- 1The Steinhardt Museum of Natural History, Israel National Center for Biodiversity Studies, Tel Aviv, Israel
- 2Department of Chemical and NanoEngineering, University of California San Diego, San Diego, CA, United States
- 3Marine Biology Research Division, Scripps Institution of Oceanography, University of California San Diego, San Diego, CA, United States
- 4The Interuniversity Institute for Marine Sciences of Eilat, Eilat, Israel
- 5School of Zoology, Tel-Aviv University, Tel Aviv, Israel
Bioerosion plays a crucial factor in shaping the structure and function of coral reef ecosystems, with bioeroders actively altering both the physical and ecological dynamics of coral substrates. Despite their importance, studying internal bioeroders in corals presents significant challenges owing to their cryptic nature within the skeletal structures. Additionally, invasive methods are often required to reveal the subtle and microscopic bioerosive alterations they induce in calcium carbonate substrates. Here, we demonstrate the effectiveness of high-resolution micro-computed tomography (μCT) in quantifying the abundance, size, distribution, and growth directions of coral bioeroders such as cryptic calcareous bivalves in the northern Red Sea. We scanned three coral species inhabited by bioeroders, followed by the utilization of three-dimensional image analysis software to identify, count, and measure each bivalve within the coral skeleton, along with quantifying boring cavity volumes. We revealed that μCT captures small boring cavities (< 1mm), providing more accurate abundance estimates of live and dead boring bivalves than the skeleton decalcification technique, with the added benefits of being rapid and non-destructive in contrast to traditional methods. Furthermore, measurements of empty cavity volumes enabled the estimations of the contribution of bioeroders to the overall coral skeletal porosity. Overall, our study highlights μCT as a practical and effective tool for studying cryptic coral bioeroders, providing novel ecological insights into bioeroder population ecology and coral-bioeroder interactions.
1 Introduction
Coral reefs, renowned for their vibrant biodiversity and ecological significance, host a rich array of species that occupy various microhabitats within their calcium carbonate formations. The sustainability of coral structures relies on maintaining an equilibrium between reef accretion, primarily driven by hermatypic corals, and processes that break down calcium carbonate substrates (Connell, 1978; Silbiger et al., 2018). While corals are the primary reef builders, they are complemented by the contributions of other organisms such as crustose coralline algae, calcareous sponges, foraminifera, and certain mollusks, all enhancing reef framework formation. This equilibrium faces constant challenges from erosion, occurring in two primary forms: physical erosion from wave action and currents, and bioerosion (often considered the predominant form of erosion), involving organisms that feed on or bore into the reef framework (Connell, 1978; Silbiger et al., 2018). While some reef inhabitants, such as corallivores (dominated by parrotfish and urchin species), directly alter the reef framework through grazing, cryptic “boring” organisms also play important yet hidden roles. Within the calcium carbonate coral skeletons, cryptic communities of bioeroding organisms thrive by actively penetrating and removing the coral’s structural materials through bioerosion activities (Glynn and Manzello, 2015; Fordyce et al., 2021; Rice et al., 2020).
Bioeroders modify the architectural complexity of reefs across multiple spatial scales, ranging from the micron to meter level (Davidson et al., 2018; Fordyce et al., 2020a; Glynn and Manzello, 2015; Roff et al., 2019). These bioeroders are broadly categorized as either surface-dwelling or internal-boring depending on their location and mode of erosive activity. External bioeroders reside on exposed reef surfaces, whereas internal bioeroders reside hidden within the calcium carbonate skeleton (Glynn and Manzello, 2015). Although the majority of internal bioeroders remain cryptic, that is, concealed within the substrates they penetrate, evidence suggests that their abundance and biomass may equal or surpass those of external bioeroders (Ginsburg, 1983; Weinstein et al., 2016). Additionally, internal bioeroders exhibit the highest taxonomic diversity among bioeroding organisms (Glynn and Manzello, 2015). The key taxa among the internal bioeroders that penetrate coral skeletons, also termed macroendoliths, include Porifera, Polychaeta, Sipuncula, Bivalvia, and Cirripedia (MacGeachy and Stearn, 1976). At the microscopic scale, microendolithic communities of cyanobacteria, chlorophytes, rhodophytes, and fungi actively penetrate structural calcium carbonate substrates (Hutchings, 1986; Perry and Harborne, 2016).
Despite the high biomass, diversity, and prevalence of internal bioeroders observed in many scleractinian corals, a comprehensive understanding of these cryptic organisms remains elusive (Schönberg et al., 2017). The profound inaccessibility of internal bioeroders significantly impedes research efforts, contributing to this knowledge gap. Moreover, the complexity of bioerosion processes, which intricately shape various facets of reef ecological functions (Fordyce et al., 2020a), poses additional challenges to understanding this phenomena. For instance, in contrast to the apparent detrimental impact on reef substrata strength, cavities generated by internal bioeroders augment habitat complexity, thereby enhancing the diversity and biomass of organisms associated with reefs (Glynn and Manzello, 2015). There is a critical need to enhance our understanding of bioeroders’ abundance, burrowing activities, and potential impacts on coral skeletal properties, as these organisms play a pivotal role in influencing coral reef resilience and stability (Schönberg et al., 2017).
To date, various methodologies have been employed to assess the abundance of internal bioeroders, including the cross-sectioning of bioeroder cavities, coral skeletal decalcification, and in situ surveys (Maher et al., 2018; Rice et al., 2019; Risk et al., 1995; Scott, 1988). While these approaches enable the direct quantification of living bioeroders in their natural habitat, they are limited in identifying cavities blocked by coral overgrowth, which may result from the death of bioeroders during the coral lifespan or coverage by other settlements. These methods provide limited data on the abundance of living bioeroders and lack information on cavity volume or the impact of bioeroders on substrate properties such as skeleton density and porosity.
Another technique utilized to gain insights into the extent and characteristic patterns of skeletal excavation by diverse organisms is the use of X-ray scans (Hein and Risk, 1975; MacGeachy and Stearn, 1976; Highsmith et al., 1983; Risk and Sammarco, 1991). However, simple X-ray radiography offers limited insights, as it is a two-dimensional imaging technique and is typically characterized by lower resolution. Computed Tomography (CT), on the other hand, offers exceptional three-dimensional (3D) high-resolution imaging, facilitating the non-destructive analysis of intricate microstructural details within coral skeletons without physically altering or destroying the original skeleton (DeCarlo et al., 2015; Fordyce et al., 2020b; Kramer et al., 2022). Previous studies utilizing CT for non-destructive analysis have quantified carbonate production, bioeroding activities, and net accretion on coral substrates (Silbiger et al., 2014, 2016, 2017; DeCarlo et al., 2015; Enochs et al., 2016, 2021; Newman et al., 2023). However, detailed methodological evaluations of CT for quantitative analysis of cryptic internal coral bioeroders, such as calcareous bivalves, are insufficient.
