milled using a mortar and pestle prior to analysis of N and ash by OEA laboratories Limited. An N to protein conversion factor of 6.25 (Angell et al., 2016) was used to calculate the crude protein content of the solid. The total fiber contents, including the soluble and insoluble contents, were corrected for the content of protein and ash as per equation:
Metal, metalloids, and minerals analysis
The metals and metalloids (25 elements) were analyzed by Inductively Coupled Plasma – Mass Spectrometer (ICP–MS) at the University of Waikato Laboratory (The University of Waikato, Te Whare Wananga o Waikato) using an Agilent 8900 ICP–MS (Agilent Technologies, Santa Clara, California, USA) controlled by MassHunter Workstation (version 4.5). Samples were digested with double-distilled 65% HNO3 and 0.6 mL of 30% H2O2 before analysis. Samples were then introduced via an SPS4 autosampler and PVC tubing; a 0.05- 0.1 mL/min micromist U-Series nebuliser was attached to a quartz Scott Type spray chamber followed by a quartz torch with 2.5 mm injector. Following this, samples were introduced to the rest of the instrument via a nickel sampler and skimmer cone, followed by an extraction omega lens. The ICP-MS was run in pulse counting mode (with a dwell time of 0.1 s- 0.3 s), with radio frequency (RF) power set to 1550 W and gas flow rates at 15L/min (plasma gas), 1.05L/min (nebuliser carrier gas), 0.1L/min (dilution gas). A five-point calibration curve, consisting of concentrations between 0.1 and 500 ppb was prepared for all trace elements using stock standard IV71-A. A separate calibration curve, consisting of concentrations between 100 and 10,000ppb was prepared for major elements (Ca, Si, P, S, K, Fe) using single-element standards. Check standards were analyzed every 20 samples and re-calibration was performed every 100 samples. Blank samples were analyzed every 10 samples to ensure minimal carryover between samples. An online internal standard containing 45 Sc, 72 Ge, 103 Rh, 193 Ir, and 205 Tl was used to monitor and correct for instrumental drift and matrix effects.
Antioxidant analysis
Oxygen Radical Absorbance Capacity (ORAC) was chosen as the measure of antioxidant capacity most relevant to human physiology due to its hydrogen atom transfer reaction (Prior et al., 2005). The ORAC assay used was based on methods by Huang et al (Huang et al., 2002), and sample preparation methods by Wu et al (Wu et al., 2004), without use of methylated β–cyclodextrin as a solubility enhancer. Lipophilic extraction used Hex: DCM (1:1 v/v), and hydrophilic extraction used acetone/water/acetic acid (70:29.5:0.5 v/v). Both were at a concentration of 100 µg/ml. Butylated hydroxytoluene (BHT) (Supelco PHR1117; pharmaceutical secondary standard) was used as a positive control (Kindleysides et al., 2012) to allow comparison between studies.
Amino acid and crude protein analysis
All amino acids (AAs), with the exception of cysteine and tryptophan were analyzed at the Australian Proteome Analysis Facility, Macquarie University, Sydney (Kulshreshtha et al., 2014) using a standard amino acid assay procedure, accredited under ISO 17025 accreditation (Accreditation number 20344). It is common for cysteine and tryptophan to be excluded as they each require separate extraction and derivatization for analysis and therefore the cost-benefit for their acquisition is poor (Angell et al., 2016). The protein content was calculated as the sum of individual amino acids to give the Total Amino Acids (TAA) and used with N percentage for the calculation of a species-specific Nitrogen-to-Protein conversion factor (NPF) as per equation below.
Statistical analyses
Differences among the species were analyzed using one factor permutational analyses of variance (PERMANOVA) conducted in Primer v7 (Primer-E Ltd., UK) using Euclidean distances resemblance matrices, 9,999 unrestricted permutations of raw data, Type III sum of squares, and inclusion of Monte Carlo P value. Post-hoc PERMANOVA tests (pairwise) were carried out for all analyses which were significant according to the PERMANOVA test. Raw, unadjusted p (MC) values are reported for all analyses. The patterns of mineral content from ICP analysis and Amino acid were visualized using non-metric multidimensional scaling (nMDS; Primer 7).
Results
Proximate and fiber profiles
Content of ash varied significantly between species, with U. stenophylloides and P. plicata (K) having the lowest ash content and the remaining species all above 30% (Table 1). All species had relatively low lipid levels, ranging from 0.5 ± 0.1% (P. plicata (T)) to 5.3 ± 0.5% (U. ralfsii) (Table 2). Ulva ralfsii, with the highest lipid levels, was significantly different from all species except U. pinnatifida. Total carbohydrate content was above 50% in all seaweed except U. ralfsii (35.1 ± 1.2%) which was significantly lower than all other species (Table 2). Insoluble fiber was highest in P. plicata (K) 42.6 ± 20.6% and lowest in U. ralfsii at 22.1 ± 2.8%. There was statistical difference found between soluble fiber results (Table 2), however this data must be reviewed with care as due to the low amount of soluble fiber from the P. plicata samples replicates had to be combined to get a result.
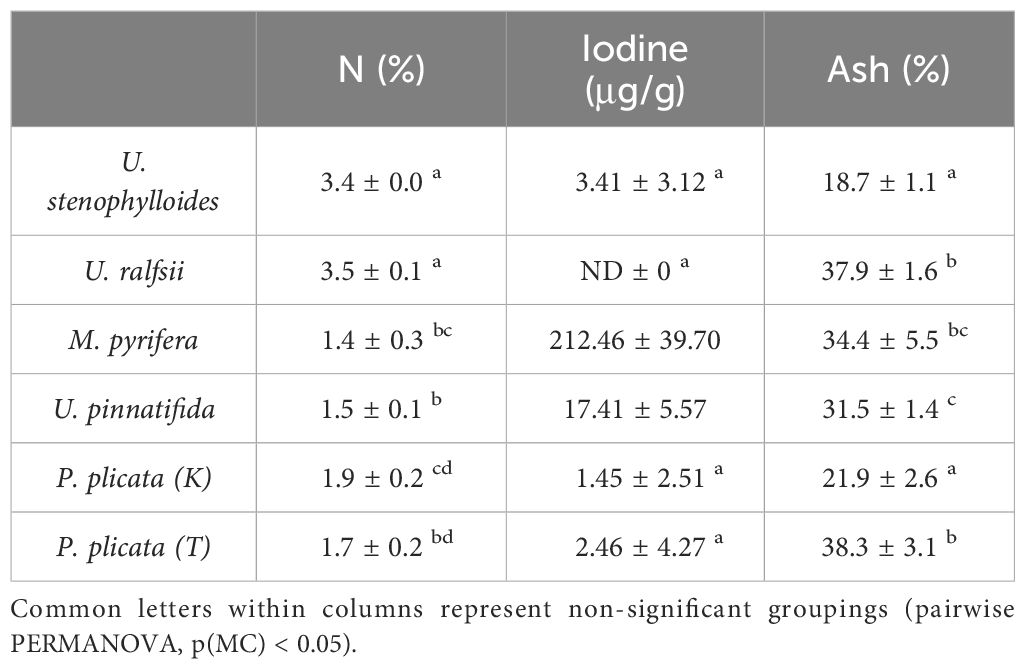
Table 1. Content (% of dry weight) of carbon (C), hydrogen (H), nitrogen (N), sulfur (S), iodine, and ash in selected New Zealand seaweed (means ± S.D, n=3).
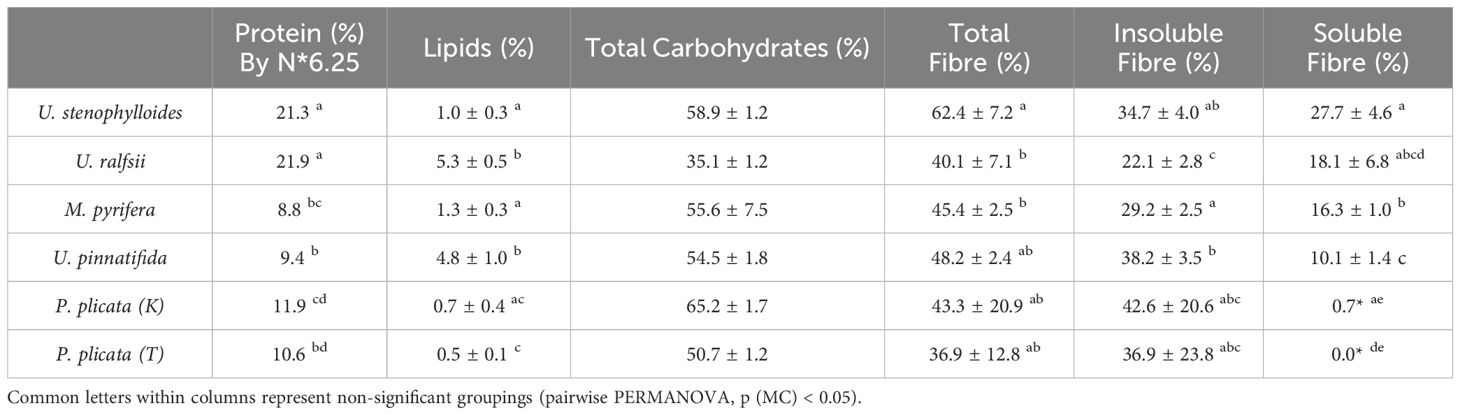
Table 2. Proximate composition (% of dry weight) of total lipids, carbohydrates, fibre, and protein for selected species of New Zealand seaweeds (means ± S.D, n = 3).
Amino acid and crude protein content
Both Ulva species were significantly different from all other species in their branched chain amino acids (BCAAs); isoleucine, leucine, valine (Table 3). All others [M. pyrifera, U. pinnatifida, P. plicata (K), and P. plicata (T)] were not significantly different from one another for isoleucine and leucine content. Valine was the only one of the three BCAAs where there were statistical differences between each phylum, but not between species within phyla. This was also the case for non-essential amino acid (NEAA) – serine, conditionally essential amino acid – glycine, and EAA – threonine. Almost all amino acids (excluding alanine, tyrosine, and methionine) had significant differences between the two Ulva spp. and the others (Table 3). It is also noted that the key drivers in both separations are a mix of NEAAs and EAAs. Protein as sum of TAA ranged from 6.4 ± 1.3% (M. pyrifera) to 15.1 ± 1.1% (U. stenophylloides) (Table 3), with both Ulva species being significantly different from all species except each other. Protein as sum of TAA (Table 3) was highest in the two species of Ulva, which differed from all other species (Table 4). Both species of Ulva had approximately two-fold higher protein content than all other species.
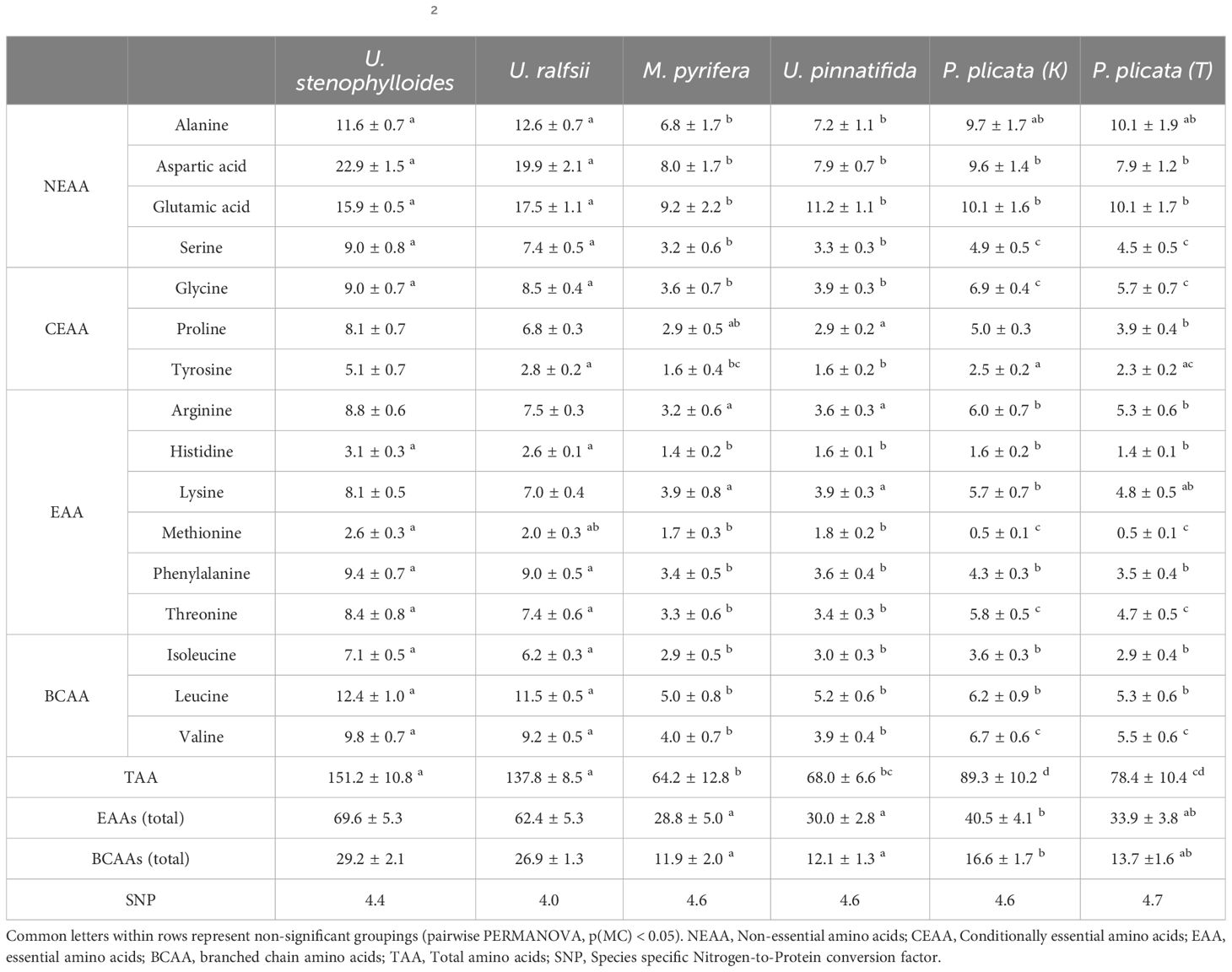
Table 3. Content of amino acids [(average ± std) -H2O; mg/g in biomass] in selected species of New Zealand seaweed.