Here, we present a case study that evaluates the effectiveness of µCT scanning in conducting high-resolution morphometric analyses of common coral-boring species in the northern Red Sea, and present evidence of the advantages of this technique over traditional methods. Furthermore, we address potential concerns regarding the use of preserved coral samples for µCT scanning. Our complementary approach provides precise quantification of internal calcareous bioeroders that can be applied to advance our understanding of the interactions between these organisms and their calcifying hosts.
2 Materials and methods
2.1 Coral collection and preparation
We selected three reef-building coral species from the Gulf of Eilat/Aqaba, Red Sea, due to their known associations with boring organisms and distinct growth forms. These species include: Stylophora pistillata (branching) associated with Leiosolenus lessepsianus (formerly Lithophaga) (Mokady et al., 1991), Astreopora myriophthalma (massive) associated with Leiosolenus simplex (Mokady et al., 1998), Echinopora forskaliana (encrusting) associated with Leiosolenus spp (Goreau et al., 1970). Coral fragments were collected using recreational and technical diving in front of the Interuniversity Institute for Marine Sciences (IUI). A. myriophthalma and E. forskaliana colonies (n=1 fragment per species) were collected at 5m, while S. pistillata samples (n=12 fragments, 1 per colony) was collected at mesophotic depths (45m) due to the high prevalence of L. lessepsianus compared to shallow specimen (unpublished data). Additionally, fragments of S. pistillata were used to assess the suitability of preserved, complete, formalin-fixed samples for histological examination after X-ray scanning, compared to samples that were not scanned. These samples were fixed with 4% formaldehyde solution in seawater for 24h, rinsed in running tap water, and preserved in 70% ethanol, following Rapuano et al. (2017).
Our scans encompassed coral samples with both bare skeletons and tissues (i.e., preserved complete formalin-fixed samples, only for S. pistillata). Although extended exposure to X-rays during µCT scanning may theoretically induce a minor temperature increase within the sample, it is important to note that this heating effect is typically minimal and not a primary concern for most applications. Nevertheless, since our samples retained tissues intended for later histological analysis (not included in the scope of this study), we conducted a comparative histological examination between fixed samples that underwent µCT scanning with those that did not. This comparison aimed to assess the impact of µCT scanning on tissue integrity for subsequent histological analyses. As a precautionary measure, we immersed the sample in distilled water, as ethanol, being highly volatile, was previously present in the solution. The coral tissues were then sealed with parafilm to create an airtight enclosure, thereby maintaining tissue moisture.
Except for these fragments, all the samples were bleached in 6% sodium hypochlorite solution for 24h for tissue removal, followed by thorough rinsing with deionized running water to remove the remaining organic matter. Finally, they were left to air-dry at room temperature for an additional 24h.
2.2 µCT scanning and quantitative analysis
High-resolution micro-computed tomography (µCT) was performed using a Nikon XT H 225ST µCT (Nikon Metrology Inc., USA) at The Steinhart Museum of Natural History, Tel Aviv University. The coral samples were scanned in a 360° rotation (in 0.5° increments, scan time = 40 min) at an isotropic voxel size of 50μm, 0.25mm stainless steel filter, voltage of 65 kV, amperage of 123μA, and exposure time of 1.15s. The reconstructions into image stacks were conducted with VGSTUDIO MAX software (Volume Graphics © 2024) which were then saved in TIFF image format for 3D volume rendering and quantitative analysis using Dragonfly software (© 2023 Object Research System (ORS) Inc.). The Range tools were used to define threshold radiodensity values to create a new region of interest (ROI) for only the CaCO3 skeleton, in which all voxels within the selected range were labeled. A label analysis was conducted on the segmented dataset to determine the surface area and bulk volume of the coral samples. Other measurements were made manually: using the Ruler tool, the length and width of each bivalve were determined by scaling the two-dimensional (2D) axes along their longitudinal and lateral dimensions, and their boring cavity volume was estimated by distinguishing and outlining the boundaries between air and solid CaCO3, segmented using the ROI Painter in Multi-slice mode, and applying a Multi-ROI to label each burrow separately.
2.3 Skeleton decalcification for bivalve abundance
The skeletal samples were utilized to evaluate and compare the efficiency and accuracy of the µCT compared to the skeleton decalcification technique for the measurement and enumeration of internal bioeroders. Initially, µCT scans were conducted on these fragments, followed by quantitative measurements, as previously described. Then, to physically evaluate bivalve abundance per sample, decalcification was conducted using a sodium citrate buffered 25% formic acid solution prepared by combining equal volumes of 50% formic acid in distilled water and 20% sodium citrate in distilled water. This specific solution decalcifies the coral skeletons efficiently while minimizing potential damage to the bivalve shells. Despite both being made of calcium carbonate, bivalve shells differ in structure from coral skeletons. Specifically, Mytilidae bivalve shells consist of three distinct layers: the periostracum, the calcium carbonate-based prismatic, and the nacreous layers (Albano, 2021). The prismatic and nacreous layers are susceptible to dissolution in a 25% formic acid solution. However, the outer organic layer (the periostracum), mainly composed of conchiolin, a proteinaceous material, serves as a protective barrier that is comparatively more resistant to the decalcifying solution (Al-Hosney et al., 2005), thereby ensuring shell integrity. Following the completion of decalcification, bivalves were carefully extracted and counted.
3 Results and discussion
Modern 3D imaging techniques, such as computed tomography, are gaining popularity in the study of calcifying organisms as this tool facilitates ecologically relevant examinations of internal structural changes (Silbiger et al., 2016; Gutiérrez et al., 2018; Fordyce et al., 2020b; Kramer et al., 2023). However, coral reef research has not yet fully harnessed its potential to provide novel ecological insights. Here, we demonstrate that µCT serves as a powerful, precise, and non-destructive tool to study quantitative demographical and morphological aspects of bioeroders in their coral hosts, and provide spatial visualization of the of the internal bioeroder population within the host skeleton.
While prior studies share the use of volumetric µCT analysis for quantifying overall bioerosion patterns and processes in coral skeletons, including that of macro bioeroders (Silbiger et al., 2014, 2016, 2017; DeCarlo et al., 2015; Enochs et al., 2016, 2021; Newman et al., 2023), a key distinction lies in our focus on specific cryptic calcareous bivalves species rather than general bioerosion measures or broader taxonomical level. By targeting these major yet understudied bioeroders, we evaluate μCT’s ability to precisely quantify their abundance, size structure, spatial distribution, and the geometry of their individual boring cavities - aspects not explicitly examined in previous studies. Moreover, our direct comparative analysis between μCT and decalcification techniques highlights μCT’s advantages as a rapid, non-destructive method for assessing bioeroder population demographics and impacts on skeletal porosity. Overall, our work provides a complementary, fine-scale perspective on coral-bioeroder interactions, demonstrating μCT as a practical tool for advanced ecological investigations in this area.