Antioxidant profiles
Total antioxidant capacity by Trolox Equivalent (TE) (Table 5) was significantly different among species, with the Phaeophycaea having greater amounts than the Chlorophyta, which in turn had more than the Rhodophyta. This was driven completely by activity in the hydrophilic antioxidant content, as lipophilic antioxidants were absent in all species.
Minerals, metals and metalloid profiles
Content of metals/metalloids differed significantly between and within species (Figure 1 and Tables 6, 7). Mineral composition of the samples was generally separated by phyla, with the two Phaeophycaea grouping together, and the Pyropia from different locations grouped together (Figure 1). The two species of Ulva were surprisingly different considering they were cultivated under identical controlled conditions. Ulva ralfsii had the highest content of Na which was significantly different from all other species reflecting the high ash content of this species (Tables 6, 7). As seen in Figure 1, Ulva spp. were not grouped together, with Co and Na the key drivers of this separation (Figure 1). Iodine ranged from non-detectable in U. ralfsii to the highest levels in M. pyrifera 212.5 ± 39.7 μg/g (Table 1); both Phaeophycaea species were significantly higher than all other species with M. pyrifera also being significantly higher than U. pinnatifida. While U. ralfsii had a significantly higher level of Pb than U. stenophylloides (2.1 mg/kg compared to 0.5 mg/kg respectively) (Tables 6, 7), this was not a significant driver in separation between species at the R value > 0.85. Ulva stenophylloides, cultivated in the same conditions, had a much lower range of 0.4 – 0.7 mg/kg of Pb. There were several components driving the separation of M. pyrifera and U. pinnatifida: namely Ba, Sr, As and K. This is unsurprising as the As levels in M. pyrifera were significantly higher than the other samples at 100 mg/kg (Tables 6, 7), correlating with the lower Na:K ratio of M. pyrifera (0.25), as compared to U. pinnatifida (0.75) (Tables 6, 7).
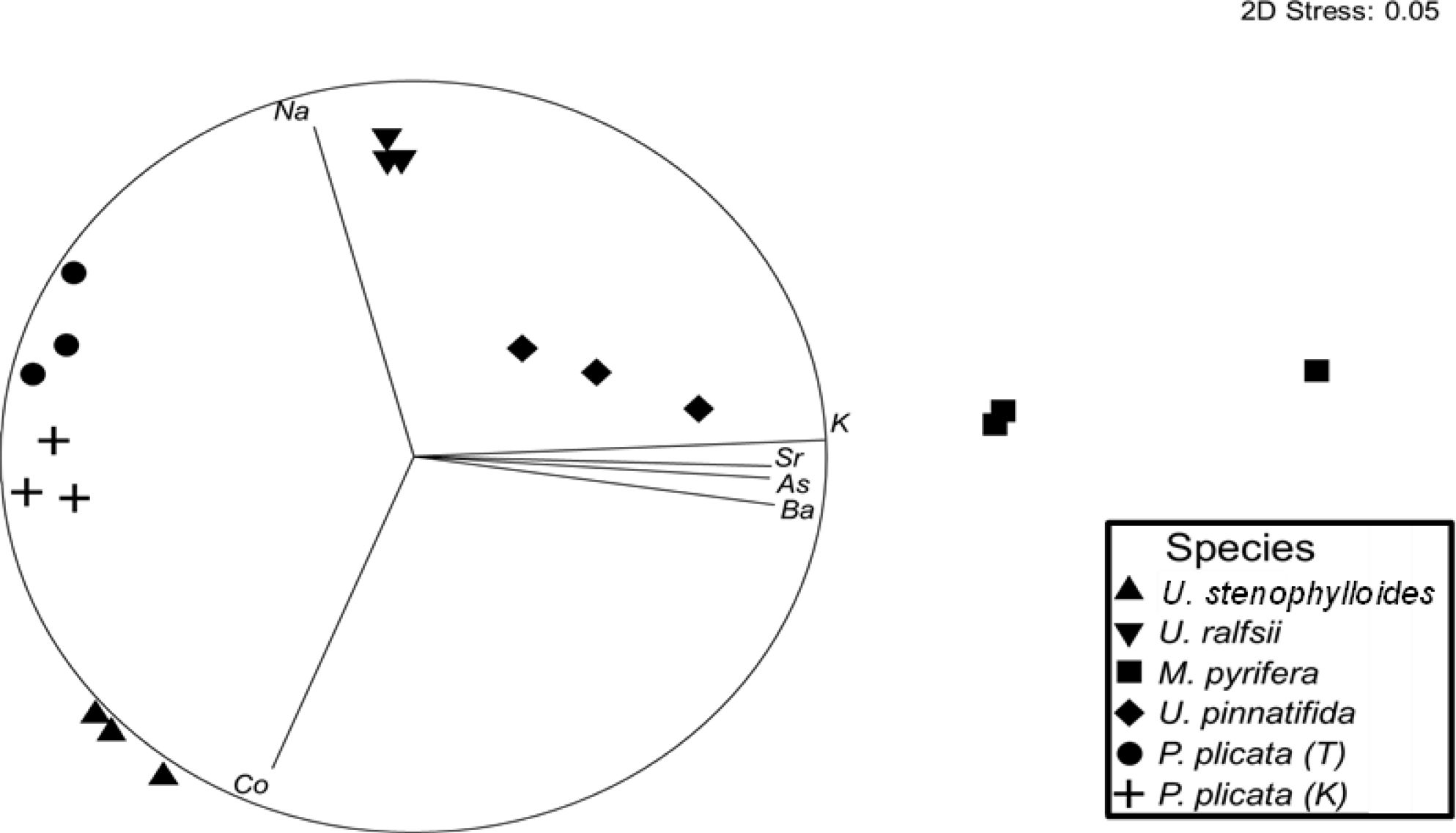
Figure 1. The nMDS scaling ordination of metals, metalloids and minerals of each sample (n = 3 biological replicates). Lines represent vector loadings of the specific minerals (Pearson's correlation, R > 0.85), with the size and direction of each vector representing the relative abundance of that element in that region of the plot.