µCT was advantageous for locating lithophagid bivalves and their boreholes (Figure 1). This group plays a significant role in internal bioerosion and contributes to the destruction of diverse calcareous substrates (Glynn and Manzello, 2015; Morton, 1983; Rice et al., 2019). Specifically, genera such as Leiosolenus and Lithophaga engage in boring activities within live and dead coral structures, respectively. These cryptic molluscs achieve peak abundances in subtidal reef zones, with certain species extending their bioerosive activities to the lower depth limits of reef-building corals (Glynn and Manzello, 2015). Our 3D analyses of coral specimens provide detailed insights into the impacts of boring bivalves on coral skeletons, enabling accurate assessments of bioerosion processes by locating the precise location, distribution, growth angle, and abundance of bioeroders within corals (Figures 1, 2), and to measure their body size with microscale precision (Figure 3. We were able to detect bivalves ranging in size from 0.5mm to 28mm, with varying sizes among the Leiosolenus species (Figure 1; Table 1). This size variation aligns with the skeletal polyp dimensions of the different coral morphologies examined. In contrast to small-polyp corals (<1.2mm in diameter for S. pistillata), large-polyp corals (5mm and 2.5mm in diameter for E. forskaliana and A. myriophthalma, respectively) are typically associated with larger skeletal features, thus providing more space for larger bivalves to settle and bore.
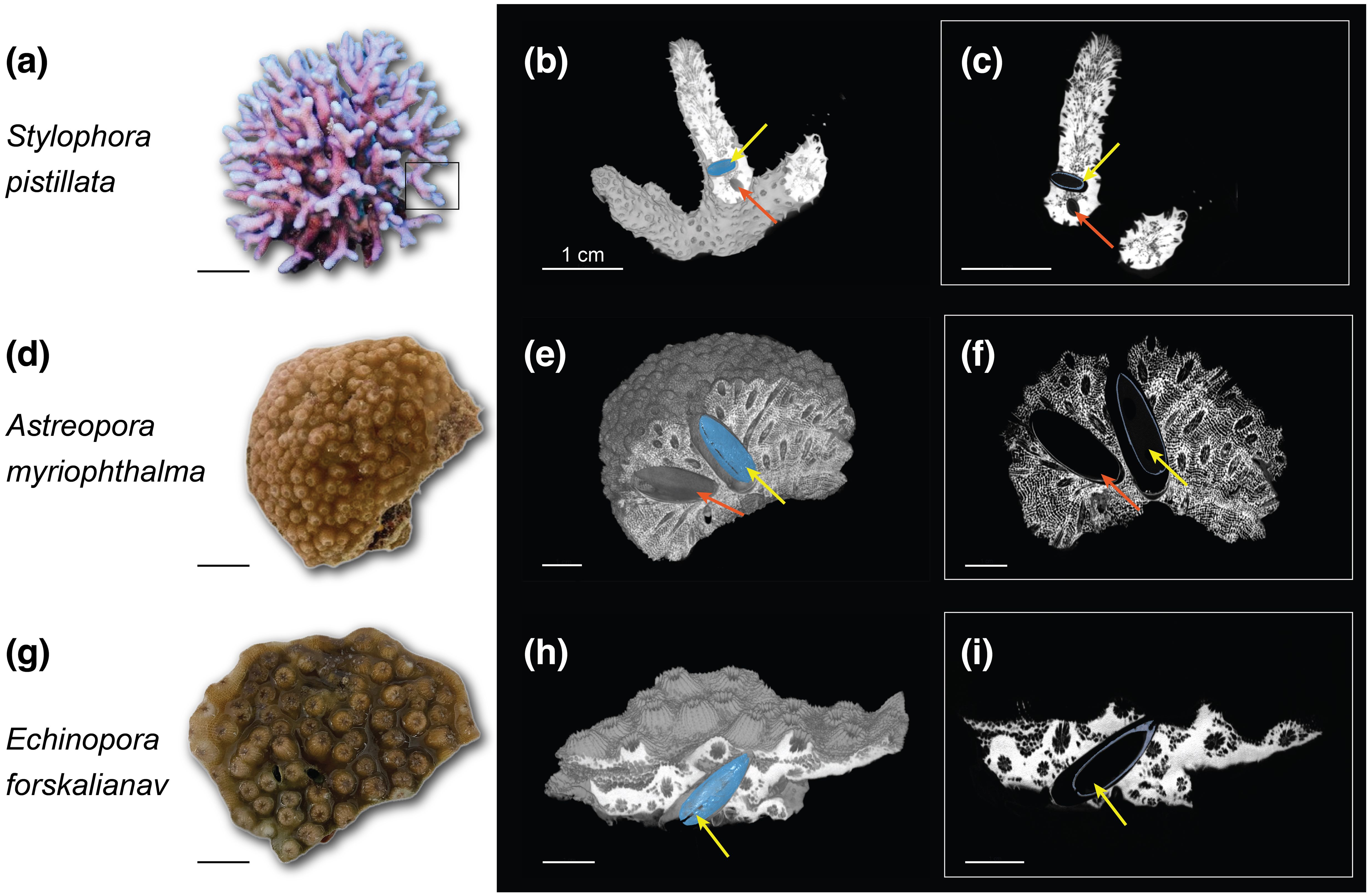
Figure 1. Three-dimensional rendering based on µCT data of three reef-building corals Stylophora pistillata, Astreopora myriophthalma, and Echinopora forskaliana. (A, D, G) Live corals; (B, E, H) 3D surface reconstructions of coral skeletons vertically clipped in the center of a bioeroder (segmented in blue) and (C, F, I) 2D reconstructions of the clipped area. Yellow arrows indicate segmented bioeroders and red arrows indicate non-segmented bioeroders. Scale bars = 1 cm.
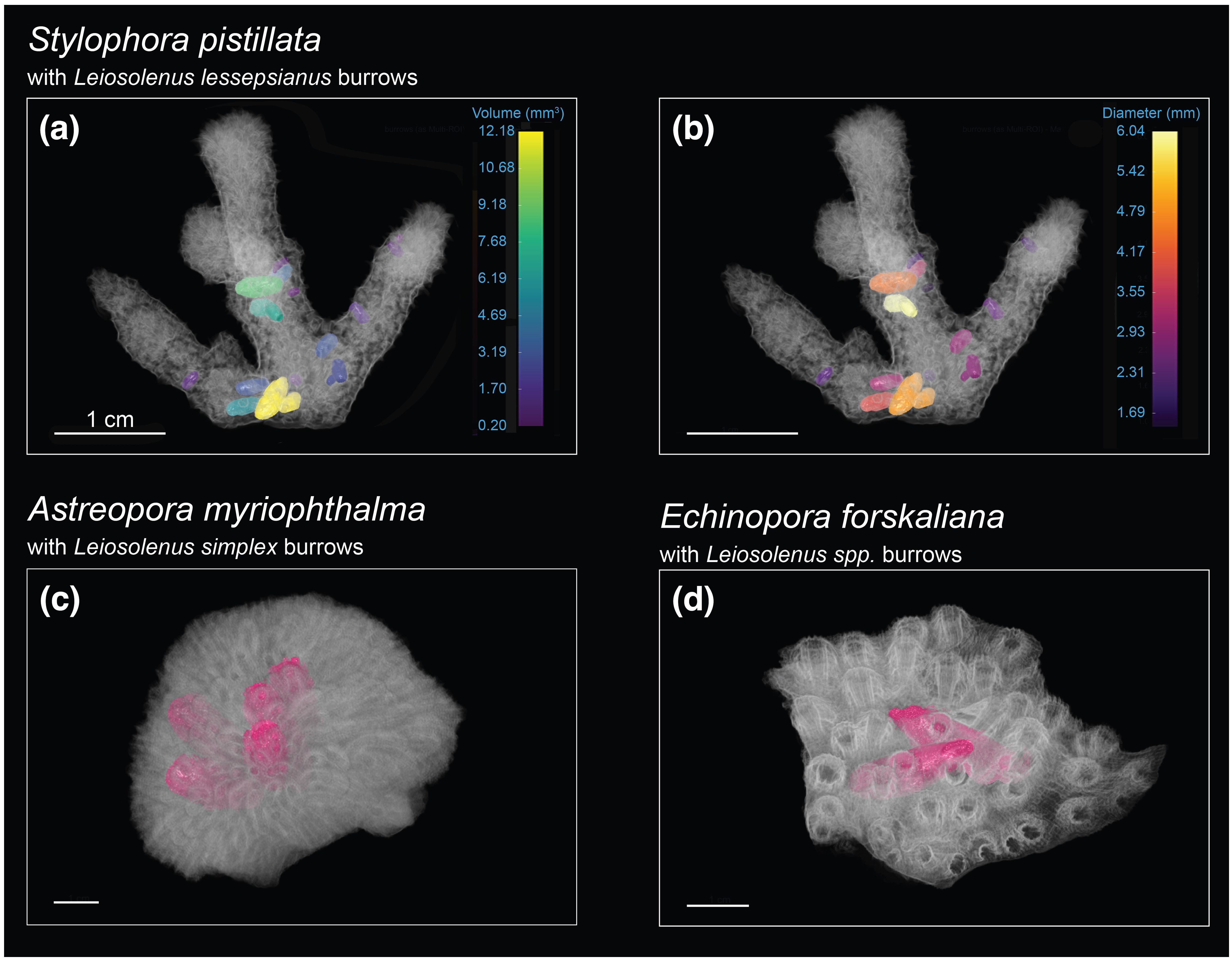
Figure 2. Volumetric µCT visualization of the location, distribution, and growth direction of active boring cavities in three coral hosts and their associated bivalves. S. pistillata borrows are colored based on (A) volume (mm3) and (B) maximum diameter (mm). (C, D) Borrows in other species are colored in pink. Scale bars = 1 cm.
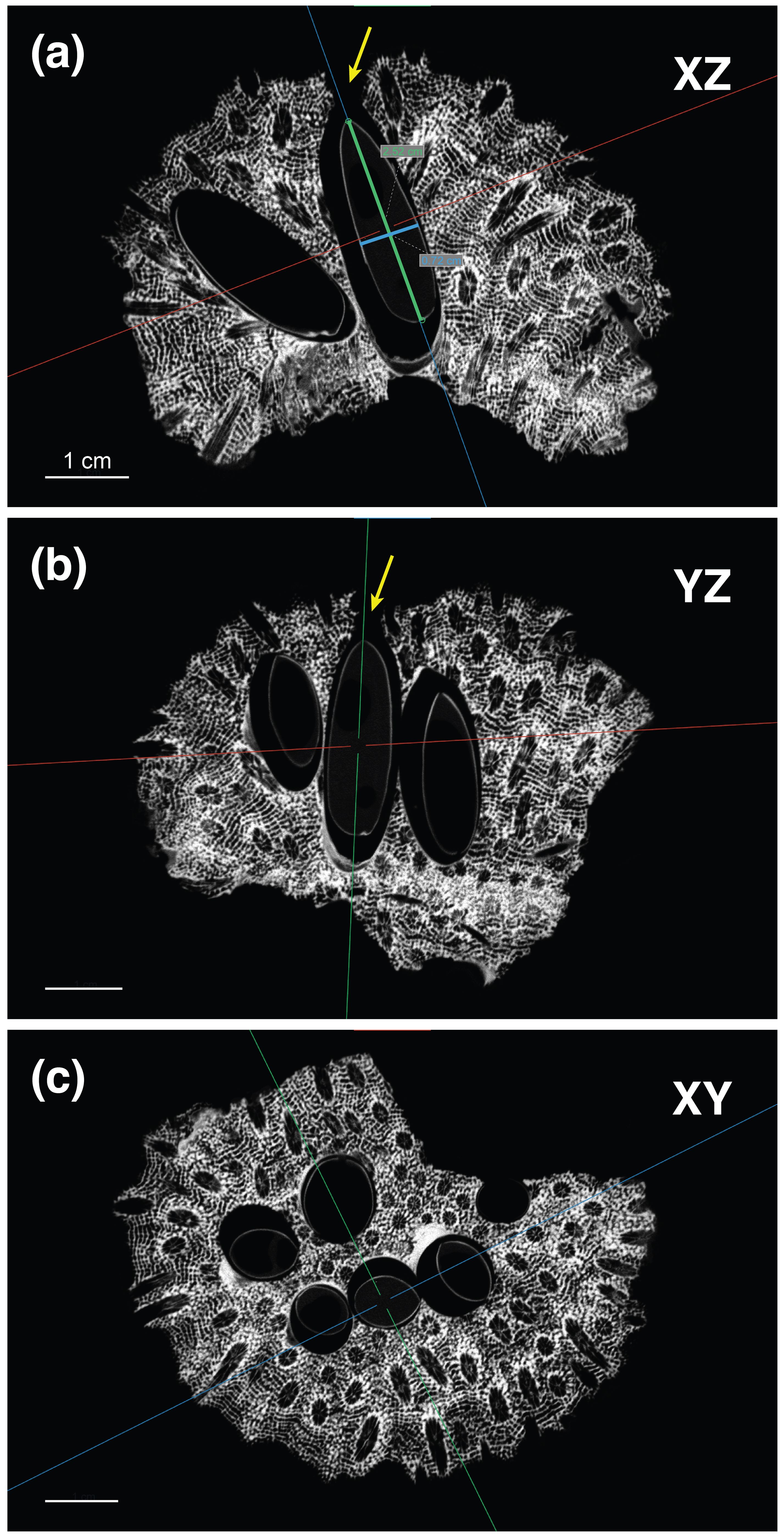
Figure 3. Example of 2D reconstructions of µCT data for Astreopora myriophthalma showing 3D cuts in the center of a bivalve. (A) The XZ (vertical), (B) YZ (vertical), and (C) XY (horizontal) planes were oriented along the given bivalve’s longitudinal axis to examine its dimensions [A: length (green line) and width (blue line)] and whether it has an opening (A, B: yellow arrows) indicating a live bivalve. Scale bars = 1 cm.