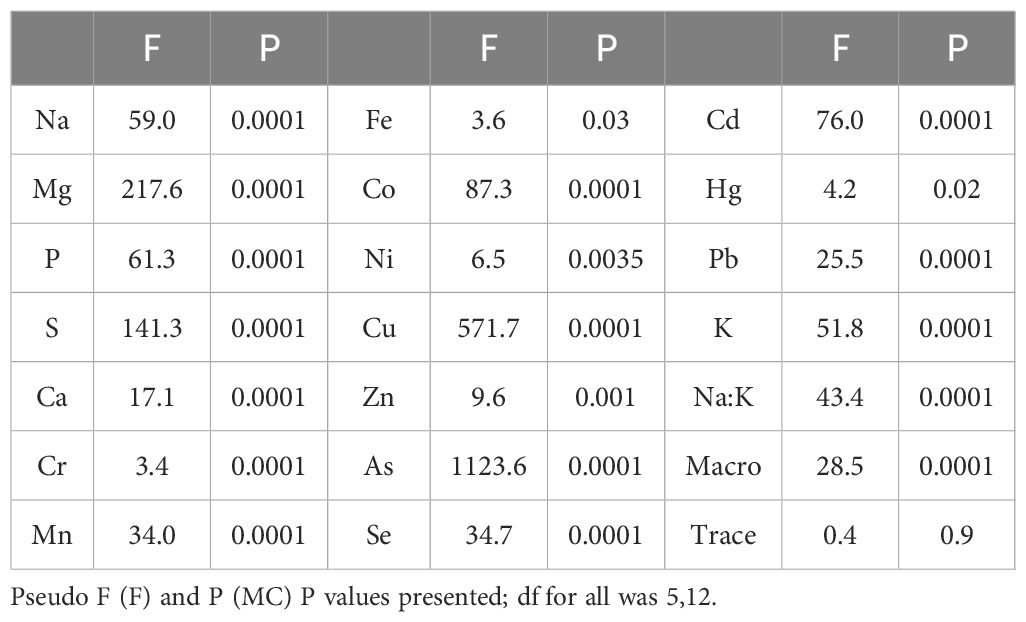
Table 6. Results of the main PERMANOVAs testing single factor analysis of ICP analysis of content of metals and metalloids.
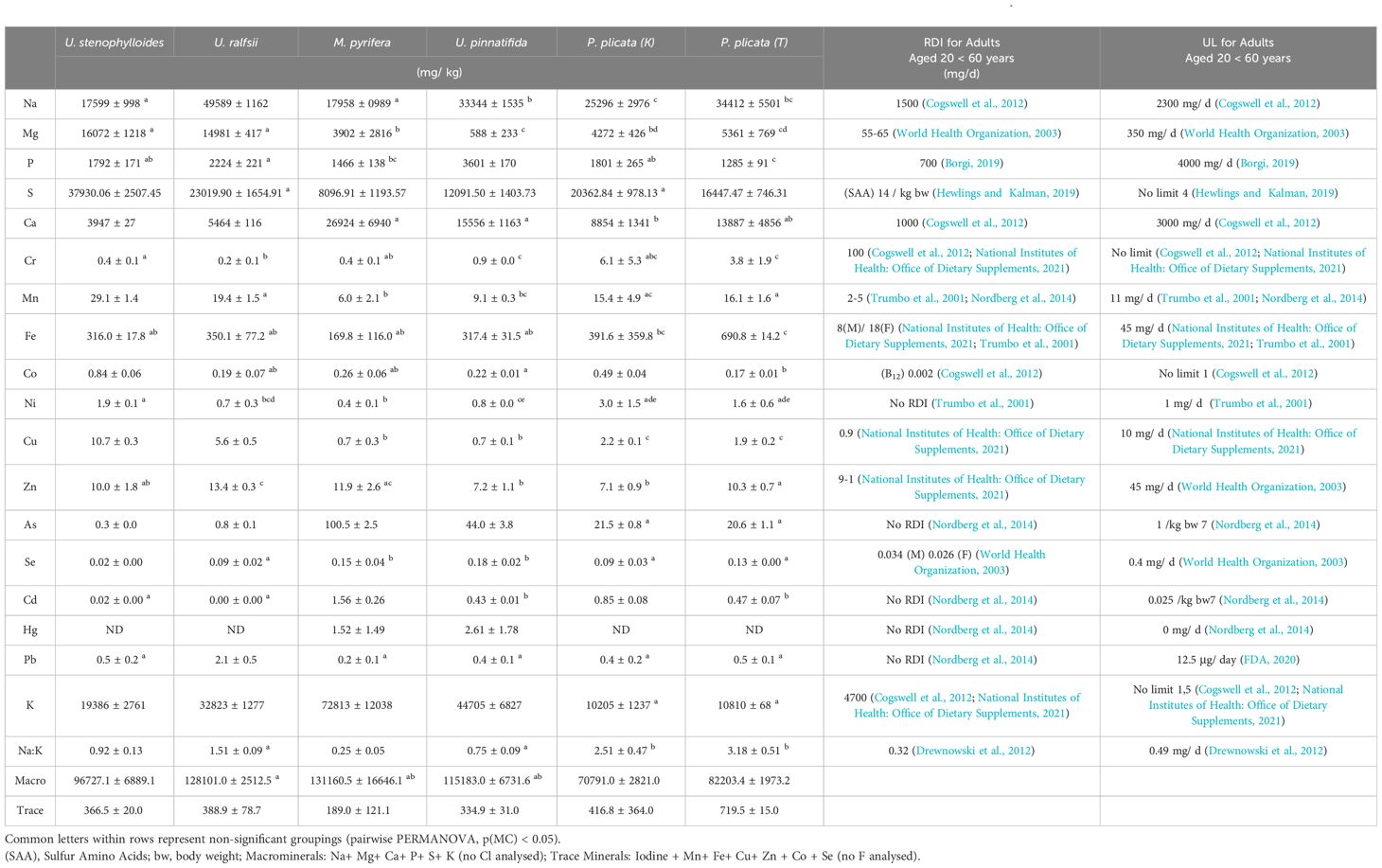
Table 7. Content (mg/ kg dry weight) of nutritionally relevant metals, metalloids, and phosphorus detected in order of atomic number (means ± S.D, n=3).
Discussion
Seaweeds represent a promising new source for future foods and nutraceuticals (Garcia-Perez et al., 2023). This study analyzed a broad profile of nutritionally relevant properties in two cultivated Ulva spp. and compared this with a range of New Zealand seaweeds collected from the wild and commercial harvest. Standard analytical techniques were used to determine their nutrition-related composition to give an indication of the potential that these seaweeds might possess for human nutrition.
Ulva ralfsii and U. stenophylloides
The crude protein content of the Ulva spp. reported here (21% dw) was comparable to Ulva from Australia that was grown in aquaculture (U. ohnoi with 18.5% dw) (Boisvert et al., 2015; Glasson et al., 2017); U. tepida with 15% dw (Magnusson et al., 2019)). Previous New Zealand wild-collected Ulva also had similar crude protein content to that found in this study (U. stenophylla with 20% dw) (Smith et al., 2010). For other proximates, U. stenophylloides mirrored the wild U. stenophylla in a prior study (Smith et al., 2010), though the U. ralfsii differed slightly from these with higher lipids and ash but lower total carbohydrates. This study’s U. ralfsii proximate profile was similar to commercially harvested U. lactuca in Canada (Magnusson et al., 2016). These were all different again from the lipid content in Norwegian studies where cultivated U. lactuca lipids (ranging 10-15%) (Roleda et al., 2021) were almost double that of this study’s U. ralfsii. Wild-harvested U. lactuca lipids were higher again (ranging 13-26% dw) (Maehre et al., 2014). The TAA of U. ralfsii (138 mg/g dw), though less so the U. stenophylloides (151 mg/g dw), was similar to sea lettuce cultivated in Norway (U. lactuca 137 mg/g dw) (Roleda et al., 2021). Wild-harvested U. fasciata from Brazil had several times higher TAA (419-431 mg/g) (de Melo et al., 2021). Antioxidant capacity of Ulva in this study were low, compared to a prior study where U. lactuca which showed up to 347 TE; though this study also noted that there can be high variation of antioxidant potential dependant on extract and concentration (de Melo et al., 2021). A prior study also found high variation in the trace minerals (micronutrients) across Norweigan cultivated Ulva (Roleda et al., 2021), while this high variation was not necessarily seen in the Ulva studied here, this may be due to the limited species and sample size. This study analyzed unprocessed Ulva spp. for broad nutritional profiles comparable with other species. A consequence of this is that potentially valuable components specific to Ulva spp. such as ulvan (Magnusson et al., 2019) were not assessed.