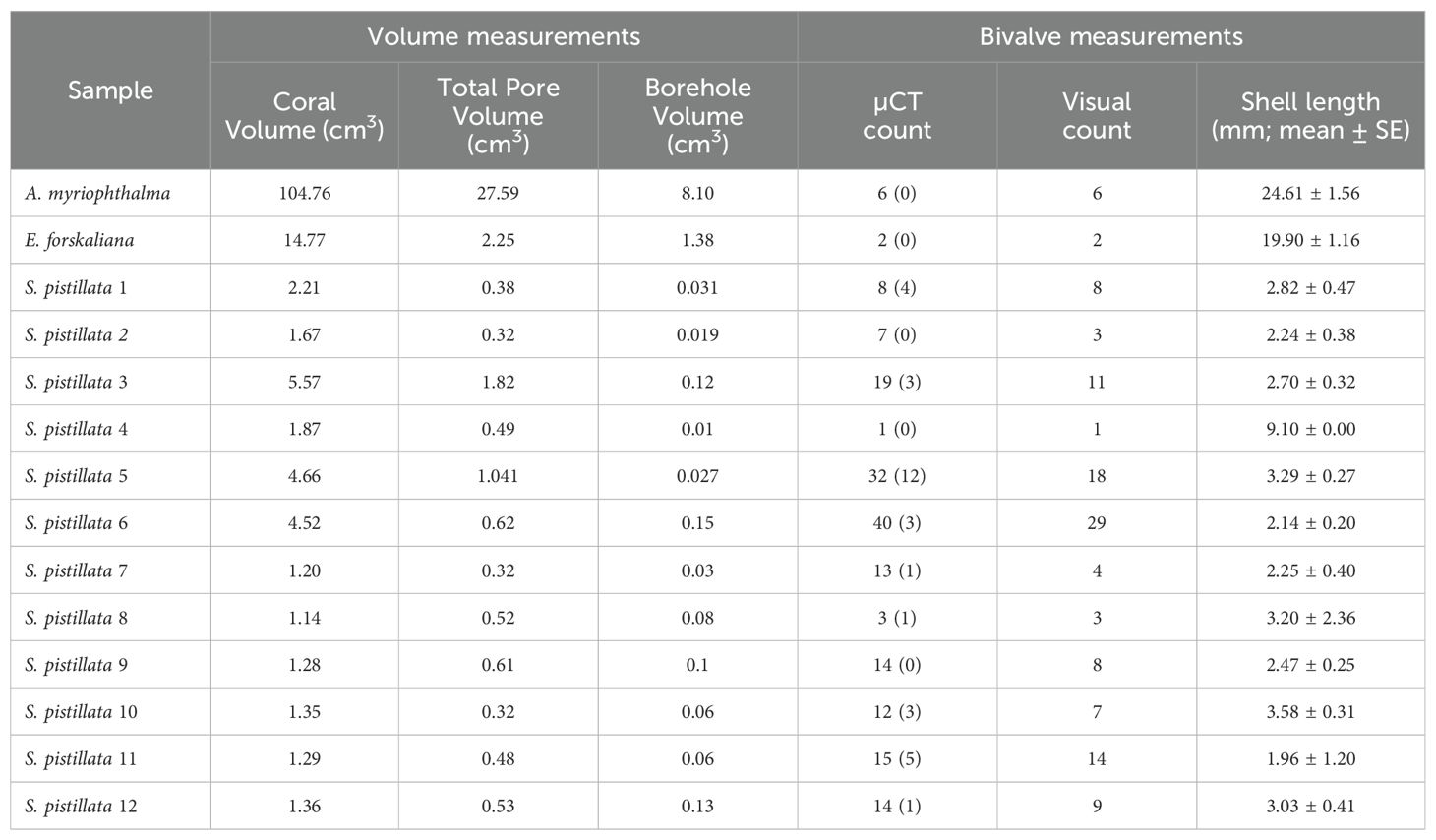
Table 1. Volume measurements of the three coral species, including their total void space (pores) within the skeleton and boreholes, and their bivalve measurements, including abundance estimations as detected by the µCT (blocked bivalves in parenthesis) and by visual inspection of decalcified samples, and their mean shell length (mean ± SE; in mm).
Assessing the body size of boring bivalve assemblagescould provide unprecedented information regarding their demographic characteristics, including recruitment events, reproductive maturity, and longevity (Dietzel et al., 2020; Kramer et al., 2020). However, reports of the size-frequency distribution of boring organisms, let alone of lithophagid bivalves, are lacking and non-existent in many species. Scott (1988) sought to assess the abundance of the internal bioeroder Leiosolenus bisulcatus within live and dead coral hosts. Coral host fragments were collected, and counts were made of both living and deceased Leiosolenus individuals. However, aside from the clear destructive and irreversible drawbacks associated with this method, it lacks the capacity to furnish details regarding burrow volume and its effect on coral porosity.
Bioerosion by bivalves is caused by the secretion of acid, which dissolves the substrate and allows them to create a burrow, typically occupying polyp spaces (Lazar and Loya, 1991). Utilizing µCT scans, we segmented and measured individual burrow volumes created by the bioeroders within the host skeleton (Figure 2). Measuring these volumes provides insights into the impact of internal bioerosion on skeletal integrity and porosity, which varies among coral species owing to factors such as morphological groups and environmental conditions (Foster et al., 2016; Hughes, 1987; Kramer et al., 2023). We showed that boring cavities in the encrusting E. forskaliana accounted for over 60% of the total porosity, boring cavities in the massive A. myriophthalma contributed nearly one-third of the coral porosity, and boring cavities in the branching S. pistillata reached up to 25% of the coral porosity (Table 1). Associating these findings with bivalve size and abundance suggests that the extent of internal bioerosion is influenced by bivalve size and coral morphology, namely, larger bivalve species create expansive boring cavities that contribute substantially to skeletal porosity.