Pyropia plicata (Tauranga and Kaikōura)
This is possibly the first study to quantify the content of crude protein specifically for New Zealand P. plicata, though this may in part be due to changing naming conventions throughout the literature (Nelson, 2013b). The P. plicata of this study had crude protein content (c. 11%) which was significantly lower than other prior reports of Pyropia which are typically 30-40% (Fleurence, 1999a; Admassu et al., 2018). A prior study which sampled wild-harvested Porphyra spp. collected in New Zealand found crude protein content at this higher level (33% dw) (Smith et al., 2010). One explanation for the degree of difference could be the seasons in which samples were collected. A prior study found Porphyra and Palmaria spp. varied significantly across different seasons with higher protein content noted in winter collections (Galland-Irmouli et al., 1999; Stack et al., 2017). Porphyra spp. from the study of Smith et al (Smith et al., 2010), was collected across the New Zealand winter period, while this study’s Pyropia was harvested at the end of spring. A study of Indian Pyropia harvested in summer may support this as it had comparable protein and lipid content to those in this study (range of 14 – 18% protein and 2-3% lipids) (Kavale et al., 2017).
This may also indicate why the antioxidant levels of this study’s Pyropia were so low compared to a prior study’s Porphyra sp. TE 150 (Kindleysides et al., 2012). Stack et al., previously found wide variation in the TE of Porphyra dioica across seasons (Stack et al., 2017), and seasonal variations in the stress metabolites have been found in New Zealand P. plicata (Diehl et al., 2019). However, due to the limited samples in this study further research would be required to verify seasonal effect on nutritional profiles of New Zealand P. plicata.
Both macro and trace minerals appeared to be comparable to those in prior studies (Galland-Irmouli et al., 1999; Cian et al., 2014). It would be valuable to examine why the Tauranga P. plicata had almost double the trace minerals (total) than the Kaikōura P. plicata. Future studies would be required to further elucidate how and why the locational difference might affect mineral content of the same seaweed species through their chemical ecology.
Macrocystis pyrifera and Undaria pinnatifida
Though this is not the first study to nutritionally profile commercial M. pyrifera and U. pinnatifida, these samples were chosen for being available for New Zealand seaweed food products. The proximates from this study appeared to mirror those in a prior study with commercial New Zealand samples – crude protein, lipid, ash and carbohydrates: 11%, 2%, 43%, and 44% for M. pyrifera; 14%, 3%, 8%, and 45% respectively for U. pinnatifida (Smith et al., 2010). Though it was noted in this comparison that the protein profiles were slightly lower and the carbohydrates slightly higher for both species. Seasonal variation, as mentioned above, has also been noted for a New Zealand study on U. pinnatifida, with highest nitrogen levels in July (Austal winter) and lowest in September (Austral spring) (Zhou et al., 2015). Also as mentioned above, a follow up study with greater sample size and seasonal harvests would be required to justify this possible cause of lower protein levels found. A prior study found that brown seaweeds sampled had greater antioxidant activity than reds, with the M. pyrifera sampled averaging c. 450 TE (Kindleysides et al., 2012). While the trend of brown seaweeds having greater activity was also seen in this study, sample size limits the ability to lay claims based on algal phyla correlations. Further, overall antioxidant levels were much lower than previously reported. Whether this was due to method of analysis, species location or season would require further investigation to determine the cause.
Minerals and heavy metals were also comparable to the prior New Zealand study (Smith et al., 2010) and also indicated higher accumulation of heavy metals by Ochrophyta as compared to Rhodophyta or Chlorophyta as previously found (Yadav et al., 2019). As the two species of Ochrophyta are available as nutritional products (Taboada et al., 2013) it is worth noting that though they contained higher levels of heavy metals (with the exception of lead) than other species analyzed in this study, the results reported here were the total amounts measured in the unprocessed seaweeds. Mercury is most toxic in its methylmercury form (Fowler et al., 2014a) and arsenic in its inorganic form (Fowler et al., 2014b) which tend to be a much smaller proportion of the total mercury and arsenic present in seaweeds (Smith et al., 2010). A more in-depth metals assessment would be required to analyze the quantities of these more harmful forms present in seaweeds if intended for nutritional consumption. Also, processing has been found to reduce the levels of iodine in seaweed (Nitschke and Stengel, 2016) so future studies investigating the effect of processing on heavy metals content in seaweeds as potential future foods would be beneficial.
Limitations and implications for future studies
There are a number of limitations within this study that impact the interpretation of the differences reported. The nutritional environment was not monitored or controlled in samples other than the cultivated Ulva spp; which is significant given the inherent variability of this in natural environment/oceans. Controlled or closely replicated growing environments (cultivated/wild), and examining the variability associated with biogeographic location or habitat types would be required to accurately portray differences among species or phyla. Conversely, if wanting to investigate the effect of location or seasonal change on a single species and their respective yield of desirable nutritional products, greater sample numbers and collections of that species with a matrix of location/season/habitat would be required. This study applied common protocols to all species to attain a broad nutritional profile across a range of New Zealand algal species which limited its scope to a reporting of these potential qualities. Also because of this broad analytical profile, chemical components that may be specific to a particular species or genus were not investigated.
Conclusions
Pyropia plicata from the North and South Island, Macrocystis pyrifera and Undaria pinnatifida from commercial supplier, and Ulva stenophylloides and Ulva ralfsii from land-based cultivation, were all analyzed for their overall (dried but otherwise unprocessed) nutritional content. Ulva spp. had approximately 20% crude protein which is comparable to other seaweed species that have been suggested as novel protein sources. Due to the limitations of this preliminary study further work would be needed to address levels of crude protein in wild Ulva and the effect of varying nutritional environments when not cultivated. This research highlights the potential found in New Zealand grown seaweeds from a variety of sources. While limited in use for interpretation of data due to sample sizes, preliminary research such as this serves to indicate prospective food and nutraceutical components yet to be harnessed. While limited in its low number of samples, this study supports the idea that Ulva has potential as a source of nutrition.
Data availability statement
The datasets presented in this study can be found in online repositories. The names of the repository/repositories and accession number(s) can be found in the article/supplementary material. The original contributions presented in the study are publicly available. This data can be found here: https://hdl.handle.net/10289/15156.
Author contributions
ZB: Conceptualization, Data curation, Formal analysis, Investigation, Methodology, Project administration, Validation, Visualization, Writing – original draft, Writing – review & editing.
Funding
The author(s) declare financial support was received for the research, authorship, and/or publication of this article. University of Waikato for Masters Research and Entrepreneurial Universities, Tertiary Education Commission (TEC) - University of Waikato Contract: Macroalgal Biotechnology #106256.