Additionally, examining the internal coral skeleton in 3D provided flexibility in identifying bivalves that were “blocked” (Table 1) by the coral skeletal overgrowth, obstructing their burrowing progress towards the coral surface. Such information can easily be overlooked during field observations of live boring bivalves or when examining a predetermined 2D slice angle of the skeleton since these approaches offer inadequate estimation for macroborers (Silbiger et al., 2016). A comparison between µCT scan examination and bivalve counting after skeleton decalcification revealed that µCT scans yielded more accurate bivalve abundance estimates in 9 of 12 S. pistillata samples (Table 1). This superiority is attributed to the ability of µCT to capture very small bivalves (< 1 mm) effectively, which is especially beneficial in corals inhabited by mostly small-sized bioeroders, such as S. pistillata. In contrast, using the skeleton decalcification method proved insufficient as it can be prone to human error and oversight in identifying and locating bivalves hidden within the tissue, and there is a risk of losing very small bivalves during the process (Figure 4). Furthermore, skeletal decalcification is significantly more time-consuming than X-ray scanning, taking up to 24h (depending on skeletal density) compared to just 1h in µCT. However, while reducing processing time, µCT scanning may potentially increase costs. Therefore, to balance accuracy and affordability, it is important to clearly state the research objective. If µCT is required, it is necessary to predetermine the optimal scan parameters required to identify and measure the structures of interest. Lastly, we demonstrated the ability to scan corals with fixed tissues as a powerful tool for advancing comprehensive research, eliminating the need for additional sampling efforts. Our investigation revealed that X-ray tomography had no adverse effects on tissue integrity compared to samples that had not undergone scanning, ensuring the suitability of the samples for subsequent histological analysis. This finding carries significant implications, offering valuable opportunities for utilizing existing sample collections and museum specimens more effectively. Traditionally, formalin-fixed coral specimens have been primarily utilized to investigate cellular composition and other histological characteristics from the preserved tissues. However, we highlight that the non-destructive nature of μCT allows to visualize and analyze their internal calcareous structures without compromising on the integrity of the tissue, thereby allowing for subsequent analyses.
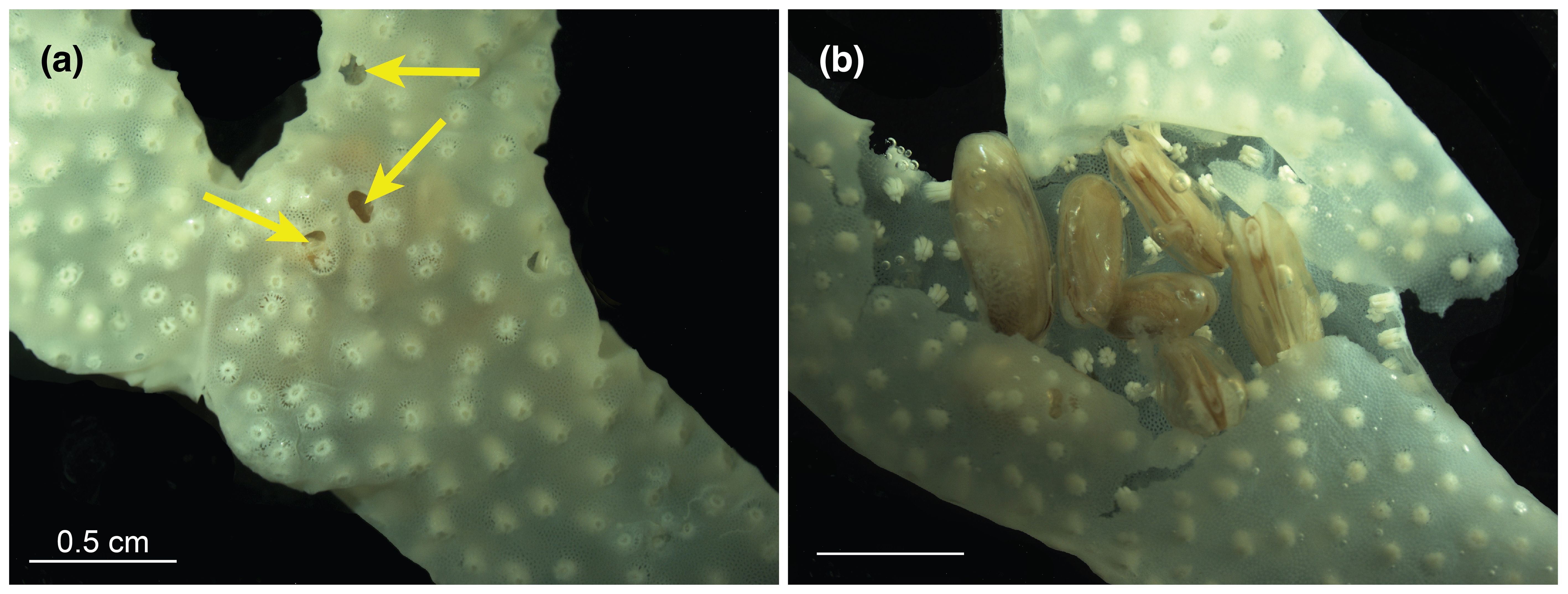
Figure 4. A decalcified S. pistillata sample (A) before and (B) after tissue examination. Scale bars = 0.5 cm. Arrows indicate burrow openings.
4 Conclusions
Our approach demonstrated that utilizing µCT for bioeroder analysis in corals surpasses the traditional 2D X-ray radiography technique, sectioning, and/or skeletal decalcification owing to its non-destructive nature, time efficiency, precision, and provision of high-resolution 3D reconstructions of internal and external coral-bivalve features. Our study highlights the advantages of µCT in quantifying bore-holes down to the sub-millimeter scale and offering high-resolution 3D visualization of their distribution within coral skeletons. Additionally, we demonstrated that with proper sample preparation, µCT can be used with fixed coral tissues, which is important for studying delicate structures or samples that need to be preserved.
As we navigate an era of climate change, investigating the impact of shifting oceanic conditions on marine calcifiers has become increasingly imperative (Fordyce et al., 2020b). Incorporating this powerful tool into our toolkit fills essential gaps in our understanding of the interaction between internal bioeroders and their coral hosts, which is necessary for unraveling the factors influencing coral health and resilience. Lastly, µCT generates digital image data that can be readily shared among researchers, fostering collaboration and advancing our collective understanding of coral ecosystems.
Data availability statement
The original contributions presented in the study are included in the article/supplementary material and figshare repository (DOI: https://doi.org/10.6084/m9.figshare.27046294.v1). Further inquiries can be directed to the corresponding author/s.
Ethics statement
The animal study was approved by The Israel Nature and Parks Authority, permit 2022/43141. The study was conducted in accordance with the local legislation and institutional requirements.
Author contributions
NK: Conceptualization, Formal analysis, Methodology, Software, Visualization, Writing – original draft, Writing – review & editing, Data curation. TA: Conceptualization, Data curation, Writing – review & editing. NG: Data curation, Writing – review & editing. MG: Data curation, Writing – review & editing. DW: Funding acquisition, Supervision, Writing – review & editing. YL: Conceptualization, Funding acquisition, Supervision, Writing – review & editing.
Funding
The author(s) declare that financial support was received for the research, authorship, and/or publication of this article. This work was supported in part by the joint United States National Science Foundation (NSF) and United States-Israel Binational Science Foundation (NSF-BSF) grant No. 2021647 to YL, and No. 2149925 to DW, and partially by the Israel Science Foundation (ISF) grant No. 640/21 to YL.