Acknowledgments
Much gratitude is extended to Chris Glasson and Marie Magnusson for their advice and expertise given. Many thanks to David Schiel and his team for assistance in collecting the Kaikōura Pyropia plicata. Similarly, a big thanks to Roger Beattie for his supply of commercial kelps. Thank you to the EU team; Peter Randrup, for the Ulva spp. cultivation; Rebecca Lawton for her assistance with the genetic identification of the Pyropia plicata, without her input in this process it would not have been possible. A special thank you to Ariane Bradenburg for her support as laboratory technician; from laboratory procedures to sample shipment for outsourced analyses, her assistance was invaluable. Lastly thank you to Chris Battershill and Kim Pritchard for endless assistance and support at every stage of this research.
Conflict of interest
The authors declares that the research was conducted in the absence of any commercial or financial relationships that could be construed as a potential conflict of interest.
Publisher’s note
All claims expressed in this article are solely those of the authors and do not necessarily represent those of their affiliated organizations, or those of the publisher, the editors and the reviewers. Any product that may be evaluated in this article, or claim that may be made by its manufacturer, is not guaranteed or endorsed by the publisher.
References
Admassu H., Abera T., Abraha B., Yang R., Zhao W. (2018). Proximate, Mineral and Amino acid Composition of Dried Laver (Porphyra spp.) Seaweed. J. Academia Ind. Res. (JAIR) 6 (9).
Angell A. R., Mata L., de Nys R., Paul N. A. (2015). Indirect and direct effects of salinity on the quantity and quality of total amino acids in Ulva ohnoi (Chlorophyta). J. Phycology. 51, 536–545. doi: 10.1111/jpy.12300
Angell A. R., Mata L., de Nys R., Paul N. A. (2016). The protein content of seaweeds: a universal nitrogen-to-protein conversion factor of five. J. Appl. Phycology 28, 511–524. doi: 10.1007/s10811-015-0650-1
Bartolo A. G., Zammit G., Peters A. F., Küpper F. C. (2020). The current state of DNA barcoding of macroalgae in the Mediterranean Sea: presently lacking but urgently required. Botanica Marina 63, 253–272. doi: 10.1515/bot-2019-0041
Bleakley S., Hayes M. (2017). ‘Algal proteins: extraction, application, and challenges concerning production’. Foods 6, 33. doi: 10.3390/foods6050033
Bocanegra A., Bastida S., Benedí J., Ródenas S., Sánchez-Muniz F. J. (2009). ‘Characteristics and nutritional and cardiovascular-health properties of seaweeds’. J. Medicinal Food 12, 236–258. doi: 10.1089/jmf.2008.0151
Boisvert C., Beaulieu L., Bonnet C., Pelletier É. (2015). Assessment of the antioxidant and antibacterial activities of three species of edible seaweeds. J. Food Biochem. 39, 377–387. doi: 10.1111/jfbc.12146
Bolton J., Robertson-Andersson D. V., Shuuluka D., Kandjengo L. (2008). Growing Ulva (Chlorophyta) in integrated systems as a commercial crop for abalone feed in South Africa: a SWOT analysis. J. Appl. Phycology 21, 575–583. doi: 10.1007/s10811-008-9385-6
Bolton J. J., Cyrus M. D., Brand M. J., Joubert M., Macey B. M. (2016). Why grow Ulva? Its potential role in the future of aquaculture. Perspect. Phycology 3, 113–120. doi: 10.1127/pip/2016/0058
Borgi L. (2019). Inclusion of phosphorus in the nutrition facts label. Clinical Journal of the American Society of Nephrology 14 (1), 139–140. doi: 10.2215/CJN.07230618
Brown M. T., Nyman M. A., Keogh J. A., Chin N. K.M. (1997). Seasonal growth of the giant kelp Macrocystis pyrifera in New Zealand. Mar. Biol. 129, 417–424. doi: 10.1007/s002270050182
Charoensiddhi S., Conlon M. A., Vuaran M. S., Franco C. M.M., Zhang W. (2016). Impact of extraction processes on prebiotic potential of the brown seaweed Ecklonia radiata by in vitro human gut bacteria fermentation. J. Funct. Foods 24, 221–230. doi: 10.1016/j.jff.2016.04.016
Cian R. E., Fajardo M. A., Alaiz M., Vioque J., González R. J., Drago S. R. (2014). Chemical composition, nutritional and antioxidant properties of the red edible seaweed Porphyra columbina. Int. J. Food Sci. Nutr. 65, 299–305. doi: 10.3109/09637486.2013.854746
Cogswell M. E., Zhang Z., Carriquiry A. L., Gunn J. P., Kuklina E. V., Saydah S. H., et al. (2012). Sodium and potassium intakes among US adults: NHANES 2003–2008. The American Journal of Clinical Nutrition 96 (3), 647–657. doi: 10.3945/ajcn.112.034413
de Melo N. S. M., Cardoso L. G., de Castro Nunes J. M., Brito G. B., Caires T. A., de Souza C. O., et al. (2021). Effects of dry and rainy seasons on the chemical composition of Ulva fasciata, Crassiphycus corneus, and Sargassum vulgare seaweeds in tropical environment. Braz. J. Bot. 44, 331–344. doi: 10.1007/s40415-021-00700-4
Diehl N., Michalik D., Zuccarello G. C., Karsten U. (2019). Stress metabolite pattern in the eulittoral red alga Pyropia plicata (Bangiales) in New Zealand – mycosporine-like amino acids and heterosides. J. Exp. Mar. Biol. Ecol. 510, 23–30. doi: 10.1016/j.jembe.2018.10.002
Drewnowski A., Maillot M., Rehm C. (2012). Reducing the sodium-potassium ratio in the US diet: a challenge for public health. The American journal of clinical nutrition 96(2), 439–444.
FDA. (2020). Lead in Food, Foodwares, and Dietary Supplements. FDA. Available online at: https://www.fda.gov/food/metals-and-your-food/lead-food-foodwares-and-dietary-supplements
Fleurence J. (1999a). Seaweed proteins: biochemical, nutritional aspects and potential uses. Trends Food Sci. Technol. 10, 25–28. doi: 10.1016/S0924-2244(99)00015-1
Fleurence J., Morançais M., Dumay J., Decottignies P., Turpin V., Munier M., et al. (2012). What are the prospects for using seaweed in human nutrition and for marine animals raised through aquaculture? Trends Food Sci. Technol. 27, 57–61. doi: 10.1016/j.tifs.2012.03.004
Fleurence J., Morançais M., Dumay J. (2018). “‘9 - seaweed proteins’,” in Proteins in food processing, 2nd ed. Ed. Yada R. Y. (Cambridge: Woodhead Publishing), 245–262. doi: 10.1016/B978-0-08-100722-8.00010-3
Fowler B. A., Alexander J., Oskarsson A. (2014a).‘Toxic metals in food’. In: Handbook on the toxicology of metals (London, NETHERLANDS: Elsevier Science & Technology). Available online at: http://ebookcentral.proquest.com/lib/waikato/detail.action?docID=1757714 (Accessed 8 October 2020).
Fowler B. A., Chou C. H.S. J., Jones R. L., Sullivan D. W. Jr, Chen C. J. (2014b).‘Arsenic’. In: Handbook on the toxicology of metals (London, NETHERLANDS: Elsevier Science & Technology). Available online at: http://ebookcentral.proquest.com/lib/waikato/detail.action?docID=1757714 (Accessed 8 October 2020).
Galland-Irmouli A.-V., Fleurence J., Lamghari R., Luçon M., Rouxel C., Barbaroux O., et al. (1999). Nutritional value of proteins from edible seaweed Palmaria palmata (dulse). J. Nutr. Biochem. 10, 353–359. doi: 10.1016/S0955-2863(99)00014-5
Garcia-Perez P., Cassani L., Garcia-Oliveira P., Xiao J., Simal-Gandara J., Prieto M. A., et al. (2023). Algal nutraceuticals: A perspective on metabolic diversity, current food applications, and prospects in the field of metabolomics. Food Chem. 409, 135295. doi: 10.1016/j.foodchem.2022.135295
Glasson C. R. K., Sims I. M., Carnachan S. M., de Nys R., Magnusson M. (2017). A cascading biorefinery process targeting sulfated polysaccharides (ulvan) from Ulva ohnoi. Algal Res. 27, 383–391. doi: 10.1016/j.algal.2017.07.001
Glasson C. R. K., Donnet L., Angell A., Vucko M. J., Lorbeer A. J., Vamvounis G., et al. (2019). Multiple response optimisation of the aqueous extraction of high quality ulvan from Ulva ohnoi. Bioresource Technol. Rep. 7. doi: 10.1016/j.biteb.2019.100262
Goff L. J., Moon D. A. (1993). Pcr amplification of nuclear and plastid genes from algal herbarium specimens and algal spores. J. Phycology 29, 381–384. doi: 10.1111/j.0022-3646.1993.00381.x
Gosch B. J., Magnusson M., Paul N. A., de Nys R. (2012). Total lipid and fatty acid composition of seaweeds for the selection of species for oil-based biofuel and bioproducts. GCB Bioenergy 4, 919–930. doi: 10.1111/j.1757-1707.2012.01175.x
Heesch S., Broom J., Neill K., Farr T., Dalen J., Nelson W. (2007). Genetic diversity and possible origins of New Zealand populations of Ulva. (Wellington, N.Z.: National Institute of Water and Atmospheric Research). New Zealand Technical Paper. No. 2007/01 . [i]–vii, [1]–219.
Hou X., Hansen J. H., Bjerre A.-B. (2015). Integrated bioethanol and protein production from brown seaweed Laminaria digitata. Bioresource Technol. 197, 310–317. doi: 10.1016/j.biortech.2015.08.091
Huang D., Ou B., Hampsch-Woodill M., Flanagan J. A., Prior R. L. (2002). High-throughput assay of oxygen radical absorbance capacity (ORAC) using a multichannel liquid handling system coupled with a microplate fluorescence reader in 96-well format. J. Agric. Food Chem. 50, 4437–4444. doi: 10.1021/jf0201529
Iwasaki H. (1967). Nutritional studies of the edible seaweed porphyra tenera. II. Nutrition of conchocelis 1. J. Phycology 3, 30–34. doi: 10.1111/j.1529-8817.1967.tb04625.x
Kavale M. G., Kazi M. A., Sreenadhan N., Murgan P. (2017). Nutritional profiling of Pyropia acanthophora var. robusta (Bangiales, Rhodophyta) from Indian waters. J. Appl. Phycology 29, 2013–2020. doi: 10.1007/s10811-017-1096-4
Kindleysides S., Quek S.-Y., Miller M. R. (2012). Inhibition of fish oil oxidation and the radical scavenging activity of New Zealand seaweed extracts. Food Chem. 133, 1624–1631. doi: 10.1016/j.foodchem.2012.02.068
Kulshreshtha G., Rathgeber B., Stratton G., Thomas N., Evans F., Critchley A., et al. (2014). Feed supplementation with red seaweeds, Chondrus crispus and Sarcodiotheca gaudichaudii, affects performance, egg quality, and gut microbiota of layer hens. Poultry Sci. 93, 2991–3001. doi: 10.3382/ps.2014-04200
Lamare M. D., Wing S. R. (2001). Calorific content of New Zealand marine macrophytes. New Z. J. Mar. Freshw. Res. 35, 335–341. doi: 10.1080/00288330.2001.9517004
Lawton R. J., Sutherland J. E., Glasson C. R. K., Magnusson M. E. (2021). Selection of temperate Ulva species and cultivars for land-based cultivation and biomass applications. Algal Res. 56, 102320. doi: 10.1016/j.algal.2021.102320
Maehre H. K., Malde M. K., Eilertsen K.-E., Elvevoll E. O. (2014). Characterization of protein, lipid and mineral contents in common Norwegian seaweeds and evaluation of their potential as food and feed: Biochemical composition of marine macroalgae. J. Sci. Food Agric. 94, 3281–3290. doi: 10.1002/jsfa.6681
Maehre H. K., Edvinsen G. K., Eilertsen K.-E., Elvevoll E. O. (2016). Heat treatment increases the protein bioaccessibility in the red seaweed dulse (Palmaria palmata), but not in the brown seaweed winged kelp (Alaria esculenta). J. Appl. Phycology 28, 581–590. doi: 10.1007/s10811-015-0587-4
Magnusson M., Carl C., Mata L., de Nys R., Paul N. A. (2016). Seaweed salt from Ulva: A novel first step in a cascading biorefinery model. Algal Res. 16, 308–316. doi: 10.1016/j.algal.2016.03.018
Magnusson M., Glasson C. R.K., Vucko M. J., Angell A., Neoh T. L., de Nys R. (2019). Enrichment processes for the production of high-protein feed from the green seaweed Ulva ohnoi. Algal Res. 41, 101555. doi: 10.1016/j.algal.2019.101555
Meynard A., Zapata J., Salas N., Betancourtt C., Pérez‐Lara G., Castañeda F., et al. (2019). Genetic and morphological differentiation of Porphyra and Pyropia species (Bangiales, Rhodophyta) coexisting in a rocky intertidal in Central Chile. J. Phycology 55, 297–313. doi: 10.1111/jpy.12829
MPI Fish quota management system | MPI - ministry for primary industries. A New Zealand government department (Ministry for Primary Industries). Available online at: https://www.mpi.govt.nz/legal/legislation-standards-and-reviews/fisheries-legislation/quota-management-system/ (Accessed 14 April 2022).
Nadeeshani H., Hassouna A., Lu J. (2022). Proteins extracted from seaweed Undaria pinnatifida and their potential uses as foods and nutraceuticals. Crit. Rev. Food Sci. Nutr. 62, 6187–6203. doi: 10.1080/10408398.2021.1898334
National Institutes of Health: Office of Dietary Supplements. (2021). Dietary supplement fact sheets. Dietary Supplement Fact Sheets. Available online at: https://ods.od.nih.gov/factsheets/.
Nelson W. A. (2013a). New Zealand seaweeds: An illustrated guide (Wellington, New Zealand: Te Papa Press).