Acknowledgments
We are grateful to the Interuniversity Institute for Marine Sciences in Eilat (IUI) for providing the facilities. We thank The Steinhardt Museum of Natural History, Dan David Center for Human Evolution and Biohistory, for their technical assistance with μCT scanning.
Conflict of interest
The authors declare that the research was conducted in the absence of any commercial or financial relationships that could be construed as a potential conflict of interest.
Publisher’s note
All claims expressed in this article are solely those of the authors and do not necessarily represent those of their affiliated organizations, or those of the publisher, the editors and the reviewers. Any product that may be evaluated in this article, or claim that may be made by its manufacturer, is not guaranteed or endorsed by the publisher.
References
Albano P. G. (2021). Biology and evolution of the Mollusca W. F. PonderD. R. Lindbergh Boca Raton, FL: CRC Press, Taylor & Francis Group 2019–2020. Two volumes: xxiii-900 + xx-870 pp. ISBN: 9780815361695 (volume 1), 9780815361848 (volume 2). Hardcover: 190 £ + 190 £. Mar. Ecol. 42, e12645. doi: 10.1111/maec.12645
Al-Hosney H., Carlos-Cuellar S., Baltrusaitis J., Grassian V. (2005). Heterogeneous uptake and reactivity of formic acid on calcium carbonate particles: A Knudsen cell reactor, FTIR and SEM study. Phys. Chem. Chem. Phys. 7, 3587–3595. doi: 10.1039/b510112c
Connell J. H. (1978). Diversity in tropical rain forests and coral reefs. Sci. (1979). 199, 1302–1310. doi: 10.1126/science.199.4335.1302
Davidson T. M., Altieri A. H., Ruiz G. M., Torchin M. E. (2018). Bioerosion in a changing world: a conceptual framework. Ecol. Lett. 21, 422–438. doi: 10.1111/ele.12899
DeCarlo T. M., Cohen A. L., Barkley H. C., Cobban Q., Young C., Shamberger K. E., et al. (2015). Coral macrobioerosion is accelerated by ocean acidification and nutrients. Geology 43, 7–10. doi: 10.1130/G36147.1
Dietzel A., Bode M., Connolly S. R., Hughes T. P. (2020). Long-term shifts in the colony size structure of coral populations along the Great Barrier Reef. Proc. R. Soc. B.: Biol. Sci. 287. doi: 10.1098/rspb.2020.1432
Enochs I. C., Manzello D. P., Kolodziej G., Noonan S. H. C., Valentino L., Fabricius K. E. (2016). Enhanced macroboring and depressed calcification drive net dissolution at high-CO2 coral reefs. Proc. R. Soc. B.: Biol. Sci. 283. doi: 10.1098/rspb.2016.1742
Enochs I. C., Toth L. T., Kirkland A., Manzello D. P., Kolodziej G., Morris J. T., et al. (2021). Upwelling and the persistence of coral-reef frameworks in the eastern tropical Pacific. Ecol. Monogr. 91. doi: 10.1002/ecm.1482
Fordyce A. J., Ainsworth T. D., Leggat W. (2020a). Microalgae, a boring bivalve and a coral—A newly described association between two coral reef bioeroders within their coral host. Integr. Organismal. Biol. 2, obaa035. doi: 10.1093/iob/obaa035
Fordyce A. J., Ainsworth T. D., Leggat W., Katherine M. (2021). Light capture, skeletal morphology, and the biomass of corals’ Boring endoliths. mSphere 6, e00060–e00021. doi: 10.1128/mSphere.00060-21
Fordyce A. J., Knuefing L., Ainsworth T. D., Beeching L., Turner M., Leggat W. (2020b). Understanding decay in marine calcifiers: Micro-CT analysis of skeletal structures provides insight into the impacts of a changing climate in marine ecosystems. Methods Ecol. Evol. 11, 1021–1041. doi: 10.1111/2041-210X.13439
Foster T., Falter J. L., McCulloch M. T., Clode P. L. (2016). Ocean acidification causes structural deformities in juvenile coral skeletons. Sci. Adv. 2, 1–8. doi: 10.1126/sciadv.1501130
Ginsburg R. N. (1983). “Geological and biological roles of cavities in coral reefs,” in Perspectives on Coral Reefs. Ed. Barnes P. L. (Townsville, Australia: AIMS), 148–153.
Glynn P. W., Manzello D. P. (2015). “Bioerosion and coral reef growth: A dynamic balance,” in Coral Reefs in the Anthropocene. Ed. Birkeland C. (Springer Netherlands, Dordrecht), 67–97. doi: 10.1007/978-94-017-7249-5_4
Goreau T. F., Goreau N. I., Yonge C. M., Neumann Y. (1970). On feeding and nutrition in Fungiacava eilatensis (Bivalvia, Mytilidae), a commensal living in fungiid corals. J. Zool. 160, 159–172. doi: 10.1111/j.1469-7998.1970.tb02901.x
Gutiérrez Y., Ott D., Töpperwien M., Salditt T., Scherber C. (2018). X-ray computed tomography and its potential in ecological research: A review of studies and optimization of specimen preparation. Ecol. Evol. 8, 7717–7732. doi: 10.1002/ece3.4149
Hein F. J., Risk M. J. (1975). Bioerosion of Coral heads: inner patch reefs, Florida reef tract. Bull. Mar. Sci. 25, 133–138.
Highsmith R. C., Lueptow R., Schonberg S. C. (1983). Growth and bioerosion of three massive corals on the Belize barrier reef. Mar. Ecol. Prog. Ser. 13, 261–271. Available at: https://api.semanticscholar.org/CorpusID:4985036.