Nelson W. A. (2013b). Pyropia plicata sp. nov. (Bangiales, Rhodophyta): naming a common intertidal alga from New Zealand. PhytoKeys 21), 17–28. doi: 10.3897/phytokeys.21.4614
Nelson W. A., D’Archino R., Neill K. F., Robinson N. M. (2021). Introduced marine macroalgae: new perspectives on species recognition and distribution in New Zealand. Botanica Marina 64, 379–393. doi: 10.1515/bot-2021-0042
Nitschke U., Stengel D. (2016). Quantification of iodine loss in edible Irish seaweeds during processing. J. Appl. Phycology 28, 3527–3533. doi: 10.1007/s10811-016-0868-6
Nordberg G. F., Fowler B. A., Nordberg M. (2014). Toxicology of Metals: Overview, definitions, concepts, and trends. In Nordberg G. F., Fowler B. A., Nordberg M. (Eds.), Handbook on the Toxicology of Metals. 4th ed. (Elsevier Science & Technology) Vol. 1, pp. 3–14. Available online at: http://ebookcentral.proquest.com/lib/7aikato/detail.action?docID=1757714
NZ Kelp (2022). Fully certified organic dried kelp (NZ Kelp). Available online at: https://www.nzkelp.co.nz/about (Accessed 14 April 2022).
O’ Connor J., Meaney S., Williams G. A., Hayes M. (2020). Extraction of protein from four different seaweeds using three different physical pre-treatment strategies. Molecules 25. doi: 10.3390/molecules25082005
Ohno M. (2006).‘Recent developments in the seaweed cultivation and industry in Japan’. In: Advances in seaweed cultivation and utilisation in Asia: proceedings of a workshop held in conjunction with the 7th Asiean Fisheries Forum, Penang (Kuala Lumpur: University of Malaya). Available online at: https://drive.google.com/file/d/1gehu4Sqv2060iPtnyb-RtuvWPIVFP5uy/view?usp=drive_web&usp=embed_facebook (Accessed 14 April 2022).
Ortiz J., Uquiche E., Robert P., Romero N., Quitral V., Llantén C., et al. (2009). Functional and nutritional value of the Chilean seaweeds Codium fragile, Gracilaria Chilensis and Macrocystis pyrifera. Eur. J. Lipid Sci. Technol. 111, 320–327. doi: 10.1002/ejlt.200800140
Pliego-Cortés H., Wijesekara I., Lang M., Bourgougnon N., Bedoux G. (2020). “Current knowledge and challenges in extraction, characterization and bioactivity of seaweed protein and seaweed-derived proteins,” in Seaweeds around the world: state of art and perspectives, vol. 95. (United Kingdom: Academic Press). doi: 10.1016/bs.abr.2019.11.008
Prior R. L., Wu X., Schaich K. (2005). Standardized methods for the determination of antioxidant capacity and phenolics in foods and dietary supplements. Journal of agricultural and food chemistry. 53 (10), 4290–4302. doi: 10.1021/jf0502698
Roleda M. Y., Lage S., Aluwini D. F., Rebours C., Brurberg M. B., Nitschke U., et al. (2021). Chemical profiling of the Arctic sea lettuce Ulva lactuca (Chlorophyta) mass-cultivated on land under controlled conditions for food applications. Food Chem. 341, 127999. doi: 10.1016/j.foodchem.2020.127999
Salcedo M. F., Colman S. L., Mansilla A. Y., Martínez M. A., Fiol D. F., Alvarez V. A., et al. (2020). ‘Amelioration of tomato plants cultivated in organic-matter impoverished soil by supplementation with Undaria pinnatifida’. Algal Res. 46, 101785. doi: 10.1016/j.algal.2019.101785
Schweikert K., Sutherland J. E., Burritt D. J., Hurd C. L. (2012). ‘Analysis of spatial and temporal diversity and distribution of Porphyra (Rhodophyta) in Southeastern New Zealand supported by the use of molecular tools’. J. Phycol. 48, 530–538. doi: 10.1111/j.1529-8817.2012.01161.x
Smith J. L., Summers G., Wong R. (2010). Nutrient and heavy metal content of edible seaweeds in New Zealand. New Z. J. Crop Hortic. Sci. 38, 19–28. doi: 10.1080/01140671003619290
Stack J., Tobin P. R., Gietl A., Harnedy P. A., Stengel D. B., FitzGerald R. J. (2017). Seasonal variation in nitrogenous components and bioactivity of protein hydrolysates from. Porphyra dioica. J. Appl. Phycology 29, 2439–2450. doi: 10.1007/s10811-017-1063-0
Taboada M. C., Millán R., Miguez M. I. (2013). Nutritional value of the marine algae wakame (Undaria pinnatifida) and nori (Porphyra purpurea) as food supplements. J. Appl. Phycology 25, 1271–1276. doi: 10.1007/s10811-012-9951-9
Trumbo P., Yates A. A., Schlicker S., Poos M. (2001). Dietary Reference Intakes: Vitamin A, Vitamin K, Arsenic, Boron, Chromium, Copper, Iodine, Iron, Manganese, Molybdenum, Nickel, Silicon, Vanadium, and Zinc. Journal of the American Dietetic Association 101 (3), 294–301. doi: 10.1016/S0002-8223(01)00078-5
White L. N., White W. L. (2020). Seaweed utilisation in New Zealand. Botanica Marina 63, 303–313. doi: 10.1515/bot-2019-0089
World Health Organization. (2003). Vitamin and Mineral Requirements in Human Nutrition: Report of a Joint FAO/WHO Expert Consultation. Albany, SWITZERLAND: World Health Organization. Available online at: http://ebookcentral.proquest.com/lib/waikato/detail.action?docID=284637.
Wu X., Beecher G. R., Holden J. M., Haytowitz D. B., Gebhardt S. E., Prior R. L. (2004). Lipophilic and hydrophilic antioxidant capacities of common foods in the United States. J. Agric. Food Chem. 52, 4026–4037. doi: 10.1021/jf049696w
Yadav P., Singh J., Mishra V. (2019). “‘Biosorption-cum-bioaccumulation of heavy metals from industrial effluent by brown algae: deep insight’,” in Microbial genomics in sustainable agroecosystems, vol. 1 . Ed. Tripathi V., et al (Springer, Singapore), 249–270. doi: 10.1007/978-981-13-8739-5_13
Keywords: algae, macroalgae, seaweed, protein, nutrition
Citation: Battershill ZV (2024) Nutritional profiling of five New Zealand seaweeds – a preliminary assessment. Front. Mar. Sci. 11:1410005. doi: 10.3389/fmars.2024.1410005
Received: 31 March 2024; Accepted: 02 July 2024;
Published: 13 August 2024.
Edited by:
Michael Packer, Independent researcher, Nelson, New ZealandReviewed by:
Tom Wheeler, Cawthron Institute, New ZealandMathew Cumming, The New Zealand Institute for Plant and Food Research Ltd, New Zealand
Copyright © 2024 Battershill. This is an open-access article distributed under the terms of the Creative Commons Attribution License (CC BY). The use, distribution or reproduction in other forums is permitted, provided the original author(s) and the copyright owner(s) are credited and that the original publication in this journal is cited, in accordance with accepted academic practice. No use, distribution or reproduction is permitted which does not comply with these terms.
*Correspondence: Zoe V. Battershill, emJhdHRlcnNoaWxsQGdtYWlsLmNvbQ==