Hughes T. (1987). Skeletal density and growth form of corals. Mar. Ecol. Prog. Ser. 35, 259–266. doi: 10.3354/meps035259
Hutchings P. A. (1986). Biological destruction of coral reefs. Coral. Reefs. 4, 239–252. doi: 10.1007/BF00298083
Kramer N., Guan J., Chen S., Wangpraseurt D., Loya Y. (2022). Morpho-functional traits of the coral Stylophora pistillata enhance light capture for photosynthesis at mesophotic depths. Commun. Biol. 5, 861. doi: 10.1038/s42003-022-03829-4
Kramer N., Tamir R., Eyal G., Loya Y. (2020). Coral morphology portrays the spatial distribution and population size-structure along a 5–100 m depth gradient. Front. Mar. Sci. 7. doi: 10.3389/fmars.2020.00615
Kramer N., Tamir R., Galindo-Martínez C. T., Wangpraseurt D., Loya Y. (2023). Light pollution alters the skeletal morphology of coral juveniles and impairs their light capture capacity. Mar. pollut. Bull. 193, 115212. doi: 10.1016/j.marpolbul.2023.115212
Lazar B., Loya Y. (1991). Bioerosion of coral reefs-A chemical approach. Limnol. Oceanogr. 36. doi: 10.4319/lo.1991.36.2.0377
MacGeachy J. K., Stearn C. W. (1976). Boring by macro-organisms in the coral montastrea annularis on Barbados reefs. Int. Rev. Hydrobiol. 61, 715–745. doi: 10.1002/iroh.19760610602
Maher R. L., Johnston M. A., Brandt M. E., Smith T. B., Correa A. M. S. (2018). Depth and coral cover drive the distribution of a coral macroborer across two reef systems. PloS One 13, e0199462–. doi: 10.1371/journal.pone.0199462
Mokady O., Bonar D. B., Arazi G., Loya Y. (1991). Coral host specificity in settlement and metamorphosis of the date mussel Lithophaga lessepsiana (Vaillant 1865). J. Exp. Mar. Biol. Ecol. 146, 205–216. doi: 10.1016/0022-0981(91)90026-S
Mokady O., Loya Y., Lazar B. (1998). Ammonium contribution from boring bivalves to their coral host–a mutualistic symbiosis? Mar. Ecol. Prog. Ser. 169, 295–301. Available at: https://www.int-res.com/abstracts/meps/v169/p295-301/.
Morton B. (1983). “4 - Coral-Associated Bivalves of the Indo-Pacific,” in Ecology, ed. Rusell-Hunter W. D. (Academic Press), 139–224. doi: 10.1016/B978-0-12-751406-2.50011-8
Newman J. E. L., Perry C. T., Lange I. D. (2023). Quantifying endolithic bioerosion rates on remote coral reefs in the Central Indian Ocean. Coral. Reefs. 42, 1163–1173. Available at: https://api.semanticscholar.org/CorpusID:261672425.
Perry C. T., Harborne A. R. (2016). “Bioerosion on modern reefs: impacts and responses under changing ecological and environmental conditions,” in Coral Reefs at the Crossroads. Eds. Hubbard D. K., Rogers C. S., Lipps J. H., Stanley George D XXXJ.R.X.X.X. (Springer Netherlands, Dordrecht), 69–101. doi: 10.1007/978-94-017-7567-0_4
Rapuano H., Brickner I., Shlesinger T., Meroz-Fine E., Tamir R., Loya Y. (2017). Reproductive strategies of the coral Turbinaria reniformis in the northern Gulf of Aqaba (Red Sea). Sci. Rep. 7, 42670. doi: 10.1038/srep42670
Rice M. M., Ezzat L., Burkepile D. E. (2019). Corallivory in the anthropocene: Interactive effects of anthropogenic stressors and corallivory on coral reefs. Front. Mar. Sci. 5. doi: 10.3389/fmars.2018.00525
Rice M. M., Maher R. L., Correa A. M. S., Moeller H. V., Lemoine N. P., Shantz A. A., et al. (2020). Macroborer presence on corals increases with nutrient input and promotes parrotfish bioerosion. Coral. Reefs. 39, 409–418. doi: 10.1007/s00338-020-01904-y
Risk M. J., Sammarco P. W. (1991). Cross-shelf trends in skeletal density of the massive coral Porites lobata from the Great Barrier Reef. Mar. Ecol. Prog. Ser. 69, 195–200. doi: 10.3354/meps069195
Risk M. J., Sammarco P. W., Edinger E. N. (1995). Bioerosion in Acropora across the continental shelf of the Great Barrier Reef. Coral. Reefs. 14, 79–86. doi: 10.1007/BF00303427
Roff G., Joseph J., Mumby P. J. (2019). Multi-decadal changes in structural complexity following mass coral mortality on a Caribbean reef. Biogeosciences. 17, 5909–5918. doi: 10.5194/bg-17-5909-2020
Schönberg C. H. L., Fang J. K.-H., Carreiro-Silva M., Tribollet A., Wisshak M. (2017). Bioerosion: the other ocean acidification problem. ICES. J. Mar. Sci. 74, 895–925. Available at: https://api.semanticscholar.org/CorpusID:202695053.
Scott P. J. B. (1988). Distribution, habitat and morphology of the Caribbean coral- and rock-boring bivalve, Lithophaga bisulcata (d’Orbigny) (Mytilidae: Lithophaginae). J. Molluscan. Stud. 54, 83–95. doi: 10.1093/mollus/54.1.83
Silbiger N. J., Donahue M. J., Brain R. E. (2017). Environmental drivers of coral reef carbonate production and bioerosion: A multi-scale analysis. Ecology 98, 2547–2560. doi: 10.1002/ecy.1946
Silbiger N. J., Guadayol Ò., Thomas F. I. M., Donahue M. J. (2014). Reefs shift from net accretion to net erosion along a natural environmental gradient. Mar. Ecol. Prog. Ser. 515, 33–44. doi: 10.3354/meps10999
Silbiger N. J., Guadayol O., Thomas F. O. M., Donahue M. J. (2016). A novel μct analysis reveals different responses of bioerosion and secondary accretion to environmental variability. PloS One 11 (14), e0153058. doi: 10.1371/journal.pone.0153058
Silbiger N. J., Nelson C. E., Remple K., Sevilla J. K., Quinlan Z. A., Putnam H. M., et al. (2018). Nutrient pollution disrupts key ecosystem functions on coral reefs. Proc. R. Soc. B.: Biol. Sci. 285, 20172718. doi: 10.1098/rspb.2017.2718
Keywords: coral reefs, bioerosion, micro-computed tomography, 3D imaging, symbiosis
Citation: Kramer N, Amit T, Gavrieli N, Gross M, Wangpraseurt D and Loya Y (2024) Quantifying attributes of boring bivalve populations in corals using micro-computed tomography. Front. Mar. Sci. 11:1407537. doi: 10.3389/fmars.2024.1407537
Received: 26 March 2024; Accepted: 09 September 2024;
Published: 24 September 2024.
Edited by:
Christian Robert Voolstra, University of Konstanz, GermanyReviewed by:
Raquel Peixoto, King Abdullah University of Science and Technology, Saudi ArabiaEdgardo Londono-Cruz, University of the Valley, Colombia
Copyright © 2024 Kramer, Amit, Gavrieli, Gross, Wangpraseurt and Loya. This is an open-access article distributed under the terms of the Creative Commons Attribution License (CC BY). The use, distribution or reproduction in other forums is permitted, provided the original author(s) and the copyright owner(s) are credited and that the original publication in this journal is cited, in accordance with accepted academic practice. No use, distribution or reproduction is permitted which does not comply with these terms.
*Correspondence: Netanel Kramer, bmF0aS5rcmFtZXJAZ21haWwuY29t
†ORCID: Netanel Kramer, orcid.org/0000-0001-5150-0732
Daniel Wangpraseurt, orcid.org/0000-0003-4834-8981
Yossi Loya, orcid.org/0000-0001-6870-9444